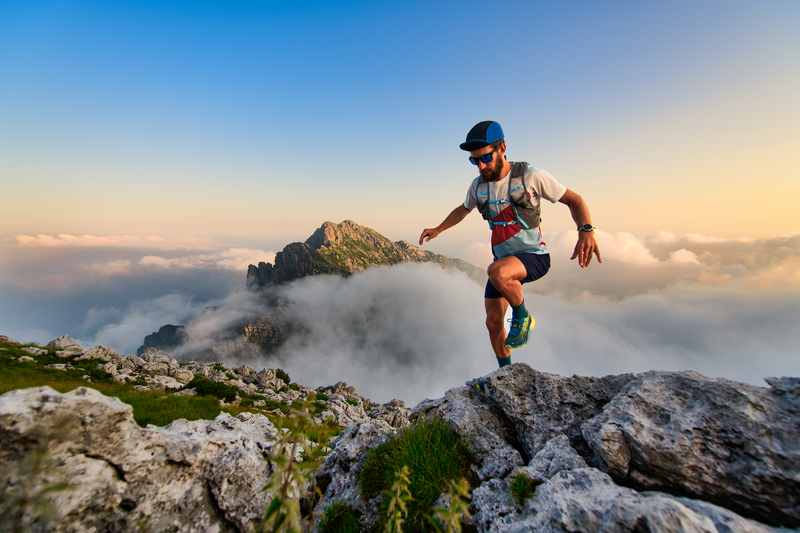
95% of researchers rate our articles as excellent or good
Learn more about the work of our research integrity team to safeguard the quality of each article we publish.
Find out more
REVIEW article
Front. Physiol. , 06 September 2023
Sec. Cell Physiology
Volume 14 - 2023 | https://doi.org/10.3389/fphys.2023.1233073
Intermedin (IMD) is a member of the calcitonin gene-related peptide (CGRP)/calcitonin (CT) superfamily, and it is expressed extensively throughout the body. The typical receptors for IMD are complexes composed of calcitonin receptor-like receptor (CLR) and receptor activity-modifying protein (RAMP), which leads to a biased activation towards Gαs. As a diagnostic and prognostic biomarker, IMD regulates the initiation and metastasis of multiple tumors. Additionally, IMD functions as a proangiogenic factor that can restrain excessive vascular budding and facilitate the expansion of blood vessel lumen, ultimately resulting in the fusion of blood vessels. IMD has protective roles in various diseases, including ischemia-reperfusion injury, metabolic disease, cardiovascular diseases and inflammatory diseases. This review systematically elucidates IMD’s expression, structure, related receptors and signal pathway, as well as its comprehensive functions in the context of acute kidney injury, obesity, diabetes, heart failure and sepsis. However, the precise formation process of IMD short peptides in vivo and their downstream signaling pathway have not been fully elucidated yet. Further in-depth studies are need to translate IMD research into clinical applications.
In 2004, Roh and Takei first discovered intermedin (IMD), which is secreted and highly expressed in the intermediate lobe of the pituitary gland (Roh et al., 2004; Takei et al., 2004; Takahashi et al., 2006). IMD belongs to the calcitonin gene-related peptide (CGRP) superfamily, which includes members such as CGRP, calcitonin (CT), amylin (AMY), and adrenomedullin (ADM) (Chang et al., 2004; Bell et al., 2016). Mammalian IMD shares a sequence similar to fish ADM2, prompting some researchers to refer to it as ADM2.
IMD is expressed in various tissues, such as the brain, kidney, liver, lung, submandibular gland, pancreas, esophagus, gastrointestinal tract, and adipose tissue (Figure 1) (Ogoshi et al., 2003; Ni et al., 2014). Also, IMD is expressed in multiple cell types, including cardiac fibroblasts, cardiomyocytes, cells of the cardiac microvasculature, arterial endothelial cells, renal mesangial cells, renal tubular epithelial cells, and others (Smith et al., 2009; Bell et al., 2012). However, its expression in plasma is relatively low, with levels ranging from 100 to 200 pg/mL (Hay et al., 2011; García-Ponce et al., 2016). Further studies are warranted to evaluate the underlying mechanisms of IMD peptides in the plasma and the enzymes responsible for their degradation.
The expression of IMD is regulated by an estrogen response element (ERE), a hypoxia response element (HRE), and an integrated stress response element (ISR) (García-Ponce et al., 2016). The expression of IMD increases in human aortic endothelial cells under various stress conditions, such as oxidative stress and serum starvation (García-Ponce et al., 2016). Studies have also found that in iodine-deficient rats, the expression of IMD was increased in thyroid follicular cells (Roh et al., 2004; Ni et al., 2014; Alexander et al., 2015).
Given its wide distribution, IMD may play critical biological roles. IMD mainly functions in an autocrine and paracrine manner, regulating hormone secretion and perfusion in major tissues and organs (Bell and McDermott, 2008). IMD is involved in regulating both prolactin secretion and estrogen-induced prolactin release (Roh et al., 2004). Additionally, IMD can regulate blood pressure in pregnant women (Alexander et al., 2015).
In this review, we systematically summarize the expression, molecular structure, receptors, signaling pathway of IMD, and its function in the context of the cardiovascular disease, metabolic syndrome, tumor blood vessel angiogenesis and remodeling, and inflammatory diseases. Our findings suggest that IMD may have broad clinical applications, but there are still some questions that need to be addressed in the future.
The human IMD gene is located on chromosome 22q13.33 and it encodes a pre-pro hormone called pre-pro-IMD, which consists of 148 amino acids and a signal peptide for secretion at its N-terminus (Chang et al., 2004). Bell et al. discovered that in vivo, the complete pre-pro-hormone is hydrolyzed at three specific sites: Arg100-Thr101, Arg107-Val108, and Tyr147-Gly148. Notably, the site Tyr147-Gly148 undergoes amidation (Bell et al., 2016). The hydrolysis of the pre-pro-hormone produces three biologically active C-terminal peptides: IMD1–40, IMD1–47, and IMD1–53, along with several prerequisite fragments, such as IMD25–56 and IMD57–92 (Figure 2) (Ni et al., 2014).
FIGURE 2. A schematic drawing illustrating the structure of the human IMD prepropeptide. The pre-pro-IMD molecule is cleaved at its C-terminal to yield three mature IMD peptides: IMD1–53, IMD1–47 (together referred to as IMD long), and IMD1–40 (known as IMD short). The precursor fragment, pre-pro-IMD25–92, is biologically inactive and undergoes cleavage at Arg56-Pro57, resulting in the formation of two fragments: propeptide-IMD25–56 and propeptide-IMD57–92.
As confirmed by sequence comparison, IMD is highly conserved across different species. The amino acid sequence of human IMD1–47 is 87% identical to that of rats and 60% similar to that of teleost fish (Ogoshi et al., 2003). While, compared to ADM of the CGRP family, only 28% of the sequences are identical. The properties of highly conserved to orthologs with low homology to paralogs indicate that IMD plays a unique role in the body (Ni et al., 2014; García-Ponce et al., 2016).
Studies have revealed that IMD and its related peptides exhibit unique synergistic and antagonistic effects on regulation of gene expression, biological processes, receptor binding, signal transduction, and the occurrence and development of diseases. Nevertheless, the precise formation process of these short peptides and their relative content in vivo have not been fully elucidated yet.
IMD is a secretory peptide that is relatively short in length (Bell and McDermott, 2008; García-Ponce, Chánez Paredes et al., 2016). The typical receptors for peptides of CGRP family are complexes composed of calcitonin receptor-like receptor (CLR) and receptor activity-modifying protein (RAMP) (Roh et al., 2004). CLR belongs to the class B G protein-coupled receptor (GPCR) subunit, which enables IMD and other members of CGRP family to interact with cells and carry out their functions (Alexander et al., 2015). RAMPs function as molecular chaperones, facilitating the translocation of CLR from the endoplasmic reticulum and Golgi bodies to the cell surface (Weston et al., 2015). They enhance the cell surface expression of CLR and play a critical role in the pharmacology of CLR (Hay and Pioszak, 2016).
There are three types of RAMPs: RAMP1, RAMP2, and RAMP3. CLR interacts with different RAMPs, leading to varying ligand selectivity. RAMPs could alter CLR ligand selectivity through allosteric effects and direct peptide contacts (Booe et al., 2018). CLR heterodimerizes with RAMP1 to form the CGRP receptor, whereas CLR heterodimerizes with RAMP2 or RAMP3 to form the AM1 and AM2 receptors, respectively (Bell et al., 2016). The peptide ligands activate each receptor with differing potencies. CGRP has the highest affinity for the CGRP receptor, while ADM has the highest affinity for the AM1 and AM2 receptors. CGRP has also been observed to bind to AM2 receptor complexes, but with an affinity that is 50-fold lower than that of ADM(Hay et al., 2011). The human ADM was also able to stimulate cyclic adenosine monophosphate (cAMP) production via the CGRP receptor, but its affinity is approximately 10 times lower than that of CGRP (Bailey and Hay, 2006).
IMD is capable of binding to all CGRP, AM1 and AM2 receptors (Zhang et al., 2016). Primarily, IMD signals via CGRP or AM2 receptors, and to a lesser extent, also via AM1 receptors (Figure 3) (García-Ponce et al., 2016; Roehrkasse et al., 2018). Although the AM2 receptor has the highest affinity for IMD, the majority of IMD’s cardiovascular effects are mediated by CGRP receptors and AM1 receptors, probably due to their high expression in the heart and blood vessels (Bell and McDermott, 2008). Furthermore, IMD may function through AMY receptors, which consist of the calcitonin receptor (CTR) and RAMPs (Owji et al., 2008). Since IMD interacts with multiple receptors, several regulatory mechanisms could be involved in mediating its biological effects. Therefore, designing specific inhibitors that target IMD based on its receptors is of great importance for its specific clinical applications (Zhang et al., 2018).
FIGURE 3. A diagram illustrates how IMD activates various downstream signaling pathways through different CLR/RAMP receptor complexes. CLR, calcitonin receptor-like receptor; RAMP, receptor activity-modifying protein.
The CLR-based receptors are known for having complex downstream signaling pathways. The interaction of CLR with different G proteins initiates various signaling pathways (Alexander et al., 2015). RAMPs are involved in modifying the G protein selectivity activated by CLR-based receptors. For example, when the IMD1-47 polypeptide is added to HEK-293 cells, the CGRP and AM2 receptors lead to a biased activation toward Gαs signaling, while the AM1 receptors show a bias toward Gαq signaling (Figure 3) (Weston et al., 2016). Besides, CGRP, ADM and IMD may activate receptors via different signaling pathways depending on the specific peptide involved. For example, the AM2 receptors show a bias toward Gαs signaling in response to ADM and change to Gαq signaling in response to CGRP. Inversely, the CGRP receptors displays a bias toward Gαs signaling when activated by CGRP, but changed to Gαi signaling when activated by ADM (Weston et al., 2016).
It is likely that the main transduction pathway for CLR-based receptors activated by IMD involves coupling to Gs, activating adenylyl cyclase (AC), and resulting in intracellular accumulation of cAMP. The accumulation of cAMP can activate protein kinase A (PKA), making the AC-cAMP-PKA pathway the most important intracellular signaling pathway of IMD. This pathway is involved in the attenuation of vascular calcification (Chen et al., 2020), alleviation of cardiac fibrosis (Zhang et al., 2022), inhibition of atherosclerosis (Dai et al., 2012), reduction of blood pressure, and improvement of cardiac function during hypertension (Yuan et al., 2012), among other effects. IMD can also increase the activity of nitric oxide synthase (NOS) and endothelial NOS (eNOS), leading to elevated nitric oxide (NO) content and enhanced L-arginine uptake in tissues (Chen et al., 2006). This mechanism is involved in the attenuation of hypoxic pulmonary vascular remodeling (Mao et al., 2014). Furthermore, NO can activate guanylate cyclase (GC) and elevate intracellular cGMP levels. Therefore, the L-arginine-NO-cGMP signal pathway also plays a significant role in the biological function of IMD.
Recently, IMD has been reported to mediate various biological functions through multiple pathways (Figure 3). These include the PI3K/Akt and AMPK pathways, which are involved in attenuating aging-associated vascular calcification (Chen et al., 2020). Additionally, IMD has been shown to activate the ERK pathway, promoting cell migration and tube formation in cultured endothelial cells (Smith et al., 2009). In hepatocellular carcinoma, IMD is involved in cell invasion through the ERK1/2-EGR1/DDIT3 signaling pathway (Xiao et al., 2021). Moreover, IMD contributes to decreased atrial fibrosis in rats after myocardial infarction operation through the TGF-β1/Smad3 signaling pathway (Ma et al., 2023). In diabetic conditions, IMD promotes bone generation via the PPARγ/NF-κB signaling pathway (Wang et al., 2021). Furthermore, IMD participates in alleviating pathological cardiac remodeling through the PPARγ-klotho pathway (Zhang et al., 2020).
However, the receptor affinity and activation of downstream signaling pathways remain unclear. In addition, there is a lack of antagonists and analogs that can be utilized to further differentiate the specific pathways of different receptors (Ni et al., 2014). Thus, the development of analogs or specific inhibitors of IMD could be crucial in comprehending the biological functions and molecular mechanisms of IMD.
The calcitonin gene-related peptide (CGRP) is widely distributed and performs critical biological functions. Calcitonin, CGRP, and amylin are essential regulators of cardiovascular diseases, kidney diseases, sepsis, inflammatory responses, and diabetes (Bell and McDermott, 2008). In a physiological state, the primary role of amylin is to regulate glucose metabolism, while calcitonin regulates calcium metabolism (Wong et al., 2014; Junfeng et al., 2016). CGRP and ADM share similar biological effects, including the regulation of cardiovascular homeostasis and vasodilation (Naot and Cornish, 2008). CGRP is also a neuropeptide that plays a major role in the sensory nervous system (Brain and Grant, 2004).
Members of ADM family are all found in fish (Takei et al., 2004). In mammals, only three peptides from ADM family, ADM, ADM2 (IMD), and ADM5 have been identified (Takei et al., 2008). ADM contains 52 amino acids and plays an important role in human tissues and organs in a paracrine manner (Hay et al., 2011). ADM primarily functions through the AM1 and AM2 receptors (Poyner et al., 2002). ADM is significantly expressed in endothelial cells and may contribute to promoting cardiovascular relaxation (García et al., 2006). ADM is also expressed in various tissues and exerts vasodilatory effects on blood vessels. Voors et al. found that the expression of ADM significantly increased during the progression of chronic heart failure (Voors et al., 2019). The short intermediate peptide pro-AMD45–92, generated during ADM hydrolysis, has demonstrated the ability to predict the progression of heart failure in clinical settings (Yuyun et al., 2015).
ADM is also extensively expressed in various types of tumors, and its expression significantly increases in response to hypoxia-inducible factor-1α (HIF-1α) under hypoxic conditions (Albertin et al., 2005). Importantly, high expression of ADM promotes the formation of blood vessels and lymphatic vessels within tumors, which contributes to tumor initiation and progression. ADM has also been implicated in many endocrine-related tumorigenesis, such as renal cell carcinoma (Deville et al., 2009), granulation cell tumor (Deville et al., 2009), hepatocellular carcinoma (Qu et al., 2015), pancreatic cancer cell (Keleg et al., 2007), invasive squamous cell carcinoma (Li et al., 2001), and neuroendocrine tumors (Li et al., 2001).
Recent studies have revealed a strong correlation between IMD and the development of tumors, as well as the prognosis of patients with tumors (Hirose et al., 2008; Ni et al., 2014). In 2008, Morimoto et al. reported for the first time that IMD expression was increased in adrenal tumors (Morimoto et al., 2008). In 2012, Guo et al. discovered that the expression of IMD is relatively low in normal hepatocytes, but it gradually increases with the degree of malignancy in hepatocellular carcinoma (HCC). Subsequent studies have shown that IMD was primarily expressed in malignant hepatic carcinoma cells and in the vicinity of tumor blood vessels, which promotes the proliferation of HCC (Guo et al., 2012). Our team has recently shown that IMD could facilitate metastasis and invasion of HCC through ERK1/2-EGR1/DDIT3 signaling cascade (Xiao et al., 2021). Lu et al. reported that the level of IMD in the peripheral blood of breast cancer patients was significantly higher than that of normal controls. Furthermore, patients who had higher IMD expression before surgery exhibited lower 5-year survival rate and overall survival rate, as well as a higher recurrence rate (Lu et al., 2015). Kong et al. also found that IMD expression was significantly increased in breast cancer samples and facilitated its metastasis by increasing ribosome biogenesis and protein translation via the Src/c-Myc signaling pathway (Kong et al., 2022). Hollander et al. showed that patients with pancreatic adenocarcinoma who had high IMD expression exhibited a shorter median survival time and poorer 5-year survival compared to those with low IMD expression (Hollander et al., 2015).
These studies suggest that IMD and its related peptides are involved in the onset and progression of multiple tumors, potentially playing important roles in cancer cell survival and invasion. Further research is needed to confirm the potential mechanism of IMD and its related peptides in tumorigenesis and development.
The process of angiogenesis is necessary to provide a suitable vascular network for tumor growth and metastasis. The primary characteristics of tumor vessels include an unstable vascular endothelial barrier, increased permeability, and dysregulation of vascular expansion, which facilitate tumor invasion. Thus, controlling tumor-associated angiogenesis is an effective approach to limit tumor progression (Fiedler and Augustin, 2006; Carmeliet and Jain, 2011). The process of blood vessel formation can be divided into two stages: vasculogenesis and angiogenesis (Morin and Tranquillo, 2013; Ibrahim and Richardson, 2017; Vallée et al., 2018). The former refers to the process of establishing a fundamental vascular network from scratch. The latter involves the growth of new blood vessels from the existing primary network, leading to the formation of a functional vascular network (Ribatti et al., 2015).
Emerging evidence demonstrates that IMD, a novel proangiogenic factor, plays an essential role in regulating angiogenesis (Smith et al., 2009). Zhang et al. identified that IMD could promote vascular remodeling and tumor angiogenesis in a tumor vascular model (Zhang et al., 2012). The exogenous IMD1–47 peptide was found to enhance blood supply and promote tumor growth (Zhang et al., 2012; Wang et al., 2018). IMD1–53 might regulate vessel function homeostasis via upregulating the L-Arg/NOS/NO pathway (Yang et al., 2006). Moreover, studies have demonstrated that IMD promotes endothelial migration and tube formation by increasing the phosphorylation of ERK, AKT, as well as the synthesis of vascular endothelial growth factor (VEGF) and VEGF receptor-2 (VEGFR-2) (Smith et al., 2009). Additionally, IMD could directly activate the phosphorylation of VEGFR-2 through CGRP receptors or AM1 receptors, providing an additional mechanism for pro-angiogenesis (Cai et al., 2010).
Regular expression of VEGF results in consistent and stable blood vessel development, whereas overexpression of VEGF leads to an excessive budding of vessels and the formation of irregular blood vessels. Xiao et al. discovered that IMD acted as a proangiogenic factor that can inhibit excessive vascular budding induced by overexpressed VEGF (Xiao et al., 2015). In addition, IMD has the ability to regulate the expression of vascular endothelial-cadherin (VEC) and prevent vascular budding caused by increased endothelial space (Zhang et al., 2012; Xiao et al., 2015). Furthermore, IMD could promote vessel fusion by inducing endothelial cells to enter a “ready-to-anchor” state and regulating VEC activity to achieve a dynamic balance between VEC complex dissociation and reconstitution (Kong et al., 2020).
The expansion of blood vessel lumen is a result of the restored proliferative ability of quiescent endothelial cells, rather than any morphological changes in the endothelial cells themselves. Studies have found that IMD could regulate the expression of CLR receptor complex and β-arrestin1/Src, facilitating their translocation into the cytoplasm to activate the downstream ERK1/2 signaling pathway (Figure 4). This activation could promote the maturation of tumor blood vessels and enhance blood perfusion (Wang et al., 2018).
FIGURE 4. The mechanism and process of IMD promote vascular growth in tumors. IMD regulates the expression of the CLR receptor complex and β-arrestin1/Src, facilitating their translocation into the cytoplasm to activate the downstream ERK1/2 signaling pathway. This activation promotes the expansion of the blood vessel lumen and enhances the maturation of tumor blood vessels.
These mechanisms demonstrate that IMD may play a crucial role in promoting angiogenesis and the fusion of tumor blood vessels, ultimately leading to the establishment of a relatively suitable and functional vascular network for tumor growth.
As a potential vascular regulator, IMD plays an important role in regulating systemic hemodynamics and electrolyte balance, as well as protecting against ischemic injury and oxidative stress. IMD is widely expressed in human hypothalamus-pituitary-adrenal axis, as well as in the heart and kidney, suggesting its role in regulating circulation and water-electrolyte metabolism (Takahashi et al., 2006; Takahashi et al., 2011). Administration of IMD into the lateral cerebral ventricle of rats caused significant and long-lasting elevations in mean arterial pressure and heart rate, indicating that IMD had the ability to activate the sympathetic nervous system (Taylor et al., 2005). Evidence suggests that IMD may promote vasodilation, increase renal perfusion, and exhibit direct diuretic effects. Intravenous administration of IMD1–47 peptides results in increased blood flow and decreased vascular resistance in various organs, including the heart, lungs, kidneys, liver, stomach, small intestine, and testis (Fujisawa et al., 2007).
Ischemia-reperfusion injury (IRI) is a significant cause of acute kidney injury (AKI) and increases the risk of developing chronic kidney disease (CKD) subsequently. IMD upregulated the mRNA and protein expression levels of VEGF and VEGFR2, while downregulating the expression levels of MMP2, MMP9 and ET-1. Thus, IMD could protect the kidney from ischemia-reperfusion injury by facilitating angiogenesis and reducing the destruction of the perivascular matrix (Wang et al., 2020). Emerging evidence suggests that the protective effects of IMD may be attributed to the regulation of mitochondrial function. Chen et al. found that IMD could mitigate the alterations in mitochondrial membrane induced by oxidative stress in brain endothelial cells (Chen et al., 2006).
Cardiovascular disease is a leading cause of morbidity and mortality, accounting for 20%–30% of all deaths in the population (Aggarwal et al., 2017; Leong et al., 2017). IMD has a protective effect on the cardiovascular system and cardiometabolic diseases. Much data has demonstrated that changes in the expression levels of IMD and its receptors can affect the development and progression of cardiovascular diseases (Hirose et al., 2008; Yuyun, et al., 2015; Bell et al., 2016). IMD may play both direct and indirect roles in maintaining cardiovascular homeostasis and improving cardiac function. For instance, IMD can directly inhibits myocardial fibrosis by downregulating TGF-β. Additionally, it indirectly regulates metabolic syndrome and reduces the risk factors associated with such diseases (Zhang et al., 2016).
Vascular calcification is considered an independent predictor of cardiovascular morbidity and mortality. IMD is a cardiovascular protective peptide that can suppress vascular calcification in rats by upregulating γ-carboxyglutamic acid (Gla) protein (Cai et al., 2010). IMD infusion significantly reduced atherosclerotic lesion areas and the number of calcified nodules in aortic roots in homocysteine-treated ApoE−/− mice. Mechanistically, IMD alleviated endoplasmic reticulum stress (ERS) activation and decreased the protein levels of ERS markers in vascular smooth muscle cells (VSMCs) and mouse peritoneal macrophages (Ren et al., 2020). Exogenous administration of IMD1–53 significantly inhibited the calcium deposition in aortas and the osteogenic trans-differentiation of vascular smooth muscle cells (VSMCs) in vitamin D3 plus nicotine (VDN)-treated old rats. Mechanistically, IMD1–53 attenuated aging-associated vascular calcification by upregulating sirt1 via activating PI3K/Akt, AMPK, and cAMP/PKA signaling (Chen et al., 2020). These suggested that IMD is a potential drug target for preventing and treating cardiovascular diseases.
These studies have demonstrated that IMD plays a crucial role in maintaining cardiovascular homeostasis and alleviating vascular diseases. As a result, it has emerged as a potential drug target for the treatment of atherosclerosis, vascular calcification, and hypertension (Ni et al., 2014; Zhang et al., 2018). For instance, the use of recombinant peptide or adeno-associated virus (AAV)-mediated IMD has been investigated as a potential method to mitigate cardiovascular disease (Zhang et al., 2018). However, the clinical application of IMD is still limited due to factors such as unclear mechanisms and insufficient experimental research.
Since the progression of heart failure is irreversible, early diagnosis and treatment are crucial as they make it easier to prevent the disease from worsening and improve the survival rate and quality of life of patients (Coiro et al., 2017; Pei et al., 2018). Studies have suggested that the expression level of IMD could serve as a prognostic indicator for heart failure (Zhang et al., 2022). In a rat model of congestive heart failure induced by left coronary artery ligation, Hirose et al. discovered a significant increase in the expression levels of IMD, CLR, and RAMPs (RAMP1/RAMP2/RAMP3) in cardiac tissues (Hirose et al., 2008). Cabiati et al. found that the expression of IMD significantly increased in the peripheral blood of patients with chronic heart failure (Cabiati et al., 2014). Short peptides derived from pre-pro-IMD25–56 and pre-pro-IMD57-92 have been detected in serum of human with heart failure (Bell et al., 2016; Arrigo et al., 2017).
Patients with acute heart failure who exhibited high IMD expression in peripheral blood showed stronger myocardial function compared to those with low IMD expression (Gan et al., 2014). Furthermore, in a rat model of angiotensin II (Ang II)-induced cardiac fibrosis, IMD was shown to alleviate cardiac fibrosis by suppressing NLRP3 inflammasome activation and endoplasmic reticulum stress. And this was achieved through inhibition of IRE1α via the cAMP/PKA pathway (Zhang et al., 2022). In rats after myocardial infarction operation, IMD improved atrial fibrosis and reduced the inducibility of atrial fibrillation through the TGF-β1/Smad3 and TGF-β1/Nox4 pathways (Ma et al., 2023). Nevertheless, in clinical practice, it is difficult to accurately determine IMD levels in serum due to its short half-life, the existence of binding proteins and the technical difficulties (Cabiati et al., 2014).
Cardiac remodeling is characterized by cardiac hypertrophy, fibrosis, dysfunction, and eventually progresses to heart failure. Cardiac remodeling was attenuated in mice overexpressing IMD. IMD markedly suppressed the phosphorylation of Ca2+/calmodulin-dependent protein kinase II (CaMKII) and the activity of calcineurin to protect against cardiac hypertrophy. This effect was achieved by upregulating klotho both in vivo and in vitro (Zhang et al., 2020).
Some studies have demonstrated a tight association between the expression of IMD and type 2 diabetes mellitus (T2DM). There was also a correlation between plasma IMD levels and body mass index (BMI), diastolic blood pressure (DBP), triglycerides, uric acid, blood urea nitrogen, fasting and 2-h postprandial blood glucose, and glycated hemoglobin (HbA1C) (Liu et al., 2022).
IMD is expressed in adipose tissue and is downregulated in diet-induced obese mice and in db/db mice (Zhang et al., 2016). Zhang et al. found that IMD mainly inhibited metabolic syndrome by targeting the adipose tissue (Zhang et al., 2016). An inverse correlation was observed between plasma levels of IMD and BMI. Further studies have revealed that IMD treatment restores the early insulin resistance induced by high-fat diet in adipose tissue, primarily through the inhibition of adipocyte MHCII antigen presentation and CD4+ T-cell activation (Zhang et al., 2016; Wang et al., 2021). Systemic administration of synthesized IMD reduced high-fat diet-induced body weight gain, as well as systemic insulin resistance, through activation of the AMP-activated protein kinase (AMPK) pathway and an increase of uncoupling protein 1 (UCP1) expression in adipose tissue (Zhang et al., 2016).
Additionally, IMD could alleviate inflammation in adipose tissue by regulating the number of macrophages present in the tissue (Soultanova et al., 2016). IMD treatment reversed advanced glycation end products-mediated M1 macrophage polarization and impaired bone regeneration in type 1 diabetes mellitus (T1DM), which was partially achieved by the peroxisome proliferator-activated receptor γ (PPARγ)-mediated inhibition of NF-κB signaling (Wang et al., 2021). IMD1–53 primarily induces the activation of M2-type macrophages and reverses the elevated M1/M2 macrophage ratio by inhibiting AMPK activity. This mechanism contributes to the reduction of inflammation in adipose tissue (Pang et al., 2016). Furthermore, during obesity, adipocytes mainly express MHC-II and function as antigen-presenting cells, initiating adaptive immune responses. Since macrophages express receptors for IMD, it could downregulate MHC-II expression in adipocytes through a cAMP-BlimP1-dependent manner (Deng et al., 2013). Based on a mouse model of atherosclerosis in diabetes, IMD attenuates macrophage phagocytosis through regulation of the long noncoding RNA Dnm3os/miR-27b-3p/SLAMF7 signaling pathway (Su et al., 2021).
Sepsis is a systemic inflammatory response syndrome and the most common critical illness in the intensive care unit (ICU), with the highest mortality rate (Angus and van der Poll, 2013; Minasyan, 2019). Despite recent advances in sepsis treatment, the mortality rate still ranges from 30% to 60% (Laroye et al., 2017; Peterzan et al., 2017). Zhang et al. conducted a retrospective analysis of clinical data from elderly patients with sepsis and found significant increases in serum levels of IMD, C-reactive protein (CRP), and procalcitonin (PCT) (Zhang et al., 2017). These findings suggest that serum IMD may serve as an indicator of the severity and prognosis of sepsis in elderly patients.
Clinical findings indicate that IMD has a protective effect in the development of sepsis and can serve as a specific marker for clinical diagnosis (Xiao et al., 2018). Vascular leakage and cytokine storms are two typical pathological characteristics of sepsis (Aird, 2003; Deutschman and Tracey, 2014; Armstrong et al., 2017). Xiao et al. discovered that IMD could provide protection against organ damage caused by vascular leakage and inflammatory response. Subsequent studies have shown that IMD could reconstruct the endothelial barrier by regulating Rab11 to promote VEC transport, which can repair the endothelial space and alleviate vascular leakage (Xiao et al., 2018).
Sepsis causes myocardial damage in approximately 40% of patients (Hato et al., 2019). Myocardial dysfunction is responsible for the death of approximately 70%–90% of sepsis patients (Hoover et al., 2015). Studies have shown that IMD can relax blood vessels, reduce blood pressure, and provide protection against myocardial ischemia (Dai et al., 2012; Mao et al., 2014). Zhu et al. discovered, in a rat model of sepsis induced by CLP, that administering exogenous IMD could increase oxygen transport, improve tissue perfusion, and enhance myocardial contractility and function (Zhu et al., 2016). Moreover, the administration of IMD peptides prior to surgery has been shown to significantly improve survival rates (Zhu et al., 2016). Interleukin-1β (IL-1β) is an inflammatory factor that plays a crucial role in the development of tissue and organ damage during sepsis. Studies have shown that IMD could reduce the expression of major inflammatory factors in sepsis by regulating the NLRP3/Caspase-1/IL-1β pathway, thereby exerting a protective effect against sepsis-induced cardiac dysfunction (Johnson et al., 2018).
Taken together, the expression level of IMD is closely associated with the development of sepsis. Thus, the levels of IMD or pre-pro-IMD peptide in plasma can serve as diagnostic markers or potential drug targets for septic syndromes. To translate the research on IMD into clinical applications, it is necessary to further elucidate the role of IMD in sepsis.
Altogether, the crucial role of IMD in regulating vascular development, cardiovascular disease, cancer, and inflammatory diseases is fundamental for its application in clinical practice (Figure 5). In-depth studies are needed to explore the signal transduction pathways of IMD and the specific roles of different active segments of IMD in regulating gene expression, biological effect, receptor binding, receptor signal transduction, as well as the genesis and development of diseases.
FIGURE 5. An overview of the multiple physiological functions of IMD, including improving cardiovascular diseases, alleviating atherosclerosis, regulating hemodynamics, improving obesity and metabolic syndrome, controlling systemic inflammation, promoting angiogenesis and tumor progression.
ZY, PW, QL, HL, and CY wrote the manuscript. WL, DW and CY revised the manuscript. All authors contributed to the article and approved the submitted version.
The National Natural Science Foundation of P.R. China (82102301). The Natural Science Foundation of Sichuan Province (2021YJ0418). Figures in the article were created with BioRender.com.
The authors declare that the research was conducted in the absence of any commercial or financial relationships that could be construed as a potential conflict of interest.
All claims expressed in this article are solely those of the authors and do not necessarily represent those of their affiliated organizations, or those of the publisher, the editors and the reviewers. Any product that may be evaluated in this article, or claim that may be made by its manufacturer, is not guaranteed or endorsed by the publisher.
Aggarwal, M., Aggarwal, B., and Rao, J. (2017). Integrative medicine for cardiovascular disease and prevention. Med. Clin. North Am. 101 (5), 895–923. doi:10.1016/j.mcna.2017.04.007
Aird, W. C. (2003). The role of the endothelium in severe sepsis and multiple organ dysfunction syndrome. Blood 101 (10), 3765–3777. doi:10.1182/blood-2002-06-1887
Albertin, G., Carraro, G., Petrelli, L., Guidolin, D., Neri, G., and Nussdorfer, G. G. (2005). Endothelin-1 and adrenomedullin enhance the growth of human adrenocortical carcinoma-derived SW-13 cell line by stimulating proliferation and inhibiting apoptosis. Int. J. Mol. Med. 15 (3), 469–474. doi:10.3892/ijmm.15.3.469
Alexander, S. P., Davenport, A. P., Kelly, E., Marrion, N., Peters, J. A., Benson, H. E., et al. (2015). The concise guide to PHARMACOLOGY 2015/16: g protein-coupled receptors. Br. J. Pharmacol. 172 (24), 5744–5869. doi:10.1111/bph.13348
Angus, D. C., and van der Poll, T. (2013). Severe sepsis and septic shock. N. Engl. J. Med. 369 (9), 2063–2851. doi:10.1056/NEJMc1312359
Armstrong, B. A., Betzold, R. D., and May, A. K. (2017). Sepsis and septic shock strategies. Surg. Clin. North Am. 97 (6), 1339–1379. doi:10.1016/j.suc.2017.07.003
Arrigo, M., Truong, Q. A., Szymonifka, J., Rivas-Lasarte, M., Tolppanen, H., Sadoune, M., et al. (2017). Mid-regional pro-atrial natriuretic peptide to predict clinical course in heart failure patients undergoing cardiac resynchronization therapy. Europace 19 (11), 1848–1854. doi:10.1093/europace/euw305
Bailey, R. J., and Hay, D. L. (2006). Pharmacology of the human CGRP1 receptor in cos 7 cells. Peptides 27 (6), 1367–1375. doi:10.1016/j.peptides.2005.11.014
Bell, D., Campbell, M., Ferguson, M., Sayers, L., Donaghy, L., O'Regan, A., et al. (2012). AM₁-receptor-dependent protection by intermedin of human vascular and cardiac non-vascular cells from ischaemia-reperfusion injury. J. Physiol. 590 (5), 1181–1197. doi:10.1113/jphysiol.2011.221895
Bell, D., Campbell, M., McAleer, S. F., Ferguson, M., Donaghy, L., and Harbinson, M. T. (2016). Endothelium-derived intermedin/adrenomedullin-2 protects human ventricular cardiomyocytes from ischaemia-reoxygenation injury predominantly via the AM- receptor. Peptides 76, 1–13. doi:10.1016/j.peptides.2015.12.005
Bell, D., Gordon, B. J., Lavery, A., Megaw, K., Kinney, M. O., and Harbinson, M. T. (2016). Plasma levels of intermedin (adrenomedullin-2) in healthy human volunteers and patients with heart failure. Peptides 76, 19–29. doi:10.1016/j.peptides.2015.12.003
Bell, D., and McDermott, B. J. (2008). Intermedin (adrenomedullin-2): a novel counter-regulatory peptide in the cardiovascular and renal systems. Br. J. Pharmacol. 153, S247–S262. doi:10.1038/sj.bjp.0707494
Booe, J. M., Warner, M. L., Roehrkasse, A. M., Hay, D. L., and Pioszak, A. A. (2018). Probing the mechanism of receptor activity-modifying protein modulation of GPCR ligand selectivity through rational design of potent adrenomedullin and calcitonin gene-related peptide antagonists. Mol. Pharmacol. 93 (4), 355–367. doi:10.1124/mol.117.110916
Brain, S. D., and Grant, A. D. (2004). Vascular actions of calcitonin gene-related peptide and adrenomedullin. Physiol. Rev. 84 (3), 903–934. doi:10.1152/physrev.00037.2003
Cabiati, M., Sabatino, L., Svezia, B., Caruso, R., Verde, A., Caselli, C., et al. (2014). Adrenomedullin and intermedin gene transcription is increased in leukocytes of patients with chronic heart failure at different stages of the disease. Peptides 55, 13–16. doi:10.1016/j.peptides.2014.01.028
Cai, Y., Xu, M. J., Teng, X., Zhou, Y. B., Chen, L., Zhu, Y., et al. (2010). Intermedin inhibits vascular calcification by increasing the level of matrix gamma-carboxyglutamic acid protein. Cardiovasc Res. 85 (4), 864–873. doi:10.1093/cvr/cvp366
Carmeliet, P., and Jain, R. K. (2011). Molecular mechanisms and clinical applications of angiogenesis. Nature 473 (7347), 298–307. doi:10.1038/nature10144
Chang, C. L., Roh, J., and Hsu, S. Y. (2004). Intermedin, a novel calcitonin family peptide that exists in teleosts as well as in mammals: a comparison with other calcitonin/intermedin family peptides in vertebrates. Peptides 25 (10), 1633–1642. doi:10.1016/j.peptides.2004.05.021
Chen, L., Kis, B., Hashimoto, H., Busija, D. W., Takei, Y., Yamashita, H., et al. (2006). Adrenomedullin 2 protects rat cerebral endothelial cells from oxidative damage in vitro. Brain Res. 1086 (1), 42–49. doi:10.1016/j.brainres.2006.02.128
Chen, Y., Zhang, L. S., Ren, J. L., Zhang, Y. R., Wu, N., Jia, M. Z., et al. (2020). Intermedin(1-53) attenuates aging-associated vascular calcification in rats by upregulating sirtuin 1. Aging (Albany NY) 12 (7), 5651–5674. doi:10.18632/aging.102934
Coiro, S., Girerd, N., Rossignol, P., Ferreira, J. P., Maggioni, A., Pitt, B., et al. (2017). Association of beta-blocker treatment with mortality following myocardial infarction in patients with chronic obstructive pulmonary disease and heart failure or left ventricular dysfunction: a propensity matched-cohort analysis from the high-risk myocardial infarction database initiative. Eur. J. Heart Fail 19 (2), 271–279. doi:10.1002/ejhf.647
Dai, X. Y., Cai, Y., Mao, D. D., Qi, Y. F., Tang, C., Xu, Q., et al. (2012). Increased stability of phosphatase and tensin homolog by intermedin leading to scavenger receptor A inhibition of macrophages reduces atherosclerosis in apolipoprotein E-deficient mice. J. Mol. Cell Cardiol. 53 (4), 509–520. doi:10.1016/j.yjmcc.2012.07.006
Deng, T., Lyon, C. J., Minze, L. J., Lin, J., Zou, J., Liu, J. Z., et al. (2013). Class II major histocompatibility complex plays an essential role in obesity-induced adipose inflammation. Cell Metab. 17 (3), 411–422. doi:10.1016/j.cmet.2013.02.009
Deutschman, C. S., and Tracey, K. J. (2014). Sepsis: current dogma and new perspectives. Immunity 40 (4), 463–475. doi:10.1016/j.immuni.2014.04.001
Deville, J. L., Bartoli, C., Berenguer, C., Fernandez-Sauze, S., Kaafarani, I., Delfino, C., et al. (2009). Expression and role of adrenomedullin in renal tumors and value of its mRNA levels as prognostic factor in clear-cell renal carcinoma. Int. J. Cancer 125 (10), 2307–2315. doi:10.1002/ijc.24568
Fiedler, U., and Augustin, H. G. (2006). Angiopoietins: a link between angiogenesis and inflammation. Trends Immunol. 27 (12), 552–558. doi:10.1016/j.it.2006.10.004
Fujisawa, Y., Nagai, Y., Miyatake, A., Miura, K., Nishiyama, A., Kimura, S., et al. (2007). Effects of adrenomedullin 2 on regional hemodynamics in conscious rats. Eur. J. Pharmacol. 558 (1-3), 128–132. doi:10.1016/j.ejphar.2006.11.043
Gan, X. B., Sun, H. J., Chen, D., Zhang, L. L., Zhou, H., Chen, L. Y., et al. (2014). Intermedin in the paraventricular nucleus attenuates cardiac sympathetic afferent reflex in chronic heart failure rats. PLoS One 9 (4), e94234. doi:10.1371/journal.pone.0094234
García, M. A., Martín-Santamaría, S., de Pascual-Teresa, B., Ramos, A., Julián, M., and Martínez, A. (2006). Adrenomedullin: a new and promising target for drug discovery. Expert Opin. Ther. Targets 10 (2), 303–317. doi:10.1517/14728222.10.2.303
García-Ponce, A., Chánez Paredes, S., Castro Ochoa, K. F., and Schnoor, M. (2016). Regulation of endothelial and epithelial barrier functions by peptide hormones of the adrenomedullin family. Tissue Barriers 4 (4), e1228439. doi:10.1080/21688370.2016.1228439
Guo, X., Schmitz, J. C., Kenney, B. C., Uchio, E. M., Kulkarni, S., and Cha, C. H. (2012). Intermedin is overexpressed in hepatocellular carcinoma and regulates cell proliferation and survival. Cancer Sci. 103 (8), 1474–1480. doi:10.1111/j.1349-7006.2012.02341.x
Hato, T., Maier, B., Syed, F., Myslinski, J., Zollman, A., Plotkin, Z., et al. (2019). Bacterial sepsis triggers an antiviral response that causes translation shutdown. J. Clin. Invest. 129 (1), 296–309. doi:10.1172/JCI123284
Hay, D. L., and Pioszak, A. A. (2016). Receptor activity-modifying proteins (RAMPs): new insights and roles. Annu. Rev. Pharmacol. Toxicol. 56, 469–487. doi:10.1146/annurev-pharmtox-010715-103120
Hay, D. L., Walker, C. S., and Poyner, D. R. (2011). Adrenomedullin and calcitonin gene-related peptide receptors in endocrine-related cancers: opportunities and challenges. Endocr. Relat. Cancer 18 (1), C1–C14. doi:10.1677/ERC-10-0244
Hirose, T., Totsune, K., Mori, N., Morimoto, R., Hashimoto, M., Nakashige, Y., et al. (2008). Increased expression of adrenomedullin 2/intermedin in rat hearts with congestive heart failure. Eur. J. Heart Fail 10 (9), 840–849. doi:10.1016/j.ejheart.2008.06.020
Hollander, L. L., Guo, X., Salem, R. R., and Cha, C. H. (2015). The novel tumor angiogenic factor, adrenomedullin-2, predicts survival in pancreatic adenocarcinoma. J. Surg. Res. 197 (2), 219–224. doi:10.1016/j.jss.2014.11.002
Hoover, D. B., Ozment, T. R., Wondergem, R., Li, C., and Williams, D. L. (2015). Impaired heart rate regulation and depression of cardiac chronotropic and dromotropic function in polymicrobial sepsis. Shock 43 (2), 185–191. doi:10.1097/SHK.0000000000000272
Ibrahim, M., and Richardson, M. K. (2017). Beyond organoids: in vitro vasculogenesis and angiogenesis using cells from mammals and zebrafish. Reprod. Toxicol. 73, 292–311. doi:10.1016/j.reprotox.2017.07.002
Johnson, M. O., Wolf, M. M., Madden, M. Z., Andrejeva, G., Sugiura, A., Contreras, D. C., et al. (2018). Distinct regulation of Th17 and Th1 cell differentiation by glutaminase-dependent metabolism. Cell 175 (7), 1780–1795. doi:10.1016/j.cell.2018.10.001
Junfeng, G., Huiyu, Z., Gang, Z., Yang, A., Yang, Y., Fei, W., et al. (2016). Protective effect of calcitonin gene-related peptide against oxidative damage in MC3T3-E1 osteoblasts. Hua Xi Kou Qiang Yi Xue Za Zhi 34 (6), 584–588. doi:10.7518/hxkq.2016.06.007
Keleg, S., Kayed, H., Jiang, X., Penzel, R., Giese, T., Büchler, M. W., et al. (2007). Adrenomedullin is induced by hypoxia and enhances pancreatic cancer cell invasion. Int. J. Cancer 121 (1), 21–32. doi:10.1002/ijc.22596
Kong, L., Xiao, F., Wang, L., Li, M., Wang, D., Feng, Z., et al. (2020). Intermedin promotes vessel fusion by inducing VE-cadherin accumulation at potential fusion sites and to achieve a dynamic balance between VE-cadherin-complex dissociation/reconstitution. MedComm 1(1), 84–102. doi:10.1002/mco2.9
Kong, L., Xiong, Y., Wang, D., Huang, L., Li, M., Feng, Z., et al. (2022). Intermedin (adrenomedullin 2) promotes breast cancer metastasis via Src/c-Myc-mediated ribosome production and protein translation. Breast Cancer Res. Treat. 195 (2), 91–103. doi:10.1007/s10549-022-06687-0
Laroye, C., Gibot, S., Reppel, L., and Bensoussan, D. (2017). Concise review: mesenchymal stromal/stem cells: a new treatment for sepsis and septic shock? Stem Cells 35 (12), 2331–2339. doi:10.1002/stem.2695
Leong, D. P., Joseph, P. G., McKee, M., Anand, S. S., Teo, K. K., Schwalm, J. D., et al. (2017). Reducing the global burden of cardiovascular disease, Part 2: prevention and treatment of cardiovascular disease. Circ. Res. 121 (6), 695–710. doi:10.1161/CIRCRESAHA.117.311849
Li, Z., Takeuchi, S., Otani, T., and Maruo, T. (2001). Implications of adrenomedullin expression in the invasion of squamous cell carcinoma of the uterine cervix. Int. J. Clin. Oncol. 6 (6), 263–270. doi:10.1007/s10147-001-8026-8
Liu, F., Duan, J. T., Teng, X., and Peng, D. Q. (2022). The increased plasma levels of intermedin in patients with type 2 diabetes mellitus. Acta Endocrinol. (Buchar) 18 (3), 271–277. doi:10.4183/aeb.2022.271
Lu, Y. M., Zhong, J. B., Wang, H. Y., Yu, X. F., and Li, Z. Q. (2015). "The prognostic value of intermedin in patients with breast cancer." Dis. Markers 2015, 862158. doi:10.1155/2015/862158
Ma, S., Yan, F., and Hou, Y. (2023). Intermedin 1-53 ameliorates atrial fibrosis and reduces inducibility of atrial fibrillation via TGF-β1/pSmad3 and Nox4 pathway in a rat model of heart failure. J. Clin. Med. 12 (4), 1537. doi:10.3390/jcm12041537
Mao, S. Z., Fan, X. F., Xue, F., Chen, R., Chen, X. Y., Yuan, G. S., et al. (2014). Intermedin modulates hypoxic pulmonary vascular remodeling by inhibiting pulmonary artery smooth muscle cell proliferation. Pulm. Pharmacol. Ther. 27 (1), 1–9. doi:10.1016/j.pupt.2013.06.004
Minasyan, H. (2019). Sepsis: mechanisms of bacterial injury to the patient. Scand. J. Trauma Resusc. Emerg. Med. 27 (1), 19. doi:10.1186/s13049-019-0596-4
Morimoto, R., Satoh, F., Murakami, O., Hirose, T., Totsune, K., Imai, Y., et al. (2008). Expression of adrenomedullin 2/intermedin in human adrenal tumors and attached non-neoplastic adrenal tissues. J. Endocrinol. 198 (1), 175–183. doi:10.1677/JOE-08-0103
Morin, K. T., and Tranquillo, R. T. (2013). In vitro models of angiogenesis and vasculogenesis in fibrin gel. Exp. Cell Res. 319 (16), 2409–2417. doi:10.1016/j.yexcr.2013.06.006
Naot, D., and Cornish, J. (2008). The role of peptides and receptors of the calcitonin family in the regulation of bone metabolism. Bone 43 (5), 813–818. doi:10.1016/j.bone.2008.07.003
Ni, X., Zhang, J., Tang, C., and Qi, Y. (2014). Intermedin/adrenomedullin2: an autocrine/paracrine factor in vascular homeostasis and disease. Sci. China Life Sci. 57 (8), 781–789. doi:10.1007/s11427-014-4701-7
Ogoshi, M., Inoue, K., and Takei, Y. (2003). Identification of a novel adrenomedullin gene family in teleost fish. Biochem. Biophys. Res. Commun. 311 (4), 1072–1077. doi:10.1016/j.bbrc.2003.10.111
Owji, A. A., Chabot, J.-G., Dumont, Y., and Quirion, R. (2008). Adrenomedullin-2/Intermedin induces cAMP accumulation in dissociated rat spinal cord cells: evidence for the existence of a distinct class of sites of action. J. Mol. Neurosci. 35 (3), 355–361. doi:10.1007/s12031-008-9062-x
Pang, Y., Li, Y., Lv, Y., Sun, L., Zhang, S., Li, Y., et al. (2016). Intermedin restores hyperhomocysteinemia-induced macrophage polarization and improves insulin resistance in mice. J. Biol. Chem. 291 (23), 12336–12345. doi:10.1074/jbc.M115.702654
Pei, H., Wang, W., Zhao, D., Wang, L., Su, G. H., and Zhao, Z. (2018). The use of a novel non-steroidal mineralocorticoid receptor antagonist finerenone for the treatment of chronic heart failure: a systematic review and meta-analysis. Med. Baltim. 97 (16), e0254. doi:10.1097/MD.0000000000010254
Peterzan, M. A., Lygate, C. A., Neubauer, S., and Rider, O. J. (2017). Metabolic remodeling in hypertrophied and failing myocardium: a review. Am. J. Physiol. Heart Circ. Physiol. 313 (3), H597–h616. doi:10.1152/ajpheart.00731.2016
Poyner, D. R., Sexton, P. M., Marshall, I., Smith, D. M., Quirion, R., Born, W., et al. (2002). International Union of Pharmacology. XXXII. The mammalian calcitonin gene-related peptides, adrenomedullin, amylin, and calcitonin receptors. Pharmacol. Rev. 54 (2), 233–246. doi:10.1124/pr.54.2.233
Qu, Z., Jiang, Y., Xu, M., Lu, M. Z., Zhou, B., and Ding, Y. (2015). Correlation of adrenomedullin with the erythropoietin receptor and microvessel density in hepatocellular carcinoma. Arch. Med. Sci. 11 (5), 978–981. doi:10.5114/aoms.2015.54852
Ren, J. L., Hou, Y. L., Ni, X. Q., Zhu, Q., Chen, Y., Zhang, L. S., et al. (2020). Intermedin(1-53) ameliorates homocysteine-promoted atherosclerotic calcification by inhibiting endoplasmic reticulum stress. J. Cardiovasc Pharmacol. Ther. 25 (3), 251–264. doi:10.1177/1074248419885633
Ribatti, D., Nico, B., and Crivellato, E. (2015). The development of the vascular system: a historical overview. Methods Mol. Biol. 1214, 1–14. doi:10.1007/978-1-4939-1462-3_1
Roehrkasse, A. M., Booe, J. M., Lee, S. M., Warner, M. L., and Pioszak, A. A. (2018). Structure-function analyses reveal a triple β-turn receptor-bound conformation of adrenomedullin 2/intermedin and enable peptide antagonist design. J. Biol. Chem. 293 (41), 15840–15854. doi:10.1074/jbc.RA118.005062
Roh, J., Chang, C. L., Bhalla, A., Klein, C., and Hsu, S. Y. T. (2004). Intermedin is a calcitonin/calcitonin gene-related peptide family peptide acting through the calcitonin receptor-like receptor/receptor activity-modifying protein receptor complexes. J. Biol. Chem. 279 (8), 7264–7274. doi:10.1074/jbc.M305332200
Smith, R. S., Gao, L., Bledsoe, G., Chao, L., and Chao, J. (2009). Intermedin is a new angiogenic growth factor. Am. J. Physiol. Heart Circ. Physiol. 297 (3), H1040–H1047. doi:10.1152/ajpheart.00404.2009
Soultanova, A., Mikulski, Z., Pfeil, U., Grau, V., and Kummer, W. (2016). Calcitonin peptide family members are differentially regulated by LPS and inhibit functions of rat alveolar NR8383 macrophages. PLoS One 11 (10), e0163483. doi:10.1371/journal.pone.0163483
Su, Y., Guan, P., Li, D., Hang, Y., Ye, X., Han, L., et al. (2021). Intermedin attenuates macrophage phagocytosis via regulation of the long noncoding RNA Dnm3os/miR-27b-3p/SLAMF7 axis in a mouse model of atherosclerosis in diabetes. Biochem. Biophys. Res. Commun. 583, 35–42. doi:10.1016/j.bbrc.2021.10.038
Takahashi, K., Kikuchi, K., Maruyama, Y., Urabe, T., Nakajima, K., Sasano, H., et al. (2006). Immunocytochemical localization of adrenomedullin 2/intermedin-like immunoreactivity in human hypothalamus, heart and kidney. Peptides 27 (6), 1383–1389. doi:10.1016/j.peptides.2005.11.004
Takahashi, K., Morimoto, R., Hirose, T., Satoh, F., and Totsune, K. (2011). Adrenomedullin 2/intermedin in the hypothalamo-pituitary-adrenal axis. J. Mol. Neurosci. 43 (2), 182–192. doi:10.1007/s12031-010-9413-2
Takei, Y., Hashimoto, H., Inoue, K., Osaki, T., Yoshizawa-Kumagaye, K., Tsunemi, M., et al. (2008). Central and peripheral cardiovascular actions of adrenomedullin 5, a novel member of the calcitonin gene-related peptide family, in mammals. J. Endocrinol. 197 (2), 391–400. doi:10.1677/JOE-07-0541
Takei, Y., Inoue, K., Ogoshi, M., Kawahara, T., Bannai, H., and Miyano, S. (2004). Identification of novel adrenomedullin in mammals: a potent cardiovascular and renal regulator. FEBS Lett. 556 (1-3), 53–58. doi:10.1016/s0014-5793(03)01368-1
Taylor, M. M., Bagley, S. L., and Samson, W. K. (2005). Intermedin/adrenomedullin-2 acts within central nervous system to elevate blood pressure and inhibit food and water intake. Am. J. Physiol. Regul. Integr. Comp. Physiol. 288 (4), R919–R927. doi:10.1152/ajpregu.00744.2004
Vallée, A., Guillevin, R., and Vallée, J. N. (2018). Vasculogenesis and angiogenesis initiation under normoxic conditions through Wnt/β-catenin pathway in gliomas. Rev. Neurosci. 29 (1), 71–91. doi:10.1515/revneuro-2017-0032
Voors, A. A., Kremer, D., Geven, C., Ter Maaten, J. M., Struck, J., Bergmann, A., et al. (2019). Adrenomedullin in heart failure: pathophysiology and therapeutic application. Eur. J. Heart Fail 21 (2), 163–171. doi:10.1002/ejhf.1366
Wang, F., Kong, L., Wang, W., Shi, L., Wang, M., Chai, Y., et al. (2021). Adrenomedullin 2 improves bone regeneration in type 1 diabetic rats by restoring imbalanced macrophage polarization and impaired osteogenesis. Stem Cell Res. Ther. 12 (1), 288. doi:10.1186/s13287-021-02368-9
Wang, L. J., Xiao, F., Kong, L. M., Wang, D. N., Li, H. Y., Wei, Y. G., et al. (2018). Intermedin enlarges the vascular lumen by inducing the quiescent endothelial cell proliferation. Arterioscler. Thromb. Vasc. Biol. 38 (2), 398–413. doi:10.1161/ATVBAHA.117.310317
Wang, Y., Mi, Y., Tian, J., Qiao, X., Su, X., Kang, J., et al. (2020). Intermedin alleviates renal ischemia-reperfusion injury and enhances neovascularization in wistar rats. Drug Des. Devel Ther. 14, 4825–4834. doi:10.2147/DDDT.S253019
Weston, C., Lu, J., Li, N., Barkan, K., Richards, G. O., Roberts, D. J., et al. (2015). Modulation of glucagon receptor pharmacology by receptor activity-modifying protein-2 (RAMP2). J. Biol. Chem. 290 (38), 23009–23022. doi:10.1074/jbc.M114.624601
Weston, C., Winfield, I., Harris, M., Hodgson, R., Shah, A., Dowell, S. J., et al. (2016). Receptor activity-modifying protein-directed G protein signaling specificity for the calcitonin gene-related peptide family of receptors. J. Biol. Chem. 291 (42), 21925–21944. doi:10.1074/jbc.M116.751362
Wong, H. K., Tang, F., Cheung, T. T., and Cheung, B. M. (2014). Adrenomedullin and diabetes. World J. Diabetes 5 (3), 364–371. doi:10.4239/wjd.v5.i3.364
Xiao, F., Li, H., Feng, Z., Huang, L., Kong, L., Li, M., et al. (2021). Intermedin facilitates hepatocellular carcinoma cell survival and invasion via ERK1/2-EGR1/DDIT3 signaling cascade. Sci. Rep. 11 (1), 488. doi:10.1038/s41598-020-80066-x
Xiao, F., Wang, D., Kong, L., Li, M., Feng, Z., Shuai, B., et al. (2018). Intermedin protects against sepsis by concurrently re-establishing the endothelial barrier and alleviating inflammatory responses. Nat. Commun. 9 (1), 2644. doi:10.1038/s41467-018-05062-2
Xiao, F., Wang, L. J., Zhao, H., Tan, C., Wang, D. N., Zhang, H., et al. (2015). Intermedin restricts vessel sprouting by inhibiting the loosening of endothelial junction. Biochem. Biophys. Res. Commun. 458 (1), 174–179. doi:10.1016/j.bbrc.2015.01.090
Yang, J. H., Pan, C. S., Jia, Y. X., Zhang, J., Zhao, J., Pang, Y. Z., et al. (2006). Intermedin1-53 activates L-arginine/nitric oxide synthase/nitric oxide pathway in rat aortas. Biochem. Biophys. Res. Commun. 341 (2), 567–572. doi:10.1016/j.bbrc.2006.01.010
Yuan, Y., Wang, X., Zeng, Q., Wu, H. M., Qi, Y. F., and Tang, C. S. (2012). Effects of continuous intermedin infusion on blood pressure and hemodynamic function in spontaneously hypertensive rats. J. Geriatr. Cardiol. 9 (1), 17–27. doi:10.3724/SP.J.1263.2012.00017
Yuyun, M. F., Narayan, H. K., and Ng, L. L. (2015). Prognostic significance of adrenomedullin in patients with heart failure and with myocardial infarction. Am. J. Cardiol. 115 (7), 986–991. doi:10.1016/j.amjcard.2015.01.027
Zhang, H., Zhang, S. Y., Jiang, C., Li, Y., Xu, G., Xu, M. J., et al. (2016). Intermedin/adrenomedullin 2 polypeptide promotes adipose tissue browning and reduces high-fat diet-induced obesity and insulin resistance in mice. Int. J. Obes. (Lond) 40 (5), 852–860. doi:10.1038/ijo.2016.2
Zhang, L. S., Liu, Y., Chen, Y., Ren, J. L., Zhang, Y. R., Yu, Y. R., et al. (2020). Intermedin alleviates pathological cardiac remodeling by upregulating klotho. Pharmacol. Res. 159, 104926. doi:10.1016/j.phrs.2020.104926
Zhang, L. S., Zhang, J. S., Hou, Y. L., Lu, W. W., Ni, X. Q., Lin, F., et al. (2022). Intermedin(1-53) inhibits NLRP3 inflammasome activation by targeting IRE1α in cardiac fibrosis. Inflammation 45 (4), 1568–1584. doi:10.1007/s10753-022-01642-z
Zhang, S. Y., Lv, Y., Zhang, H., Gao, S., Wang, T., Feng, J., et al. (2016). Adrenomedullin 2 improves early obesity-induced adipose insulin resistance by inhibiting the class II MHC in adipocytes. Diabetes 65 (8), 2342–2355. doi:10.2337/db15-1626
Zhang, S. Y., Xu, M. J., and Wang, X. (2018). Adrenomedullin 2/intermedin: a putative drug candidate for treatment of cardiometabolic diseases. Br. J. Pharmacol. 175 (8), 1230–1240. doi:10.1111/bph.13814
Zhang, W., Wang, L. J., Xiao, F., Wei, Y., Ke, W., and Xin, H. B. (2012). Intermedin: a novel regulator for vascular remodeling and tumor vessel normalization by regulating vascular endothelial-cadherin and extracellular signal-regulated kinase. Arterioscler. Thromb. Vasc. Biol. 32 (11), 2721–2732. doi:10.1161/ATVBAHA.112.300185
Zhang, Y., Zhu, F., Du, W., He, Y., Guo, L., Yan, Z., et al. (2017). Evaluation function of intermedin on prognosis of elderly patients with sepsis. Zhonghua Wei Zhong Bing Ji Jiu Yi Xue 29 (8), 679–683. doi:10.3760/cma.j.issn.2095-4352.2017.08.002
Keywords: Intermedin (IMD), calcitonin gene-related peptide (CGRP), tumor, cardiovascular diseases, metabolic syndrome, sepsis
Citation: Yang Z, Li H, Wu P, Li Q, Yu C, Wang D and Li W (2023) Multi-biological functions of intermedin in diseases. Front. Physiol. 14:1233073. doi: 10.3389/fphys.2023.1233073
Received: 02 June 2023; Accepted: 21 August 2023;
Published: 06 September 2023.
Edited by:
Jian Wang, University of California, San Diego, United StatesReviewed by:
Ting Wang, University of California, San Diego, United StatesCopyright © 2023 Yang, Li, Wu, Li, Yu, Wang and Li. This is an open-access article distributed under the terms of the Creative Commons Attribution License (CC BY). The use, distribution or reproduction in other forums is permitted, provided the original author(s) and the copyright owner(s) are credited and that the original publication in this journal is cited, in accordance with accepted academic practice. No use, distribution or reproduction is permitted which does not comply with these terms.
*Correspondence: Denian Wang, d2FuZ2RlbmlhbjYyM0AxNjMuY29t; Weimin Li, d2VpbWkwMDNAc2N1LmVkdS5jbg==
†These authors have contributed equally to this work
Disclaimer: All claims expressed in this article are solely those of the authors and do not necessarily represent those of their affiliated organizations, or those of the publisher, the editors and the reviewers. Any product that may be evaluated in this article or claim that may be made by its manufacturer is not guaranteed or endorsed by the publisher.
Research integrity at Frontiers
Learn more about the work of our research integrity team to safeguard the quality of each article we publish.