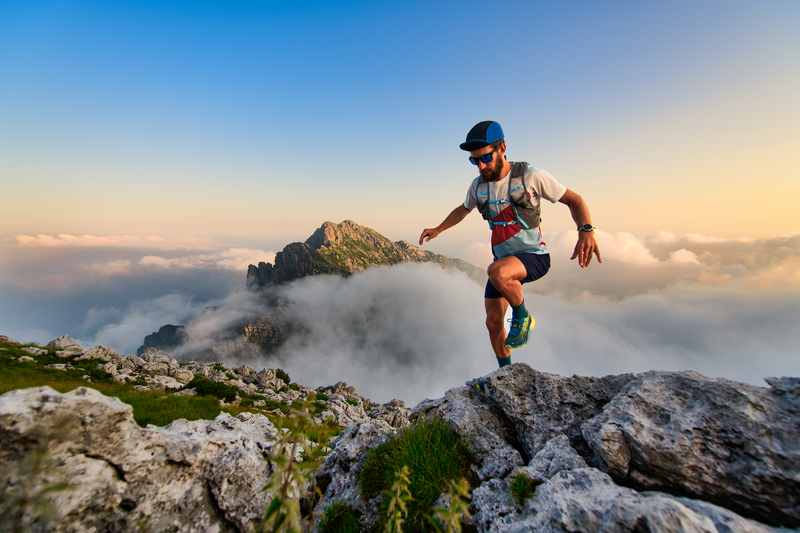
95% of researchers rate our articles as excellent or good
Learn more about the work of our research integrity team to safeguard the quality of each article we publish.
Find out more
MINI REVIEW article
Front. Physiol. , 09 October 2023
Sec. Skeletal Physiology
Volume 14 - 2023 | https://doi.org/10.3389/fphys.2023.1232698
This article is part of the Research Topic Skeletal stem/progenitor cells and their environment in bone regeneration View all 11 articles
At the macroscale, bones experience a variety of compressive and tensile loads, and these loads cause deformations of the cortical and trabecular microstructure. These deformations produce a variety of stimuli in the cellular microenvironment that can influence the differentiation of marrow stromal cells (MSCs) and the activity of cells of the MSC lineage, including osteoblasts, osteocytes, and chondrocytes. Mechanotransduction, or conversion of mechanical stimuli to biochemical and biological signals, is thus part of a multiscale mechanobiological process that drives bone modeling, remodeling, fracture healing, and implant osseointegration. Despite strong evidence of the influence of a variety of mechanical cues, and multiple paradigms proposed to explain the influence of these cues on tissue growth and differentiation, even a working understanding of how skeletal cells respond to the complex combinations of stimuli in their microenvironments remains elusive. This review covers the current understanding of what types of microenvironmental mechanical cues MSCs respond to and what is known about how they respond in the presence of multiple such cues. We argue that in order to realize the vast potential for harnessing the cellular microenvironment for the enhancement of bone regeneration, additional investigations of how combinations of mechanical cues influence bone regeneration are needed.
The response of skeletal cells to mechanical stimuli is fundamental to understanding, treating, and preventing orthopaedic injuries and diseases. The fact that bone is responsive to mechanical stimulation is well documented: athletes whose bodies experience more intense loading have increased bone mass (Bennell et al., 1997), while astronauts lose bone mass after spending time in low-gravity environments (Orwoll et al., 2013). Distraction osteogenesis, a surgical process of lengthening and reshaping a bone, improves healing outcomes in treatment of non-union by providing controlled levels of mechanical stimulation (Kanellopoulos and Soucacos, 2006; Fu et al., 2021), while metal implants can locally weaken bone due to stress shielding (Sumner, 2015; Augat and von Rüden, 2018). Mechanical cues also have a strong influence on the outcomes of fracture healing (Augat et al., 2021) and implant osseointegration (Mavrogenis et al., 2009). Bone responds to mechanical cues through multiple mechanisms, including osteocyte signaling, which plays an importantrole in bone remodeling, and the differentiation of MSCs. In the context of bone regeneration, mechanobiologically driven differentiation of MSCs is particularly important, as it determines the cell and tissue types that will form as a fracture heals. There is a long standing belief that the mechanoresponsiveness of bone, if well understood, could be routinely harnessed for therapeutic benefit in many clinical contexts. Indeed, in the words of Julius Wolff more than a century ago, “the remodelling force is a therapeutic force of immeasurable magnitude” (Wolff, 1986).
The field of orthopaedics has sought to translate this therapeutic force in a variety of ways to enhance bone regeneration. As reviewed by Mavčič and Antolič (2012), Augat et al. (2021), and Huang et al. (2013), numerous studies have attempted to enhance healing by regulating the magnitude and frequency of loading at different stages of the healing process. While some studies have achieved promising results, these results have yet to be generalized to actionable guidelines for other scenarios, or even other patients, due to the complex dependence of the mechanical stimuli on parameters such as fracture geometry and location, as well as to other factors such as patient age and co-morbidities. The first of these causes—the complexity of the relevant mechanics—arises from the fact that similar loading of bones at the macroscale may result in distinctly different microenvironmental stimuli in different patients, in different regions of bone (Figure 1) and over time as the tissue microstructure changes with adaptation. When bones experience forces, whether through load bearing or muscle contraction, the cortical and trabecular microstructures deform. The same is true for the soft tissues and woven bone that form in the initial and intermediate stages of fracture healing, and in the periosteum (McBride et al., 2011). These deformations push and pull on the cells residing within the complex geometries of bone tissue (Vaughan et al., 2015) and drive the flow of marrow and extracellular fluid around cells (Metzger et al., 2015). These local stimuli—tissue strains, fluid-based stresses, and geometric cues—constitute the cellular mechanical microenvironment.
FIGURE 1. Two regions of trabecular bone from the same mouse vertebra, each subjected to the same simulated macroscale stimulus (uniaxial compression of 2,500 μϵ) experience different distributions of microscale stimuli, such as octahedral shear strain (A,D) induced by the applied compression and fluid shear stress (B,E) due to the flow of marrow induced by the compression. 2D histograms (C,F) illustrate the difference in distributions of shear strain and fluid shear stress between the two regions of bone. The most prevalent combination of the two micro-stimuli in each region is denoted by an orange circle at (0.75 mPa, 0.035%) (C) and (2.75 mPa, 0.265%) (F).
Hence, in order to understand how and why bones adapt and heal in the ways that they do, focus has shifted from the macroscale stimuli that whole bones receive to the microscale stimuli that skeletal cells experience. However, the relative influence and the optimal levels of the various microenvironmental stimuli are not well known, particularly in complex microenvironments with a variety of different stimuli. By reviewing the evidence for the influence of these specific stimuli, individually and in combination, on MSC differentiation and the prevailing theories of how combinations of them act to regulate bone regeneration, we aim to demonstrate the potential for further harnessing the mechanical microenvironment and to identify the critical questions that must be answered in order to do so. While this review will primarily focus on the microenvironmental stimuli that influence osteogenic differentiation of MSCs in the context of bone regeneration following trauma, chondrogenic differentiation of MSCs and chondrocyte-to-osteoblast transdifferentiation also play important roles in endochondral ossification and can both be regulated by many of the same types of microenvironmental stimuli as are discussed for osteogenic differentiation of MSCs (Wong et al., 2018; McDermott et al., 2019).
Exogenous stimuli are those induced by applied mechanical loads. These stimuli arise from both the solid and fluid compartments of bone, and include solid strain, fluid shear stress, and hydrostatic pressure. Applied loads are often transient in nature, so the microenvironment is characterized also by the frequency and loading history of these exogenous stimuli, not just the instantaneous magnitudes.
A large body of work provides evidence [as reviewed by Scott et al. (2008) and Steward and Kelly (2015)] that cyclic strains can influence MSC differentiation and that the magnitude and frequency of loading are relevant factors. MSCs are capable of sensing both tensile and compressive strains, and they respond to the two in distinct ways. Cyclic tensile strains have been frequently associated with osteogenic differentiation of MSCs on both 2D substrates (Qi et al., 2008; Kearney et al., 2010) and 3D soft scaffolds (Haudenschild et al., 2009; Yu et al., 2021) while cyclic compression of MSCs in 3D constructs has been shown to promote both osteogenesis (Schreivogel et al., 2019) and chondrogenesis (Pelaez et al., 2009). Additionally, stretch-activated cation channels (SACC) have been implicated as an important component of the response to tissue strain-based deformations of cells and have been associated with synthesis of both glycosaminoglycans [GAGs, McMahon et al. (2008)] and collagen I (Kearney et al., 2010). The synthesis of matrix components as downstream effect of mechanical stimulation is a main mechanism by which the mechano-responsiveness of MSCs and osteoblasts are regulated, as changes in the matrix will likely modulate the cellular microenvironment. This general type of regulatory loop is referred to as mechanomics (Knothe Tate et al., 2016). Haudenschild et al. (2009) identified that α- and β-catenin, which are relevant in cytoskeletal mechanics and the osteogenically important Wnt signaling pathway, are regulated differently by the type of strain, with α-catenin upregulated by compressive strains and β-catenin upregulated by tensile/distortional stretch.
Despite these findings, the optimal strain magnitudes and frequencies for promoting osteogenesis are difficult to ascertain in a broadly applicable manner. This can be attributed to two main causes: differences in experimental setups and measured outputs, and the presence of other stimuli that are not always accounted for. Studies often use different loading conditions (e.g., uniaxial, biaxial, bending-based stretch), different culture conditions (e.g., serum vs. serum-free media), study different cell types (e.g., MSCs, osteoblasts, osteoblast-like cells), and measure the expression of different osteogenic markers (e.g., RUNX2, osteopontin, osteocalcin). These differences make quantitative agreement and reproducibility across studies difficult to assess. The presence of confounding factors amplifies this challenge. Differences among substrates in regards to other cues that cells experience, such as stiffness and/or curvature present another barrier to comparing results across studies. Additionally, macroscale tensile or compressive loading of 3D structures like bone can easily result in cells experiencing combinations of both tension and compression at the microscale (Niebur et al., 2000; Fields et al., 2010). Further, the fact that cells exist within aqueous environments means that strain-based stimulation doesn’t occur independently of fluid-based stimuli; however, the latter are typically not accounted for when examining the influence of strain.
A variety of studies have emphasized the importance of fluid shear stress, due to both oscillatory flow and continuous unidirectional flow, in skeletal cell mechanobiology. Arnsdorf et al. (2009) demonstrated that oscillatory fluid flow promotes osteogenic differentiation of MSCs by activating RhoA, a regulator of ROCKII and subsequently cytoskeletal tension and organization. Corrigan et al. (2018) found that calcium channel transient receptor potential subfamily V member 4 (TRPV4) is critical for flow-based mechanotransduction in MSCs and is strongly associated with mechanosensitivity of the primary cilium. A variety of studies have demonstrated that parallel flow over a flat monolayer of MSCs can induce osteogenic behavior when the shear stress is on the order of 1 Pa in constant (Reich and Frangos, 1991; Yourek et al., 2010) and oscillatory (Li et al., 2004; Stavenschi et al., 2017) flow conditions, with both the magnitude and frequency of flow being influential factors (Stavenschi et al., 2017). Interestingly, studies that examine flow through 3D scaffolds report that much lower shear stresses, on the order of 1 mPa, are associated with increased osteogenesis while stresses above approximately 10 mPa are detrimental to cell viability (Porter et al., 2005; Melke et al., 2018). This discrepancy may be indicative of a broader difference between cell-microenvironment interactions in 2D vs. 3D contexts (Baker and Chen, 2012). In addition to regulation of MSC differentiation, fluid shear stress has been shown to induce immunomodulatory behavior in MSCs (Skibber et al., 2022). Along with changes in fluid shear stress, fluid flow is also associated with changes in hydrostatic pressure. High cyclically applied hydrostatic pressure (∼100–1,000 kPa) has been associated with chondrogenic differentiation of MSCs (Wagner et al., 2008; Stavenschi and Hoey, 2019) while lower pressures (∼10–300 kPa) have been associated with osteogenesis (Burger et al., 1992; Tang et al., 2017; Reinwald and El Haj, 2018).
Not only does fluid flow apply forces to cells, it also distributes nutrients. The flow of nutrient- and oxygen-carrying fluid helps to ensure the distribution of nutrients to cells throughout a 3D environment to maintain cell viability and enable proliferation and differentiation (Karande et al., 2004; Amini and Nukavarapu, 2014). Donahue et al. (2003) found that cells were significantly less responsive to shear stress in nutrient-free media, further demonstrating the difficulty of separating the effects of chemotransport and fluid flow.
In the absence of externally applied loads, there are still physical cues endogenous to the microenvironment. These factors, which include curvature as well as matrix/substrate stiffness, influence cell adhesion and cytoskeletal tension and are capable of driving MSC differentiation.
As reviewed by Werner et al. (2020), cells are capable of sensing curvatures at both a subcellular length scale (primarily through focal adhesion placement and growth) and length scales greater than or equal to the size of the cell (due to interactions between stress fibers and the nucleus). For MSCs cultured on hemispherical concave and convex surfaces, Werner et al. (2017) found that convex curvatures increased osteogenic gene expression while concave curvatures increased cell migration speeds. Werner et al. (2019) additionally introduced the notion of direction-dependent “perceived curvature” on non-spherical anisotropic curvatures (such as a cylindrical curvature). The perceived curvature acknowledges that cells oriented along the long axis of a cylinder experience a different amount of curvature and therefore undergo less bending than cells oriented perpendicular to the long axis; MSCs were observed to alter their migration behavior, ostensibly to avoid this bending. Callens et al. (2023) studied pre-osteoblasts on patterned substrates with a broader range of curvatures and found that groups of cells preferentially pattern surfaces with at least one negative principal curvature (i.e., concave-saddle), though over time groups of cells are able overcome convexities through cell-cell interactions that result in the formation of cell sheets that bridge unfavorable curvatures. Yang et al. (2022) found that saddle-like surfaces (triply periodic minimal surface-based scaffolds) promoted both osteogenesis and angiogenesis in vivo. The magnitude of curvature, in addition to the shape of curvature, is relevant to MSCs, with Swanson et al. (2022) finding that small spherical pores with curvatures in the range (16.0, 33.3) mm−1 maintained the stemness of MSCs while larger pores with curvatures in the range (4.7, 8.0) mm−1 promoted osteogenic differentiation, however there is a lack of thorough examination of how variations in curvature magnitude influence osteogenic behavior of MSCs, particularly when coupled with variations in curvature shape. The lack of consensus as to what constitutes an “optimal” curvature for osteogenesis may be attributable to the possibility that different processes are stimulated by different curvatures, for example, a curvature that promotes osteogenic differentiation may differ from a curvature that promotes tissue formation.
The curvature of a surface influences how cells attach to it, making surface curvature an important determinant of cell shape (Werner et al., 2017). McBeath et al. (2004) found that cell shape regulates RhoA, which in turn regulates the switch between adipogenic and osteogenic differentiation, with cells becoming osteoblasts when they were allowed to spread, and adipocytes when they were maintained as round. Similarly, Kilian et al. (2010) found that seeding MSCs on 2D islands of different shapes led to different lineage commitments, with shapes that had higher aspect ratios or concave subcellular curvatures generally found to increase cytoskeletal contractility and osteogenic differentiation. This association among curvature, cell shape, and the cytoskeleton emphasizes the mechanical nature of sensing of microenvironmental curvature. Through focal adhesions and cytoskeletal mechanics, MSCs are also able to sense the local stiffness of their microenvironment. When seeded on substrates of varying stiffnesses, MSCs were found to undergo morphological changes and exhibited neurogenic, myogenic, or osteogenic differentiation depending on substrate stiffness, further implicating cytoskeletal contractility as a key sensory mechanism of the physical microenvironment (Engler et al., 2006). There has been extensive study of the role of matrix/substrate stiffness and the interactions between MSCs and the extracellular matrix more generally, which have been reviewed by Assis-Ribas et al. (2018) and Lv et al. (2015).
There are, of course, many non-mechanical factors that influence osteogenesis and bone regeneration. As reviewed by Hayrapetyan et al. (2015), there are various hormones, cytokines, and signaling pathways that are critical to osteogenic differentiation. Bone regeneration is also strongly coupled to other physiological processes, including angiogenesis which delivers oxygen and essential nutrients [as reviewed by Kanczler and Oreffo (2008)], the immune/inflammatory response which plays an essential role in initiating the repair process [as reviewed by Claes et al. (2012)], and the presence of extracellular matrix proteins which have also been shown to play a significant role in mediating the behavior of MSCs (Datta et al., 2006). The influence of nutrients can be particularly relevant in vitro, as the choice of media can strongly influence the differentiation of MSCs (Ho et al., 2011; Kyllönen et al., 2013). While these chemical and biological factors are indeed essential to the bone regeneration process, they too occur within the context of a mechanical environment and are coupled with the mechanobiological response of skeletal cells.
The simultaneous presence of multiple stimuli in the microenvironments of skeletal cells in vivo (Moraes et al., 2011) makes it difficult to draw general conclusions about skeletal mechanobiology from examining only individual stimuli. Relatively few studies have attempted to directly quantify the effects of multiple mechanical cues acting concurrently, leaving open questions about how cells respond to combinations of cues.
Jiao et al. (2022) examined the synergistic effects of adhesion morphology and fluid shear stress by applying different levels of flow to cells seeded on differently shaped micro patterned substrates. Their results demonstrated that fluid shear stress and adhesion morphology could work cooperatively or antagonistically to regulate osteogenesis, with osteogenically favorable adhesion morphologies enhancing the osteogenic response induced by fluid flow and unfavorable morphologies blunting its influence. Further, they found that fluid shear stress had no effect on cell shape or spreading, indicating that the two cues regulate osteogenesis through different mechanisms.
Additional studies on the influence of multiple concurrent mechanical cues are based on observation of tissue differentiation in vivo, often in the context of bone regeneration following fracture. These studies have formulated hypotheses of how mechanical stimuli lead to local tissue differentiation into bone, cartilage, or fibrous tissue. Different models have considered different pairings of tensile, compressive, and shear stresses and strains as well as fluid flow velocities and hydrostatic pressures. Prendergast et al. (1997), Carter et al. (1998), and Claes and Heigele (1999) each proposed paradigms to predict tissue differentiation within a healing fracture callus. A comparative analysis by Isaksson et al. (2006a) between the mechanoregulatory models of Carter et al. (1998), Claes and Heigele (1999), Lacroix and Prendergast (2002), and a model based on deviatoric strain by Isaksson et al. (2006b) and found that the model by Lacroix and Prendergast (2002), which is an extension of the model proposed by Prendergast et al. (1997) and postulates that tissue differentiation depends on combinations of shear strain and fluid flow velocity, was the most consistent with experimental data, matching those data in most, but not all, cases that were examined. Song et al. (2012) examined similar relationships between MSC lineage commitment and stress and strain by measuring local cellular deformations and the expression of lineage-associated genes. Despite efforts towards a working mechanobiological theory of bone regeneration, more work is needed to fully unify the influence of the various endogenous, exogenous, and non-mechanical factors into a robust and clinically translatable predictive model.
All of the aforementioned models consider how combinations of stimuli impact cell and tissue differentiation in regions such as a fracture callus where there is preliminary granulation tissue present. A different class of mechanobiological models considers how various cues promote the growth of new tissue into pore space, which is relevant in both bone remodeling and the osseointegration of bone tissue engineering scaffolds and other implants. A series of models by Geris and colleagues (Guyot et al., 2014; Guyot et al., 2016; Mehrian et al., 2018) predicts neotissue growth due to curvature, fluid shear stress, and metabolic factors (oxygen, glucose, pH) for scaffolds in perfusion bioreactors. Another growth model considers the growth and remodeling of trabecular bone (Aland et al., 2020) using both strain energy density and volumetric compression as possible strain-based remodeling stimuli. Both types of models describe important parts of the mechanobiological response of bone, but neither captures its full scope.
The cellular microenvironment contains a variety of mechanical stimuli that both individually and collectively appear to regulate cellular activity and mediate osteogenesis during bone repair and regeneration. While the fact that these stimuli, including curvature, stiffness, strain, fluid shear stress, and hydrostatic pressure, are influential has been convincingly demonstrated, a thorough quantitative understanding of their influence at various magnitudes, frequencies, and durations is still lacking, particularly when multiple stimuli act concurrently. Such an understanding could enable new therapies for enhanced bone regeneration, patient-specific treatment plans, and improved design of orthopaedic implants. In the absence of that understanding, progress on these fronts is likely to be slow.
Mechanobiological considerations are increasingly being made in the treatment of bone fractures, as well as injuries in other tissues, as described for example, by the revised definition of the term “mechanotherapy” (Huang et al., 2013; Thompson et al., 2016). Several methods have been proposed to provide controlled levels of mechanical stimulation to skeletal cells, including low intensity vibration (LIV) and low intensity pulsed ultrasound (LIPUS), which have in at least some studies shown a potential for improving bone regeneration (Thompson et al., 2016); however, recent studies have questioned the efficacy of these treatments and called for additional investigation into the situations when such treatments may provide benefit, further demonstrating the need for a thorough understanding of how MSCs respond to microenvironmental stimuli (Lou et al., 2017; Searle et al., 2023). Using the mechanobiological model of Prendergast et al. (1997) and Miramini et al. (2015) demonstrated that different locking compression plate configurations can yield different mechanical microenvironments and tissue differentiation patterns, highlighting the potential of using enhanced understanding of mechanical microenvironments to impact specific approaches to fracture fixation.
Patient-specific treatments are particularly relevant in the context of aging-related changes to bone. The mechanoresponsiveness of bone has been shown to be altered by aging in both animals (Turner et al., 1995) and humans (Kohrt, 2001), though it remains unclear whether this alteration is due to diminished mechanosensitivity of cells or to microstructural changes that alter the microenvironmental stimuli that they receive. Furthering the understanding of both the microstructural changes associated with aging and the effects of aging on cell mechanoresponsiveness could support the development of treatments and activity guidelines for both improved fracture healing and maintenance of bone mass that are specific to an individual’s age and health.
In surgical situations that call for the use of orthopaedic implants, the design of those implants offers an opportunity to apply the understanding of skeletal mechanobiology to custom-designed microenvironments. Among the most direct possible applications is in the design and development of bone tissue engineering scaffolds. Microenvironmentally-informed scaffold architectures could be used to regulate the stimuli that cells seeded on their surfaces perceive in order to enhance tissue growth. This could lead to the development of artificial bone grafts that are both safer and more effective than auto- or allografts. Other relevant applications include improving the osseointegration of joint replacement implants and developing fracture fixation implants that regulate the allowable motion of a fracture site to improve healing outcomes.
In order to fully realize the potential of harnessing the mechanical microenvironment, further work is needed. Future studies that assess the influence of individual or combinations of stimuli should quantify both the applied macroscale stimuli as well as the local microscale stimuli. This broader accounting of stimuli would enhance the applicability of findings and make them relevant beyond the scope of particular experimental setups. Additionally, efforts should be made to account for stimuli beyond those being directly investigated, for example, compression-induced fluid flow. To this end, computational simulation offers a powerful tool for analysis of microenvironments (Figure 1) that can be paired with experimental results (Schulte et al., 2013). Another step to improving the robustness and generalizability of studies examining the effect of microenvironmental stimuli on osteogenesis is to examine multiple markers of osteogenic differentiation, with an eye towards developing a minimum standard set of readouts. Osteocalcin, osteopontin, and RUNX2 are all commonly used markers of osteogenic differentiation; however many studies examine only one, making it difficult to compare results between studies.
Overall, the importance of mechanical cues to bone regeneration highlights the importance of elucidating the mechanoresponsiveness of skeletal cells to combinations of microenvironmental stimuli. Doing so has the potential to address a variety of key clinical needs and answer major questions about the nature of skeletal mechanobiology.
TJ: Writing–Original Draft and Conceptualization. EM: Conceptualization, Writing–Reviewing and Editing, Supervision, and Funding acquisition. All authors contributed to the article and approved the submitted version.
The funding was provided by the National Institutes of Health/National Institute on Aging (Grant #AG073671 to EM).
The authors declare that the research was conducted in the absence of any commercial or financial relationships that could be construed as a potential conflict of interest.
All claims expressed in this article are solely those of the authors and do not necessarily represent those of their affiliated organizations, or those of the publisher, the editors and the reviewers. Any product that may be evaluated in this article, or claim that may be made by its manufacturer, is not guaranteed or endorsed by the publisher.
Aland S., Stenger F., Müller R., Deutsch A., Voigt A. (2020). A phase field approach to trabecular bone remodeling. Front. Appl. Math. Statistics 6, 12. doi:10.3389/fams.2020.00012
Amini A. R., Nukavarapu S. P. (2014). Oxygen-tension controlled matrices for enhanced osteogenic cell survival and performance. Ann. Biomed. Eng. 42, 1261–1270. doi:10.1007/s10439-014-0990-z
Arnsdorf E. J., Tummala P., Kwon R. Y., Jacobs C. R. (2009). Mechanically induced osteogenic differentiation - the role of RhoA, ROCKII and cytoskeletal dynamics. J. Cell Sci. 122, 546–553. doi:10.1242/jcs.036293
Assis-Ribas T., Forni M. F., Winnischofer S. M. B., Sogayar M. C., Trombetta-Lima M. (2018). Extracellular matrix dynamics during mesenchymal stem cells differentiation. Dev. Biol. 437, 63–74. doi:10.1016/j.ydbio.2018.03.002
Augat P., Hollensteiner M., von Rüden C. (2021). The role of mechanical stimulation in the enhancement of bone healing. Injury 52, S78–S83. doi:10.1016/j.injury.2020.10.009
Augat P., von Rüden C. (2018). Evolution of fracture treatment with bone plates. Injury 49, S2–S7. doi:10.1016/S0020-1383(18)30294-8
Baker B. M., Chen C. S. (2012). Deconstructing the third dimension-how 3D culture microenvironments alter cellular cues. J. Cell Sci. 125, 3015–3024. doi:10.1242/jcs.079509
Bennell K. L., Malcolm S. A., Khan K. M., Thomas S. A., Reid S. J., Brukner P. D., et al. (1997). Bone mass and bone turnover in power athletes, endurance athletes, and controls: A 12-month longitudinal study. Bone 20, 477–484. doi:10.1016/S8756-3282(97)00026-4
Burger E. H., Klein-Nulend J., Veldhuijzen J. P. (1992). Mechanical stress and osteogenesis in vitro. J. Bone Mineral Res. 7, S397–S401. doi:10.1002/jbmr.5650071406
Callens S. J. P., Fan D., van Hengel I. A. J., Minneboo M., Díaz-Payno P. J., Stevens M. M., et al. (2023). Emergent collective organization of bone cells in complex curvature fields. Nat. Commun. 14, 855. doi:10.1038/s41467-023-36436-w
Carter D. R., Beaupré G. S., Giori N. J., Helms J. A. (1998). Mechanobiology of skeletal regeneration. Clin. Orthop. Relat. Res. 355, S41–S55. doi:10.1097/00003086-199810001-00006
Claes L. E., Heigele C. A. (1999). Magnitudes of local stress and strain along bony surfaces predict the course and type of fracture healing. J. Biomechanics 32, 255–266. doi:10.1016/S0021-9290(98)00153-5
Claes L., Recknagel S., Ignatius A. (2012). Fracture healing under healthy and inflammatory conditions. Nat. Rev. Rheumatol. 8, 133–143. doi:10.1038/nrrheum.2012.1
Corrigan M. A., Johnson G. P., Stavenschi E., Riffault M., Labour M. N., Hoey D. A. (2018). TRPV4-mediates oscillatory fluid shear mechanotransduction in mesenchymal stem cells in part via the primary cilium. Sci. Rep. 8, 3824. doi:10.1038/s41598-018-22174-3
Datta N., Pham Q. P., Sharma U., Sikavitsas V. I., Jansen J. A., Mikos A. G. (2006). In vitro generated extracellular matrix and fluid shear stress synergistically enhance 3D osteoblastic differentiation. Proc. Natl. Acad. Sci. U. S. A. 103, 2488–2493. doi:10.1073/pnas.0505661103
Donahue T. L., Haut T. R., Yellowley C. E., Donahue H. J., Jacobs C. R. (2003). Mechanosensitivity of bone cells to oscillating fluid flow induced shear stress may be modulated by chemotransport. J. Biomechanics 36, 1363–1371. doi:10.1016/S0021-9290(03)00118-0
Engler A. J., Sen S., Sweeney H. L., Discher D. E. (2006). Matrix elasticity directs stem cell lineage specification. Cell 126, 677–689. doi:10.1016/j.cell.2006.06.044
Fields A. J., Lee G. L., Keaveny T. M. (2010). Mechanisms of initial endplate failure in the human vertebral body. J. Biomechanics 43, 3126–3131. doi:10.1016/j.jbiomech.2010.08.002
Fu R., Feng Y., Liu Y., Yang H. (2021). Mechanical regulation of bone regeneration during distraction osteogenesis. Med. Nov. Technol. Devices 11, 100077. doi:10.1016/j.medntd.2021.100077
Guyot Y., Papantoniou I., Chai Y. C., Van Bael S., Schrooten J., Geris L. (2014). A computational model for cell/ECM growth on 3D surfaces using the level set method: a bone tissue engineering case study. Biomechanics Model. Mechanobiol. 13, 1361–1371. doi:10.1007/s10237-014-0577-5
Guyot Y., Papantoniou I., Luyten F. P., Geris L. (2016). Coupling curvature-dependent and shear stress-stimulated neotissue growth in dynamic bioreactor cultures: a 3D computational model of a complete scaffold. Biomechanics Model. Mechanobiol. 15, 169–180. doi:10.1007/s10237-015-0753-2
Haudenschild A. K., Hsieh A. H., Kapila S., Lotz J. C. (2009). Pressure and distortion regulate human mesenchymal stem cell gene expression. Ann. Biomed. Eng. 37, 492–502. doi:10.1007/s10439-008-9629-2
Hayrapetyan A., Jansen J. A., Van Den Beucken J. J. (2015). Signaling pathways involved in osteogenesis and their application for bone regenerative medicine. Tissue Eng. - Part B Rev. 21, 75–87. doi:10.1089/ten.teb.2014.0119
Ho S. T. B., Tanavde V. M., Hui J. H., Lee E. H. (2011). Upregulation of adipogenesis and chondrogenesis in MSC serum-free culture. Cell Med. 2, 27–41. doi:10.3727/215517911x575984
Huang C., Holfeld J., Schaden W., Orgill D., Ogawa R. (2013). Mechanotherapy: revisiting physical therapy and recruiting mechanobiology for a new era in medicine. Trends Mol. Med. 19, 555–564. doi:10.1016/j.molmed.2013.05.005
Isaksson H., van Donkellar C. C., Huiskes R., Ito K. (2006a). Corroboration of mechanoregulatory algorithms for tissue differentiation during fracture healing: comparison with in vivo results. J. Orthop. Res. 24, 898–907. doi:10.1002/jor.20118
Isaksson H., Wilson W., van Donkelaar C. C., Huiskes R., Ito K. (2006b). Comparison of biophysical stimuli for mechano-regulation of tissue differentiation during fracture healing. J. Biomechanics 39, 1507–1516. doi:10.1016/j.jbiomech.2005.01.037
Jiao F., Xu J., Zhao Y., Ye C., Sun Q., Liu C., et al. (2022). Synergistic effects of fluid shear stress and adhesion morphology on the apoptosis and osteogenesis of mesenchymal stem cells. J. Biomed. Mater. Res. - Part A 110, 1636–1644. doi:10.1002/jbm.a.37413
Kanczler J. M., Oreffo R. O. (2008). Osteogenesis and angiogenesis: the potential for engineering bone. Eur. Cells Mater. 15, 100–114. doi:10.22203/eCM.v015a08
Kanellopoulos A. D., Soucacos P. N. (2006). Management of nonunion with distraction osteogenesis. Injury 37, S51–S55. doi:10.1016/j.injury.2006.02.041
Karande T. S., Ong J. L., Agrawal C. M. (2004). Diffusion in musculoskeletal tissue engineering scaffolds: design issues related to porosity, permeability, architecture, and nutrient mixing. Ann. Biomed. Eng. 32, 1728–1743. doi:10.1007/s10439-004-7825-2
Kearney E. M., Farrell E., Prendergast P. J., Campbell V. A. (2010). Tensile strain as a regulator of mesenchymal stem cell osteogenesis. Ann. Biomed. Eng. 38, 1767–1779. doi:10.1007/s10439-010-9979-4
Kilian K. A., Bugarija B., Lahn B. T., Mrksich M. (2010). Geometric cues for directing the differentiation of mesenchymal stem cells. Proc. Natl. Acad. Sci. U. S. A. 107, 4872–4877. doi:10.1073/pnas.0903269107
Knothe Tate M. L., Gunning P. W., Sansalone V. (2016). Emergence of form from function—mechanical engineering approaches to probe the role of stem cell mechanoadaptation in sealing cell fate. BioArchitecture 6, 85–103. doi:10.1080/19490992.2016.1229729
Kohrt W. M. (2001). Aging and the osteogenic response to mechanical loading. Int. J. Sport Nutr. 11, S137–S142. doi:10.1123/ijsnem.11.s1.s137
Kyllönen L., Haimi S., Mannerström B., Huhtala H., Rajala K. M., Skottman H., et al. (2013). Effects of different serum conditions on osteogenic differentiation of human adipose stem cells in vitro. Stem Cell Res. Ther. 4, 17. doi:10.1186/scrt165
Lacroix D., Prendergast P. J. (2002). A mechano-regulation model for tissue differentiation during fracture healing: analysis of gap size and loading. J. Biomechanics 35, 1163–1171. doi:10.1016/S0021-9290(02)00086-6
Li Y. J., Batra N. N., You L., Meier S. C., Coe I. A., Yellowley C. E., et al. (2004). Oscillatory fluid flow affects human marrow stromal cell proliferation and differentiation. J. Orthop. Res. 22, 1283–1289. doi:10.1016/j.orthres.2004.04.002
Lou S., Lv H., Li Z., Zhang L., Tang P. (2017). The effects of low-intensity pulsed ultrasound on fresh fracture: A meta-analysis. Med. (United States) 96, e8181. doi:10.1097/MD.0000000000008181
Lv H., Li L., Sun M., Zhang Y., Chen L., Rong Y., et al. (2015). Mechanism of regulation of stem cell differentiation by matrix stiffness. Stem Cell Res. Ther. 6, 103–111. doi:10.1186/s13287-015-0083-4
Mavčič B., Antolič V. (2012). Optimal mechanical environment of the healing bone fracture/osteotomy. Int. Orthop. 36, 689–695. doi:10.1007/s00264-012-1487-8
Mavrogenis A. F., Dimitriou R., Parvizi J., Babis G. C. (2009). Biology of implant osseointegration. J Musculoskelet Neuronal Interact 9, 61-71.
McBeath R., Pirone D. M., Nelson C. M., Bhadriraju K., Chen C. S. (2004). Cell shape, cytoskeletal tension, and RhoA regulate stem cell lineage commitment. Dev. Cell 6, 483–495. doi:10.1016/S1534-5807(04)00075-9
McBride S. H., Dolejs S., Brianza S., Knothe U., Knothe Tate M. L. (2011). Net change in periosteal strain during stance shift loading after surgery correlates to rapid de novo bone generation in critically sized defects. Ann. Biomed. Eng. 39, 1570–1581. doi:10.1007/s10439-010-0242-9
McDermott A. M., Herberg S., Mason D. E., Collins J. M., Pearson H. B., Dawahare J. H., et al. (2019). Recapitulating bone development through engineered mesenchymal condensations and mechanical cues for tissue regeneration. Sci. Transl. Med. 11, 7756. doi:10.1126/scitranslmed.aav7756
McMahon L. A., Campbell V. A., Prendergast P. J. (2008). Involvement of stretch-activated ion channels in strain-regulated glycosaminoglycan synthesis in mesenchymal stem cell-seeded 3D scaffolds. J. Biomechanics 41, 2055–2059. doi:10.1016/j.jbiomech.2008.03.027
Mehrian M., Guyot Y., Papantoniou I., Olofsson S., Sonnaert M., Misener R., et al. (2018). Maximizing neotissue growth kinetics in a perfusion bioreactor: an in silico strategy using model reduction and bayesian optimization. Biotechnol. Bioeng. 115, 617–629. doi:10.1002/bit.26500
Melke J., Zhao F., Rietbergen B., Ito K., Hofmann S. (2018). Localisation of mineralised tissue in a complex spinner flask environment correlates with predicted wall shear stress level localisation. Eur. Cells Mater. 36, 57–68. doi:10.22203/eCM.v036a05
Metzger T. A., Schwaner S. A., LaNeve A. J., Kreipke T. C., Niebur G. L. (2015). Pressure and shear stress in trabecular bone marrow during whole bone loading. J. Biomechanics 48, 3035–3043. doi:10.1016/j.jbiomech.2015.07.028
Miramini S., Zhang L., Richardson M., Pirpiris M., Mendis P., Oloyede K., et al. (2015). Computational simulation of the early stage of bone healing under different configurations of locking compression plates. Comput. Methods Biomechanics Biomed. Eng. 18, 900–913. doi:10.1080/10255842.2013.855729
Moraes C., Sun Y., Simmons C. A. (2011). Micro)managing the mechanical microenvironment. Integr. Biol. 3, 959–971. doi:10.1039/c1ib00056j
Niebur G. L., Feldstein M. J., Yuen J. C., Chen T. J., Keaveny T. M. (2000). High-resolution finite element models with tissue strength asymmetry accurately predict failure of trabecular bone. J. Biomechanics 33, 1575–1583. doi:10.1016/S0021-9290(00)00149-4
Orwoll E. S., Adler R. A., Amin S., Binkley N., Lewiecki E. M., Petak S. M., et al. (2013). Skeletal health in long-duration astronauts: nature, assessment, and management recommendations from the NASA bone summit. J. Bone Mineral Res. 28, 1243–1255. doi:10.1002/jbmr.1948
Pelaez D., Charles Huang C. Y., Cheung H. S. (2009). Cyclic compression maintains viability and induces chondrogenesis of human mesenchymal stem cells in fibrin gel scaffolds. Stem Cells Dev. 18, 93–102. doi:10.1089/scd.2008.0030
Porter B., Zauel R., Stockman H., Guldberg R., Fyhrie D. (2005). 3-D computational modeling of media flow through scaffolds in a perfusion bioreactor. J. Biomechanics 38, 543–549. doi:10.1016/j.jbiomech.2004.04.011
Prendergast P. J., Huiskes R., Søballe K. (1997). ESB Research Award 1996. Biophysical stimuli on cells during tissue differentiation at implant interfaces. J. Biomechanics 30, 539–548. doi:10.1016/S0021-9290(96)00140-6
Qi M. C., Hu J., Zou S. J., Chen H. Q., Zhou H. X., Han L. C. (2008). Mechanical strain induces osteogenic differentiation: cbfa1 and ets-1 expression in stretched rat mesenchymal stem cells. Int. J. Oral Maxillofac. Surg. 37, 453–458. doi:10.1016/j.ijom.2007.12.008
Reich K. M., Frangos J. A. (1991). Effect of flow on prostaglandin E2 and inositol trisphosphate levels in osteoblasts. Am. J. Physiology - Cell Physiology 261, C428–C432. doi:10.1152/ajpcell.1991.261.3.c428
Reinwald Y., El Haj A. J. (2018). Hydrostatic pressure in combination with topographical cues affects the fate of bone marrow-derived human mesenchymal stem cells for bone tissue regeneration. J. Biomed. Mater. Res. - Part A 106, 629–640. doi:10.1002/jbm.a.36267
Schreivogel S., Kuchibhotla V., Knaus P., Duda G. N., Petersen A. (2019). Load-induced osteogenic differentiation of mesenchymal stromal cells is caused by mechano-regulated autocrine signaling. J. Tissue Eng. Regen. Med. 13, 1992–2008. doi:10.1002/term.2948
Schulte F. A., Ruffoni D., Lambers F. M., Christen D., Webster D. J., Kuhn G., et al. (2013). Local mechanical stimuli regulate bone formation and resorption in mice at the tissue level. PLoS ONE 8, e62172. doi:10.1371/journal.pone.0062172
Scott A., Khan K. M., Duronio V., Hart D. A. (2008). Mechanotransduction in human bone: in vitro cellular physiology that underpins bone changes with exercise. Sports Med. 38, 139–160. doi:10.2165/00007256-200838020-00004
Searle H. K., Lewis S. R., Coyle C., Welch M., Griffin X. L. (2023). Ultrasound and shockwave therapy for acute fractures in adults. Cochrane Database Syst. Rev. 2023, CD008579. doi:10.1002/14651858.CD008579.pub4
Skibber M. A., Olson S. D., Prabhakara K. S., Gill B. S., Cox C. S. (2022). Enhancing mesenchymal stromal cell Potency: inflammatory licensing via mechanotransduction. Front. Immunol. 13, 874698. doi:10.3389/fimmu.2022.874698
Song M. J., Brady-Kalnay S. M., McBride S. H., Phillips-Mason P., Dean D., Tate M. L. (2012). Mapping the mechanome of live stem cells using a novel method to measure local strain fields in situ at the fluid-cell interface. PLOS ONE 7, e43601. doi:10.1371/JOURNAL.PONE.0043601
Stavenschi E., Hoey D. A. (2019). Pressure-induced mesenchymal stem cell osteogenesis is dependent on intermediate filament remodeling. FASEB J. 33, 4178–4187. doi:10.1096/fj.201801474RR
Stavenschi E., Labour M. N., Hoey D. A. (2017). Oscillatory fluid flow induces the osteogenic lineage commitment of mesenchymal stem cells: the effect of shear stress magnitude, frequency, and duration. J. Biomechanics 55, 99–106. doi:10.1016/j.jbiomech.2017.02.002
Steward A. J., Kelly D. J. (2015). Mechanical regulation of mesenchymal stem cell differentiation. J. Anat. 227, 717–731. doi:10.1111/joa.12243
Sumner D. R. (2015). Long-term implant fixation and stress-shielding in total hip replacement. J. Biomechanics 48, 797–800. doi:10.1016/j.jbiomech.2014.12.021
Swanson W. B., Omi M., Woodbury S. M., Douglas L. M., Eberle M., Ma P. X., et al. (2022). Scaffold Pore curvature influences μsc fate through differential cellular organization and YAP/TAZ activity. Int. J. Mol. Sci. 23, 4499. doi:10.3390/ijms23094499
Tang X., Teng S., Liu C., Jagodzinski M. (2017). Influence of hydrodynamic pressure on the proliferation and osteogenic differentiation of bone mesenchymal stromal cells seeded on polyurethane scaffolds. J. Biomed. Mater. Res. - Part A 105, 3445–3455. doi:10.1002/jbm.a.36197
Thompson W. R., Scott A., Terry Loghmani M., Ward S. R., Warden S. J. (2016). Understanding mechanobiology: Physical therapists as a force in mechanotherapy and musculoskeletal regenerative rehabilitation. Phys. Ther. 96, 560–569. doi:10.2522/ptj.20150224
Turner C. H., Takano Y., Owan I. (1995). Aging changes mechanical loading thresholds for bone formation in rats. J. Bone Mineral Res. 10, 1544–1549. doi:10.1002/jbmr.5650101016
Vaughan T. J., Voisin M., Niebur G. L., McNamara L. M. (2015). Multiscale modeling of trabecular bone marrow: understanding the micromechanical environment of mesenchymal stem cells during osteoporosis. J. Biomechanical Eng. 137. doi:10.1115/1.4028986
Wagner D. R., Lindsey D. P., Li K. W., Tummala P., Chandran S. E., Smith R. L., et al. (2008). Hydrostatic pressure enhances chondrogenic differentiation of human bone marrow stromal cells in osteochondrogenic medium. Ann. Biomed. Eng. 36, 813–820. doi:10.1007/s10439-008-9448-5
Werner M., Blanquer S. B., Haimi S. P., Korus G., Dunlop J. W., Duda G. N., et al. (2017). Surface curvature differentially regulates stem cell migration and differentiation via altered attachment morphology and nuclear deformation. Adv. Sci. 4, 1600347. doi:10.1002/advs.201600347
Werner M., Kurniawan N. A., Bouten C. V. (2020). Cellular geometry sensing at different length scales and its implications for scaffold design. Materials 13, 963. doi:10.3390/ma13040963
Werner M., Petersen A., Kurniawan N. A., Bouten C. V. (2019). Cell-Perceived substrate curvature dynamically coordinates the direction, speed, and persistence of stromal cell migration. Adv. Biosyst. 3, e1900080. doi:10.1002/adbi.201900080
Wong S. A., Rivera K. O., Miclau T., Alsberg E., Marcucio R. S., Bahney C. S. (2018). Microenvironmental regulation of chondrocyte plasticity in endochondral repair-A new frontier for developmental engineering. Front. Bioeng. Biotechnol. 6, 58. doi:10.3389/fbioe.2018.00058
Yang Y., Xu T., Bei H. P., Zhang L., Tang C. Y., Zhang M., et al. (2022). Gaussian curvature-driven direction of cell fate toward osteogenesis with triply periodic minimal surface scaffolds. Proc. Natl. Acad. Sci. U. S. A. 119, e2206684119. doi:10.1073/pnas.2206684119
Yourek G., McCormick S. M., Mao J. J., Reilly G. C. (2010). Shear stress induces osteogenic differentiation of human mesenchymal stem cells. Regen. Med. 5, 713–724. doi:10.2217/RME.10.60
Keywords: bone, mechanobiology, marrow stromal cells (MSCs), mechanical microenvironment, osteogenesis
Citation: Josephson TO and Morgan EF (2023) Harnessing mechanical cues in the cellular microenvironment for bone regeneration. Front. Physiol. 14:1232698. doi: 10.3389/fphys.2023.1232698
Received: 01 June 2023; Accepted: 25 September 2023;
Published: 09 October 2023.
Edited by:
Celine Colnot, Institut National de la Santé et de la Recherche Medicale, INSERM U955, FranceReviewed by:
Diane Wagner, Indiana University-Purdue University Indianapolis, United StatesCopyright © 2023 Josephson and Morgan. This is an open-access article distributed under the terms of the Creative Commons Attribution License (CC BY). The use, distribution or reproduction in other forums is permitted, provided the original author(s) and the copyright owner(s) are credited and that the original publication in this journal is cited, in accordance with accepted academic practice. No use, distribution or reproduction is permitted which does not comply with these terms.
*Correspondence: Timothy O. Josephson, dG9qQGJ1LmVkdQ==
Disclaimer: All claims expressed in this article are solely those of the authors and do not necessarily represent those of their affiliated organizations, or those of the publisher, the editors and the reviewers. Any product that may be evaluated in this article or claim that may be made by its manufacturer is not guaranteed or endorsed by the publisher.
Research integrity at Frontiers
Learn more about the work of our research integrity team to safeguard the quality of each article we publish.