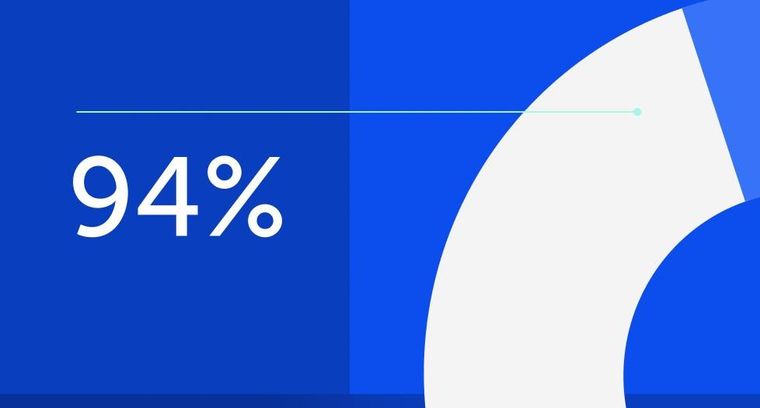
94% of researchers rate our articles as excellent or good
Learn more about the work of our research integrity team to safeguard the quality of each article we publish.
Find out more
REVIEW article
Front. Physiol., 04 July 2023
Sec. Renal Physiology and Pathophysiology
Volume 14 - 2023 | https://doi.org/10.3389/fphys.2023.1231621
This article is part of the Research TopicThe Immune System, Gut Microbiota, and Cross-Talk in Diabetic Kidney DiseaseView all 6 articles
With the increasing incidence of diabetes, diabetic kidney disease has become a major cause of chronic kidney disease. The role of the gut microbiota in diabetes and its related complications have been extensively investigated; the modulatory effect of the gut microbiota on the host depends on several gut microbial metabolites, particularly short-chain fatty acids, secondary bile acids, and trimethylamine N-oxide. In this review, we focused on the evidence related to the pathogenic role of each of the gut microbial metabolites in diabetic nephropathy. The main novel therapies targeting the gut microbiota include probiotics, dietary prebiotics, synbiotic supplements, and faecal microbiota transplants, although there is no standard treatment principle. Further research is therefore needed to elucidate the link between gut microbes and diabetic nephropathy, and more therapeutic targets should be explored to treat diabetic nephropathy with dysbiosis of the gut microbes.
Diabetes mellitus (DM) has become one of the fastest-growing public health problems in both developed and developing countries. According to the 2019 International Diabetes Federation statistics, 463 million people had diabetes in 2019, and 579 million are expected to have diabetes by 2030, and 700 million (51%) by 2045 (Saeedi et al., 2019).
Diabetic nephropathy (DN) is one of the most serious complications of diabetes, and it is also emerging as a major cause of chronic kidney disease (Raval et al., 2020). The pathogenesis of DN is complex and multifactorial, which involves multiple pathways and mediators (Pérez-Morales et al., 2019), including haemodynamic abnormalities, metabolic disturbances and hormone synthesis (Tavafi, 2013). The renin-angiotensin-aldosterone system (RAAS), the formation of advanced glycosylation end products (AGE), activation of transforming growth factor-β1 (TGF-β1), connective tissue growth factor (CTGF), protein kinase C (PKC), mitogen-activated protein kinases (MAPKs) and reactive oxygen species (ROS) are some of the important pathways in the development of DN. Each of these pathways contributes to disease progression through multiple mediators or by interacting with other pathways. For example, oxidative stress causes damages to Ang II, and conversely, RAAS causes damages to oxidative stress; Nicotinamide adenine phosphate dehydrogenase (NADPH) oxidase increases TGF-β, and conversely, TGF-β increases ROS through activation of NADPH oxidase (Samsu, 2021). Among these multifactorial pathogenesis, hyperglycemia is the main cause of DN development (VR et al., 2019). Genetic factors and environmental factors such as diet and lifestyle are widely recognised as contributing to diabetes and its complications, but there is also growing evidence for the involvement of gut microbes (Zhang et al., 2013; Sabatino et al., 2017); endotoxins, proteins, and metabolites from the gut microbiota are associated with kidney disease (Felizardo et al., 2019; Plata et al., 2019; Wu et al., 2020b; Wang et al., 2020), and interventions targeting these causative factors could be potential therapeutic targets to slow the progression of diabetic nephropathy.
In healthy populations, the genus Bacteroides and phylum thick-walled bacteria account for more than 90% of the intestinal flora, and the latter includes the genera Clostridium, Bacteroides, Eubacterium, Prevotella, Porphyromonas, Ruminococcaceae and Lactobacillus. In addition, other species of lower abundance include those belonging to Proteus species (Enterobacteriaceae, Helicobacter pylori, etc.), Actinobacteria (Bifidobacterium spp. and Corynebacterium aerogenes), Methanococcus, and Microphylum resveratrol (Arumugam et al., 2011). We have counted the intestinal microflora species studied in relation to diabetic nephropathy (Table 1). Some important pathogenic bacteria (e.g., Klebsiella pneumoniae, Bacteroides immobilis, Enterobacteriaceae spp., and Legionella spp.) proliferate abnormally in end-stage renal disease and DN (Vaziri et al., 2013; Kåhrström et al., 2016). In an animal study, researchers found that the intestinal flora of DN mice showed an abnormal ratio of thick-walled phylum/Bacteroides, that Heterobacteria and Bacillus anaerobicus may contribute to a decrease in glomerular filtration rate, and that Blautia spp. may be a protective factor in DN (Li Y. et al., 2020a). In a recent study, Chen et al. (2022) found that the DN group had the highest proportion of the thick-walled phylum, followed by the genera Bacteroides, Clostridium, and Proteus, whereas the non-DN group had the largest proportion of the genus Bacteroides, followed by thick-walled phylum, genus Proteus, and genus Clostridium. The main differences between the two groups were Odoribacter splanchnicus, Clostridium cellulolyticum rumen, Kleizzia adler, Lachnospiraceae NK4A136, Ruminococcaceae NK4A214, Aureobasidium spp., Biliophilus spp, UBA 1819, Ruminococcaceae UCG-004, Anaerobic spp., and Ruminococcaceae NK4A214. Among them, the O. splanchnicus, Clostridium decidua, Clostridium adelicum, and Lachnospiraceae NK4A136 groups were closely associated with short-chain fatty acid (SCFA) production (Chen et al., 2022). A study confirmed significant differences in the abundance of gut microbiota and changes in bacterial populations in the diabetic group compared to healthy controls and in the DN group compared to the diabetic group. This study also confirmed that Prevotella was more abundant in the gut microbiota of healthy controls than in those with diabetes and DN (Tao et al., 2019). The above findings imply that the abundance of certain flora species is higher in patients with DN than in non-DN patients. It is mainly caused by dysbiosis of the intestinal flora, which is manifested by an increase in the species and the number of conditioned pathogens.
An increasing number of studies have found that gut microbiota plays a key role in the development of DN (Wu et al., 2020a; Cai et al., 2022). In an animal study, the abundance of gut microbiota changed significantly between the DM and DN groups, particularly the relative abundance of the SCFA-producing bacteria Oncococcidae, Sarcococcidae, and Streptococcus lactis, and changes in the gut microbiota may play an important role in the progression of DN (Cai et al., 2022). The gut microbiota-derived metabolite phenyl sulfate caused proteinuria and foot cell damage in DN rats (Kikuchi et al., 2019). In a recent study, to assess the effect of gut barrier damage on renal injury in diabetic conditions, investigators induced DN in wild-type and mitochondrial antiviral signalling protein (MAVS)-knockout mice by unilateral nephrectomy and streptozotocin treatment and found that MAVS knockout diabetic mice exhibited more severe glomerular and tubular injury than wild-type diabetic mice. Studies have confirmed the role of MAVS signalling in maintaining the integrity of the intestinal barrier in patients with diabetes (Linh et al., 2022). These findings suggest that dysregulation of the intestinal microbiota is involved in the pathogenesis of DN.
It has been demonstrated that four phyla of lipopolysaccharide-producing Gram-negative bacteria, which includes the phyla Anaplasma, Aspergillus, Verruciformes, and Clostridium, have been identified as relatively abundant in the gut microbiota of diabetic nephropathy compared to the healthy group (Salguero et al., 2019). By comparing the metabolic profiles of the intestinal flora metabolites of different primary conditions in a study on chronic kidney disease, it was founded that more OTU values were detected in the microbiota of patients with diabetic nephropathy compared to the healthy population and hypertensive renal impairment, in which the relative abundance of Lactobacillus hominis and Lactobacillus mucosus was higher. Fusobacterium spp., Megasphaeralsdeni spp., Ruminococcus gnavus spp. and Lactobacillus spp. were also found able to correlate more precisely with L-Proline and Stearic acid were found able to differentiate diabetic nephropathy patients from healthy participants (Chen et al., 2023).
In recent years, host-intestinal flora interactions have become an integral part of host homeostasis. In the context of diabetic nephropathy, there is growing evidence to support a bidirectional microbiota-renal crosstalk, which is particularly evident during progressive renal dysfunction. Indeed, in diabetic nephropathy, the “healthy” microbiota structure is disrupted and gut microbes produce large amounts of uremic solutes, leading to renal damage; on the other hand, the uremic state leads to changes in microbial metabolism and composition due to reduced renal clearance, thus creating a vicious cycle in which In this vicious circle, ecological disorders and renal dysfunction will gradually worsen. Therefore, diabetic nephropathy and intestinal flora are mutually influential and causal.
Intestinal inflammation and epithelial barrier breakdown accelerate systemic translocation of the bacterial-derived uremic toxins including indoxyl sulphate, p-cresyl sulphate, and TMAO, and cause oxi-dative stress injury to the kidney, cardiovascular and endocrine systems (Chen et al., 2019). Glomerular filtration rate decreases with podocyte injury and death, disrupting the homeostasis of the gut microbiota as uremic toxins accumulate in the circulation, i.e., gut microbial dysbiosis (Plata et al., 2019). Subsequently, SCFA production decreases, whereas levels of typical uremic toxins such as p-cresol sulfate, indolyl sulfate, and trimethylamine N-oxide (TMAO) increase, ultimately worsening DN (Feng et al., 2021). In addition, changes in the intestinal microenvironment result in increased intestinal permeability, leading to increased secretion of bacterial components such as lipopolysaccharides (Potrykus et al., 2021). These endotoxins bind to signalling molecules such as toll-like receptors to recruit inflammatory cytokines and contribute to the development of chronic systemic inflammation (Ni et al., 2022) (Figure 1).
FIGURE 1. After the imbalance of intestinal flora in DN patients, the production of SCFA decreases and the level of TMAO increases. In addition, the increase of intestinal permeability leads to the increase of LPS permeability. Through different signal pathways, it leads to the thickening of renal basement membrane, podocyte and renal tubule damage, and promotes renal fibrosis.
SCFAs are one of the main metabolites of the microbially-mediated metabolism of fibre in the gut, leading to fermentation (He et al., 2020). It is a subset of fatty acids, containing six or fewer carbon molecules, and shows beneficial effects on the kidney. SCFAs play a role in bioregulation by inhibiting histone deacetylases (HDACs) and activating G protein receptors, thereby reducing the inflammatory response and lowering mean arterial pressure (Magliocca et al., 2022). SCFAs include acetate, propionate, and butyrate, which are produced by microbial communities through the fermentation of indigestible carbohydrates (Cong et al., 2022). Acetate and propionate are mainly produced by Bacteroidetes, whereas butyrate is mainly produced by Firmicutes (Levy et al., 2016).
The SCFA butyrate plays a positive role in the progression of diabetes and certain types of kidney disease (Machado et al., 2012; Guo et al., 2018; Xu et al., 2018), although its mechanism is not yet clear. Butyrate is suggested to alleviate DN by mediating the miR-7a-5p/P311/TGF-β1 pathway (Du et al., 2020). Transforming growth factor-β1 (TGF-β1) is the initial factor that triggers the fibrosis signalling cascade (Chang et al., 2016). P311 is an RNA-binding protein that stimulates TGF-β1 translation in several cell types (Yao et al., 2015). Butyrate supplementation attenuated kidney damage and apoptosis in a mouse model of genetic diabetes (db/db) as well as high glucose-induced fibrosis in SV40-MES-13 cells and inhibited TGF-β1 and P311 expression (Du et al., 2020). Oxidative stress is a key factor in the development of DN (Turkmen, 2017). The nuclear factor erythroid 2-related factor 2 (NRF2) plays a key role in cellular defence against oxidative stress (Kobayashi et al., 2016). Sodium butyrate (NaB), a known activator of NRF2, showed a DN-preventive effect (Zhou et al., 2022). A study that treated streptozotocin-induced diabetic C57BL/6Nrf2-knockout and the corresponding wild-type mice with NaB for 20 weeks found that diabetic mice not treated with NaB showed significant renal pathological changes such as oxidative damage, inflammation, apoptosis, fibrosis, and proteinuria. The results confirmed that NaB inhibited HDAC activity and increased the expression of NRF2 and its downstream targets, heme oxygenase 1 and NAD(P) H dehydrogenase quinone 1. Thus, NaB improved DN by activating NRF2 to inhibit HDAC activity (Dong et al., 2017). A recent study found anti-inflammatory effects of NaB on glomerular thylakoid cells in a mouse model of DN and high glucose-induced mouse glomerular mesangial cells (Gu et al., 2019). Gasdermin D (GSDMD) is a newly identified key executing protein for pyroptosis that is cleaved by inflammatory cysteine aspartase (Deng et al., 2021; Zuo et al., 2021). High glucose was found to increase propidium iodide (PI)-positive cells, promote the release of lactate dehydrogenase (LDH), interleukin-1 ß (IL-1β), and release of interleukin-18(IL-18); GSDMD, GSDMD N-terminal structural domain (GSDMD-n), and cleaved protein level caspase-1 levels were also elevated. NaB further enhanced the release of LDH by inhibiting caspase 1-GSDMD, which further enhanced LDH release and the number of PI-positive cells. In addition, NaB or Ac-YVAD-CMK, a caspase inhibitor, reversed the high glucose-induced nuclear factor-κB (NF-κB)/NF-κB inhibitor α (IκB-α) signalling pathway. Thus, NaB ameliorated high glucose-induced pyroptosis in glomerular endothelial cells via the caspase 1-GSDMD classical scorch death pathway involving the NF-κB/IκB-α signalling pathway. Exogenous NaB has been shown in different animal models to improve DN by reducing inflammation and oxidative stress as well as improving fibrosis, cell scorching, and DNA damage (Khan and Jena, 2014; Dong et al., 2017; Xu et al., 2018). Exogenous NaB also protected human glomerular thylakoid cells from high glucose-induced scorch death (Gu et al., 2019). These studies suggest that butyrate may serve as a potential therapeutic target for DN.
Whereas increasing evidence has indicated that butyrate may benefit the host through its anti-diabetic effects, some studies have suggested that acetate may exacerbate disease progression in DN. In a study using diabetic rats as a model, increased serum levels of acetate were found to be associated with increased expression of G protein-coupled receptor 43 (GPR43) in the kidney (Hu et al., 2020). Under high glucose conditions, acetate significantly increased the expression of GPR43 in human kidney 2 (HK-2) cells. Further analysis showed that GPR43 small interfering (si) RNA treatment significantly reduced lipid accumulation in HK-2 cells, even in the presence of acetate plus high glucose, which correlated with reduced protein levels of 3-hydroxy-3-methylglutaryl coenzyme A, low-density lipoprotein receptor, CD36, and chemokine ligand 16. This study confirmed that excess acetate production by the gut microbiota disrupted cholesterol homeostasis through the activation of GPR43, leading to tubulointerstitial damage in patients with DN Another animal study found that diabetic rats had abnormal gut flora, increased plasma acetate levels, increased urinary protein, thickened glomerular basement membranes, and loss of renal peduncle peduncles compared to controls (Lu et al., 2020). In addition, protein levels of angiotensin II, angiotensin-converting enzyme, and angiotensin II type 1 receptor were significantly increased in the kidneys of DM rats. The administration of broad-spectrum antibiotics to DM rats not only completely killed most intestinal flora but also significantly reduced plasma acetic acid levels, inhibited intrarenal activation of the renin-angiotensin (RAS) system (by inhibiting the aforementioned three indicators of RAS activation), and attenuated renal injury. This result suggests that dysregulation of the gut microbiota may be associated with intrarenal RAS activation in early DN. In contrast, plasma acetate levels positively correlated with intrarenal angiotensin II protein expression, inferring that acetate may also be involved in renal injury in early DN (Lu et al., 2020).
Acetic acid, propionic acid, and butyric acid are the main SCFA metabolites produced by gut microbes. However, several studies have focused on butyrate and acetate, and consequently, the potential effects of propionic acid on pathophysiological effects have been underestimated. There is a lack of robust research evidence on the effects of propionate on DN, although researchers have demonstrated that propionate can reduce fatty acid levels in the liver and plasma, reduce food intake, exert immunosuppressive effects, and possibly improve tissue insulin sensitivity (Al-Lahham et al., 2010; Pingitore et al., 2017; Yoshida et al., 2019). Propionate can reduce gluconeogenesis by activating AMP-activated protein kinase (AMPK) to downregulate glucose-6-phosphatase and phosphoenolpyruvate carboxykinase. In addition, siRNA-mediated knockdown of the propionate receptor GPR43 prevented propionate-induced AMPK activation and eliminated gluconeogenesis inhibition (Yoshida et al., 2019). A study group investigating the role of propionate in improving glucose homeostasis in vivo and its mechanism of action found that it enhanced glucose-stimulated insulin release and maintained ß-cell mass by inhibiting apoptosis (Pingitore et al., 2017). Propionate improves the high-glucose environment in patients with diabetes by modulating insulin sensitivity; thus, it is reasonable to infer that propionate has a positive impact on the progression of diabetic nephropathy.
TMAO is mainly derived from trimethylamine (TMA) oxidation by intestinal flora. Intestinal microorganisms metabolise and produce TMA from ingested nutrients such as lecithin and choline; these nutrients enter the liver via the portal circulation, where they are oxidised by flavin monooxygenase 3 or other flavin monooxygenases to produce TMAO (Bennett et al., 2013; Koeth et al., 2013). TMAO is a well-known pro-atherogenic sclerosing agent that promotes atherogenesis and is associated with cardiovascular risk (Bennett et al., 2013). Some studies have suggested that gut microbial dysbiosis contributes to diabetic microvascular damage, possibly because of the effects of changes in gut microbiota composition on atherosclerosis and endothelial dysfunction, but there is no conclusive evidence for this correlation (Roberts et al., 2018; Tanase et al., 2020). Evidence indicates that TMAO influences the progression of DN. In an experiment using streptozotocin-induced DN mice as a model, the mice were divided into mild proteinuria (MP) and severe proteinuria (SP) groups. The microbiota composition differed between the two groups, with a decrease in the abundance of thick-walled bacteria and a significant increase in heterobacteria in the SP group, and an increase in thick-walled bacteria in the MP group. This was associated with an increase in blood TMAO levels, which ultimately led to weight gain and increased glucose levels, accelerating the progression of DN. In addition, the lipopolysaccharide produced by the harmful bacteria led to a breakdown of the intestinal barrier, which allowed additional lipopolysaccharide and TMAO to enter the bloodstream and exacerbate renal damage (Li Y. et al., 2020a). In another animal study, TMAO exacerbated renal inflammation and promoted tubulointerstitial damage and renal fibrosis by activating NLRP3 inflammatory vesicles, thereby accelerating the development of DN (Fang et al., 2021b). A recent clinical trial also suggested that alternating gut microflora may be involved in the development of DN and that TMAO and chronic inflammation may be important factors in the development of DN (Yang et al., 2022).
Protein-bound uremic toxins such as indoxyl sulfate (IS), p-cresol sulfate (pCS), p-toluene glucosinolate (pCG) and phenyl sulfate are derived from the metabolism of aromatic amino acids, tyrosine, phenylalanine and tryptophan by intestinal microorganisms (Fang et al., 2021a). Escherichia coli, Proteus vulgaris, Paramecium coli, Bacillus liquefaciens, and Bacillus mimicus digest tryptophan to indole and metabolise it to IS in the liver (Keszthelyi et al., 2009). IS directly induces tubulointerstitial injury, oxidative stress and inflammation in post-gonadectomy mice (Ji et al., 2018; Tanaka et al., 2020) and human renal proximal tubular epithelial (HK-2) cells (Ellis et al., 2018), it has also been shown to be associated with the progression of DKD in patients with T1D and T2D, as well as in animal models of diabetes, and its elevation is mainly associated with changes in albuminuria and estimated glomerular filtration rate (Atoh et al., 2009; van der Kloet et al., 2012; Gooding et al., 2019).
Both pCS and pCG are derived from tyrosine, which is converted to p-cresol in the fermentation of Bacteroidetaceae, Bifidobacteria, Clostridia, Enterococcaceae, Eubacteriaceae, Clostridia, Lachnospiraceae, and Lactobacillariidae, and subsequently combined with sulphate or glucuronide to form pCS or pCG respectively (Russell et al., 2013). pCS can directly affect cell viability and induce cell death as well as induce reactive oxygen species and inflammatory cytokines in HK-2 cells (Watanabe et al., 2013; Park et al., 2019). pCG can cause phenotypic changes in proximal renal tubular cells (Mutsaers et al., 2015). These protein-bound uremic toxins are not only markers for the risk of DN development, but also risk factors that directly contribute to the development of DN. However, the molecular mechanisms of these urotoxins in DN are still unclear and needed further studies.
For the treatment of DN, glycaemic control is central and the use of hypoglycaemic drugs has been shown to have an impact on the gut microbiota. Metformin is a first-line hypoglycaemic drug that inhibits intestinal glucose uptake and hepatic glycogen output via AMPK-dependent and non-AMPK-dependent pathways and is widely used to reduce hyperglycaemia (Xourgia et al., 2019). Emerging evidence suggests that alterations in gut microbiota are associated with the antidiabetic effects of metformin in mucin-degrading bacteria in patients with type 2 diabetes (de la Cuesta-Zuluaga et al., 2017). An investigator improved glucose intolerance by transplanting gut microbiota from metformin-treated patients into germ-free mice (Wu et al., 2017). In rodent and human studies, metformin has altered diet-related transgenic components, leading to reduced microbial population diversity (Lee and Ko, 2014; Shin et al., 2014; de la Cuesta-Zuluaga et al., 2017).
Another class of hypoglycaemic agents alpha-glucosidase inhibitors, such as acarbose, is an oligosaccharide analogue produced by Acti noplanes, play an important role in reducing postprandial hyperglycaemia by competitively inhibiting alpha-glucosidase activity on the brush border of the small intestinal mucosa, and further reducing the absorption of monosaccharides by the intestinal epithelium (Mushtaq et al., 2023). The reduction in carbohydrate hydrolysis induced by acarbose may have altered microbial fermentation and further reduced lipopolysaccharide and inflammatory cytokines in type 2 diabetes (Su et al., 2015). In an animal study comparing the effects of metformin, acarbose and selegiline on intestinal microorganisms, alpha diversity analysis found that acarbose reduced microbial abundance and diversity. Acarbose selectively increased bacteria including rumenococci 2 spp. and bifidobacteria spp (Zhang et al., 2019).
Glucagon-like peptide-1 (GLP-1) is an enterostatin hormone that delays gastric motility, suppresses appetite, stimulates glucose-dependent insulin secretion and reduces glucagon secretion. Commonly used drugs include DPP-4 inhibitors (e.g., ligliptin, etc.) and GLP-1 receptor agonists (e.g., liraglutide, etc.) (Drucker, 2018). In a study on the effects of sitagliptin on the gut microbiota of rodents, it was found that sitagliptin partially reversed experimentally induced microecological dysbiosis, with an increase in the thick-walled and Tenericutes phylum, a decrease in the anthropoid phylum and a change in the population of SCFA-producing bacteria (Yan et al., 2016). In another animal study it was confirmed that similar results were obtained with vildagliptin treatment (Zhang et al., 2017). In an animal study, acute administration of the glucagon-like peptide-1 receptor agonist (GLP-1RA) liraglutide to mice increased levels of caseinolytic protease B (a component of E. coli) and norepinephrine (NE) in the cecum. The results of this study strongly suggest that GLP-1RA causes a surge of E. coli due to sympathetic activation receptor agonist on changes in the gut bacterium and the underlying mechanisms (Kato et al., 2021).
Sodium-glucose cotransporter protein (SGLT2) inhibitor that inhibits glucose reabsorption by inhibiting sodium-glucose cotransporter protein 2 in the proximal renal tubule (Heerspink et al., 2016). A study in diabetic mice, in which the gut microbial composition of the subjects was assessed by 16s rRNA sequencing, showed that diabetic mice treated with dagliflozin exhibited a favourable reduction in the ratio of the thick-walled phylum/Bacteroidetes compared to the diet alone group (Lee et al., 2018).
In an animal study, it was explored whether the effects of Sacubitril/Valsartan on DN were related to the gut microbiota and associated plasma metabolic profile. It was found that the Sac/Val intervention partially reshaped the composition of the ancient microbiota, reducing the abundance of some harmful bacteria and increasing the abundance of beneficial bacteria-in particular, the abundance of SCFAs-producing bacteria. This may be one of the mechanisms by which Sac/Val therapy reduces renal fibrosis. Furthermore, through functional prediction analysis, the study verified that Sac/Val not only reshaped the structure of the gut flora in DN patients, but also improved the function of the gut microbiome (Wang et al., 2022).
Although there are more research evidence on strong link between gut microbial dysbiosis and anti-diabetic therapeutic agents, the main effects produced by most antidiabetic drugs are a reduction in the thick-walled phylum/Bacteroides phylum ratio and an increase in the metabolites of the gut microbial fraction. However, more studies are needed to confirm the mechanism of action of antidiabetic drugs, especially the newer drugs (DPP-4 inhibitors, GLP-1 receptor agonists, sodium-glucose cotransport protein (SGLT2) inhibitors) on intestinal microbes.
DN is one of the most serious complications of diabetes and can lead to end-stage renal failure and disability. Changes in the gut microbiota are usually manifested by an increase in the proportion of multiple pathogenic bacteria and a decrease in the proportion of probiotic bacteria (Kikuchi et al., 2019; Tao et al., 2019). Given the serious health implications and economic burden associated with diabetic nephropathy, it is particularly important to find effective interventions to slow its progression. Novel therapies are currently focused on restoring a balanced intestinal environment (ecosystem) using probiotics, dietary prebiotics, and synbiotic supplements (Lopes et al., 2018) (Table 2).
In a systematic review and meta-analysis, probiotics were shown to have beneficial effects on metabolic indicators in patients with DN, including renal function, glucose homeostasis, lipid metabolism, inflammation, and oxidative stress. Probiotics may be an effective and low-cost treatment for patients with DN (Dai et al., 2022). In a study assessing the effects of oral single-strain or multi-strain probiotic preparations on high-fat diet-induced obesity in mice, the separation between the ability of probiotics to suppress adipose tissue inflammation and limit weight gain was reported. The multi-strain mixture was able to improve fat deposition, insulin resistance, and dyslipidaemia through adipose tissue immune cell remodelling (mainly affecting macrophages). The mixture altered fatty acid uptake at the intestinal level and restored the expression levels of the SCFA receptor GPR43 (Alard et al., 2016). In another clinical trial, it was found that, compared with placebo, 12 weeks after the intake of probiotic supplements, the significantly reduced expression of hs CRP in serum, malondialdehyde (MDA), AGEs, urea, creatinine and IL-1 genes and the significantly increased concentration of glutathione (GSH) in plasma in patients with diabetes nephropathy were related, but did not affect other markers of inflammation and oxidative stress, as well as TNF- α And TGF- ß Gene expression (Mafi et al., 2018). Probiotic intake can help reduce inflammatory factors through SCFAs produced by the gut microbes and reduce the production of hydrogen peroxide radicals (March et al., 2017; Lopes et al., 2018). The beneficial effects of probiotics on oxidative damage may be mediated through the production of butyrate in follicles and the reduction of lipid peroxidation (Jiang et al., 2016). In an animal experiment, diabetic rats treated with Lactobacillus plantarum or Lactobacillus rhamnosus-fermented yoghurt showed the following effects: a significant reduction in blood glucose and α-amylase concentrations; a significant increase in high-density lipoprotein cholesterol concentration and improved lipid distribution; and inhibition of nuclear factor κB and increase in Bcl-2 concentrations (Negm El-Dein et al., 2022). This study conclusively established that the selected Lactobacillus spp. had hypoglycaemic potential and could be used as functional nutritional anti-diabetic supplements.
Of all the exogenous factors affecting the gut microbiome, a long-term diet appears to have, by far, the greatest impact (Xu and Knight, 2015), and it has attracted attention as a therapeutic route to re-establishing a symbiotic relationship. As hyperglycaemia is the main cause of the development of DN (VR et al., 2019), glycaemic control is the cornerstone of DN treatment. Several studies have suggested that dietary prebiotic or symbiotic supplements may modulate glucose metabolism through the modification of intestinal flora, thereby slowing the progression of diabetic complications (Nikbakht et al., 2018; Carvalho et al., 2019; Bock et al., 2021).
There are various types of prebiotics, including oligosaccharides, polysaccharides (e.g., Spirulina, Chlorella, etc.), some natural plant extracts (e.g., vegetables, herbs, wild plants, etc.), protein hydrolysates, polyols, etc. (Yadav et al., 2022). Oligofructose (FOS), a widely commercially available source of prebiotics, has also been shown to improve intestinal disorders and reduce inflammation in patients with diabetes (Pengrattanachot et al., 2022). In animal experiments using rats with type 1 diabetes induced by streptozotocin, FOS showed a protective effect on the kidneys and improvd inflammation and insulin sensitivity (Gobinath et al., 2010). Dietary fibre is a polysaccharide that modulates gut microbiota composition (Cronin et al., 2021). In another animal study, dietary fibre was found to protect mice from the clinical and histological manifestations of DN without altering blood glucose levels. Supplementation with the microbial-derived acetate and butyrate provided similar protection by binding to the metabolite-sensing receptors GPR43 and GPR109A. This study reveals a role for the gut microbiota in attempting to delay the progression of DN through dietary modulation, with SCFA being a key mediator of the protective effect produced by fibre depletion (Li Y. J. et al., 2020b).
Faecal microbiota transplantation (FMT) may affect gut microbiota composition (Wang et al., 2019). Its success depends largely on the diversity and composition of donor faecal microbes (Zheng et al., 2022). FMT is a new theory and promising technique for the treatment of intestinal microbiome disorders. However, its application is still greatly limited by the risk of disease transmission between donor and recipient, patient acceptance, poor outcomes, and the uncertain impact on the recipient’s immune system (Wang et al., 2019). FMT is a successful treatment for recurrent Clostridium difficile infection and is more commonly used in studies of inflammatory bowel disease (Imdad et al., 2018). However, it is now also increasingly being applied in extraintestinal diseases, including haematological disorders, neurological disorders, and metabolic diseases such as diabetes and autoimmune diseases, and has shown some positive results (Wang et al., 2019). In a mouse model of experimental DN, the potential role of gut microbiota was hypothesized to involve renal function regulation, which was validated by the role of the FMT gut microbiome and SCFA produced by it in the treatment of DN (Li Y. et al., 2020a). In addition, Faecalibacterium prausnitzi can be used as a diagnostic and therapeutic biomarker for FMT (Chen et al., 2020; Zhang et al., 2020). The gut structure can be restored by transplanting F. prausnitzii, which could be used as a potential treatment against inflammation and diabetes (Ganesan et al., 2018; Xu et al., 2020). Other studies have also demonstrated that FMT from healthy donors significantly reduced foot cell damage in diabetic rats, thereby delaying kidney damage (Lu et al., 2021).
There is currently a growing interest in the complex relationship between gut microbes and disease, and an increasing number of researchers are focusing on the area of gut flora and kidney disease, particularly the impact of metabolites of gut microbes on the progression of diabetic nephropathy. We know that the gut microbiota is a regulator of glucose and lipid metabolism and immune inflammation, acting as a link between host and environmental influences. The composition of the gut microbiota of patients with DN differs from that of the healthy population. Studies have demonstrated the relevance of metabolites following gut microbial dysbiosis to the progression of DN. Metabolites such as short-chain fatty acids (SCFA), bile acids (BA) and uremic toxins (TMAO) cause damage to the renal tubules through different signalling pathways, promote renal fibrosis and influence the progression of DN. Although supplementation with probiotics, dietary prebiotics, synbiotic supplements and faecal microbiota transplantation can prevent the progression of DN, improve blood glucose levels, maintain the stability of the body’s environment and reduce the inflammatory response, thus help to improve the quality of life of this group of patients. However, we need to know more about the relationship between gut microbial metabolites and DN to identify additional therapeutic targets to slow the progression of diabetic nephropathy by improving gut microbial dysregulation.
ET and JY wrote the first draft. FW, LZ, and YS reviewed and finalized the content of the manuscript. All authors contributed to the article and approved the submitted version.
This research was funded by the National Natural Science Foundation of China (No. 82270723), and the Chongqing Talent Program Project (cstc2021ycjh-bgzxm0090).
The authors declare that the research was conducted in the absence of any commercial or financial relationships that could be construed as a potential conflict of interest.
All claims expressed in this article are solely those of the authors and do not necessarily represent those of their affiliated organizations, or those of the publisher, the editors and the reviewers. Any product that may be evaluated in this article, or claim that may be made by its manufacturer, is not guaranteed or endorsed by the publisher.
Al-Lahham, S. H., Peppelenbosch, M. P., Roelofsen, H., Vonk, R. J., and Venema, K. (2010). Biological effects of propionic acid in humans; metabolism, potential applications and underlying mechanisms. Biochim. Biophys. Acta 1801 (11), 1175–1183. doi:10.1016/j.bbalip.2010.07.007
Alard, J., Lehrter, V., Rhimi, M., Mangin, I., Peucelle, V., Abraham, A. L., et al. (2016). Beneficial metabolic effects of selected probiotics on diet-induced obesity and insulin resistance in mice are associated with improvement of dysbiotic gut microbiota. Environ. Microbiol. 18 (5), 1484–1497. doi:10.1111/1462-2920.13181
Arumugam, M., Raes, J., Pelletier, E., Le Paslier, D., Yamada, T., Mende, D. R., et al. (2011). Enterotypes of the human gut microbiome. Nature 473 (7346), 174–180. doi:10.1038/nature09944
Atoh, K., Itoh, H., and Haneda, M. (2009). Serum indoxyl sulfate levels in patients with diabetic nephropathy: Relation to renal function. Diabetes Res. Clin. Pract. 83 (2), 220–226. doi:10.1016/j.diabres.2008.09.053
Bennett, B. J., de Aguiar Vallim, T. Q., Wang, Z., Shih, D. M., Meng, Y., Gregory, J., et al. (2013). Trimethylamine-N-oxide, a metabolite associated with atherosclerosis, exhibits complex genetic and dietary regulation. Cell Metab. 17 (1), 49–60. doi:10.1016/j.cmet.2012.12.011
Bock, P. M., Telo, G. H., Ramalho, R., Sbaraini, M., Leivas, G., Martins, A. F., et al. (2021). The effect of probiotics, prebiotics or synbiotics on metabolic outcomes in individuals with diabetes: A systematic review and meta-analysis. Diabetologia 64 (1), 26–41. doi:10.1007/s00125-020-05295-1
Cai, K., Ma, Y., Cai, F., Huang, X., Xiao, L., Zhong, C., et al. (2022). Changes of gut microbiota in diabetic nephropathy and its effect on the progression of kidney injury. Endocrine 76 (2), 294–303. doi:10.1007/s12020-022-03002-1
Carvalho, C. M., Gross, L. A., de Azevedo, M. J., and Viana, L. V. (2019). Dietary fiber intake (supplemental or dietary pattern rich in fiber) and diabetic kidney disease: A systematic review of clinical trials. Nutrients 11 (2), 347. doi:10.3390/nu11020347
Chang, A. S., Hathaway, C. K., Smithies, O., and Kakoki, M. (2016). Transforming growth factor-β1 and diabetic nephropathy. Am. J. Physiol. Ren. Physiol. 310 (8), F689–f696. doi:10.1152/ajprenal.00502.2015
Chen, H. T., Huang, H. L., Xu, H. M., Luo, Q. L., He, J., Li, Y. Q., et al. (2020). Fecal microbiota transplantation ameliorates active ulcerative colitis. Exp. Ther. Med. 19 (4), 2650–2660. doi:10.3892/etm.2020.8512
Chen, R., Zhu, D., Yang, R., Wu, Z., Xu, N., Chen, F., et al. (2022). Gut microbiota diversity in middle-aged and elderly patients with end-stage diabetic kidney disease. Ann. Transl. Med. 10 (13), 750. doi:10.21037/atm-22-2926
Chen, T. H., Cheng, C. Y., Huang, C. K., Ho, Y. H., and Lin, J. C. (2023). Exploring the relevance between gut microbiota-metabolites profile and chronic kidney disease with distinct pathogenic factor. Microbiol. Spectr. 11 (1), e0280522. doi:10.1128/spectrum.02805-22
Chen, Y. Y., Chen, D. Q., Chen, L., Liu, J. R., Vaziri, N. D., Guo, Y., et al. (2019). Microbiome-metabolome reveals the contribution of gut-kidney axis on kidney disease. J. Transl. Med. 17 (1), 5. doi:10.1186/s12967-018-1756-4
Cong, J., Zhou, P., and Zhang, R. (2022). Intestinal microbiota-derived short chain fatty acids in host health and disease. Nutrients 14 (9), 1977. doi:10.3390/nu14091977
Cronin, P., Joyce, S. A., O'Toole, P. W., and O'Connor, E. M. (2021). Dietary fibre modulates the gut microbiota. Nutrients 13 (5), 1655. doi:10.3390/nu13051655
Dai, Y., Quan, J., Xiong, L., Luo, Y., and Yi, B. (2022). Probiotics improve renal function, glucose, lipids, inflammation and oxidative stress in diabetic kidney disease: A systematic review and meta-analysis. Ren. Fail 44 (1), 862–880. doi:10.1080/0886022x.2022.2079522
de la Cuesta-Zuluaga, J., Mueller, N. T., Corrales-Agudelo, V., Velásquez-Mejía, E. P., Carmona, J. A., Abad, J. M., et al. (2017). Metformin is associated with higher relative abundance of mucin-degrading akkermansia muciniphila and several short-chain fatty acid-producing microbiota in the gut. Diabetes Care 40 (1), 54–62. doi:10.2337/dc16-1324
Deng, J., Tan, W., Luo, Q., Lin, L., Zheng, L., and Yang, J. (2021). Long non-coding RNA MEG3 promotes renal tubular epithelial cell pyroptosis by regulating the miR-18a-3p/GSDMD pathway in lipopolysaccharide-induced acute kidney injury. Front. Physiol. 12, 663216. doi:10.3389/fphys.2021.663216
Dong, W., Jia, Y., Liu, X., Zhang, H., Li, T., Huang, W., et al. (2017). Sodium butyrate activates NRF2 to ameliorate diabetic nephropathy possibly via inhibition of HDAC. J. Endocrinol. 232 (1), 71–83. doi:10.1530/joe-16-0322
Drucker, D. J. (2018). Mechanisms of action and therapeutic application of glucagon-like peptide-1. Cell Metab. 27 (4), 740–756. doi:10.1016/j.cmet.2018.03.001
Du, Y., Yang, Y. T., Tang, G., Jia, J. S., Zhu, N., and Yuan, W. J. (2020). Butyrate alleviates diabetic kidney disease by mediating the miR-7a-5p/P311/TGF-β1 pathway. Faseb J. 34 (8), 10462–10475. doi:10.1096/fj.202000431R
Ellis, R. J., Small, D. M., Ng, K. L., Vesey, D. A., Vitetta, L., Francis, R. S., et al. (2018). Indoxyl sulfate induces apoptosis and hypertrophy in human kidney proximal tubular cells. Toxicol. Pathol. 46 (4), 449–459. doi:10.1177/0192623318768171
Fang, Q., Liu, N., Zheng, B., Guo, F., Zeng, X., Huang, X., et al. (2021a). Roles of gut microbial metabolites in diabetic kidney disease. Front. Endocrinol. (Lausanne) 12, 636175. doi:10.3389/fendo.2021.636175
Fang, Q., Zheng, B., Liu, N., Liu, J., Liu, W., Huang, X., et al. (2021b). Trimethylamine N-oxide exacerbates renal inflammation and fibrosis in rats with diabetic kidney disease. Front. Physiol. 12, 682482. doi:10.3389/fphys.2021.682482
Felizardo, R. J. F., Watanabe, I. K. M., Dardi, P., Rossoni, L. V., and Câmara, N. O. S. (2019). The interplay among gut microbiota, hypertension and kidney diseases: The role of short-chain fatty acids. Pharmacol. Res. 141, 366–377. doi:10.1016/j.phrs.2019.01.019
Feng, Z., Wang, T., Dong, S., Jiang, H., Zhang, J., Raza, H. K., et al. (2021). Association between gut dysbiosis and chronic kidney disease: A narrative review of the literature. J. Int. Med. Res. 49 (10), 3000605211053276. doi:10.1177/03000605211053276
Ganesan, K., Chung, S. K., Vanamala, J., and Xu, B. (2018). Causal relationship between diet-induced gut microbiota changes and diabetes: A novel strategy to transplant Faecalibacterium prausnitzii in preventing diabetes. Int. J. Mol. Sci. 19 (12), 3720. doi:10.3390/ijms19123720
Gobinath, D., Madhu, A. N., Prashant, G., Srinivasan, K., and Prapulla, S. G. (2010). Beneficial effect of xylo-oligosaccharides and fructo-oligosaccharides in streptozotocin-induced diabetic rats. Br. J. Nutr. 104 (1), 40–47. doi:10.1017/s0007114510000243
Gooding, J., Cao, L., Whitaker, C., Mwiza, J. M., Fernander, M., Ahmed, F., et al. (2019). Meprin β metalloproteases associated with differential metabolite profiles in the plasma and urine of mice with type 1 diabetes and diabetic nephropathy. BMC Nephrol. 20 (1), 141. doi:10.1186/s12882-019-1313-2
Gu, J., Huang, W., Zhang, W., Zhao, T., Gao, C., Gan, W., et al. (2019). Sodium butyrate alleviates high-glucose-induced renal glomerular endothelial cells damage via inhibiting pyroptosis. Int. Immunopharmacol. 75, 105832. doi:10.1016/j.intimp.2019.105832
Guo, Y., Xiao, Z., Wang, Y., Yao, W., Liao, S., Yu, B., et al. (2018). Sodium butyrate ameliorates streptozotocin-induced type 1 diabetes in mice by inhibiting the HMGB1 expression. Front. Endocrinol. (Lausanne) 9, 630. doi:10.3389/fendo.2018.00630
He, J., Zhang, P., Shen, L., Niu, L., Tan, Y., Chen, L., et al. (2020). Short-chain fatty acids and their association with signalling pathways in inflammation, glucose and lipid metabolism. Int. J. Mol. Sci. 21 (17), 6356. doi:10.3390/ijms21176356
Heerspink, H. J., Perkins, B. A., Fitchett, D. H., Husain, M., and Cherney, D. Z. (2016). Sodium glucose cotransporter 2 inhibitors in the treatment of diabetes mellitus: Cardiovascular and kidney effects, potential mechanisms, and clinical applications. Circulation 134 (10), 752–772. doi:10.1161/circulationaha.116.021887
Hu, Z. B., Lu, J., Chen, P. P., Lu, C. C., Zhang, J. X., Li, X. Q., et al. (2020). Dysbiosis of intestinal microbiota mediates tubulointerstitial injury in diabetic nephropathy via the disruption of cholesterol homeostasis. Theranostics 10 (6), 2803–2816. doi:10.7150/thno.40571
Imdad, A., Nicholson, M. R., Tanner-Smith, E. E., Zackular, J. P., Gomez-Duarte, O. G., Beaulieu, D. B., et al. (2018). Fecal transplantation for treatment of inflammatory bowel disease. Cochrane Database Syst. Rev. 11 (11), Cd012774. doi:10.1002/14651858.CD012774.pub2
Ji, C., Luo, Y., Zou, C., Huang, L., Tian, R., and Lu, Z. (2018). Effect of astragaloside IV on indoxyl sulfate-induced kidney injury in mice via attenuation of oxidative stress. BMC Pharmacol. Toxicol. 19 (1), 53. doi:10.1186/s40360-018-0241-2
Jiang, H., Zhang, Y., Xu, D., and Wang, Q. (2021). Probiotics ameliorates glycemic control of patients with diabetic nephropathy: A randomized clinical study. J. Clin. Lab. Anal. 35 (4), e23650. doi:10.1002/jcla.23650
Jiang, S., Xie, S., Lv, D., Zhang, Y., Deng, J., Zeng, L., et al. (2016). A reduction in the butyrate producing species Roseburia spp. and Faecalibacterium prausnitzii is associated with chronic kidney disease progression. Ant. Van Leeuwenhoek 109 (10), 1389–1396. doi:10.1007/s10482-016-0737-y
Kåhrström, C. T., Pariente, N., and Weiss, U. (2016). Intestinal microbiota in health and disease. Nature 535 (7610), 47. doi:10.1038/535047a
Kato, S., Sato, T., Fujita, H., Kawatani, M., and Yamada, Y. (2021). Effects of GLP-1 receptor agonist on changes in the gut bacterium and the underlying mechanisms. Sci. Rep. 11 (1), 9167. doi:10.1038/s41598-021-88612-x
Keszthelyi, D., Troost, F. J., and Masclee, A. A. (2009). Understanding the role of tryptophan and serotonin metabolism in gastrointestinal function. Neurogastroenterol. Motil. 21 (12), 1239–1249. doi:10.1111/j.1365-2982.2009.01370.x
Khan, S., and Jena, G. (2014). Sodium butyrate, a HDAC inhibitor ameliorates eNOS, iNOS and TGF-β1-induced fibrogenesis, apoptosis and DNA damage in the kidney of juvenile diabetic rats. Food Chem. Toxicol. 73, 127–139. doi:10.1016/j.fct.2014.08.010
Kikuchi, K., Saigusa, D., Kanemitsu, Y., Matsumoto, Y., Thanai, P., Suzuki, N., et al. (2019). Gut microbiome-derived phenyl sulfate contributes to albuminuria in diabetic kidney disease. Nat. Commun. 10 (1), 1835. doi:10.1038/s41467-019-09735-4
Kobayashi, E. H., Suzuki, T., Funayama, R., Nagashima, T., Hayashi, M., Sekine, H., et al. (2016). Nrf2 suppresses macrophage inflammatory response by blocking proinflammatory cytokine transcription. Nat. Commun. 7, 11624. doi:10.1038/ncomms11624
Koeth, R. A., Wang, Z., Levison, B. S., Buffa, J. A., Org, E., Sheehy, B. T., et al. (2013). Intestinal microbiota metabolism of L-carnitine, a nutrient in red meat, promotes atherosclerosis. Nat. Med. 19 (5), 576–585. doi:10.1038/nm.3145
Lee, D. M., Battson, M. L., Jarrell, D. K., Hou, S., Ecton, K. E., Weir, T. L., et al. (2018). SGLT2 inhibition via dapagliflozin improves generalized vascular dysfunction and alters the gut microbiota in type 2 diabetic mice. Cardiovasc Diabetol. 17 (1), 62. doi:10.1186/s12933-018-0708-x
Lee, H., and Ko, G. (2014). Effect of metformin on metabolic improvement and gut microbiota. Appl. Environ. Microbiol. 80 (19), 5935–5943. doi:10.1128/aem.01357-14
Levy, M., Thaiss, C. A., and Elinav, E. (2016). Metabolites: Messengers between the microbiota and the immune system. Genes Dev. 30 (14), 1589–1597. doi:10.1101/gad.284091.116
Li, Y., Su, X., Gao, Y., Lv, C., Gao, Z., Liu, Y., et al. (2020a). The potential role of the gut microbiota in modulating renal function in experimental diabetic nephropathy murine models established in same environment. Biochim. Biophys. Acta Mol. Basis Dis. 1866 (6), 165764. doi:10.1016/j.bbadis.2020.165764
Li, Y. J., Chen, X., Kwan, T. K., Loh, Y. W., Singer, J., Liu, Y., et al. (2020b). Dietary fiber protects against diabetic nephropathy through short-chain fatty acid-mediated activation of G protein-coupled receptors GPR43 and GPR109A. J. Am. Soc. Nephrol. 31 (6), 1267–1281. doi:10.1681/asn.2019101029
Linh, H. T., Iwata, Y., Senda, Y., Sakai-Takemori, Y., Nakade, Y., Oshima, M., et al. (2022). Intestinal bacterial translocation contributes to diabetic kidney disease. J. Am. Soc. Nephrol. 33 (6), 1105–1119. doi:10.1681/asn.2021060843
Lopes, R., Balbino, K. P., Jorge, M. P., Ribeiro, A. Q., Martino, H. S. D., and Alfenas, R. C. G. (2018). Modulation of intestinal microbiota, control of nitrogen products and inflammation by pre/probiotics in chronic kidney disease: A systematic review. Nutr. Hosp. 35 (3), 722–730. doi:10.20960/nh.1642
Lu, C. C., Hu, Z. B., Wang, R., Hong, Z. H., Lu, J., Chen, P. P., et al. (2020). Gut microbiota dysbiosis-induced activation of the intrarenal renin-angiotensin system is involved in kidney injuries in rat diabetic nephropathy. Acta Pharmacol. Sin. 41 (8), 1111–1118. doi:10.1038/s41401-019-0326-5
Lu, J., Chen, P. P., Zhang, J. X., Li, X. Q., Wang, G. H., Yuan, B. Y., et al. (2021). GPR43 deficiency protects against podocyte insulin resistance in diabetic nephropathy through the restoration of AMPKα activity. Theranostics 11 (10), 4728–4742. doi:10.7150/thno.56598
Machado, R. A., Constantino Lde, S., Tomasi, C. D., Rojas, H. A., Vuolo, F. S., Vitto, M. F., et al. (2012). Sodium butyrate decreases the activation of NF-κB reducing inflammation and oxidative damage in the kidney of rats subjected to contrast-induced nephropathy. Nephrol. Dial. Transpl. 27 (8), 3136–3140. doi:10.1093/ndt/gfr807
Mafi, A., Namazi, G., Soleimani, A., Bahmani, F., Aghadavod, E., and Asemi, Z. (2018). Metabolic and genetic response to probiotics supplementation in patients with diabetic nephropathy: A randomized, double-blind, placebo-controlled trial. Food Funct. 9 (9), 4763–4770. doi:10.1039/c8fo00888d
Magliocca, G., Mone, P., Di Iorio, B. R., Heidland, A., and Marzocco, S. (2022). Short-chain fatty acids in chronic kidney disease: Focus on inflammation and oxidative stress regulation. Int. J. Mol. Sci. 23 (10), 5354. doi:10.3390/ijms23105354
March, D. S., Graham-Brown, M. P., Stover, C. M., Bishop, N. C., and Burton, J. O. (2017). Intestinal barrier disturbances in haemodialysis patients: Mechanisms, consequences, and therapeutic options. Biomed. Res. Int. 2017, 5765417. doi:10.1155/2017/5765417
Mazruei Arani, N., Emam-Djomeh, Z., Tavakolipour, H., Sharafati-Chaleshtori, R., Soleimani, A., and Asemi, Z. (2019). The effects of probiotic honey consumption on metabolic status in patients with diabetic nephropathy: A randomized, double-blind, controlled trial. Probiotics Antimicrob. Proteins 11 (4), 1195–1201. doi:10.1007/s12602-018-9468-x
Miraghajani, M., Zaghian, N., Mirlohi, M., Feizi, A., and Ghiasvand, R. (2017). The impact of probiotic soy milk consumption on oxidative stress among type 2 diabetic kidney disease patients: A randomized controlled clinical trial. J. Ren. Nutr. 27 (5), 317–324. doi:10.1053/j.jrn.2017.04.004
Mushtaq, A., Azam, U., Mehreen, S., and Naseer, M. M. (2023). Synthetic α-glucosidase inhibitors as promising anti-diabetic agents: Recent developments and future challenges. Eur. J. Med. Chem. 249, 115119. doi:10.1016/j.ejmech.2023.115119
Mutsaers, H. A., Caetano-Pinto, P., Seegers, A. E., Dankers, A. C., van den Broek, P. H., Wetzels, J. F., et al. (2015). Proximal tubular efflux transporters involved in renal excretion of p-cresyl sulfate and p-cresyl glucuronide: Implications for chronic kidney disease pathophysiology. Toxicol Vitro 29 (7), 1868–1877. doi:10.1016/j.tiv.2015.07.020
Negm El-Dein, A., Ezzat, A., Aly, H. F., Awad, G., and Farid, M. (2022). Lactobacillus-fermented yogurt exerts hypoglycemic, hypocholesterolemic, and anti-inflammatory activities in STZ-induced diabetic Wistar rats. Nutr. Res. 108, 22–32. doi:10.1016/j.nutres.2022.10.003
Ni, Y., Zheng, L., Nan, S., Ke, L., Fu, Z., and Jin, J. (2022). Enterorenal crosstalks in diabetic nephropathy and novel therapeutics targeting the gut microbiota. Acta Biochim. Biophys. Sin. (Shanghai) 54 (10), 1406–1420. doi:10.3724/abbs.2022140
Nikbakht, E., Khalesi, S., Singh, I., Williams, L. T., West, N. P., and Colson, N. (2018). Effect of probiotics and synbiotics on blood glucose: A systematic review and meta-analysis of controlled trials. Eur. J. Nutr. 57 (1), 95–106. doi:10.1007/s00394-016-1300-3
Park, J. S., Choi, H. I., Kim, D. H., Kim, C. S., Bae, E. H., Ma, S. K., et al. (2019). Alpha-lipoic acid attenuates p-cresyl sulfate-induced renal tubular injury through suppression of apoptosis and autophagy in human proximal tubular epithelial cells. Biomed. Pharmacother. 112, 108679. doi:10.1016/j.biopha.2019.108679
Pengrattanachot, N., Thongnak, L., and Lungkaphin, A. (2022). The impact of prebiotic fructooligosaccharides on gut dysbiosis and inflammation in obesity and diabetes related kidney disease. Food Funct. 13 (11), 5925–5945. doi:10.1039/d1fo04428a
Pérez-Morales, R. E., Del Pino, M. D., Valdivielso, J. M., Ortiz, A., Mora-Fernández, C., and Navarro-González, J. F. (2019). Inflammation in diabetic kidney disease. Nephron 143 (1), 12–16. doi:10.1159/000493278
Pingitore, A., Chambers, E. S., Hill, T., Maldonado, I. R., Liu, B., Bewick, G., et al. (2017). The diet-derived short chain fatty acid propionate improves beta-cell function in humans and stimulates insulin secretion from human islets in vitro. Diabetes Obes. Metab. 19 (2), 257–265. doi:10.1111/dom.12811
Plata, C., Cruz, C., Cervantes, L. G., and Ramírez, V. (2019). The gut microbiota and its relationship with chronic kidney disease. Int. Urol. Nephrol. 51 (12), 2209–2226. doi:10.1007/s11255-019-02291-2
Potrykus, M., Czaja-Stolc, S., Stankiewicz, M., Kaska, Ł., and Małgorzewicz, S. (2021). Intestinal microbiota as a contributor to chronic inflammation and its potential modifications. Nutrients 13 (11), 3839. doi:10.3390/nu13113839
Raval, N., Kumawat, A., Kalyane, D., Kalia, K., and Tekade, R. K. (2020). Understanding molecular upsets in diabetic nephropathy to identify novel targets and treatment opportunities. Drug Discov. Today 25 (5), 862–878. doi:10.1016/j.drudis.2020.01.008
Roberts, A. B., Gu, X., Buffa, J. A., Hurd, A. G., Wang, Z., Zhu, W., et al. (2018). Development of a gut microbe-targeted nonlethal therapeutic to inhibit thrombosis potential. Nat. Med. 24 (9), 1407–1417. doi:10.1038/s41591-018-0128-1
Russell, W. R., Duncan, S. H., Scobbie, L., Duncan, G., Cantlay, L., Calder, A. G., et al. (2013). Major phenylpropanoid-derived metabolites in the human gut can arise from microbial fermentation of protein. Mol. Nutr. Food Res. 57 (3), 523–535. doi:10.1002/mnfr.201200594
Sabatino, A., Regolisti, G., Cosola, C., Gesualdo, L., and Fiaccadori, E. (2017). Intestinal microbiota in type 2 diabetes and chronic kidney disease. Curr. Diab Rep. 17 (3), 16. doi:10.1007/s11892-017-0841-z
Saeedi, P., Petersohn, I., Salpea, P., Malanda, B., Karuranga, S., Unwin, N., et al. (2019). Global and regional diabetes prevalence estimates for 2019 and projections for 2030 and 2045: Results from the international diabetes federation diabetes atlas, 9th edition, Diabetes Res. Clin. Pract. 157, 107843. doi:10.1016/j.diabres.2019.107843
Salguero, M. V., Al-Obaide, M. A. I., Singh, R., Siepmann, T., and Vasylyeva, T. L. (2019). Dysbiosis of Gram-negative gut microbiota and the associated serum lipopolysaccharide exacerbates inflammation in type 2 diabetic patients with chronic kidney disease. Exp. Ther. Med. 18 (5), 3461–3469. doi:10.3892/etm.2019.7943
Samsu, N. (2021). Diabetic nephropathy: Challenges in pathogenesis, diagnosis, and treatment. Biomed. Res. Int. 2021, 1497449. doi:10.1155/2021/1497449
Shang, J., Cui, W., Guo, R., Zhang, Y., Wang, P., Yu, W., et al. (2022). The harmful intestinal microbial community accumulates during DKD exacerbation and microbiome-metabolome combined validation in a mouse model. Front. Endocrinol. (Lausanne) 13, 964389. doi:10.3389/fendo.2022.964389
Shin, N. R., Lee, J. C., Lee, H. Y., Kim, M. S., Whon, T. W., Lee, M. S., et al. (2014). An increase in the Akkermansia spp. population induced by metformin treatment improves glucose homeostasis in diet-induced obese mice. Gut 63 (5), 727–735. doi:10.1136/gutjnl-2012-303839
Su, B., Liu, H., Li, J., Sunli, Y., Liu, B., Liu, D., et al. (2015). Acarbose treatment affects the serum levels of inflammatory cytokines and the gut content of bifidobacteria in Chinese patients with type 2 diabetes mellitus. J. Diabetes 7 (5), 729–739. doi:10.1111/1753-0407.12232
Tanaka, S., Watanabe, H., Nakano, T., Imafuku, T., Kato, H., Tokumaru, K., et al. (2020). Indoxyl sulfate contributes to adipose tissue inflammation through the activation of NADPH oxidase. Toxins (Basel) 12 (8), 502. doi:10.3390/toxins12080502
Tanase, D. M., Gosav, E. M., Neculae, E., Costea, C. F., Ciocoiu, M., Hurjui, L. L., et al. (2020). Role of gut microbiota on onset and progression of microvascular complications of type 2 diabetes (T2DM). Nutrients 12 (12), 3719. doi:10.3390/nu12123719
Tao, S., Li, L., Li, L., Liu, Y., Ren, Q., Shi, M., et al. (2019). Understanding the gut-kidney axis among biopsy-proven diabetic nephropathy, type 2 diabetes mellitus and healthy controls: An analysis of the gut microbiota composition. Acta Diabetol. 56 (5), 581–592. doi:10.1007/s00592-019-01316-7
Tavafi, M. (2013). Diabetic nephropathy and antioxidants. J. Nephropathol. 2 (1), 20–27. doi:10.5812/nephropathol.9093
Turkmen, K. (2017). Inflammation, oxidative stress, apoptosis, and autophagy in diabetes mellitus and diabetic kidney disease: The four horsemen of the apocalypse. Int. Urol. Nephrol. 49 (5), 837–844. doi:10.1007/s11255-016-1488-4
van der Kloet, F. M., Tempels, F. W., Ismail, N., van der Heijden, R., Kasper, P. T., Rojas-Cherto, M., et al. (2012). Discovery of early-stage biomarkers for diabetic kidney disease using ms-based metabolomics (FinnDiane study). Metabolomics 8 (1), 109–119. doi:10.1007/s11306-011-0291-6
Vaziri, N. D., Wong, J., Pahl, M., Piceno, Y. M., Yuan, J., DeSantis, T. Z., et al. (2013). Chronic kidney disease alters intestinal microbial flora. Kidney Int. 83 (2), 308–315. doi:10.1038/ki.2012.345
Vr, A. L. B. V. R., Tan, S. H., Candasamy, M., and Bhattamisra, S. K. (2019). Diabetic nephropathy: An update on pathogenesis and drug development. Diabetes Metab. Syndr. 13 (1), 754–762. doi:10.1016/j.dsx.2018.11.054
Wang, J. W., Kuo, C. H., Kuo, F. C., Wang, Y. K., Hsu, W. H., Yu, F. J., et al. (2019). Fecal microbiota transplantation: Review and update. J. Formos. Med. Assoc. 118 (Suppl. 1), S23–s31. doi:10.1016/j.jfma.2018.08.011
Wang, P., Guo, R., Bai, X., Cui, W., Zhang, Y., Li, H., et al. (2022). Sacubitril/Valsartan contributes to improving the diabetic kidney disease and regulating the gut microbiota in mice. Front. Endocrinol. (Lausanne) 13, 1034818. doi:10.3389/fendo.2022.1034818
Wang, X., Yang, S., Li, S., Zhao, L., Hao, Y., Qin, J., et al. (2020). Aberrant gut microbiota alters host metabolome and impacts renal failure in humans and rodents. Gut 69 (12), 2131–2142. doi:10.1136/gutjnl-2019-319766
Watanabe, H., Miyamoto, Y., Honda, D., Tanaka, H., Wu, Q., Endo, M., et al. (2013). p-Cresyl sulfate causes renal tubular cell damage by inducing oxidative stress by activation of NADPH oxidase. Kidney Int. 83 (4), 582–592. doi:10.1038/ki.2012.448
Wu, H., Esteve, E., Tremaroli, V., Khan, M. T., Caesar, R., Mannerås-Holm, L., et al. (2017). Metformin alters the gut microbiome of individuals with treatment-naive type 2 diabetes, contributing to the therapeutic effects of the drug. Nat. Med. 23 (7), 850–858. doi:10.1038/nm.4345
Wu, I. W., Gao, S. S., Chou, H. C., Yang, H. Y., Chang, L. C., Kuo, Y. L., et al. (2020a). Integrative metagenomic and metabolomic analyses reveal severity-specific signatures of gut microbiota in chronic kidney disease. Theranostics 10 (12), 5398–5411. doi:10.7150/thno.41725
Wu, I. W., Lin, C. Y., Chang, L. C., Lee, C. C., Chiu, C. Y., Hsu, H. J., et al. (2020b). Gut microbiota as diagnostic tools for mirroring disease progression and circulating nephrotoxin levels in chronic kidney disease: Discovery and validation study. Int. J. Biol. Sci. 16 (3), 420–434. doi:10.7150/ijbs.37421
Xourgia, E., Papazafiropoulou, A., Papanas, N., and Melidonis, A. (2019). Anti-diabetic treatment leads to changes in gut microbiome. Front. Biosci. (Landmark Ed. 24 (4), 688–699. doi:10.2741/4743
Xu, J., Liang, R., Zhang, W., Tian, K., Li, J., Chen, X., et al. (2020). Faecalibacterium prausnitzii-derived microbial anti-inflammatory molecule regulates intestinal integrity in diabetes mellitus mice via modulating tight junction protein expression. J. Diabetes 12 (3), 224–236. doi:10.1111/1753-0407.12986
Xu, Y. H., Gao, C. L., Guo, H. L., Zhang, W. Q., Huang, W., Tang, S. S., et al. (2018). Sodium butyrate supplementation ameliorates diabetic inflammation in db/db mice. J. Endocrinol. 238 (3), 231–244. doi:10.1530/joe-18-0137
Xu, Z., and Knight, R. (2015). Dietary effects on human gut microbiome diversity. Br. J. Nutr. 113 (0), S1–S5. doi:10.1017/s0007114514004127
Yadav, M. K., Kumari, I., Singh, B., Sharma, K. K., and Tiwari, S. K. (2022). Probiotics, prebiotics and synbiotics: Safe options for next-generation therapeutics. Appl. Microbiol. Biotechnol. 106 (2), 505–521. doi:10.1007/s00253-021-11646-8
Yan, X., Feng, B., Li, P., Tang, Z., and Wang, L. (2016). Microflora disturbance during progression of glucose intolerance and effect of sitagliptin: An animal study. J. Diabetes Res. 2016, 2093171. doi:10.1155/2016/2093171
Yang, M., Zhang, R., Zhuang, C., Wu, Y., Yang, Q., Yu, Z., et al. (2022). Serum trimethylamine N-oxide and the diversity of the intestinal microbial flora in type 2 diabetes complicated by diabetic kidney disease. Clin. Lab. 68 (5). doi:10.7754/Clin.Lab.2021.210836
Yao, Z., Yang, S., He, W., Li, L., Xu, R., Zhang, X., et al. (2015). P311 promotes renal fibrosis via TGFβ1/Smad signaling. Sci. Rep. 5, 17032. doi:10.1038/srep17032
Yoshida, H., Ishii, M., and Akagawa, M. (2019). Propionate suppresses hepatic gluconeogenesis via GPR43/AMPK signaling pathway. Arch. Biochem. Biophys. 672, 108057. doi:10.1016/j.abb.2019.07.022
Zhang, M., Feng, R., Yang, M., Qian, C., Wang, Z., Liu, W., et al. (2019). Effects of metformin, acarbose, and sitagliptin monotherapy on gut microbiota in Zucker diabetic fatty rats. BMJ Open Diabetes Res. Care 7 (1), e000717. doi:10.1136/bmjdrc-2019-000717
Zhang, Q., Xiao, X., Li, M., Yu, M., Ping, F., Zheng, J., et al. (2017). Vildagliptin increases butyrate-producing bacteria in the gut of diabetic rats. PLoS One 12 (10), e0184735. doi:10.1371/journal.pone.0184735
Zhang, W., Zou, G., Li, B., Du, X., Sun, Z., Sun, Y., et al. (2020). Fecal microbiota transplantation (FMT) alleviates experimental colitis in mice by gut microbiota regulation. J. Microbiol. Biotechnol. 30 (8), 1132–1141. doi:10.4014/jmb.2002.02044
Zhang, X., Shen, D., Fang, Z., Jie, Z., Qiu, X., Zhang, C., et al. (2013). Human gut microbiota changes reveal the progression of glucose intolerance. PLoS One 8 (8), e71108. doi:10.1371/journal.pone.0071108
Zheng, L., Ji, Y. Y., Wen, X. L., and Duan, S. L. (2022). Fecal microbiota transplantation in the metabolic diseases: Current status and perspectives. World J. Gastroenterol. 28 (23), 2546–2560. doi:10.3748/wjg.v28.i23.2546
Zhou, T., Xu, H., Cheng, X., He, Y., Ren, Q., Li, D., et al. (2022). Sodium butyrate attenuates diabetic kidney disease partially via histone butyrylation modification. Mediat. Inflamm. 2022, 7643322. doi:10.1155/2022/7643322
Keywords: diabetic nephropathy, gut microbes, metabolites, novel therapeutics, therapeutic targets
Citation: Tian E, Wang F, Zhao L, Sun Y and Yang J (2023) The pathogenic role of intestinal flora metabolites in diabetic nephropathy. Front. Physiol. 14:1231621. doi: 10.3389/fphys.2023.1231621
Received: 30 May 2023; Accepted: 26 June 2023;
Published: 04 July 2023.
Edited by:
Zhanzheng Zhao, Zhengzhou University, ChinaReviewed by:
Yiding Zhang, First Affiliated Hospital of Zhengzhou University, ChinaCopyright © 2023 Tian, Wang, Zhao, Sun and Yang. This is an open-access article distributed under the terms of the Creative Commons Attribution License (CC BY). The use, distribution or reproduction in other forums is permitted, provided the original author(s) and the copyright owner(s) are credited and that the original publication in this journal is cited, in accordance with accepted academic practice. No use, distribution or reproduction is permitted which does not comply with these terms.
*Correspondence: Jurong Yang, eWpyOTIzQDE2My5jb20=
Disclaimer: All claims expressed in this article are solely those of the authors and do not necessarily represent those of their affiliated organizations, or those of the publisher, the editors and the reviewers. Any product that may be evaluated in this article or claim that may be made by its manufacturer is not guaranteed or endorsed by the publisher.
Research integrity at Frontiers
Learn more about the work of our research integrity team to safeguard the quality of each article we publish.