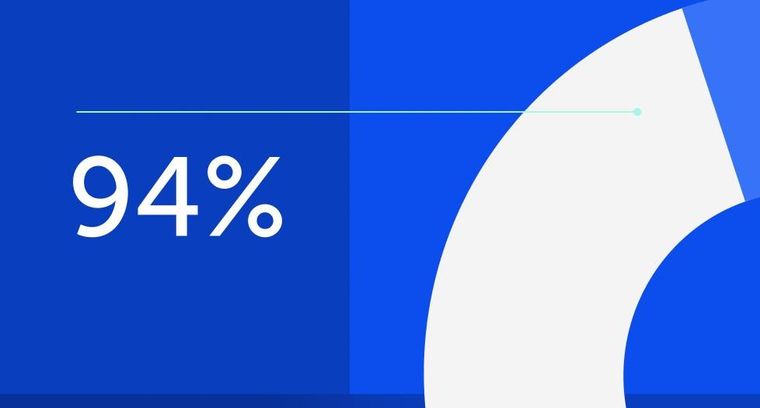
94% of researchers rate our articles as excellent or good
Learn more about the work of our research integrity team to safeguard the quality of each article we publish.
Find out more
REVIEW article
Front. Physiol., 29 June 2023
Sec. Aquatic Physiology
Volume 14 - 2023 | https://doi.org/10.3389/fphys.2023.1226068
Maintaining normal pH levels in the body fluids is essential for homeostasis and represents one of the most tightly regulated physiological processes among vertebrates. Fish are generally ammoniotelic and inhabit diverse aquatic environments that present many respiratory, acidifying, alkalinizing, ionic and osmotic stressors to which they are able to adapt. They have evolved flexible strategies for the regulation of acid-base equivalents (H+, NH4+, OH− and HCO3−), ammonia and phosphate to cope with these stressors. The gills are the main regulatory organ, while the kidneys play an important, often overlooked accessory role in acid-base regulation. Here we outline the kidneys role in regulation of acid-base equivalents and two of the key ‘urinary buffers’, ammonia and phosphate, by integrating known aspects of renal physiology with recent advances in the molecular and cellular physiology of membrane transport systems in the teleost kidneys. The renal transporters (NHE3, NBC1, AE1, SLC26A6) and enzymes (V-type H+ATPase, CAc, CA IV, ammoniagenic enzymes) involved in H+ secretion, bicarbonate reabsorption, and the net excretion of acidic and basic equivalents, ammonia, and inorganic phosphate are addressed. The role of sodium-phosphate cotransporter (Slc34a2b) and rhesus (Rh) glycoproteins (ammonia channels) in conjunction with apical V-type H+ ATPase and NHE3 exchangers in these processes are also explored. Nephrocalcinosis is an inflammation-like disorder due to the precipitation of calcareous material in the kidneys, and is listed as one of the most prevalent pathologies in land-based production of salmonids in recirculating aquaculture systems. The causative links underlying the pathogenesis and etiology of nephrocalcinosis in teleosts is speculative at best, but acid-base perturbation is probably a central pathophysiological cause. Relevant risk factors associated with nephrocalcinosis are hypercapnia and hyperoxia in the culture water. These raise internal CO2 levels in the fish, triggering complex branchial and renal acid-base compensations which may promote formation of kidney stones. However, increased salt loads through the rearing water and the feed may increase the prevalence of nephrocalcinosis. An increased understanding of the kidneys role in acid-base and ion regulation and how this relates to renal diseases such as nephrocalcinosis will have applied relevance for the biologist and aquaculturist alike.
Acid-base regulation is vital for all vertebrates to maintain homeostasis of intra- and extracellular pH. Maintenance of normal pH levels is essential for homeostasis in many physiological systems and one of the most tightly regulated physiological processes among vertebrates. In contrast to terrestrial animals, fish live in aquatic environments, and must thus utilize different strategies to regulate acid-base equivalents (H+, NH4+, OH− and HCO3−), ammonia and phosphate. Recent reviews (Tresguerres et al., 2020; Zimmer and Perry, 2022) provide a useful overview of acid-base regulation and ammonia excretion in fish, and since the two processes are intimately related, they will be considered together. For both processes, there is general accord that the gills constitute the major regulatory site, while the kidneys play an important accessory role. In terms of acid-base balance, excretion of H+ or NH4+ both represent acidic equivalent excretion, whereas excretion of OH− or HCO3− both represent basic equivalent excretion (Hills, 1973; Davenport, 1974). Disturbances of acidic or basic equivalents are traditionally termed “metabolic disturbances”. The respiratory gases CO2 and NH3 certainly affect the pH of the body fluids (CO2 is acidifying, whereas NH3 is alkalinizing), but in classic acid-base terms, these are not considered to be acidic or basic equivalents, but rather “respiratory factors” that affect acid-base homeostasis. As the typical pH of fish body fluids (7.2—8.2) is far above the pK of the CO2 - HCO3− interconversion, and far below the pK of the NH4+ - NH3 interconversion, HCO3− and NH4+ are the species that greatly predominate - generally >95% in terms of concentration in the body fluids (Randall and Wright, 1989). However, it is the uncharged gases (CO2 and NH3) that move easily across cell membranes, whereas the movements of the charged ions HCO3− and NH4+ (basic and acidic equivalents respectively) must be facilitated by transporters or channels.
Nephrocalcinosis is an inflammation-like disorder due to precipitation of calcareous material in the kidneys. In salmonid aquaculture, the prevalence of nephrocalcinosis appears to increase, and is primarily considered a problem in land-based systems (i.e., ‘freshwater and brackish water’) but is also observed in the seawater phase (Klykken et al., 2022a). The causative links underlying the pathogenesis and etiology of nephrocalcinosis in teleosts are speculative at best, but acid-base perturbations is assumed to be a central pathophysiological cause (Minarova et al., 2023). In order to introduce preventive measures and possible treatments, a better understanding of the exact pathophysiology and mechanisms that cause nephrocalcinosis is required. Previously, we have described the function and role of the kidneys in ion and water regulation in response to salinity (Takvam et al., 2021). Thus, this review aims to I) describe the kidneys role in regulation of acid-base equivalents and the two primary ‘urinary buffers’, ammonia and phosphate used by freshwater and marine teleosts, and II) integrate known aspects of renal physiology with recent advances in the molecular understanding of membrane transport systems involved in acid-base regulation, ammonia and phosphate excretion via the renal tubules. Where knowledge on these membrane transporters in the teleost kidneys is limited, we will take advantage of mechanisms described for gills and/or the mammalian literature. Our main aim is to integrate recent molecular findings on transport pathways, with classical information on the function of the teleost kidneys in acid-base regulation, ammonia, and phosphate excretion. Finally, how this synthesis applies to pathophysiology and increased prevalence of nephrocalcinosis in modern land-based aquaculture is outlined.
Common factors that challenge acid-base balance in fish include: i) exhaustive exercise (Mommsen and Hochachka, 1988; Wood, 1988; Wood, 1991a) and environmental hypoxia (Kobayashi and Wood, 1980), both of which generate acidic equivalents by adenylate breakdown and lactate production, resulting in metabolic acidosis, sometimes exacerbated by CO2 buildup (respiratory acidosis) or ameliorated by CO2 washout (respiratory alkalosis) due to hyperventilation; ii) high environmental PCO2, often associated with algal respiration, which causes respiratory acidosis, and is a particular issue in closed system aquaculture (Ellis et al., 2017); iii) environmental hyperoxia, often associated with algal photosynthesis, as well as oxygen injection in aquaculture (McArley et al., 2021), which causes hypoventilation and associated CO2 retention resulting in respiratory acidosis (Wood and Jackson, 1980; Wood, 1991b); iv) the post-feeding “alkaline tide” (metabolic alkalosis) caused by HCl secretion in the stomach and matching NaHCO3 addition to the bloodstream during digestion (Bucking and Wood, 2008; Cooper and Wilson, 2008); v) The post-feeding “acidic tide” in agastric fish caused by NaHCO3 secretion in the intestine and matching HCl addition to the bloodstream (Wood et al., 2010); vi) low environmental pH associated with acidic precipitation which induces metabolic acidosis (Wood, 1989); and vii) the decrease in the “strong ion difference” that results in a decrease of plasma HCO3− concentration (metabolic acidosis) when freshwater fish move into seawater (Maxime et al., 1991), and increase of plasma HCO3− (metabolic alkalosis) that occurs when the transfer is in the opposite direction (Maxime et al., 1990). Some of the factors that challenge acid-base regulation in fish also cause internal ammonia generation and increased ammonia excretion, including i) exhaustive exercise (Wood, 1988); ii) feeding (Karlsson et al., 2006; Bucking and Wood, 2008; Zimmer et al., 2010); iii) freshwater-to-seawater transfer (Wood and Nawata, 2011); iv) exposure to low environmental pH (McDonald and Wood, 1981), whereas v) exposure to high environmental pH inhibits ammonia excretion and causes internal ammonia accumulation (Wilkie and Wood, 1996), and vi) exposure to high environmental ammonia (HEA), another common complication in aquaculture (Beamish and Tandler, 1990; Handy et al., 1993), usually has a biphasic effect, with initial ammonia uptake and then later augmented ammonia excretion by the fish (Nawata et al., 2007; Nawata et al., 2010; Zimmer et al., 2010; Wood and Nawata, 2011).
Relative to the gills, the approximate contribution of the kidneys to the net excretion of acidic equivalents is generally small, based on the studies conducted in teleost fishes so far (Table 1). For example, in freshwater trout, renal excretion accounted for 7% of the compensation during hyperoxia (Wheatly et al., 1984), 16% during hypercapnia (Perry et al., 1987), 5% during the post-feeding alkaline tide (Bucking et al., 2010), 8% during recovery from exhaustive exercise (Wood, 1988), and 12% during recovery from hypoxia-induced lactacidosis (Kobayashi and Wood, 1980; Table 1). In the channel catfish, Ictalurus punctatus, the contribution of the kidneys to compensation of some of these same disturbances was greater, but still accounted for less than 33% overall (Cameron, 1980; Cameron and Kormanik, 1982). However, an important exception is the metabolic acidosis of low environmental pH (4.0–4.5) exposure in rainbow trout (Oncorhynchus mykiss), where renal excretion of acidic equivalents accounted for 100% of the compensatory response that occurred, as the gills continued to take up acidic equivalents throughout the exposure period (McDonald and Wood, 1981; Wood et al., 1999). Indeed, this treatment induced the highest urinary excretion rate of acidic equivalents ever recorded in a freshwater fish.
TABLE 1. Approximate percent (%) contribution to net excretion of acidic equivalents by the gills and kidneys in teleost fishes.
Under normal circumstances in fasting, resting fish, the net excretion of acid-base equivalents through both the renal and branchial routes is negligible, as the animals are in acid-base balance at this time. However, the kidneys make an incredibly important, often overlooked, contribution to this steady state, by actively reabsorbing the vast majority of the HCO3− (i.e., basic equivalents) that has been filtered from the blood plasma into the primary urine in the glomeruli. This is undoubtedly important in both freshwater and seawater teleosts, but quantitatively more so in the former where the glomerular filtration rates (GFRs) are higher. To illustrate this with one example, in the resting freshwater rainbow trout, the filtered load of HCO3− was about 48 μmol kg-1 h-1 (the product of a GFR of about 5.3 mL kg-1 h-1 and a plasma HCO3− concentration of 9 μmol mL-1) essentially all of which was reabsorbed in the tubules so that none was excreted (Curtis and Wood, 1992). This represented 13% of the entire plasma HCO3− pool of 360 μmol kg-1. Without this reabsorption, the entire pool of basic equivalents would have been lost in less than 8 h! Furthermore, when the trout were loaded with excess HCO3− by infusion with an isosmotic solution of NaHCO3, the HCO3− reabsorption rate increased 2.5-fold. A similar increase in the rate of active HCO3− reabsorption occurs during the compensation of respiratory acidosis caused be environmental hyperoxia (Wheatly et al., 1984) and environmental hypercapnia (Perry et al., 1987). This ensures that the basic equivalents accumulated via uptake at the gills are not lost through the kidneys. Indeed, in these circumstances, not only do the tubules reabsorb all the HCO3− filtered at the glomeruli, they also secrete additional acidic equivalents that are excreted on a net basis. When the respiratory acidosis stimulus is relieved, the HCO3− reabsorption and acid secretion mechanisms are rapidly suppressed, while urine flow rate (UFR) continues unchanged, so that large amounts of basic equivalents (HCO3−), now excess to requirements, can be rapidly excreted via the urine. Interestingly, in the plainfin midshipman (Porichthys notatus), an aglomerular marine fish, restoration of normal plasma HCO3− levels after suspension of environmental hypercapnia was very slow, presumably reflecting the lack of a direct glomerular filtration route for renal excretion of basic equivalents (Perry et al., 2010).
In freshwater fish, the urinary bladder plays an important role in reabsorbing major ions from the tubular urine, thereby contributing to the ion-poor nature of the finally excreted urine, but does not seem to be involved in the addition or removal of acidic or basic equivalents (Curtis and Wood, 1991; Curtis and Wood, 1992). In marine fish, the UFRs are much lower, and urine is held in the bladder for very long periods during which time additional water is reabsorbed and the salts become even more concentrated (Hickman and Trump, 1969; Beyenbach and Kirschner, 1975; Takvam et al., 2021a). Whereas freshwater trout urinate about twice per hour (Curtis and Wood, 1991), marine plaice (Pleuronectes platessa) urinate only once every 3–5 days (Fletcher, 1990)! Urine pH is generally very acidic in marine fish, 1.0–2.0 pH units below that of the blood plasma. This probably serves to minimize the precipitation of Ca2+ and Mg2+ salts in the tubules and bladder during this prolonged residence period, though it is not completely successful (Hickman and Trump, 1969; Miles, 1971). The urinary response to systemic acid-base disturbances appeared to be negligible in two marine teleosts, the shorthorn sculpin Myoxocephalus scorpius (Hodler et al., 1955) and the English sole Parophrys vetulus (McDonald et al., 1982). Maren et al. (1992) presented an opposing view, but the methods of their experiments on the long-horned sculpin Myocephalus octodemispinosus were less than ideal. The low UFRs and the mandatory production of an acidic urine to prevent precipitates are probably important factors limiting the kidneys performance in acid-base regulation in marine fish. Please see illustration on regulation of acid-base equivalents (Figure 1) for a general overview.
FIGURE 1. Schematic overview showing the main transport directions of acidic (H+, NH4+) and basic (HCO3-) equivalents and the uncharged respiratory gases CO2 (acidic) and NH3 (alkaline) involved in acid-base homeostasis in the teleost kidney. The primary urine filtrate from the glomerulus (and bowman`s capsule) contains bicarbonate (HCO3-), sodium (Na+) and dibasic phosphate (HPO42-) that enters the tubule for either excretion or reabsorption as they move along the proximal and distal tubule and collecting duct. Extracellular carbonic anhydrase (CA-IV) on the tubule apical membrane facing the lumen catalyzes the dehydration reaction of a filtered HCO3- (appearing in the primary urine) with a H+ secreted by the tubule cell. The H+ originates from the action of intracellular cytosolic carbonic anhydrase (CAc) that catalyzes the hydration of CO2, thereby permitting the HCO3- ion to be returned to the blood. The back diffusion of CO2 from the urine to the tubule refuels this reaction. The uncharged gases (CO2 and NH3) move easily across cell membranes, whereas the movements of the charged ions HCO3- and NH4+ must be facilitated by transporters or channels. The two major “urinary buffers” are ammonia and phosphate. Once most of the HCO3- has been reabsorbed, any additional H+ ions that are secreted stay in the urine, titrating NH3 to NH4+, and dibasic phosphate (HPO42-) to monobasic phosphate (H2PO4-), both of which are excreted. Another mechanism of NH4+ appearance in the urine is also shown and may be quantitatively more important, based on mammalian studies. This is the oxidative metabolism of glutamine (or other amino acids) which produces equimolar amounts of NH4+ which is secreted into the urine, and HCO3- which is returned to the blood plasma. Note that by both of these schemes, for every acidic equivalent excreted, a basic equivalent (HCO3-) is added to the blood plasma. The different transport mechanisms occur in several segments of the nephron and the model is not intended to illustrate any specific segment, only the mechanisms.
In mammals (Weiner and Verlander, 2017), the reabsorption of HCO3− depends on the tubular secretion of H+ (linked to Na+ reabsorption via a Na+/H+ exchanger and/or a Na+ channel coupled to a v-type H+ATPase) and is dependent on the enzyme carbonic anhydrase (CA). Intracellular CA catalyzes the hydration of CO2 to form the H+ ion that is secreted across the apical membrane and the HCO3− ion that is returned to the blood across the basolateral membrane, by a Na+-HCO3- co-transporter. Extracellular CA in the tubule lumen catalyzes the dehydration reaction of the secreted H+ with HCO3− appearing in the glomerular filtrate. The net effect is that for every one H+ ion secreted, one HCO3− ion and one Na+ ion are removed from the filtrate, while one HCO3− (albeit a different HCO3−) and one Na+ ion are returned to the blood. CO2 from the dehydration reaction in the urine diffuses back into the renal tubule cell to refuel the H+ secretion process. When secreted H+ ions exceed filtered HCO3− ions, they are buffered in the urine by inorganic phosphate or ammonia, and are excreted, contributing to net acidic equivalent excretion; as a result, a new basic equivalent (HCO3−) is synthesized on a net basis, and returned to the blood. The whole process is largely powered by Na+, K+ ATPase (NKA), though v-type H+ATPase may also contribute.
All available evidence indicates that the same model applies in fish (Gilmour and Perry, 2009). At the whole animal level, when freshwater trout increase their renal excretion of acidic equivalents, they utilize two major urinary “buffers”, ammonia and phosphate, as in mammals (Hills, 1973; Pitts, 1974). If these were not present, even small amounts of H+ secretion in the tubules would drive urine pH so low that the pumping processes would falter. The buffers modulate this; acidic equivalents appear in the urine by the titration of NH3 to NH4+, and the titration of dibasic phosphate (HPO42-) to monobasic phosphate (H2PO4−). At least in rainbow trout, NH4+ appears to be the major buffer mobilized during metabolic acidosis (McDonald and Wood, 1981; Wood et al., 1999) while phosphate predominates during respiratory acidosis (Wheatly et al., 1984; Perry et al., 1987; Wood et al., 1999). In practical terms, the acidic equivalents carried by phosphate can be measured as “titratable acidity (TA)” by titration of the urine back to the blood pH with strong base, while the NH4+ component, which escapes this titration, is measured directly, and any HCO3− in the urine is subtracted, so that net acidic excretion is equal to ([TA]—[HCO3-] + [NH4+]) x UFR (Hills, 1973).
At a molecular level, intracellular CA (cytosolic CAc) has been localized to NKA-rich cells in both proximal and distal tubule cells, whereas extracellular CA (membrane-bound CA IV) occurred in both the apical and basolateral membranes of NKA-rich cells, but only in the proximal tubules (Georgalis et al., 2006). Furthermore, respiratory acidosis induced by environmental hypercapnia increased mRNA of both isoforms with CAc rising quickly and CA IV rising slowly, whereas the protein expression of only CAc increased. Both general CA blockade (with acetazolamide) and selective CA IV blockade (with F3500) increased urinary Na+ and HCO3− excretion, especially during hypercapnia (Georgalis et al., 2006). Very recently, the salmonid specific CA IV paralogs have been described in the rainbow trout where CA IVb was located in the kidneys, but not the CA IVa (Nelson et al., 2023). The authors point out that several other tissue-specfic differences occur in rainbow trout and highlight the potential divergent roles of the CA IV paralogs in gas exchange and ion/acid-base balance, at least in salmonids. A Na+/H+ exchanger (NHE3, SLC9A3) and a v-type H+ATPase were co-localized to the apical membranes of NKA-rich cells in the proximal tubule of trout (Ivanis et al., 2008), and an anion exchanger (SLC26A1) was found in these same cells (Katoh et al., 2006). The mRNA and protein expression of the NHE3 increased during hypercapnia (Ivanis et al., 2008). Earlier studies had shown that mRNA and protein levels of v-type H+ATPase, and mRNA levels of Na+-HCO3- co-transporter (NBC1) also increased in the trout kidneys during hypercapnia (Perry and Fryer, 1997; Perry et al., 2003a; b).
There is only limited support for this “rainbow trout model” in other species. CAc has also been found in proximal tubule cells of marine winter flounder, Pleuronectes americanus (Pelis and Renfro, 2004). NHE3 has been found in the cells of the distal nephron of the euryhaline mangrove rivulus, Kryptolebias marmoratus (Cooper et al., 2013). However, NHE3 mRNA could not be detected in the kidneys of the European seabass, Dicentrarchus librax in either freshwater or seawater (L’Honoré et al., 2020). In the common carp, Cyprinus carpio, mRNA expression of v-type H+ATPase and NHE3 increased in the kidneys during prolonged metabolic acidosis caused by low environmental pH exposure, but protein expression did not change (Wright et al., 2014). However, these mRNA responses were not seen in goldfish, Carassius auratus subjected to similar low pH exposure (Lawrence et al., 2015). Recently profiling of transporters along the length of the nephron in goldfish appears to follow the model previously demonstrated (Fehsenfeld and Wood, 2018). Here the NHE3, v-type H+ATPase, CA IIa (cytosolic, similar to CAc of trout), anion exchanger AE1 (similar to SLC26A1 of trout) and NBC1 are all expressed in the proximal tubule and NHE3 is upregulated at both protein and mRNA levels with relocation to the apical membrane, by the metabolic acidosis following feeding in this agastric species. Basolaterally located AE1 is simultaneously downregulated at the protein level in all sections, in accord with less HCO3− secretion and greater urine acidification. However, the situation in the goldfish is complicated by findings that v-type H+-ATPase is exclusively basolateral in distribution, which fits with observations that goldfish urine is usually highly alkaline under control conditions (Lawrence et al., 2015). Furthermore, expression levels for some of these transporters are comparable or higher in other sections of the nephron (e.g., NHE3 and CAIIa in the distal tubule, connecting tubule, and collecting duct; v-type H+-ATPase, AE1, and NBC1 in all sections), with mixed responses to feeding (Fehsenfeld and Wood, 2018). These observations correlate with microelectrode measurements showing that net H+ secretion is not localized but occurs along the entire length of the isolated nephron in the goldfish, with elevated rates in all sections following feeding (Fehsenfeld et al., 2019). Interestingly, in this same investigation, blocker studies indicated that v-type H+-ATPase was important in the proximal and distal tubule, NHE3 was important in the proximal and connecting tubules, and CA IIa was important in H+ secretion in all sections. Studies on a wider range of species are clearly needed. Please see Figure 2 for a detailed overview of current ideas on renal transport of acid-base equivalents.
FIGURE 2. Basic transport mechanisms involved in reabsorption of bicarbonate (HCO3-) in the proximal tubule of teleost kidney. The basolateral Na+- K+ -ATPase (NKA) and apical V-type H+-ATPase generate favorable transepithelial membrane potentials used by some of the other ion transport pathways. Extracellular carbonic anhydrase (CA-IV) on the tubule membrane dehydrates HCO3- in the glomerular filtrate (primary urine) utilizing the H+ secreted by an apical Na+/H+ exchanger (NHE3) and/or by a V-type H+-ATPase coupled to a Na+ channel. The NHE3 is an abundant but less powerful secondary transport mechanism that cannot secrete H+ against low urine pH. The H+-ATPase is a less abundant but powerful enzymatic transport mechanism that can secrete against very low urine pH. Inside tubule cells cytosolic (CAc) catalyzes the hydration of CO2 forming HCO3- to be returned to the blood via a basolateral Na+-HCO3- co-transporter (NBC1) and/or anion exchanger AE1. If the Na+-coupled secretion mechanisms (NHE3, H+-ATPase) for H+ secretion cannot match the rate of HCO3- filtration, then net HCO3- excretion occurs, while if the H+ secretion rate exceeds the rate of HCO3- filtration, then net H+ excretion occurs. Note that after all HCO3- is reabsorbed continued net H+ excretion is dependent on the presence of urinary buffers (see figure 1).
Given the reduced role of the kidneys acid-base regulation in marine fish discussed earlier, and the general alkalizing effect of transfer to more dilute salinity (Maxime et al., 1990), we might expect that euryhaline fish would increase expression of the renal H+ secretion/HCO3− reabsorption system when transferred from seawater to more dilute salinity. However, exactly the opposite happened (downregulation of mRNA for v-type H+ATPase, CAc, CA IV, NHE3, and NBC1) when 100% seawater-acclimated trout were transferred to 25% seawater (Gilmour et al., 2012). The authors suggested that since intestinal HCO3− secretion was reduced, there would be less need for HCO3− conservation by the kidneys. Similarly, in European sea bass, mRNA expressions of anion exchangers AE1 and SLC26A6 were downregulated after transfer of seawater-acclimated European sea bass to freshwater (L’Honoré et al., 2020). The opposite response was observed during smoltification and after SW transfer in Atlantic salmon, Salmo salar where the salmonid-specific slc26a6a1 was upregulated in the kidneys (Takvam et al., 2021b). This transporter appears to play an important role in SO42- secretion in the kidneys of euryhaline teleosts (Watanabe and Takei, 2012; Kato and Watanabe, 2016; Takvam et al., 2021a). On the contrary the salmonid-specific slc26a6a2 was only expressed in FW gills while the slc26a6a1 was expressed equally in FW and SW intestine (Takvam et al., 2021b). Interestingly, the SLC26A6A has been linked to HCO3− transport in the intestine of mefugu, Takifugu obscurus (Kurita et al., 2008) and toadfish, Opsanus beta (Grosell et al., 2009), while in the gills the same transporter has been linked to both Cl− and HCO3− transport in FW acclimated teleosts (Perry and Gilmour, 2006; Deighweiher et al., 2008; Leguen et al., 2015). This emphasize that the SLC26A6 transporter likely performs different tasks and exhibits a broad ion specificity depending on the tissue in question.
In mammals (Weiner and Verlander, 2017), most of the ammonia entering the urine is produced by the metabolism of amino acids, principally glutamine, in the renal tubule cells; very little enters via glomerular filtration. While all sections of the nephron can produce ammonia, the proximal tubule is the principal site of ammoniagenesis. The substrate glutamine is taken up by specific transporters, both from the urine and from the venous blood. Each NH3 diffusing into the urine traps a H+ and thereby becomes trapped itself in the lumen (“diffusion trapping”). While this was the original, classical interpretation of the mechanism of urinary buffering by ammonia (Pitts, 1974), modern evidence suggests that it is a minor component in the proximal tubule, and that most ammonia enters as NH4+ via transporters or channels (Weiner and Verlander, 2017). Nevertheless, the metabolic breakdown of amino acids, most importantly glutamine, produces equimolar amounts of HCO3− and NH4+ on a net basis. For every NH4+ ion secreted into the urine across the apical membrane, a HCO3− ion is added to the blood across the basolateral membrane, and therefore, NH4+ excreted in the urine represents acidic equivalent excretion, in accord with classical theory (Hills, 1973; Pitts, 1974), regardless of the mechanism by which it entered. Recent research has revealed that further down the tubule, complex recycling and reabsorption of both NH3 and NH4+ occurs in accord with the countercurrent arrangement of mammalian nephrons, resulting in elevated ammonia concentrations in the interstitial fluid. The final net addition of ammonia to the urine (as much as 60%–80% of the total) returns ammonia to the urine from the interstitium, and occurs mainly in the collecting duct, with some contributions by the distal tubule and connecting tubule.
Under normal circumstances in fasting, resting fish, the excretion of ammonia in the urine generally accounts for only a few percent of whole body ammonia excretion, but often a larger fraction of less abundant N-wastes such as urea, creatine, creatinine, uric acid and trimethylamine oxide (Wood, 1993; Wood, 1995; Wood, 2001). Some exceptions exist. In cutthroat (Oncorhynchus clarki henshawi) adapted to the high pH (9.4) of Lake Lahontan, the renal contribution to whole body ammonia excretion (10%) was high relative to many other studies on salmonids in freshwater (Wright et al., 2014). In the obligate air-breathing fish, the Amazonian pirarucu, Arapaima gigas, which has a relatively low gill area, the kidneys accounted for about 20% of total ammonia excretion (Wood et al., 2020). The few urinary ammonia excretion measurements available for fish in seawater indicate a very low % contribution to whole body efflux (McDonald et al., 1982; Wood et al., 2005). The very low UFRs of marine fish undoubtedly constrain the capacity of the kidneys for ammonia excretion. We are aware of no comparable information on the percentage of phosphate excretion by the kidneys in either freshwater or seawater fish. However, under normal circumstances, the absolute concentration of phosphate in the urine is generally comparable that of ammonia on a molar basis (Curtis and Wood, 1991; Curtis and Wood, 1992; Lawrence et al., 2015). In goldfish injected with exogenous phosphate, Kaune and Hentschel (1987) reported that 65% of the phosphate load was excreted by the kidneys, largely by secretion into the urine. As noted earlier, the urinary excretion rates of both phosphate and ammonia increase markedly during the compensation of respiratory and metabolic acidosis, in accord with the roles of these molecules as key urinary buffers in fish (McDonald and Wood, 1981; Wheatly et al., 1984; Perry et al., 1987; Wood et al., 1999; Lawrence et al., 2015), just as in mammals (Hills, 1973; Pitts, 1974). The phosphate is likely mobilized from bone and scales as deficiencies appear to give bone deformaties (Lall and Kaushik, 2021), whereas ammonia originates from amino acid metabolism (Ip and Chew, 2010). Please see illustration of ammonia and phosphate excretion (Figure 1) for a general overview.
In ureotelic mammals, plasma levels of ammonia are extremely low, so ammonia enters the urine almost entirely by secretion. However, the molecular mechanisms of ammonia transport remain controversial and incompletely understood (Weiner and Verlander, 2017). In the proximal tubule, apical NHE3 seems to play a predominant role, with NH4+ substituting on the H+ site, such that NH4+ secretion is coupled to Na+ reabsorption. NH4+ movement through apical K+ channels, as well as the classic “diffusion trapping” mechanism, with NH3 diffusion coupled to apical H+ secretion by NHE3 and/or v-type H+ATPase, may also contribute, again facilitating coupling to Na+ reabsorption. The sodium, potassium, two chloride cotransporter type 2 (NKCC2) seems to be the principal reabsorptive transporter contributing to ammonia recycling in the loop of Henle, with NH4+ substituting on the K+ site. The final net addition of ammonia in the collecting duct seems to be largely mediated by two Rhesus (Rh) glycoproteins, which are channels that facilitate the diffusion of ammonia. Rhbg, which is exclusively basolateral, serves for basolateral uptake from the interstitium into the tubular cells, while Rhcg, which is both apical and basolateral, serves for apical secretion while supplementing basolateral uptake. Controversy continues as to whether the Rh proteins facilitate NH3 movement (perhaps true for Rhcg), NH4+ movement, or both (perhaps true for Rhbg). NH4+ substitution on the K+ site of basolateral NKA also contributes to basolateral uptake in the collecting duct. HCN2, a hyperpolarization-activated cyclic nucleotide gated channel (subtype 2) has also been implicated in basolateral NH4+ uptake. On the apical membrane, the “diffusion trapping mechanism” applies, with v-type H+ATPase as well as H+, K+ ATPase providing the apical H+ secretion (and perhaps also direct NH4+ transport by the H+ site), while Rhcg facilitates the apical diffusion of NH3.
In ammoniotelic fish, plasma ammonia concentrations are higher than those in ureotelic mammals, but still very low (micromolar) relative to urinary levels (millimolar). Therefore, as in mammals the great majority of ammonia enters the urine by tubular secretion rather than glomerular filtration, probably produced in the tubule cells by deamination and deamidation of glutamine and other amino acids. In a range of freshwater species, urine/plasma ratios of ammonia are high, and increase further during compensation of acidosis, indicating increased secretion (Cameron and Wood, 1978; McDonald and Wood, 1981; Wood et al., 1999; Wright et al., 2014; Lawrence et al., 2015; Thin et al., 2019). Renal activities of potential ammoniagenic enzymes (phosphate-dependent glutaminase, glutamate dehydrogenase, ɑ-ketoglutarate dehydrogenase, alanine aminotransferase, aspartase aminotransferase, phosphoenolpyruvate carboxykinase) are substantial, and some increase during experimental acidoses (Walton and Cowey, 1977; 1982; King and Goldstein, 1983; Wood et al., 1999; Lawrence et al., 2015), as well as mRNA for glutamate dehydrogenase (Hirata et al., 2003; Fehsenfeld and Wood, 2018). At least in the goldfish, glutamate dehydrogenase mRNA is expressed in all sections of the nephron, increasing during acidosis, and with highest levels in the proximal tubule, again as in mammals (Fehsenfeld and Wood, 2018). Given the lack of a countercurrent morphology, it seems likely that the ammonia transport mechanisms in fish will not necessarily parallel those in mammals. Nevertheless, the same basic elements appear to be present, with many similarities.
As noted earlier, one parallel is the presence of NHE3 and v-type H+ATPase in the proximal tubule of trout (Ivanis et al., 2008) and goldfish (Fehsenfeld and Wood., 2018). Furthermore, in the kidneys of the freshwater Nile tilapia Oreochromis niloticus, H+, K+ ATPase mRNA is expressed, with apical protein localization only in NKA-rich cells of the collecting tubule, another clear parallel to the mammalian model (Barnawi et al., 2020). Rhbg is expressed at the mRNA level in the kidneys of freshwater rainbow trout (Nawata et al., 2007) and the mangrove rivulus, K. marmoratus (Cooper et al., 2013); in the latter, there was apical co-localization of the protein with NHE3 in distal tubule cells. However, neither Rhbg nor Rhcg transcripts were detected in the kidneys of the pufferfish (Nakada et al., 2007a). Conversely, in both goldfish (Lawrence et al., 2015) and common carp (Wright et al., 2014), Rhcg (two isoforms) and Rhbg are present. Rhcg1a mRNA and protein were upregulated in response to the metabolic acidosis of low environmental pH exposure in the carp, whereas Rhcg1b mRNA responded in the same way in the goldfish. In the carp, Rhbg mRNA decreased during acidosis, though protein expression did not change (Wright et al., 2014). More detailed profiling in the goldfish revealed that Rhbg protein was basolaterally located, Rhcg1b was apical, whereas Rhcg1a was expressed in both membranes, in both proximal and distal tubules (Fehsenfeld and Wood, 2018). Rhcg1b mRNA and protein were both upregulated in the distal tubule, connecting tubule and collecting duct in response to the metabolic acidosis associated with feeding, whereas Rhcg1a mRNA and protein showed a more modest response in the distal tubule only. Rhbg mRNA was upregulated in the connecting tubule and collecting duct, but downregulated in the distal tubule, accompanied by decreased expression of the protein (Fehsenfeld and Wood, 2018). There was also strong expression of Rhcg1 in the kidneys of the zebrafish at both mRNA and protein levels, with localization to the apical membrane in the collecting duct and distal tubule (Nakada et al., 2007a). Please see Figure 3 for a detailed overview of current ideas on renal transport mechanisms in ammonia regulation.
FIGURE 3. Basic transport mechanisms involved in secretion of ammonia in the proximal and distal/collecting tubule of teleost kidney. The basolateral Na+- K+ -ATPase (NKA) and apical V-type H+-ATPase generate favorable transepithelial membrane potentials used by some of the other ion transport pathways in the proximal and distal/collecting tubule (left side and right side; 1 and 2). The rhesus (Rh) glycoproteins are channels that facilitate the diffusion of ammonia; although NH3 is shown as the form moving through them, there is considerable uncertainty as to whether they facilitate the movement of NH3, NH4+, or both. Rhbg and Rhcg1a enable the basolateral uptake of ammonia. Secretion of NH3 into the proximal tubule lumen is facilitated by apically located Rhcg1b and/or Rhcg1a channels (left side). Apical V-type H+ ATPase (proton pump) and NHE3 (Na+/H+ exchanger) both may transport H+ to the lumen, enabling the “diffusion trapping” mechanism (NH3 + H+) to form NH4+. The diffusion trapping mechanism is important to avoid NH3 reabsorption back to the tubule cells and maintains the gradient for NH3 secretion, ensuring that NH4+ can ultimately be excreted through the urine. In the proximal tubule, NHE3 may play a dominant role, not only exchanging H+ against Na+ for diffusion trapping but also directly exchanging NH4+ (from the oxidation of glutamine and other amino acids) for Na+ (left side). The NHE3-mediated diffusion trapping mechanism is also found in the distal/collecting tubule; there, the Rhcg1a channels perform the basolateral uptake of ammonia (right side; 1). However, in the distal/collecting tubule a second mechanism is present (right side; 2). Here the basolateral uptake of ammonia is possible through the Rhbg channel and apical secretion via the apical Rhcg1b. The diffusion trapping mechanism is possible via the H+, K+ ATPase, providing the apical H+ secretion needed to form NH4+ for later excretion in the urine. Both NH4+ substitutions on the K+ site of the basolateral Na+, K+ ATPase and a HCN2 (a hyperpolarization-activated cyclic nucleotide gated channel (subtype 2)) have also been hypothesized as a possible NH4+ basolateral uptake mechanisms in the collecting duct (not shown).
With respect to other putative ammonia transport mechanisms seen in mammals, various HCN genes, including HCN2b are expressed at the mRNA level in the goldfish kidneys, and several (though not HCN2b) respond to feeding and/or HEA exposure (Fehsenfeld and Wood, 2020). NKCC2 (the principal NH4+ transporter of the loop of Henle in mammals) is detectable at the mRNA level in the kidneys of seawater acclimated European sea bass, but is downregulated after transfer to freshwater (L'Honoré et al., 2020), so it is unclear whether it is involved in NH4+ transport. NH4+ substitution on the K+ site of renal NKA probably occurs, as in kidneys of the Amazonia pirarucu, NH4+ was more effective than K+ in activating the activity of this enzyme (Hochachka et al., 1978). Interestingly, in this obligate air-breather, unusually high urinary ammonia excretion was apparently coupled to high urinary HCO3− excretion (Wood et al., 2020). Studies of the specific mechanisms of ammonia regulation in the teleost kidneys are limited to a few number of species to date. Hence, both the localization and specific role of Rh proteins are still under debate and more research is required.
A comprehensive review of phosphate handing by teleosts, with a particular focus on the kidneys, has been presented by Verri and Werner (2019). Unlike ammonia, phosphate is originally obtained mainly from the diet and stored in the bones (Vielma and Lall, 1998). Plasma phosphate levels (millimolar) are much higher than ammonia levels (micromolar). Under control conditions, phosphate filtered at the glomeruli is normally strongly reabsorbed by the tubules in freshwater fish (Vielma and Lall, 1998), but during experimental acidosis, this reabsorption slows and may change over to tubular secretion (Wheatly et al., 1984; Perry et al., 1987; Wood et al., 1999; Lawrence et al., 2015). Tubular secretion may normally dominate in marine teleosts. In the marine winter flounder, Pseudopleuronectes americanus, secretion is driven by a type-II sodium-phosphate cotransporter (NaPi-II), now termed Slc34a2b, that is sorted to the basolateral membrane of the second part of the proximal tubule, whereas in the collecting tubule and collecting duct, the same transporter is located in the apical membranes and appears to drive reabsorption (Gupta and Renfro, 1989; Elger et al., 1998; Verri and Werner, 2019). Low peritubular pH strongly stimulated tubular phosphate secretion in an in vitro culture system (Gupta and Renfro, 1991). Both mRNA and protein for a reabsorptive NaPi-II cotransporter (now slc34a1) were identified in the kidneys of the freshwater rainbow trout, only in the first part of the proximal tubule, with localization to the apical membrane (Sugiura et al., 2003). This transporter is upregulated in response to low dietary phosphate in trout (Coloso et al., 2003; Sugiura and Ferraris, 2004), but we are aware of no information on its response to acid-base disturbance.
Based on the preceding material, we can conclude that the kidneys plays an important (and often overlooked) role in the regulation and removal of acid-base equivalents and ammonia in fish, especially in freshwater. Overall, the mechanistic details of acid-base regulation and ammonia excretion by the renal tubules of teleost fish are clearly complex and remain elusive, especially at the gene and molecular level (see Figure 4; locations of known transporters in the nephron). However, there are many aspects of the regulation that are understood at the macro level, where the integration of emerging genetic and molecular methods could further illuminate the mechanisms involved (see Future Research Direction). Traditionally, some of the most devastating diseases attacking fish in aquaculture have been kidney diseases—for example, proliferative kidney disease (PKD; Hedrick et al., 1993), bacterial kidney disease (BKD; Fryer and Sanders, 1981), nephrocalcinosis (Fivelstad et al., 2018), and hemorrhagic smolt syndrome (HSS; Byrne et al., 1998; Krasnov et al., 2019), all of which have severe fish health and welfare consequences. Knowledge of the kidney’s roles in acid-base, ammonia and phosphate regulation will be vital to better understand how the kidneys respond to changes in water quality and feed composition but also in kidney disease. This will be important especially in land-based intensive farming. Although these aspects definitely applies to other cultivated species in land-based aquaculture the main focus will be on Atlantic salmon and Rainbow trout as perturbations in the kidney is one of the leading welfare issues during land-based rearing of these species.
FIGURE 4. Overview of the localization of the transporters involved in acid-base, ammonia and phosphate regulation in teleost fish. Based on the current understanding and molecular analyses in teleosts, putative locations of various transporters in the nephron are presented for fish (G; Glomerulus, PT; Proximal tubule, DT; Distal tubule, CT; Collecting tubule and CD; Collecting duct). For the regulation pattern in different situations (e.g. changes in acid-base status, water chemistry, fasted versus non-fasted states) the reader is referred to the text. The CAc are found in all sections of the nephron PT, DT and CT. While similar location has been found for the CA IV it is not present in the CT. The NHE3, NBC1 AE1, Rhcg (1a and 1b), Rhbg and NKA are found in all segments. The V-type H+ ATPase are found only in the PT, and the H+, K+ ATPase only in the CT. Apical (orange), basolateral (blue) and multiple locations (dark red) are shown. The CAc (grey) is found in the cytosol of the cell.
Since the mid-2000, investments in larger and technologically more sophisticated recirculating aquaculture systems (RAS) have increased significantly, with the emergence of new production related challenges and risks (Daalsgard et al., 2013; Fossmark et al., 2021; Davidson et al., 2023). Recycling water permits reduced consumption of intake water per unit biomass produced, constant high temperature, use of continuous light and increased salinity which has resulted in increased average smolt size, from 50 to 100 g in 2008 to approximately 300–500 g in 2022, with several companies aiming to produce smolts up to 600–800 g and future prospects of a 1.000 g fish (Ytresøyl et al., 2023). Despite RAS facilities providing greater control of rearing conditions, the industry has experienced increased incidents of disease-related production disorders such as mineral precipitation in the kidneys (Fivelstad et al., 2018; Klykken et al., 2022a; Klykken et al., 2022b) and hemorrhagic smolt syndrome (Nylund et al., 2003; Krasnov et al., 2019), which have negative implications for growth, health and general performance during the land-based phase (Sommerset et al., 2022). These production disorders are likely a consequence of the rapid changes in technology from flow-through systems (FTS) to RAS, and associated with this, altered rearing protocols for more intensive production (changes in photoperiod, respiratory gas levels, temperature, salinity, stocking density, feed composition and use of buffers and alkalizing reagents). These may ultimately change the overall chemistry of the culture water to sub-optimal conditions which in turn may result in physiological perturbations. However, nephrocalcinosis appear to an issue also in FTS systems (Klykken et al., 2022a). Oxygenation of rearing water without removal of accumulated CO2 combined with high fish density and potentially low water renewal rate may result in increased blood PCO2 and respiratory acidosis associated with both hypercapnia and hyperoxia (Skov, 2019; McArley et al., 2021). Complex interactions occur between pH, temperature and salinity and the formation of carbonate compounds, often referred to as the carbonate system. This is normally affected by the partial pressure of dissolved carbon dioxide (PCO2), and the concentrations of bicarbonate ion (HCO3−), carbonate (CO32-) ion, hydrogen ion (H+) (usually expressed indirectly as the negative logarithm, pH), dissolved inorganic carbon (DIC) and the total alkalinity (TA).
Moving juvenile fish from FTS to RAS gives a larger degree of control, yet more demanding technology and complex interactions in pH, temperature, alkalinity, oxygen saturation and suspended solids, which in turn have direct consequences on dissolved total CO2 (DIC), nitrogen, ammonia and nitrate (Daalsgard et al., 2013; Becke et al., 2020; Fossmark et al., 2021; Davidson et al., 2023). In addition, the use of salinity (in feed or water) has implications on kidney function (Hickman and Trump, 1969; Kodzhahinchev et al., 2017) and the cardiovascular system (Olson and Hoagland, 2008; Brijs et al., 2017; Sundell et al., 2018). In humans these systems are intrinsically connected, and the same is probably true in fish. For example, chronic kidney disease (CKD) impacts the cardiovascular system and increases the risk of heart failure (Said and Hernandez, 2014; Mullens et al., 2020). We do not yet know whether chronic long-term nephrocalcinosis has similar impacts on the cardiovascular system in fish.
Nephrocalcinosis, a disease of aquaculture that has been known for almost 50 years (Landhoilt, 1975), is a renal pathology where a mechanistic understanding of the kidney`s function in acid-base regulation will be critical. Nephrocalcinosis is a widespread disorder found in salmonids (Gillespie and Evans, 1979; Harrison and Richards, 1979; Smart et al., 1979; Fivelstad et al., 1999; 2003; 2018; Klykken et al., 2022a; Minarova et al., 2023), Nile tilapia (Oreochromis niloticus; Chen et al., 2001; 2003), sea bass (Dicentrarchus labrax; Arciuli et al., 2015), Atlantic cod (Gadus morhua; Damsgard et al., 2011), spotted wolffish (Anarhichas minor; Foss et al., 2003; Béland et al., 2020), clownfish (Amphiprion ocellaris; Blazer and Wolke, 1983), cobia (Rachycentron canadum; Klosterhoff et al., 2015), southern flounder (Paralichthys lethostigma; Appelgate et al., 2016), Atlantic wolffish (Anarhichas lupus; Béland et al., 2020), longsnout seahorse (Hippocampus reidi Ginsburg; Lewisch, et al., 2013), lumpfish (Cyclopterus lumpus) and ballan wrasse (Labrus bergylta) (Erkinharju et al., 2021), and is listed as one of the most prevalent perturbations in Norwegian salmonid culture, particularly with respect to land-based productions of large smolt and post-smolts (Sommerset et al., 2022). The physiological perturbations underlying precipitation of minerals in the kidneys are probably related to elevated concentrations in the diet or in the water of the constituents involved in forming the precipitate, principally calcium, magnesium, and phosphate (Smart et al., 1979), and/or because changes in the water chemistry cause changes in internal physiology which promote the formation of these “kidney stones”. Bacterial and viral pathogens are probably not the primary cause (Landholt, 1975), but there is a need to characterize the microbiome associated with the precipitates, as well as their precise mineral composition in fish raised in culture waters of different composition and fed diets of different composition. According to Klykken et al. (2022a), there are at least 5 different chemical compositions for kidney stones in Atlantic salmon, involving precipitates of calcium, magnesium, phosphate, and carbonate. The most prevalent was amorphous carbonate apatite (Ca10(PO4)6CO3).
In mammals the carbonate apatite (Ca10(PO4)6CO3) forms and precipitates at a pH ≥ 6.8 indicating that there is a specific pH threshold in the pre-urine/urine that needs to be sustained in order for stones to form or not form (Olszynski et al., 2015). Similarly, struvite (MgNH4PO4 6H2O) is commonly found in both mammals and salmonids alike (Olzinsky et al., 2015; Klykken et al., 2022a), and in mammals this appears to form and precipitate at pH ≥ 7.2 (Olzinsky et al., 2015). As noted earlier, urine pHs are generally higher in FW fish (similar to blood plasma pH, 7.0—8.0) while the urine pH is more acidic in marine fish, 1.0–2.0 pH units below that of the blood plasma (Hickman and Trump, 1969). This may be one reason why nephrocalcinosis generally has been observed to be reduced when salmonids have been transported to SW (Klykken et al., 2022a). However, little is known about the specific pH threshold for stone formation in the urine of salmonids. More studies are required to better understand changes in urine pH, but the interpretation of such results should be done with caution as pH changes in the final urine may not reflect pH changes locally in the different segments of the nephron where stones likely starts to form. In vitro experiments recreating a similar environment (artificial urine), similar to those performed by Olzinsky et al. (2015), are needed in fish. Environmental changes that increase the urinary pH of salmonids may be a significant risk factor, as higher pH in mammals definitely increased precipitation events of both carbonate apatite and struvite.
The association between nephrocalcinosis and elevated water PCO2 (hypercapnia) was originally documented by Smart et al. (1979) and has now been confirmed by numerous studies (e.g.,; Fivelstad et al., 1999; 2003; Foss et al., 2003; Hosfeld et al., 2008; Petochi et al., 2011). A recent review (Skov, 2019) outlined effects of environmental hypercapnia in fish culture. Elevated PCO2 in the production water will initially affect oxygen transport and acid-base regulation, which in turn may result in increased prevalence of nephrocalcinosis. When hypercapnia is combined with hyperoxia, the incidence of nephrocalcinosis increases, presumably due to greater PCO2 build-up in the fish (Wood and Jackson, 1980; Hobe et al., 1984; Wood, 1991b; Skov, 2019), which causes respiratory acidosis. In turn, the fish compensates by increasing the concentrations of inorganic phosphate, total ammonia, and acidic equivalents in the finally excreted urine, thereby retaining and raising blood plasma HCO3− concentrations to compensate the respiratory acidosis as outlined earlier (Wood and Jackson, 1980; Wheatly et al., 1984; Perry et al., 1987; Wood, 1991b; Wood et al., 1999). Urine pH falls only slightly due to the buffering action of ammonia and phosphate, and urinary calcium and magnesium concentrations increase only slightly (Wheatly et al., 1984; Perry et al., 1987). A recent diagnostic case study reported 5-fold greater PCO2 and 10-fold greater HCO3− concentration in the blood plasma of rainbow trout that were afflicted with a high incidence of nephrocalcinosis in an aquaculture facility with water hypercapnia and hyperoxia (Minarova et al., 2023). Hence, while HCO3− concentrations may not be high in the finally excreted urine, they may be excessively high in the primary urine formed by glomerular filtration, as well as in the surrounding peritubular blood plasma. This, combined with greatly elevated PCO2, ammonia and phosphate, and slightly elevated calcium and magnesium concentrations in the urine may be important for mineral precipitates to form and grow. A model, incorporating this idea, is offered in the next section and Figure 5.
FIGURE 5. Hypothesis on the physiological and molecular mechanisms leading to the formation and aggregation of kidney stones in nephrocalcinosis. During elevated PCO2 and PO2 levels fish will experience chronic respiratory acidosis where blood pH is reduced and where the pH has been compensated by HCO3- retention for which the kidney tubules have a key compensatory role. If water PCO2 and PO2 drop in the tank then PCO2 in the blood plasma and primary urine will quickly fall while HCO3- in the plasma and primary urine will stay high for some time. The pH will quickly rise in the blood plasma and primary urine during this period, creating optimal conditions for nucleation, precipitation, and aggregation events to occur in urine that still has elevated Ca2+, Mg2+, NH4+, HPO42- and HCO3- concentrations. During this event the kidney stones will start forming at pH above 6.8 (carbonate apatite; Ca10(PO4)6CO3) and 7.2 (struvite; MgNH4PO4 ·6H2O). This may be the proximate trigger mechanism. Increased salt load in the water (12-15 ppt), salted feed and/or calcium and sodium bicarbonate buffers may further increase the Ca2+, Mg2+ and HCO3- having a cumulative effect. For more details regarding the transport pathways the reader is referred to Figure 1, Figure 2, Figure 3.
Although environmental hypercapnia causes acid-base perturbations and is a well-established risk factor for development of nephrocalcinosis in farmed salmon (Skov, 2019), it is clearly not the only culprit. Atlantic salmon post-smolts exposed to high total CO2 levels (5–40 mg L-1; see Skov et al. , 2019, for conversion to PCO2 units) and brackish water (12 ppt) for 12 weeks did not exhibit any signs of nephrocalcinosis until after 6 weeks in full strength seawater (<5 mg L-1 CO2; 34 ppt) (Mota et al., 2019; 2020). Similarly, Good and co-authors (2018) found no evidence of nephrocalcinosis in Atlantic salmon exposed to 20 mg L-1 CO2 for 384 days. Salmon in the latter study experienced higher water alkalinity (233–241 mg L-1) and freshwater conditions, while seawater alkalinity ranged between 116 and 165 mg L-1 in the study by Mota and co-authors (2019). Both studies used NaHCO3 buffer to maintain alkalinity. Interestingly, Nile tilapia reared in water buffered with NaHCO3 exhibited higher plasma HCO3− concentrations and only modest prevalence of nephrocalcinosis whilst tilapia reared in FW buffered by CaCO3 displayed the opposite response, low plasma HCO3− concentrations and high prevalence of nephrocalcinosis (Chen et al., 2001). Hence, both salinities and the specific composition of the ions in the water may affect the prevalence of nephrocalcinosis differently. Divalent cations (Ca2+, Mg2+) and the divalent anion (HPO42-) in the water and/or feed are probably important risk factors. Elevated Ca2+ and Mg2+ are often a result of adding seawater to increase rearing salinity (Table 2). Additionally, the use of calcium-containing buffers and/or increased salt load via the feed may have osmoregulatory impacts, including kidney dysfunction. How much of an impact depends on type of ions and concentrations and the manner in which the fish are exposed (i.e., via the feed or via the water).
TABLE 2. General overview of water quality parameteres (ranges) in modern freshwater (FW) and brackish water (BW) recirculating aquaculture systems (RAS) in Norway.
In mammals, low GFR has been identified as one of several causes underlying the formation of kidney stones (Gillen et al., 2005; Worcester et al., 2006) but also high dietary salt and protein intake (Ferraro et al., 2020). Interestingly, GFR in freshwater acclimated fish is high, producing copious amounts of dilute urine (i.e., high UFR), while GFR in seawater acclimated fish is lower, resulting in low UFR of a urine rich in divalent ions (Hickman, 1968a; 1968b; Takvam et al., 2021a). Based on the mammalian model (Gillen et al., 2005; Worcester et al., 2006; Ferraro et al., 2020), the expectations would be that more salinity (through feed and water) would reduce GFR and be a risk factor for developing nephrocalcinosis. Commercial diets often contain high salt levels (Na+, Cl−, Ca2+, Mg2+ and inorganic phosphate). Furthermore, commercial feed given to fish in the freshwater stage usually contains ingredients from marine sources which increases the salt load (Shaw et al., 2011; Phillip et al., 2022). From a biological standpoint, this is unnatural for fish in freshwater. In freshwater, fish usually drink very little. Increased salinity of the water will increase drinking rate, though the threshold for this effect is unclear (Damsgaard et al., 2020), and increased salt content of the food will have the same effect (Pyle et al., 2003). Interestingly in mammals, higher salt intake appears to be a big risk factor while drinking adequate levels of water appears to somewhat offset the risks of developing kidney stones (Ferraro et al., 2020). How many of these mechanisms in the mammalian model that can be translated to the fish model is unclear, but it is noteworthy that nephrocalcinosis in euryhaline salmonids, has been observed in both freshwater and in brackish water. Although brackish water (12–15 ppt) has been pointed out as a potential risk factor (Klykken et al., 2022a), other triggers are in play earlier in the production as farmers report incidences of nephrocalcinosis down to 7 g fish which may point to components in the feed. Future research should address these interactions between salt load (water and feed), urine production rates (GFR and UFR) and link this to water chemistry levels discussed here at various specific life-cycle stages during production. Yet, the proximate mechanism by which nephrocalcinosis develops in teleost fishes remains unknown almost 50 years after its first discovery (Landholt, 1975).
In light of our understanding of both ion and water homeostasis (Takvam et al., 2021a) and acid-base balance (current review), we suggest a new model for this proximate mechanism (Figure 5). Increased risk of nephrocalcinosis may be coming from the increased salt load in feed (commercial diets; high in Mg2+, Ca2+ and PO42-) and the addition of buffers in the water such as calcium hydroxide (Ca(OH)2) and sodium bicarbonate (NaHCO3) (most commonly used buffers in RAS) in the early freshwater phase. However, we suggest that the key condition for kidney stone formation may start when fish are experiencing elevated PCO2 and PO2 levels in the water of their holding tanks. These fish will be in the condition of a chronic respiratory acidosis where the pH has been compensated by HCO3− retention in the body fluids, which is achieved by excretion of acidic equivalents in the urine (see Figures 1–3 for a recap of the mechanisms). Aquaculture facilities report that nephrocalcinosis often occurs when salmonids held in these conditions are moved to new tanks for grow-out (Sommerset et al., 2022). If water PCO2 and PO2 are lower in the new tanks, then PCO2 in the blood plasma and primary urine will quickly fall while [HCO3−] in the plasma and primary urine will stay high for some time (e.g., Hobe et al., 1984; Wheatly et al., 1984; Perry et al., 1987; Perry et al., 2010). This may be the proximate trigger. The pH will quickly rise in the blood plasma and primary urine during this period, creating optimal conditions for nucleation, precipitation, and aggregation events to occur in urine that still has elevated Ca2+, Mg2+, NH4+, HPO42- and HCO3− concentrations. As discussed earlier, the kidney stones will form at pHs above 6.8 (carbonate apatite) and 7.2 (struvite), and this process can occur in less than 2 h for the former, somewhat longer for the latter (Olyniszy et al., 2015):
To make matters worse, once stones start to form, this will further increase the pH of the pre-urine, thereby accelerating the rate of aggregation (Olzinsky et al., 2015). Once the stones aggregate locally into larger stones in the nephron tubules, inflammation and cell damage will disrupt multiple transport mechanisms, and create mechanical blockages to flow. Reduced urine flow itself will further accelerate stone formation. Keep in mind that this is still very early in the process, and it may take weeks or months for the damages to be visible through the use of X-ray or macro-scoring (Klykken et al., 2022b). The issues may further continue as producers use more salinity in the water (brackish water; 12–15 ppt), salted feed (even higher in salts than commercial feed) and/or calcium and bicarbonate buffers (only in RAS) which may further increase Ca2+, Mg2+ and HCO3− levels, having a cumulative effect. Long-term pathophysiological disturbances in ion and water regulation, acid-base regulation, hormone balance, immune response and metabolism will occur, impacting overall fish health and welfare. In the future, better understanding of the urine pH in different environmental settings and pH thresholds for aggregation of kidney stones of different composition will be useful. As these aggregation processes likely start very early, disrupting transport mechanisms in the nephron, an important objective will be to identify key biomarkers involved in the different transport pathways that may help us detect disturbances very early, such that adjustments in O2 and CO2 levels, water chemistry, and feed composition can be done early in development in modern aquaculture.
Section-specific profiling of gene and protein expression in all sections of the fish nephron is a promising advance. The detailed localization of the relevant transporters in acid-base, ammonia, phosphate, calcium, and magnesium regulation to the specific segments (proximal I-, proximal II-, distal-, collecting tubule and collecting duct) provides important information. This can be done through either manual separation (Fehsenfeld and Wood, 2018) or laser capture micro-dissection (Madsen et al., 2020). Combining such methods with the application of scanning ion-selective electrode techniques (SIET; Fehsenfeld et al., 2018) and/or electrophysiology (Kurita et al., 2008; Weiner and Verlander, 2011) using various experimental pharmacological inhibitors/activators and pre-treatments should be pursued with a range of teleost species to better understand the function of these different transport pathways. Additionally, single-cell technologies such as RNAseq and transcriptomic profiling of specific nephron regions would be beneficial to better understand transport mechanisms in physiology and during the development of kidney disease (Potter, 2018). These technologies are already being applied in clinical nephropathology in humans where the main drawbacks at this point are high costs per sample and analytical complexity (Stewart and Clatworthy, 2020).
Advances in immunochemical and molecular approaches in the past decade have been accompanied by a decline in the use of more traditional physiological methods, approaches that give a more holistic understanding of kidney function in fish at the whole organism level. Only by utilizing catheterization techniques can you collect urine, measure urine flow rate (UFR) and glomerular filtration rate (GFR), whereby the researcher can determine the rates of excretion, secretion, reabsorption, and plasma clearance of different solutes (e.g., acid-base equivalents, ammonia, phosphate, etc.) in fish (Wood and Patrick, 1994). This becomes a very powerful approach when combined with either section-specific mRNA localization and expression (in situ hybridization) and/or protein localization (immunohistochemistry and Western blot), and/or SIET analysis of transport rates and/or single-cell technologies, all of which are useful when finding the location of the target solute carrier or channel. These can often also identify whether the transporter is located on the apical or basolateral membrane of the tubule cell. Several of the transporters addressed in the current review are likely located in the same cell(s). Thus, co-localization of transporters/channels is another approach that would deepen our understanding of the co-operative actions these have in dealing with acid-base, ammonia and phosphate regulation. We now know, for example, that Rh proteins, Na+ and H+ transport pathways, and carbonic anhydrase are organized into a functional metabolon in the freshwater gill (Wright and Wood, 2009; Ito et al., 2013). The same may be true for these and other transport systems in the fish kidneys (Takvam et al., 2021a). In addtion, a better understanding of paracellular transport (tight junctions proteins; claudins) in conjuction to the trancellular transport (adressed in this review) and their role in acid-base balance, which convincingly have been demonstrated to play an important role in the goldfish kidneys (Figure 3; Fehsenfeld et al., 2019). Future studies should include the individual and collective roles of paracellular and trancellular transport pathways in the fish kidneys. In the future these integrative approaches should be combined in order to better understand complex issues in fish. For example, formation of kidney stones (nephrocalcinosis) is a major issue in intensive aquaculture but so far approaches have usually focused on diagnostic tools (histopathology, blood chemistry, X-ray analyses e.g., Harrison and Richards, 1979; Klykken et al., 2022a; Klykken et al., 2022b; Minarova et al., 2023) rather than on understanding the underlying molecular and physiological mechanisms or the consequences of the disease for normal renal function. While such diagnostic approaches can provide very useful tools to detect the disease, they bring us no closer to finding the root cause of the problem, which requires more integrative understanding on how these transport systems work in the kidneys. The collection of extensive water chemistry and feed composition data in combination with this integrative understanding would be valuable towards solving the problem.
Research on mammalian models is more extensive and often better funded due to the many underlying pathological conditions of renal physiology in humans. Fish researchers can learn a great deal from following the mammalian kidney literature as highlighted in several previous sections in this review. However, benefits may also go in the opposite direction: the ability of euryhaline fishes to rapidly adapt to very different environments, and the plasticity of their kidneys where drastic changes in filtration and flow rates (GFR and UFR) as well as substantial shifts (upregulation, downregulation, and net reversal of direction) in their transport systems may provide a powerful experimental model. For example, human kidney disease is often related to reductions in glomerular filtration rates and changes in transport mechanisms. Normal teleost kidney function in freshwater (high GFRs and UFRs) may be a proxy for normal function of the human kidneys, whereas normal teleost kidney function in seawater (low GFRs and UFRs) may be a proxy for abnormal function of the human kidneys. The ability of many teleost fishes to acclimate to high ammonia, hypercapnic, hyperoxic, hypoxic, acidic, and alkaline environments is remarkable, and renal acid-base regulatory function plays a key role in these adjustments. Human diseases ranging from obstructed breathing to liver failure and diabetic coma present some of these same physiological impacts on the human kidneys. Indeed, most of the protein domains related to kidney diseases in humans are present in fish which is why the zebrafish is one of the most popular experimental models for research for human disease (e.g., Bradford et al., 2017). Collectively, increasing our knowledge on the kidneys both at a molecular and physiological levels will be beneficial in addressing these issues in the future as kidney disease is a large and ongoing problem in both mammals and fish.
CW and MT drafted the first version of the manuscript. TN actively contributed to writing of the manuscript. HK made the figures supported by MT, TN, and CW. All authors contributed to the article and approved the submitted version.
CW’s contribution was supported by NSERC (Canada) Discovery grants (RGPIN-2017-03843 and RGPIN-2023-03714). TN, MT, and HK’s contribution was supported by Norwegian Research council (NORDFORSK-332568).
The authors declare that the research was conducted in the absence of any commercial or financial relationships that could be construed as a potential conflict of interest.
All claims expressed in this article are solely those of the authors and do not necessarily represent those of their affiliated organizations, or those of the publisher, the editors and the reviewers. Any product that may be evaluated in this article, or claim that may be made by its manufacturer, is not guaranteed or endorsed by the publisher.
Applegate, J. R., Lewbart, G. A., Daniels, H., Gill, A., and Stoskopf, M. K. (2016). Calcium urolithiasis in a breeding population of southern flounder (Paralichthys lethostigma) housed in a low salinity environment. Veterinary Q. 36 (1), 50–54. doi:10.1080/01652176.2015.1123823
Arciuli, M., Brunetti, A., Fiocco, D., Zacchino, V., Centoducati, G., Aloi, A., et al. (2015). A multidisciplinary study of the extracutaneous pigment system of European sea bass (Dicentrarchus labrax L). A possible relationship between kidney disease and dopa oxidase activity level. Fish Shellfish Immunol. 42 (1), 184–192. doi:10.1016/j.fsi.2014.10.031
Barnawi, E. A., Doherty, J. E., Ferreira, P. G., and Wilson, J. M. (2020). Extra-gastric expression of the proton pump H+/K+-ATPase in the gills and kidney of the teleost Oreochromis niloticus. J. Exp. Biol. J. Exp. Biol. 223 (16), jeb214890. doi:10.1242/jeb.214890
Beamish, F. W. H., and Tandler, A. (1990). Ambient ammonia, diet and growth in lake trout. Aquat. Toxicol. 17 (2), 155–166. doi:10.1016/0166-445X(90)90028-N
Becke, C., Schumann, M., Geist, J., and Brinker, A. (2020). Shape characteristics of suspended solids and implications in different salmonid aquaculture production systems. Aquaculture 516, 734631. doi:10.1016/j.aquaculture.2019.734631
Béland, K., Wong, E., St-Cyr, J. F., and Lair, S. (2020). High occurrence rate of xanthomatosis and nephrocalcinosis in aquarium-housed Atlantic wolffish Anarhichas lupus and spotted wolffish A. minor. Dis. Aquatic Org. 139, 223–232. doi:10.3354/dao03477
Beyenbach, K. W., and Kirschner, L. B. (1975). Kidney and urinary bladder functions of the rainbow trout in Mg and Na excretion. Am. J. Physiology-Legacy Content 229 (2), 389–393. doi:10.1152/ajplegacy.1975.229.2.389
Blazer, V. S., and Wolke, R. E. (1983). Ceroid deposition, retinal degeneration and renal calcium oxalate crystals in cultured clownfish, Amphiprion ocellaris. J. Fish Dis. 6 (4), 365–376. doi:10.1111/j.1365-2761.1983.tb00088.x
Bradford, Y. M., Toro, S., Ramachandran, S., Ruzicka, L., Howe, D. G., Eagle, A., et al. (2017). Zebrafish models of human disease: Gaining insight into human disease at ZFIN. ILAR J. 58 (1), 4–16. doi:10.1093/ilar/ilw040
Brijs, J., Sandblom, E., Dekens, E., Näslund, J., Ekström, A., and Axelsson, M. (2017). Cardiac remodeling and increased central venous pressure underlie elevated stroke volume and cardiac output of seawater-acclimated rainbow trout. Am. J. Physiology-Regulatory, Integr. Comp. Physiology 312 (1), R31-R39–R39. doi:10.1152/ajpregu.00374.2016
Bucking, C., Landman, M. J., and Wood, C. M. (2010). The role of the kidney in compensating the alkaline tide, electrolyte load, and fluid balance disturbance associated with feeding in the freshwater rainbow trout, Oncorhynchus mykiss. Comp. Biochem. Physiology Part A Mol. Integr. Physiology 156 (1), 74–83. doi:10.1016/j.cbpa.2009.12.021
Bucking, C., and Wood, C. M. (2008). The alkaline tide and ammonia excretion after voluntary feeding in freshwater rainbow trout. J. Exp. Biol. 211 (15), 2533–2541. doi:10.1242/jeb.015610
Byrne, P. J., MacPhee, D. D., Ostland, V. E., Johnson, G., and Ferguson, H. W. (1998). Haemorrhagic kidney syndrome of Atlantic salmon, Salmo salar L. J. Fish Dis. 21 (2), 81–91. doi:10.1046/j.1365-2761.1998.00071.x
Cameron, J. N. (1980). Body fluid pools, kidney function, and acid-base regulation in the freshwater catfish Ictalurus punctatus. J. Exp. Biol. 86 (1), 171–185. doi:10.1242/jeb.86.1.171
Cameron, J. N., and Kormanik, G. A. (1982). The acid-base responses of gills and kidneys to infused acid and base loads in the channel catfish, Ictalurus punctatus. J. Exp. Biol. 99 (1), 143–160. doi:10.1242/jeb.99.1.143
Cameron, J. N., and Wood, C. M. (1978). Renal function and acid–base regulation in two amazonian erythrinid fishes: Hoplias malabaricus, a water breather, and hoplerythrinus unitaeniatus, a facultative air breather. Can. J. Zoology 56 (4), 917–930. doi:10.1139/z78-127
Chen, C. Y., Wooster, G. A., Getchell, R. G., Bowser, P. R., and Timmons, M. B. (2003). Blood chemistry of healthy, nephrocalcinosis-affected and ozone-treated tilapia in a recirculation system, with application of discriminant analysis. Aquaculture 218 (1-4), 89–102. doi:10.1016/S0044-8486(02)00499-4
Chen, C. Y., Wooster, G. A., Getchell, R. G., Bowser, P. R., and Timmons, M. B. (2001). Nephrocalcinosis in nile tilapia from a recirculation aquaculture system: A case report. J. Aquatic Animal Health 13 (4), 368–372. doi:10.1577/1548-8667(2001)013<0368:NINTFA>2.0.CO;2
Coloso, R. M., King, K., Fletcher, J. W., Weis, P., Werner, A., and Ferraris, R. P. (2003). Dietary P regulates phosphate transporter expression, phosphatase activity, and effluent P partitioning in trout culture. J. Comp. Physiology B 173, 519–530. doi:10.1007/s00360-003-0360-x
Cooper, C. A., Wilson, J. M., and Wright, P. A. (2013). Marine, freshwater and aerially acclimated mangrove rivulus (Kryptolebias marmoratus) use different strategies for cutaneous ammonia excretion. Am. J. Physiology-Regulatory, Integr. Comp. Physiology 304 (8), R599–R612. doi:10.1152/ajpregu.00228.2012
Cooper, C. A., and Wilson, R. W. (2008). Post-prandial alkaline tide in freshwater rainbow trout: Effects of meal anticipation on recovery from acid–base and ion regulatory disturbances. J. Exp. Biol. 211 (15), 2542–2550. doi:10.1242/jeb.015586
Curtis, B. J., and Wood, C. M. (1991). The function of the urinary bladder in vivo in the freshwater rainbow trout. J. Exp. Biol. 155 (1), 567–583. doi:10.1242/jeb.155.1.567
Curtis, J. B., and Wood, C. M. (1992). Kidney and urinary bladder responses of freshwater rainbow trout to isosmotic NaCl and NaHCO3 infusion. J. Exp. Biol. 173 (1), 181–203. doi:10.1242/jeb.173.1.181
Dalsgaard, J., Lund, I., Thorarinsdottir, R., Drengstig, A., Arvonen, K., and Pedersen, P. B. (2013). Farming different species in RAS in Nordic countries: Current status and future perspectives. Aquac. Eng. 53, 2–13. doi:10.1016/j.aquaeng.2012.11.008
Damsgaard, C., McGrath, M., Wood, C. M., Richards, J. G., and Brauner, C. J. (2020). Ion-regulation, acid/base-balance, kidney function, and effects of hypoxia in coho salmon,Oncorhynchus kisutch, after long-term acclimation to different salinities. Aquaculture 528, 735571. doi:10.1016/j.aquaculture.2020.735571
Damsgård, B., Bjørklund, F., Johnsen, H. K., and Toften, H. (2011). Short-and long-term effects of fish density and specific water flow on the welfare of Atlantic cod, Gadus morhua. Aquaculture 322, 184–190. doi:10.1016/j.aquaculture.2011.09.025
Davenport, H. W. (1974). The ABC of acid-base chemistry: The elements of physiological blood-gas chemistry for medical students and physicians. University of Chicago Press.
Davidson, J., Redman, N., Crouse, C., and Vinci, B. (2023). Water quality, waste production, and off-flavor characterization in a depuration system stocked with market-size Atlantic salmon Salmo salar. J. World Aquac. Soc. 54 (1), 96–112. doi:10.1111/jwas.12920
Deigweiher, K., Koschnick, N., Portner, H. O., and Lucassen, M. (2008). Acclimation of ion regulatory capacities in gills of marine fish under environmental hypercapnia. Am. J. Physiology-Regulatory, Integr. Comp. Physiology 295 (5), R1660–R1670. doi:10.1152/ajpregu.90403.2008
Elger, M., Werner, A., Herter, P., Kohl, B., Kinne, R. K. H., and Hentschel, H. (1998). Na-Pi cotransport sites in proximal tubule and collecting tubule of winter flounder (Pleuronectes americanus). Am. J. Physiology-Renal Physiology 274 (2), F374–F383. doi:10.1152/ajprenal.1998.274.2.F374
Ellis, R. P., Urbina, M. A., and Wilson, R. W. (2017). Lessons from two high CO 2 worlds–future oceans and intensive aquaculture. Glob. change Biol. 23 (6), 2141–2148. doi:10.1111/gcb.13515
Erkinharju, T., Dalmo, R. A., Hansen, M., and Seternes, T. (2021). Cleaner fish in aquaculture: Review on diseases and vaccination. Rev. Aquac. 13 (1), 189–237. doi:10.1111/raq.12470
Fehsenfeld, S., Kolosov, D., Wood, C. M., and O'Donnell, M. J. (2019). Section-specific H+ flux in renal tubules of fasted and fed goldfish. J. Exp. Biol. 222 (13), jeb200964. doi:10.1242/jeb.200964
Fehsenfeld, S., and Wood, C. M. (2020). A potential role for hyperpolarization-activated cyclic nucleotide-gated sodium/potassium channels (HCNs) in teleost acid-base and ammonia regulation. Comp. Biochem. Physiology Part B Biochem. Mol. Biol. 248, 110469. doi:10.1016/j.cbpb.2020.110469
Fehsenfeld, S., and Wood, C. M. (2018). Section-specific expression of acid-base and ammonia transporters in the kidney tubules of the goldfish Carassius auratus and their responses to feeding. Am. J. Physiology-Renal Physiology 315 (6), F1565-F1582–F1582. doi:10.1152/ajprenal.00510.2017
Ferraro, P. M., Bargagli, M., Trinchieri, A., and Gambaro, G. (2020). Risk of kidney stones: Influence of dietary factors, dietary patterns, and vegetarian–vegan diets. Nutrients 12 (3), 779. doi:10.3390/nu12030779
Fivelstad, S., Hosfeld, C. D., Medhus, R. A., Olsen, A. B., and Kvamme, K. (2018). Growth and nephrocalcinosis for Atlantic salmon (Salmo salar L) post-smolt exposed to elevated carbon dioxide partial pressures. Aquaculture 482, 83–89. doi:10.1016/j.aquaculture.2017.09.012
Fivelstad, S., Olsen, A. B., Åsgård, T., Baeverfjord, G., Rasmussen, T., Vindheim, T., et al. (2003). Long-term sublethal effects of carbon dioxide on atlantic salmon smolts (Salmo salar L): Ion regulation, haematology, element composition, nephrocalcinosis and growth parameters. Aquaculture 215 (1-4), 301–319. doi:10.1016/S0044-8486(02)00048-0
Fivelstad, S., Olsen, A. B., Kløften, H., Ski, H., and Stefansson, S. (1999). Effects of carbon dioxide on Atlantic salmon (Salmo salar L) smolts at constant pH in bicarbonate rich freshwater. Aquaculture 178 (1-2), 171–187. doi:10.1016/S0044-8486(99)00125-8
Fletcher, C. R. (1990). Urine production and urination in the plaice Pleuronectes platessa. Comp. Biochem. Physiology Part A Physiology 96 (1), 123–129. doi:10.1016/0300-9629(90)90052-T
Foss, A., Røsnes, B. A., and Øiestad, V. (2003). Graded environmental hypercapnia in juvenile spotted wolffish (anarhichas minor olafsen): Effects on growth, food conversion efficiency and nephrocalcinosis. Aquaculture 220 (1-4), 607–617. doi:10.1016/S0044-8486(02)00613-0
Fossmark, R. O., Attramadal, K. J., Nordøy, K., Østerhus, S. W., and Vadstein, O. (2021). A comparison of two seawater adaptation strategies for Atlantic salmon post-smolt (Salmo salar) grown in recirculating aquaculture systems (RAS): Nitrification, water and gut microbiota, and performance of fish. Aquaculture 532, 735973. doi:10.1016/j.aquaculture.2020.735973
Fryer, J. L., and Sanders, J. E. (1981). Bacterial kidney disease of salmonid fish. Annu. Rev. Microbiol. 35 (1), 273–298. doi:10.1146/annurev.mi.35.100181.001421
Georgalis, T., Gilmour, K. M., Yorston, J., and Perry, S. F. (2006). Roles of cytosolic and membrane-bound carbonic anhydrase in renal control of acid-base balance in rainbow trout, Oncorhynchus mykiss. Am. J. Physiology-Renal Physiology 291 (2), F407–F421. doi:10.1152/ajprenal.00328.2005
Gillen, D. L., Worcester, E. M., and Coe, F. L. (2005). Decreased renal function among adults with a history of nephrolithiasis: A study of NHANES III. Kidney Int. 67 (2), 685–690. doi:10.1111/j.1523-1755.2005.67128.x
Gillespie, D. C., and Evans, R. E. (1979). Composition of granules from kidneys of rainbow trout (Salmo gairdneri) with nephrocalcinosis. J. Fish. Board Can. 36 (6), 683–685. doi:10.1139/f79-100
Gilmour, K. M., and Perry, S. F. (2009). Carbonic anhydrase and acid–base regulation in fish. J. Exp. Biol. 212 (11), 1647–1661. doi:10.1242/jeb.029181
Gilmour, K. M., Perry, S. F., Esbaugh, A. J., Genz, J., Taylor, J. R., and Grosell, M. (2012). Compensatory regulation of acid–base balance during salinity transfer in rainbow trout (Oncorhynchus mykiss). J. Comp. Physiology B 182, 259–274. doi:10.1007/s00360-011-0617-8
Good, C., Davidson, J., Terjesen, B. F., Takle, H., Kolarevic, J., Bæverfjord, G., et al. (2018). The effects of long-term 20 mg/L carbon dioxide exposure on the health and performance of Atlantic salmon Salmo salar post-smolts in water recirculation aquaculture systems. Aquac. Eng. 81, 1–9. doi:10.1016/j.aquaeng.2018.01.003
Grosell, M., Mager, E. M., Williams, C., and Taylor, J. R. (2009). High rates of HCO3– secretion and Cl– absorption against adverse gradients in the marine teleost intestine: The involvement of an electrogenic anion exchanger and H+-pump metabolon? J. Exp. Biol. 212 (11), 1684–1696. doi:10.1242/jeb.027730
Gupta, A., and Renfro, J. L. (1989). Control of phosphate transport in flounder renal proximal tubule primary cultures. Am. J. Physiology-Regulatory, Integr. Comp. Physiology 256 (4), R850–R857. doi:10.1152/ajpregu.1989.256.4.R850
Gupta, A., and Renfro, J. L. (1991). Effects of pH on phosphate transport by flounder renal tubule primary cultures. Am. J. Physiology-Regulatory, Integr. Comp. Physiology 260 (4), R704–R711. doi:10.1152/ajpregu.1991.260.4.R704
Harrison, J. G., and Richards, R. H. (1979). The pathology and histopathology of nephrocalcinosis in rainbow trout Salmo gairdneri Richardson in fresh water. J. Fish Dis. 2 (1), 1–12. doi:10.1111/j.1365-2761.1979.tb00134.x
Hedrick, R. P., MacConnell, E., and De Kinkelin, P. (1993). Proliferative kidney disease of salmonid fish. Annu. Rev. Fish Dis. 3, 277–290. doi:10.1016/0959-8030(93)90039-E
Hickman, C. P. (1968a). Glomerular filtration and urine flow in the euryhaline southern flounder, Paralichthys lethostigma, in seawater. Can. J. Zoology 46 (3), 427–437. doi:10.1139/z68-061
Hickman, C. P. (1968b). Urine composition and kidney tubular function in southern flounder, Paralichthys lethostigma, in sea water. Can. J. zoology 46 (3), 439–455. doi:10.1139/z68-062
Hickman, C. P., and Trump, B. F. (1969). “The kidney,” in Fish physiology. Editors W. S. Hoar, and D. J. Randall (Cambridge, CA: Academic), 91–239. doi:10.1016/S1546-5098(08)60083-7
Hills, A. G. (1973). Acid-base balance; chemistry, physiology, pathophysiology. New York: Williams & Wilkins.
Hirata, T., Kaneko, T., Ono, T., Nakazato, T., Furukawa, N., Hasegawa, S., et al. (2003). Mechanism of acid adaptation of a fish living in a pH 3.5 lake. Am. J. Physiology-Regulatory, Integr. Comp. Physiology 284 (5), R1199–R1212. doi:10.1152/ajpregu.00267.2002
Hōbe, H., Wood, C. M., and Wheatly, M. G. (1984). The mechanisms of acid-base and ionoregulation in the freshwater rainbow trout during environmental hyperoxia and subsequent normoxia. I. Extra-and intracellular acid-base status. Respir. Physiol. 55 (2), 139–154. doi:10.1016/0034-5687(84)90019-7
Hochachka, P. W., Moon, T. W., Bailey, J., and Hulbert, W. C. (1978). The osteoglossid kidney: Correlations of structure, function, and metabolism with transition to air breathing. Can. J. Zoology 56 (4), 820–832. doi:10.1139/z78-114
Hodler, J., Heinemann, H. O., Fishman, A. P., and Smith, H. W. (1955). Urine pH and carbonic anhydrase activity in the marine dogfish. Am. J. Physiology-Legacy Content 183 (1), 155–162. doi:10.1152/ajplegacy.1955.183.1.155
Hosfeld, C. D., Engevik, A., Mollan, T., Lunde, T. M., Waagbø, R., Olsen, A. B., et al. (2008). Long-term separate and combined effects of environmental hypercapnia and hyperoxia in Atlantic salmon (Salmo salar L) smolts. Aquaculture 280 (1-4), 146–153. doi:10.1016/j.aquaculture.2008.05.009
Ip, Y. K., and Chew, S. F. (2010). Ammonia production, excretion, toxicity, and defense in fish: A review. Front. physiology 1, 134. doi:10.3389/fphys.2010.00134
Ito, Y., Kobayashi, S., Nakamura, N., Miyagi, H., Esaki, M., Hoshijima, K., et al. (2013). Close association of carbonic anhydrase (CA2a and CA15a), Na+/H+ exchanger (Nhe3b), and ammonia transporter Rhcg1 in zebrafish ionocytes responsible for Na+ uptake. Front. Physiology 4, 59. doi:10.3389/fphys.2013.00059
Ivanis, G., Braun, M., and Perry, S. F. (2008). Renal expression and localization of SLC9A3 sodium/hydrogen exchanger and its possible role in acid-base regulation in freshwater rainbow trout (Oncorhynchus mykiss). Am. J. Physiology-Regulatory, Integr. Comp. Physiology 295 (3), R971–R978. doi:10.1152/ajpregu.90328.2008
Karlsson, A., Eliason, E. J., Mydland, L. T., Farrell, A. P., and Kiessling, A. (2006). Postprandial changes in plasma free amino acid levels obtained simultaneously from the hepatic portal vein and the dorsal aorta in rainbow trout (Oncorhynchus mykiss). J. Exp. Biol. 209 (24), 4885–4894. doi:10.1242/jeb.02597
Kato, A., and Watanabe, T. (2016). Renal sulfate regulation. Eel Physiol., 249–281. doi:10.1201/b15365-10
Katoh, F., Tresguerres, M., Lee, K. M., Kaneko, T., Aida, K., and Goss, G. G. (2006). Cloning of rainbow trout SLC26A1: Involvement in renal sulfate secretion. Am. J. Physiology-Regulatory, Integr. Comp. Physiology 290 (5), R1468–R1478. doi:10.1152/ajpregu.00482.2005
Kaune, R., and Hentschel, H. (1987). Stimulation of renal phosphate secretion in the stenohaline freshwater teleost: Carassius auratus gibelio bloch. Comp. Biochem. physiology. A, Comp. Physiology 87 (2), 359–362. doi:10.1016/0300-9629(87)90136-8
King, P. A., and Goldstein, L. E. O. N. (1983). Renal ammonia excretion and production in goldfish, Carassius auratus, at low environmental pH. Am. J. Physiology-Regulatory, Integr. Comp. Physiology 245 (4), R590–R599. doi:10.1152/ajpregu.1983.245.4.R590
Klosterhoff, M., Pedrosa, V., Sampaio, L. A., Ramos, L., Tesser, M. B., and Romano, L. A. (2015). Nephrocalcinosis and kidney stones in Rachycentron canadum. Bull. Eur. Assoc. Fish Pathologists 35, 138–147.
Klykken, C., Dalum, A. S., Reed, A. K., Attramadal, K., Olsen, R. E., and Boissonnot, L. (2022b). Radiological detection of nephrocalcinosis in farmed Atlantic salmon Salmo salar L. J. fish Dis. 45 (12), 1883–1888. doi:10.1111/jfd.13704
Klykken, C., Reed, A. K., Dalum, A. S., Olsen, R. E., Moe, M. K., Attramadal, K. J. K., et al. (2022a). Physiological changes observed in farmed Atlantic salmon (Salmo salar L) with nephrocalcinosis. Aquaculture 554, 738104. doi:10.1016/j.aquaculture.2022.738104
Kobayashi, K. A., and Wood, C. M. (1980). The response of the kidney of the freshwater rainbow trout to true metabolic acidosis. J. Exp. Biol. 84 (1), 227–244. doi:10.1242/jeb.84.1.227
Kodzhahinchev, V., Kovacevic, D., and Bucking, C. (2017). Identification of the putative goldfish (Carassius auratus) magnesium transporter SLC41a1 and functional regulation in the gill, kidney, and intestine in response to dietary and environmental manipulations. Comp. Biochem. Physiology Part A Mol. Integr. Physiology 206, 69–81. doi:10.1016/j.cbpa.2017.01.016
Krasnov, A., Sommerset, I., Søfteland, T., Afanasyev, S., Boysen, P., and Lund, H. (2019). Consequences of haemorrhagic smolt syndrome (HSS) for the immune status of atlantic salmon (Salmo salar L)(Case study). Biology 9 (1), 1. doi:10.3390/biology9010001
Kurita, Y., Nakada, T., Kato, A., Doi, H., Mistry, A. C., Chang, M. H., et al. (2008). Identification of intestinal bicarbonate transporters involved in formation of carbonate precipitates to stimulate water absorption in marine teleost fish. Am. J. Physiol. 294, R1402–R1412. doi:10.1152/ajpregu.00759.2007
L'Honoré, T., Farcy, E., Blondeau-Bidet, E., and Lorin-Nebel, C. (2020). Inter-individual variability in freshwater tolerance is related to transcript level differences in gill and posterior kidney of European sea bass. Gene 741, 144547. doi:10.1016/j.gene.2020.144547
Lall, S. P., and Kaushik, S. J. (2021). Nutrition and metabolism of minerals in fish. Animals 11 (09), 2711. doi:10.3390/ani11092711
Landholt, M. L. (1975) “Visceral granuloma and nephrocalcinosis of trout,” in The pathology of fishes. Editors W. E. Ribelin, and G. Migaki (Madison, Wisconsin: The University of Wisconsin Press), 793–799.
Lawrence, M. J., Wright, P. A., and Wood, C. M. (2015). Physiological and molecular responses of the goldfish (Carassius auratus) kidney to metabolic acidosis, and potential mechanisms of renal ammonia transport. J. Exp. Biol. 218 (13), 2124–2135. doi:10.1242/jeb.117689
Leguen, I., Le Cam, A., Montfort, J., Peron, S., and Fautrel, A. (2015). Transcriptomic analysis of trout gill ionocytes in fresh water and sea water using laser capture microdissection combined with microarray analysis. PloS one 10 (10), e0139938. doi:10.1371/JOURNAL.PONE.0139938
Lewisch, E., Kucera, M., Tappert, R., Tessadri, R., Tappert, M., and Kanz, F. (2013). Occurrence of nephrolithiasis in a population of longsnout seahorse, H ippocampus reidi G insburg, and analysis of a nephrolith. J. Fish Dis. 36 (2), 163–167. doi:10.1111/jfd.12013
Madsen, S. S., Bollinger, R. J., Brauckhoff, M., and Engelund, M. B. (2020). Gene expression profiling of proximal and distal renal tubules in Atlantic salmon (Salmo salar) acclimated to fresh water and seawater. Am. J. Physiology-Renal Physiology 319 (3), F380-F393–F393. doi:10.1152/ajprenal.00557.2019
Maren, T. H., Fine, A., Swenson, E. R., and Rothman, D. (1992). Renal acid-base physiology in marine teleost, the long-horned sculpin (Myoxocephalus octodecimspinosus). Am. J. Physiology-Renal Physiology 263 (1), F49–F55. doi:10.1152/ajprenal.1992.263.1.F49
Maxime, V., Pennec, J. P., and Peyraud, C. (1991). Effects of direct transfer from freshwater to seawater on respiratory and circulatory variables and acid-base status in rainbow trout. J. Comp. Physiology B 161 (6), 557–568. doi:10.1007/BF00260745
Maxime, V., Peyraud-Waitzenergger, M., Claireaux, G., and Peyraud, C. (1990). Effects of rapid transfer from sea water to fresh water on respiratory variables, blood acid-base status and O2 affinity of haemoglobin in Atlantic salmon (Salmo salar L). J. Comp. Physiology B 160 (1), 31–39. doi:10.1007/BF00258760
McArley, T. J., Sandblom, E., and Herbert, N. A. (2021). Fish and hyperoxia—from cardiorespiratory and biochemical adjustments to aquaculture and ecophysiology implications. Fish Fish. 22 (2), 324–355. doi:10.1111/faf.12522
McDonald, D. G., Walker, R. L., Wilkes, P. R. H., and Wood, C. M. (1982). H+ excretion in the marine teleost Parophrys vetulus. J. Exp. Biol. 98 (1), 403–414. doi:10.1242/jeb.98.1.403
McDonald, D. G., and Wood, C. M. (1981). Branchial and renal acid and ion fluxes in the rainbow trout, Salmo gairdneri, at low environmental pH. J. Exp. Biol. 93 (1), 101–118. doi:10.1242/jeb.93.1.101
Miles, H. M. (1971). Renal function in migrating adult coho salmon. Comp. Biochem. Physiology Part A Physiology 38 (4), 787–826. doi:10.1016/0300-9629(71)90220-9
Minarova, H., Palikova, M., Kopp, R., Maly, O., Mares, J., Mikulikova, I., et al. (2023). Nephrocalcinosis in farmed salmonids: Diagnostic challenges associated with low performance and sporadic mortality. Front. Veterinary Sci. 10, 1121296. doi:10.3389/fvets.2023.1121296
Mommsen, T. P., and Hochachka, P. W. (1988). The purine nucleotide cycle as two temporally separated metabolic units: A study on trout muscle. Metabolism 37 (6), 552–556. doi:10.1016/0026-0495(88)90170-9
Mota, V. C., Nilsen, T. O., Gerwins, J., Gallo, M., Kolarevic, J., Krasnov, A., et al. (2020). Molecular and physiological responses to long-term carbon dioxide exposure in Atlantic salmon (Salmo salar). Aquaculture 519, 734715. doi:10.1016/j.aquaculture.2019.734715
Mota, V. C., Nilsen, T. O., Gerwins, J., Gallo, M., Ytteborg, E., Baeverfjord, G., et al. (2019). The effects of carbon dioxide on growth performance, welfare, and health of Atlantic salmon post-smolt (Salmo salar) in recirculating aquaculture systems. Aquaculture 498, 578–586. doi:10.1016/j.aquaculture.2018.08.075
Mullens, W., Damman, K., Testani, J. M., Martens, P., Mueller, C., Lassus, J., et al. (2020). Evaluation of kidney function throughout the heart failure trajectory–a position statement from the Heart Failure Association of the European Society of Cardiology. Eur. J. heart Fail. 22 (4), 584–603. doi:10.1002/ejhf.1697
Nakada, T., Hoshijima, K., Esaki, M., Nagayoshi, S., Kawakami, K., and Hirose, S. (2007a). Localization of ammonia transporter Rhcg1 in mitochondrion-rich cells of yolk sac, gill, and kidney of zebrafish and its ionic strength-dependent expression. Am. J. Physiology-Regulatory, Integr. Comp. Physiology 293 (4), R1743–R1753. doi:10.1152/ajpregu.00248.2007
Nakada, T., Westhoff, C. M., Kato, A., and Hirose, S. (2007b). Ammonia secretion from fish gill depends on a set of Rh glycoproteins. FASEB J. 21 (4), 1067–1074. doi:10.1096/fj.06-6834com
Nawata, C. M., Hirose, S., Nakada, T., Wood, C. M., and Kato, A. (2010). Rh glycoprotein expression is modulated in pufferfish (Takifugu rubripes) during high environmental ammonia exposure. J. Exp. Biol. 213 (18), 3150–3160. doi:10.1242/jeb.044719
Nawata, C. M., Hung, C. C., Tsui, T. K., Wilson, J. M., Wright, P. A., and Wood, C. M. (2007). Ammonia excretion in rainbow trout (Oncorhynchus mykiss): Evidence for Rh glycoprotein and H+-ATPase involvement. Physiol. Genomics 31 (3), 463–474. doi:10.1152/physiolgenomics.00061.2007
Nelson, C., Dichiera, A. M., Jung, E. H., and Brauner, C. J. (2023). An atlas of plasma-accessible carbonic anhydrase availability in the model teleost, the rainbow trout. J. Comp. Physiology B 193, 293–305. doi:10.1007/s00360-023-01484-7
Nylund, A., Plarre, H., Hodneland, K., Devold, M., Aspehaug, V., Aarseth, M., et al. (2003). Haemorrhagic smolt syndrome (HSS) in Norway: Pathology and associated virus-like particles. Dis. aquatic Org. 54 (1), 15–27. doi:10.3354/dao054015
Olson, K. R., and Hoagland, T. M. (2008). Effects of freshwater and saltwater adaptation and dietary salt on fluid compartments, blood pressure, and venous capacitance in trout. Am. J. Physiology-Regulatory, Integr. Comp. Physiology 294 (3), R1061–R1067. doi:10.1152/ajpregu.00698.2007
Olszynski, M., Prywer, J., and Torzewska, A. (2015). Effect of size and shape of nanosilver particles on struvite and carbonate apatite precipitation. Cryst. Growth & Des. 15 (7), 3307–3320. doi:10.1021/acs.cgd.5b00425
Pelis, R. M., and Renfro, J. L. (2004). Role of tubular secretion and carbonic anhydrase in vertebrate renal sulfate excretion. Am. J. Physiology-Regulatory, Integr. Comp. Physiology 287 (3), R491–R501. doi:10.1152/ajpregu.00084.2004
Perry, S. F., Braun, M. H., Genz, J., Vulesevic, B., Taylor, J., Grosell, M., et al. (2010). Acid–base regulation in the plainfin midshipman (Porichthys notatus): An aglomerular marine teleost. J. Comp. Physiology B 180, 1213–1225. doi:10.1007/s00360-010-0492-8
Perry, S. F., and Fryer, J. N. (1997). Proton pumps in the fish gill and kidney. Fish Physiology Biochem. 17, 363–369. doi:10.1023/A:1007746217349
Perry, S. F., Furimsky, M., Bayaa, M., Georgalis, T., Shahsavarani, A., Nickerson, J. G., et al. (2003a). Integrated responses of Na+/HCO3− cotransporters and V-type H+-ATPases in the fish gill and kidney during respiratory acidosis. Biochimica Biophysica Acta (BBA)-Biomembranes 1618 (2), 175–184. doi:10.1016/j.bbamem.2003.09.015
Perry, S. F., and Gilmour, K. M. (2006). Acid–base balance and CO2 excretion in fish: Unanswered questions and emerging models. Respir. physiology Neurobiol. 154 (1-2), 199–215. doi:10.1016/j.resp.2006.04.010
Perry, S. F., Malone, S., and Ewing, D. (1987). Hypercapnic acidosis in the rainbow trout (Salmo gairdneri). II. Renal ionic fluxes. Can. J. zoology 65 (4), 896–902. doi:10.1139/z87-143
Perry, S. F., Shahsavarani, A., Georgalis, T., Bayaa, M., Furimsky, M., and Thomas, S. L. Y. (2003b). Channels, pumps, and exchangers in the gill and kidney of freshwater fishes: Their role in ionic and acid-base regulation. J. Exp. Zoology Part A Comp. Exp. Biol. 300 (1), 53–62. doi:10.1002/jez.a.10309
Petochi, T., Di Marco, P., Priori, A., Finoia, M. G., Mercatali, I., and Marino, G. (2011). Coping strategy and stress response of European sea bass Dicentrarchus labrax to acute and chronic environmental hypercapnia under hyperoxic conditions. Aquaculture 315 (3-4), 312–320. doi:10.1016/j.aquaculture.2011.02.028
Philip, A. J. P., Fjelldal, P. G., Remø, S. C., Selvam, C., Hamre, K., Espe, M., et al. (2022). Dietary electrolyte balance of Atlantic salmon (Salmo salar) freshwater feeds: Impact on osmoregulation, mineral metabolism and performance in seawater. Aquaculture 546, 737305. doi:10.1016/j.aquaculture.2021.737305
Potter, S. S. (2018). Single-cell RNA sequencing for the study of development, physiology and disease. Nat. Rev. Nephrol. 14 (8), 479–492. doi:10.1038/s41581-018-0021-7
Pyle, G. G., Kamunde, C. N., McDonald, D. G., and Wood, C. M. (2003). Dietary sodium inhibits aqueous copper uptake in rainbow trout (Oncorhynchus mykiss). J. Exp. Biol. 206 (3), 609–618. doi:10.1242/jeb.00114
Randall, D. J., and Wright, P. A. (1989). The interaction between carbon dioxide and ammonia excretion and water pH in fish. Can. J. Zoology 67 (12), 2936–2942. doi:10.1139/z89-416
Said, S., and Hernandez, G. T. (2014). The link between chronic kidney disease and cardiovascular disease. J. Nephropathol. 3 (3), 99–104. doi:10.12860/jnp.2014.19
Shaw, H. M., Saunders, R. L., Hall, H. C., and Henderson, E. B. (2011). Effect of dietary sodium chloride on growth of atlantic salmon (Salmo salar). J. Fish. Res. Board Can. 32 (10), 1813–1819. doi:10.1139/f75-215
Skov, P. V. (2019). “CO2 in aquaculture,” in Fish physiology (Academic Press), 37, 287–321. doi:10.1016/bs.fp.2019.07.004
Smart, G. R., Knox, D., Harrison, J. G., Ralph, J. A., Richard, R. H., and Cowey, C. B. (1979). Nephrocalcinosis in rainbow trout Salmo gairdneri Richardson; the effect of exposure to elevated CO2 concentrations. J. Fish Dis. 2 (4), 279–289. doi:10.1111/j.1365-2761.1979.tb00170.x
Sommerset, I., Walde, C. S., Bang Jensen, B., Wiik-Nielsen, J., Bornø, G., Oliveira, V. H. S., et al. (2022). Norwegian fish health report 2021. Oslo, Norway: Norwegian Veterinary.
Stewart, B. J., and Clatworthy, M. R. (2020). Applying single-cell technologies to clinical pathology: Progress in nephropathology. J. Pathol. 250, 693–704. doi:10.1002/path.5417
Sugiura, S. H., and Ferraris, R. P. (2004). Contributions of different NaPi cotransporter isoforms to dietary regulation of P transport in the pyloric caeca and intestine of rainbow trout. J. Exp. Biol. 207 (12), 2055–2064. doi:10.1242/jeb.00971
Sugiura, S. H., McDaniel, N. K., and Ferraris, R. P. (2003). In vivo fractional Pi absorption and NaPi-II mRNA expression in rainbow trout are upregulated by dietary P restriction. Am. J. Physiology-Regulatory, Integr. Comp. Physiology 285 (4), R770–R781. doi:10.1152/ajpregu.00127.2003
Sundell, E., Morgenroth, D., Brijs, J., Ekström, A., Gräns, A., and Sandblom, E. (2018). Seawater acclimation affects cardiac output and adrenergic control of blood pressure in rainbow trout (Oncorhynchus mykiss)—Implications for salinity variations now and in the future. Conserv. Physiol. 6 (1), coy061. doi:10.1093/conphys/coy061
Takvam, M., Denker, E., Gharbi, N., Kryvi, H., and Nilsen, T. O. (2021b). Sulfate homeostasis in Atlantic salmon is associated with differential regulation of salmonid-specific paralogs in gill and kidney. Physiol. Rep. 9, e15059. doi:10.14814/phy2.15059
Takvam, M., Wood, C. M., Kryvi, H., and Nilsen, T. O. (2021a). Ion transporters and osmoregulation in the kidney of teleost fishes as a function of salinity. Front. Physiology 12, 664588. doi:10.3389/fphys.2021.664588
Thinh, P. V., Thanh Huong, D. T., Gam, L. T. H., Damsgaard, C., Phuong, N. T., Bayley, M., et al. (2019). Renal acid excretion contributes to acid–base regulation during hypercapnia in air-exposed swamp eel (Monopterus albus). J. Exp. Biol. 222 (9), jeb198259. doi:10.1242/jeb.198259
Tresguerres, M., Clifford, A. M., Harter, T. S., Roa, J. N., Thies, A. B., Yee, D. P., et al. (2020). Evolutionary links between intra-and extracellular acid–base regulation in fish and other aquatic animals. J. Exp. Zoology Part A Ecol. Integr. Physiology 333 (6), 449–465. doi:10.1002/jez.2367
Verri, T., and Werner, A. (2019). Type II Na+-phosphate cotransporters and phosphate balance in teleost fish. Pflügers Archiv-European J. Physiology 471, 193–212. doi:10.1007/s00424-018-2239-4
Vielma, J., and Lall, S. P. (1998). Control of phosphorus homeostasis of Atlantic salmon (Salmo salar) in fresh water. Fish Physiology Biochem. 19, 83–93. doi:10.1023/A:1007757321695
Walton, M. J., and Cowey, C. B. (1977). Aspects of ammoniogenesis in rainbow trout, Salmo gairdneri. Comp. Biochem. Physiology Part B Comp. Biochem. 57 (2), 143–149. doi:10.1016/0305-0491(77)90164-X
Walton, M. J., and Cowey, C. B. (1982). Aspects of intermediary metabolism in salmonid fish. Comp. Biochem. Physiology Part B Comp. Biochem. 73 (1), 59–79. doi:10.1016/0305-0491(82)90201-2
Watanabe, T., and Takei, Y. (2012). Vigorous SO42–influx via the gills is balanced by enhanced SO42–excretion by the kidney in eels after seawater adaptation. J. Exp. Biol. 215 (10), 1775–1781. doi:10.1242/jeb.063511
Weiner, I. D., and Verlander, J. W. (2017). Ammonia transporters and their role in acid-base balance. Physiol. Rev. 97 (2), 465–494. doi:10.1152/physrev.00011.2016
Weiner, I. D., and Verlander, J. W. (2011). Role of NH3 and NH4+ transporters in renal acid-base transport. Am. J. Physiology-Renal Physiology 300 (1), F11–F23. doi:10.1152/ajprenal.00554.2010
Wheatly, M. G., Hōbe, H., and Wood, C. M. (1984). The mechanisms of acid-base and ionoregulation in the freshwater rainbow trout during environmental hyperoxia and subsequent normoxia. II. The role of the kidney. Respir. Physiol. 55 (2), 155–173. doi:10.1016/0034-5687(84)90020-3
Wilkie, M. P., and Wood, C. M. (1996). The adaptations of fish to extremely alkaline environments. Comp. Biochem. Physiology Part B Biochem. Mol. Biol. 113 (4), 665–673. doi:10.1016/0305-0491(95)02092-6
Wood, C. M. (1991a). Acid-base and ion balance, metabolism, and their interactions, after exhaustive exercise in fish. J. Exp. Biol. 160 (1), 285–308. doi:10.1242/jeb.160.1.285
Wood, C. M. (1988). Acid-base and ionic exchanges at gills and kidney after exhaustive exercise in the rainbow trout. J. Exp. Biol. 136 (1), 461–481. doi:10.1242/jeb.136.1.461
Wood, C. M. (1991b). Branchial ion and acid-base transfer in freshwater teleost fish: Environmental hyperoxia as a probe. Physiol. Zool. 64 (1), 68–102. doi:10.1086/physzool.64.1.30158514
Wood, C. M., Bucking, C., and Grosell, M. (2010). Acid–base responses to feeding and intestinal Cl–uptake in freshwater-and seawater-acclimated killifish, Fundulus heteroclitus, an agastric euryhaline teleost. J. Exp. Biol. 213 (15), 2681–2692. doi:10.1242/jeb.039164
Wood, C. M. (1995). “Excretion,” in Physiological ecology of the pacific salmon. Editors C. Groot, L. Margolis, and W. C. Clarke (Vancouver: Government of Canada Special Publications Branch. UBC Press), 381–438.
Wood, C. M., Hopkins, T. E., Hogstrand, C., and Walsh, P. J. (1995). Pulsatile urea excretion in the ureagenic toadfish Opsanus beta: An analysis of rates and routes. J. Exp. Biol. 198 (8), 1729–1741. doi:10.1242/jeb.198.8.1729
Wood, C. M. (2001). Influence of feeding, exercise, and temperature on nitrogen metabolism and excretion. Fish. Physiol. 20, 201–238. doi:10.1016/S1546-5098(01)20007-7
Wood, C. M., and Jackson, E. B. (1980). Blood acid-base regulation during environmental hyperoxia in the rainbow trout (Salmo gairdneri). Respir. Physiol. 42 (3), 351–372. doi:10.1016/0034-5687(80)90125-5
Wood, C. M., Kajimura, M., Mommsen, T. P., and Walsh, P. J. (2005). Alkaline tide and nitrogen conservation after feeding in an elasmobranch (Squalus acanthias). J. Exp. Biol. 208 (14), 2693–2705. doi:10.1242/jeb.01678
Wood, C. M., Milligan, C. L., and Walsh, P. J. (1999). Renal responses of trout to chronic respiratory and metabolic acidoses and metabolic alkalosis. Am. J. Physiology-Regulatory, Integr. Comp. Physiology 277 (2), R482–R492. doi:10.1152/ajpregu.1999.277.2.R482
Wood, C. M., and Nawata, C. M. (2011). A nose-to-nose comparison of the physiological and molecular responses of rainbow trout to high environmental ammonia in seawater versus freshwater. J. Exp. Biol. 214 (21), 3557–3569. doi:10.1242/jeb.057802
Wood, C. M., and Patrick, M. L. (1994). “Methods for assessing kidney and urinary bladder function in fish,” in Biochemistry and molecular biology of fishes. Editors P. W. Hochachka, and T. P. Mommsen (New York: Elsevier), 3, 127–143.
Wood, C. M., Pelster, B., Braz-Mota, S., and Val, A. L. (2020). Gills versus kidney for ionoregulation in the obligate air-breathing Arapaima gigas, a fish with a kidney in its air-breathing organ. J. Exp. Biol. 223 (20), jeb232694. doi:10.1242/jeb.232694
Wood, C. M. (1989). “The physiological problems of fish in acid waters,” in Acid toxicity and aquatic animals, society for experimental biology seminar series. Editors R. Morris, D. J. A. Brown1`, E. W. Taylor, and J. A. Brown Cambridge University Press, 125–148.
Worcester, E. M., Parks, J. H., Evan, A. P., and Coe, F. L. (2006). Renal function in patients with nephrolithiasis. J. urology 176 (2), 600–603. doi:10.1016/j.juro.2006.03.095
Wright, P. A., and Wood, C. M. (2009). A new paradigm for ammonia excretion in aquatic animals: Role of rhesus (Rh) glycoproteins. J. Exp. Biol. 212 (15), 2303–2312. doi:10.1242/jeb.023085
Wright, P. A., Wood, C. M., and Wilson, J. M. (2014). Rh versus pH: The role of rhesus glycoproteins in renal ammonia excretion during metabolic acidosis in a freshwater teleost fish. J. Exp. Biol. 217 (16), 2855–2865. doi:10.1242/jeb.098640
Ytrestøyl, T., Hjelle, E., Kolarevic, J., Takle, H., Rebl, A., Afanasyev, S., et al. (2023). Photoperiod in recirculation aquaculture systems and timing of seawater transfer affect seawater growth performance of Atlantic salmon (Salmo salar). J. World Aquac. Soc. 54 (1), 73–95. doi:10.1111/jwas.12880
Zimmer, A. M., Nawata, C. M., and Wood, C. M. (2010). Physiological and molecular analysis of the interactive effects of feeding and high environmental ammonia on branchial ammonia excretion and Na+ uptake in freshwater rainbow trout. J. Comp. Physiology B 180 (8), 1191–1204. doi:10.1007/s00360-010-0488-4
Keywords: acid-base regulation, kidney stones, patophysiology, transporters and channels, nephron tubule, aquaculture, water quality (WQ), fish
Citation: Takvam M, Wood CM, Kryvi H and Nilsen TO (2023) Role of the kidneys in acid-base regulation and ammonia excretion in freshwater and seawater fish: implications for nephrocalcinosis. Front. Physiol. 14:1226068. doi: 10.3389/fphys.2023.1226068
Received: 20 May 2023; Accepted: 12 June 2023;
Published: 29 June 2023.
Edited by:
Carlo C. Lazado, Fisheries and Aquaculture Research (Nofima), NorwayReviewed by:
Dennis Kolosov, California State University San Marcos, United StatesCopyright © 2023 Takvam, Wood, Kryvi and Nilsen. This is an open-access article distributed under the terms of the Creative Commons Attribution License (CC BY). The use, distribution or reproduction in other forums is permitted, provided the original author(s) and the copyright owner(s) are credited and that the original publication in this journal is cited, in accordance with accepted academic practice. No use, distribution or reproduction is permitted which does not comply with these terms.
*Correspondence: Marius Takvam, bS50YWt2YW1AdWliLm5v; Tom O. Nilsen, VG9tLm5pbHNlbkB1aWIubm8=
Disclaimer: All claims expressed in this article are solely those of the authors and do not necessarily represent those of their affiliated organizations, or those of the publisher, the editors and the reviewers. Any product that may be evaluated in this article or claim that may be made by its manufacturer is not guaranteed or endorsed by the publisher.
Research integrity at Frontiers
Learn more about the work of our research integrity team to safeguard the quality of each article we publish.