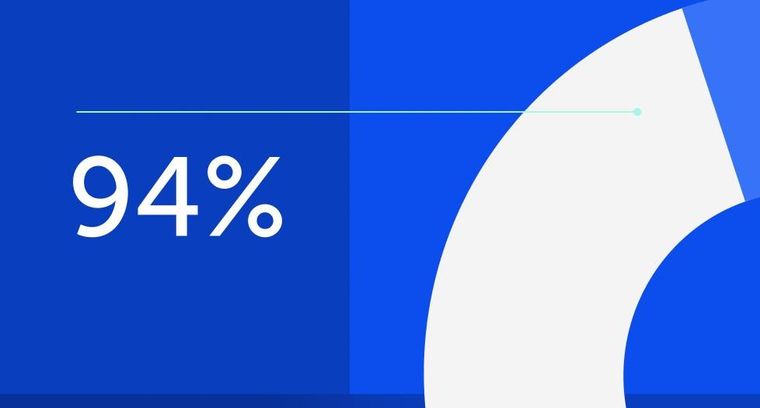
94% of researchers rate our articles as excellent or good
Learn more about the work of our research integrity team to safeguard the quality of each article we publish.
Find out more
REVIEW article
Front. Physiol., 27 July 2023
Sec. Integrative Physiology
Volume 14 - 2023 | https://doi.org/10.3389/fphys.2023.1210085
This article is part of the Research Topic72nd Annual Meeting of the Italian Society of Physiology: New Perspectives in Physiological ResearchView all 13 articles
Cytosolic Ca2+ signals are organized in complex spatial and temporal patterns that underlie their unique ability to regulate multiple cellular functions. Changes in intracellular Ca2+ concentration ([Ca2+]i) are finely tuned by the concerted interaction of membrane receptors and ion channels that introduce Ca2+ into the cytosol, Ca2+-dependent sensors and effectors that translate the elevation in [Ca2+]i into a biological output, and Ca2+-clearing mechanisms that return the [Ca2+]i to pre-stimulation levels and prevent cytotoxic Ca2+ overload. The assortment of the Ca2+ handling machinery varies among different cell types to generate intracellular Ca2+ signals that are selectively tailored to subserve specific functions. The advent of novel high-speed, 2D and 3D time-lapse imaging techniques, single-wavelength and genetic Ca2+ indicators, as well as the development of novel genetic engineering tools to manipulate single cells and whole animals, has shed novel light on the regulation of cellular activity by the Ca2+ handling machinery. A symposium organized within the framework of the 72nd Annual Meeting of the Italian Society of Physiology, held in Bari on 14–16th September 2022, has recently addressed many of the unexpected mechanisms whereby intracellular Ca2+ signalling regulates cellular fate in healthy and disease states. Herein, we present a report of this symposium, in which the following emerging topics were discussed: 1) Regulation of water reabsorption in the kidney by lysosomal Ca2+ release through Transient Receptor Potential Mucolipin 1 (TRPML1); 2) Endoplasmic reticulum-to-mitochondria Ca2+ transfer in Alzheimer’s disease-related astroglial dysfunction; 3) The non-canonical role of TRP Melastatin 8 (TRPM8) as a Rap1A inhibitor in the definition of some cancer hallmarks; and 4) Non-genetic optical stimulation of Ca2+ signals in the cardiovascular system.
An increase in intracellular Ca2+ concentration ([Ca2+]i) can operate over a very wide dynamic range to specifically regulate a multitude of cellular functions (Berridge et al., 2003). Neurotransmitter release from presynaptic terminals, as well as insulin exocytosis from pancreatic β-cells, occur within microseconds on the elevation in [Ca2+]i, while the intracellular Ca2+ oscillations that drive gene expression may last for a few hours (Berridge et al., 2003; Clapham, 2007). An additional mechanism that enriches the versatility of intracellular Ca2+ signalling is represented by the spatial location of the Ca2+ sources, which can be physically coupled to different Ca2+-dependent decoders (Berridge et al., 2003; Bagur and Hajnoczky, 2017; Ong et al., 2019; Barak and Parekh, 2020). Environmental cues generate a complex choreography of intracellular Ca2+ signals (Berridge et al., 2003; Clapham, 2007), whose spatio-temporal malleability enables one single ion messenger to control as many different functions as fertilization (Moccia et al., 2006), cell cycle (Lim et al., 2003) and proliferation (Faris et al., 2019; Faris et al., 2022), migration (Fiorio Pla et al., 2012; Zuccolo et al., 2018b), differentiation (Maione et al., 2022), contraction (Bers, 2008; Landstrom et al., 2017), metabolism (Patella et al., 2015), angiogenesis (Bernardini et al., 2019; Moccia et al., 2019b; Scarpellino et al., 2020), vasculogenesis (Moccia et al., 2012; Moccia et al., 2013; Zuccolo et al., 2018a), and, more recently, neurovascular coupling (Negri et al., 2021c; Soda et al., 2023). The multifaceted nature of intracellular Ca2+ signalling can be further appreciated by recalling that, depending on the Ca2+ source and on the Ca2+-dependent target, an increase in [Ca2+]i may induce opposing cellular responses, e.g., proliferation (Faris et al., 2022) and apoptosis (Astesana et al., 2021; Faris et al., 2023), vascular smooth muscle cell contraction (Knot and Nelson, 1998) and relaxation (Nelson et al., 1995), neuronal depolarization (Menigoz et al., 2016) and hyperpolarization (Tiwari et al., 2018), long-term potentiation (Ezra-Nevo et al., 2018; Soda et al., 2019; Locatelli et al., 2021) and long-term depression (Hirano, 2013). Dysregulation of the sophisticated machinery that orchestrates the Ca2+ response to physiological signals can, therefore, trigger or exacerbate a growing list of life-threatening disorders, such as neurodegenerative (Lim et al., 2014; Lim et al., 2021a) and cardiovascular (Venetucci et al., 2012; Moccia et al., 2019a) disorders, severe combined immunodeficiency (SCID) (Vaeth et al., 2020), and cancer (Moccia, 2018; Prevarskaya et al., 2018).
The Ca2+ response to environmental cues in non-excitable cells is usually triggered by the phospholipase C-dependent production of inositol-1,4-5-trisphosphate (InsP3), which mobilizes Ca2+ from what is regarded the most abundant intracellular Ca2+ reservoir, namely, the endoplasmic reticulum (ER) (Berridge et al., 2003; Clapham, 2007). InsP3 gates the ionotropic InsP3 receptors (InsP3Rs), which are non-selective cation channel located on ER cisternae, in the presence of a permissive concentration of ambient Ca2+ (Prole and Taylor, 2019). Repetitive events of InsP3-evoked Ca2+ release may be spatially confined to peripheral InsP3Rs, which are located in close proximity to plasmalemmal Gq-Protein Coupled Receptors (GqPCRs) (Keebler and Taylor, 2017; Thillaiappan et al., 2017), or can propagate as regenerative Ca2+ waves through the mechanism of Ca2+-induced Ca2+ release (CICR) (Bootman et al., 1997). Ryanodine receptors (RyRs), which represent the main Ca2+-releasing channel in the sarcoplasmic reticulum (SR) and may also be present in the ER, support InsP3-evoked regenerative Ca2+ waves in some, but not all (Moccia et al., 2019b), cell types (Santulli et al., 2018). Depletion of the ER/SR Ca2+ content due to cyclic Ca2+ extrusion in the extracellular milieu by plasma membrane Ca2+-ATPase or Na+/Ca2+ exchanger (NCX) (Moccia et al., 2002; Berra-Romani et al., 2023) is prevented by the activation of store-operated Ca2+ entry (SOCE) (Lewis, 2020; Moccia et al., 2023). SOCE requires the dynamic interplay between Stromal Interaction Molecules 1 and 2 (STIM1 and STIM2, respectively), which serve as sensor of ER Ca2+ concentration ([Ca2+]ER), and the Ca2+-selective channels, Orai1-3, on the plasma membrane (Lewis, 2020; Moccia et al., 2023). In excitable cells, membrane depolarization evoked by excitatory synaptic transmission (Locatelli et al., 2021) or spontaneous diastolic depolarization (Eisner et al., 2017) can lead to extracellular Ca2+ entry through multiple types of voltage-operated Ca2+ channels (VOCCs), which can be followed by CICR through RyRs and/or InsP3Rs (Bading, 2013; Eisner et al., 2017). In both excitable and non-excitable cells, extracellular Ca2+ entry is further mediated by the Transient Receptor Potential (TRP) family of non-selective cation channels, most of which are polymodal Ca2+-permeable channels able to sense chemical, thermal and mechanical signals and thereby execute the most appropriate cellular response (Curcic et al., 2019; Vangeel and Voets, 2019; Diver et al., 2022). The advent of novel high-speed, 2D and 3D time-lapse imaging techniques, single-wavelength and genetic Ca2+ indicators, as well as the development of novel genetic engineering tools to manipulate single cells and whole animals, has shed novel light on the regulation of cellular activity by the Ca2+ handling machinery (Lim et al., 2016a; Bagur and Hajnoczky, 2017; Tapella et al., 2020; Berra-Romani et al., 2021; Leoni et al., 2021; Longden et al., 2021; Marta et al., 2022). For instance, it has been recognized that ER cisternae may establish dynamic contacts with other intracellular organelles, such as mitochondria (Csordas et al., 2010; Csordas et al., 2018; Bartok et al., 2019; Lim et al., 2021a; Sanchez-Vazquez et al., 2023) and lysosomes (Kilpatrick et al., 2013; Atakpa et al., 2018; Faris et al., 2022), to shape intracellular Ca2+ signals. The Ca2+-dependent inter-organellar communication between ER and mitochondria has long been known to dictate cellular fate (Loncke et al., 2021; Bonora et al., 2022). We now know that, although both InsP3Rs in ER cisternae and mitochondria in the cytosol are quite motile, they can establish temporary interactions at mitochondria-associated ER membranes (MAMs) to increase mitochondrial Ca2+ in an InsP3-dependent manner and stimulate cellular bioenergetics (Gherardi et al., 2020; Katona et al., 2022). However, stress conditions, such as those that can lead to neurodegenerative disorders, can alter the distance between the ER and mitochondria and, thereby, impair mitochondrial Ca2+ uptake and cellular bioenergetics that contributes to cell dysfunction (Lim et al., 2021a; Lim et al., 2023). An unexpected mode of Ca2+-dependent inter-organellar communication has also been described at the membrane contact sites between ER and lysosomes (Kilpatrick et al., 2013; Ronco et al., 2015). Herein, the second messenger nicotinic acid adenine dinucleotide phosphate (NAADP), which can also be synthesized upon GqPCR or tyrosine kinase receptor (TKR) activation, gates two pore channels (TPCs) to mediate lysosomal Ca2+ release and prime ER-embedded InsP3Rs for InsP3-dependent activation (Patel, 2015; Galione et al., 2023). Lysosomal Ca2+ can also be mobilized by TRP Mucolipin 1 (TRPML1), which plays a crucial role in autophagic progression (Medina et al., 2015; Di Paola et al., 2018). TRPML1-mediated Ca2+ signals were thought to be confined to the perilysosomal Ca2+ space (Medina et al., 2015), but recent studies unexpectedly reported TRPML1-induced global Ca2+ signals via the Ca2+-dependent recruitment of RyRs and InsP3Rs (Kilpatrick et al., 2016; Thakore et al., 2020). An additional dogma that has recently turn into a signalling revolution regards the same operation mode of ion channels. Channel proteins do more than simply conducting biologically relevant ions (Montes de Oca Balderas, 2022). Indeed, emerging evidence indicates that ion channels can signal in a flux-independent mode, thereby widening their potential impact on cell physiology (Borowiec et al., 2014; Vrenken et al., 2016; Chinigo et al., 2020; Pressey and Woodin, 2021; Arcangeli et al., 2023). For instance, the intracellular domains of some VOCCs, i.e., CaV1.2 (Gomez-Ospina et al., 2006) and CaV2.1 (Kordasiewicz et al., 2006), as well as some isoforms of the accessory CaVβ subunit (Hibino et al., 2003), can translocate into the nucleus and induce Ca2+-independent gene expression. Furthermore, some ionotropic receptors, such as N-methyl-D-aspartate (NMDA) receptors (Montes de Oca Balderas and Aguilera, 2015; Negri et al., 2021a) and type A γ-aminobutyric acid (GABA) receptors (Negri et al., 2022b), can signal an increase in [Ca2+]i in a flux-independent manner due to their ability to interact with their corresponding metabotropic receptors. Several members of the TRP superfamily can also function in a non-canonical mode. For instance, TRP Melastatin type 7 (TRPM7) channel promotes most of its effect thought the intrinsic kinase activity that is located within its COOH-terminus (Desai et al., 2012; Faouzi et al., 2017; Cai et al., 2018), whereas TRP Canonical type 1 (TRPC1) does not need to mediate Ca2+ to induce proliferation in human umbilical cord vein endothelial cells (Abdullaev et al., 2008). Finally, the versatility of the Ca2+ handling machinery has been exploited to design alternative therapeutic avenues for many diseases that are still waiting for an effective treatment. For instance, a light-operated Ca2+ permeable channel (LOC) has been generated by introducing plant-derived photosensory domain into a cytoplasmic loop of the Orai1 channel (He et al., 2021). Optogenetic intervention by this novel LOC proved effective to suppress excessive hematopoietic stem cell self-renewal and to alleviate neurodegeneration in a model of amyloidosis (He et al., 2021).
A symposium organized within the framework of the 72nd Annual Meeting of the Italian Society of Physiology, held in Bari on 14–16th September 2022, has recently addressed many of the unexpected mechanisms whereby intracellular Ca2+ signalling regulates cellular fate in healthy and disease. The symposium, named “Ca2+ signalling: unexpected new roles for the usual suspect”, gathered together four renowned Italian physiologists, who informed a numerous and very interested audience about their novel findings regarding the following topics: 1) the role of TRPML1 in Ca2+-mediated water reabsorption in the kidney (Prof. Andrea Gerbino, University of Bari Aldo Moro); 2) the modulation of the ER-mitochondria distance to fuel cellular metabolism in astrocytes and prevent neurodegeneration in Alzheimer’s disease (Prof. Dmitry Lim, University of Piemonte Orientale, Novara); 3) the non-canonical role of TRP Melastatin 8 (TRPM8) in the definition of some cancer hallmarks (Prof. Alessandra Fiorio Pla, University of Turin); and 4) the use of novel light-sensitive organic actuators to stimulate angiogenesis and control cardiac cells pacing (Prof. Francesco Lodola, University of Milan-Bicocca). Herein, we present a full report of the symposium and discuss the implications for the Ca2+ signalling field of the novel findings that were presented during each lecture.
Lysosomes are multifunctional organelles: apart from well-defined digestive tasks (Xu and Ren, 2015), lysosomes act as a regulatory hub integrating multiple cues to modulate a wide spectrum of intracellular signaling pathways (Ballabio, 2016). Lysosomal vesicles are emerging as a novel Ca2+ reservoir that can finely modulate cellular fate through local or global Ca2+ signals (Patel and Cai, 2015; Galione, 2019; Galione et al., 2023). Throughout the whole process, lysosomes can freely diffuse and deliver/reuptake Ca2+ in the close proximity of target organelles such as ER, mitochondria and secretory vesicles. Understanding how lysosomes establish the Ca2+-dependent cross-talk with surrounding organelles that orchestrate the Ca2+ response to physiological cues is crucial to appreciate how defective lysosomal Ca2+ signalling underpins life-threatening diseases, such as cancer (Faris et al., 2018), viral infections (Moccia et al., 2021a), hypertension (Negri et al., 2021b) and arrhythmias (Negri et al., 2021b), and lysosomal storage disorders (Kiselyov et al., 2010; Lloyd-Evans et al., 2010; Morgan et al., 2011).
The lysosomal matrix is strongly acidic with a pH of around 4.6 originated by the continuous activity of a vesicular H+-proton pump ATPase (V-ATPase) (Xu and Ren, 2015). Lysosomes can actively accumulate large amount of free Ca2+ (0.5 mM) through a mechanism that is still highly debated (Yang et al., 2019). Refilling with the Ca2+ of the lysosomal matrix could be driven either by a putative H+/Ca2+ exchanger in a pH-dependent manner (Christensen et al., 2002; Ronco et al., 2015; Melchionda et al., 2016) or by extracellular Ca2+ entry through endocytosis or SOCE (Gerasimenko et al., 1998; Sbano et al., 2017). Lysosomal Ca2+ can be released into the cytosol through TPCs (Patel, 2015), of which two isoforms exist in mammals (i.e., TPC1 and TPC2), and TRPML1 (Faris et al., 2018). TPCs are gated by NAADP, which can be produced upon GqPCR or TKR activation on the plasma membrane, and phosphatidylinositol-3, 5-bisphosphate (PIP2) (Patel, 2015; Galione et al., 2023). Intriguingly, planar lysosomal patch-clamp recording showed that NAADP evoked TPC2-mediated currents that were equally mediated by Na+ and Ca2+, while those gated by PIP2 were relatively Na+-selective (Gerndt et al., 2020). TPCs can be located at membrane contact sites (MCSs) between lysosomes and ER (Kilpatrick et al., 2017; Faris et al., 2022), where they are physiologically activated by NAADP to release lysosomal Ca2+ and evoke global Ca2+ signals via Ca2+-induced Ca2+ release through InsP3Rs and/or ryanodine receptors (Patel, 2015; Galione et al., 2023). According to the “trigger-hypothesis” (Galione, 2019; Galione et al., 2023), the InsP3-induced Ca2+ response to a plethora of extracellular stimuli, including glutamate (Foster et al., 2018; Zuccolo et al., 2019), acetylcholine (Aley et al., 2013), foetal bovine serum (Faris et al., 2019), and vascular endothelial growth factor (VEGF) (Moccia et al., 2021b), is initiated by the NAADP-sensitive lysosomal TPCs. TRPML1 is a non-selective cation permeable channel that mediates lysosomal Ca2+, Fe2+, and Zn2+ release into the cytosol in response by either endogenous agonists, such as phosphatidylinositol 3,5-bisphosphate [PI(3,5)P2] (Gan et al., 2022) and reactive oxygen species (ROS) (Zhang et al., 2016) or synthetic ligands, such as ML-SA1 (Kilpatrick et al., 2016). TRPML1 usually mediates local events of Ca2+ release that stimulate autophagy by inducing the nuclear translocation of the Ca2+-sensitive transcription factor, TFEB (Medina et al., 2015; Di Paola et al., 2018). Furthermore, TRPML1-induced local Ca2+ release modulates additional lysosomal functions, including lysosomal exocytosis, membrane trafficking and biogenesis (Di Paola et al., 2018; Medina, 2021). Recent evidence, however, showed that local lysosomal Ca2+ release through TRPML1 can also lead to global elevations in [Ca2+]i via CICR through InsP3Rs (Kilpatrick et al., 2016) or RyRs (Thakore et al., 2020). The Ca2+-dependent crosstalk between TRPML1 and ER/SR-resident Ca2+-permeable channels is, however, seemingly looser as compared to TPCs. In agreement with this evidence, a recent investigation showed that local Ca2+ release events through TRPML1 control water homeostasis in the renal collecting duct (CD, Figure 1) (Scorza et al., 2023).
FIGURE 1. Schematic diagram showing the effect of TRPML1 activation on AQP2-mediated water reabsorption in mouse renal collecting duct cells. ML-SA1 triggers TRPML1-dependent local Ca2+ events that are sustained by the endoplasmic reticulum (ER) Ca2+ content. Activation of the Ca2+/calcineurin/NFAT pathway determines depolymerization of the actin cytoskeleton, thus leading to accumulation of AQP2 at the apical plasma membrane and enhancing water membrane permeability. The putative role of lysosomal Ca2+ signaling events as switch for changes in AQP2 expression level through the modulation of the transcriptional activity of NFAT needs further investigation (question mark). Created with BioRender.com (agreement number: FY259UYCKW).
Facultative water reabsorption in CD cells is finely tuned by a plethora of intracellular signaling mediators and transcription factors (Knepper et al., 2015). Antidiuresis is activated upon the release of the antidiuretic hormone (ADH) by the posterior pituitary gland. Specific binding of ADH with the vasopressin type 2 receptor (V2R), which is localized in principal cells of the CD, stimulates the cAMP/protein kinase A (PKA) axis leading to the apical fusion of the water channel aquaporin 2 (AQP2)-harboring vesicles (Zhao et al., 2023). The rapid apical accumulation of AQP2 boosts water permeability that, in the presence of the strong osmotic gradient in the kidney medulla, is responsible for water reabsorption in the interstitium. The ADH-dependent increase in [Ca2+]i is likewise important to enable the proper fusion of AQP2 vesicles with the plasma membrane. Therefore, it does not come as a surprise that Ca2+ signaling events can independently influence AQP2 expression and translocation even in the absence of cAMP-mediated cues (Chou et al., 2000; Procino et al., 2015; Mamenko et al., 2016; Tomilin et al., 2019). For instance, the antidiabetic drug rosiglitazone facilitates AQP2 apical accumulation and water reabsorption by inducing massive Ca2+ influx upon the specific activation of Transient Receptor Potential Vanilloid 6 (TRPV6) channel (Procino et al., 2015). In addition, a wide variety of TRP channels have been reported in CD cells and CD-derived cultures (Woudenberg-Vrenken et al., 2009). The activation of these channels orchestrates Ca2+ responses that are mainly driven by remarkable Ca2+ influx often associated with additional Ca2+ release from the ER. These robust Ca2+ signals can rapidly invade the bulk of the cytosol thus engaging a number of Ca2+-dependent molecular effectors localized throughout the cell. Conversely, only scarce information is currently available regarding the role of local Ca2+ signals in AQP2-mediated water homeostasis. A recent investigation provided the first evaluation of lysosomal Ca2+ signaling events in renal CD cells, which were evoked by either blocking the vacuolar H-ATPase (V-ATPase) with bafilomycin A1 to deplete the lysosomal Ca2+ pool (Morgan et al., 2015) or activating TRPML1 with the synthetic agonist ML-SA1 (Kilpatrick et al., 2016) (Figure 1). In CD cells, both lysosomal agonists induced robust and long-lasting cytosolic Ca2+ oscillations sustained by tonic ER Ca2+ release through InsP3Rs but not directly associated to lysosomal Ca2+-triggered CICR (Scorza et al., 2023), as widely reported for TPCs (Macgregor et al., 2007; Brailoiu et al., 2009; Kilpatrick et al., 2013; Faris et al., 2019; Moccia et al., 2021b; Faris et al., 2022). This finding strongly suggests that InsP3-mediated ER Ca2+ release drives lysosomal Ca2+ refilling in CD cells. ML-SA1 and bafilomycin A1 differentially modulated AQP2 translocation to the apical membrane and actin polymerization in the cytosol, since only ML-SA1 specifically elicited submaximal water reabsorption in collecting duct cells (Scorza et al., 2023) (Figure 1). Even though ML-SA1 increased water permeability to the same extent as submaximal doses of the cAMP increasing agents forskolin and IBMX, TRPML1 activation was unable to switch on the cAMP/PKA pathway. Currently, the cytosolic Ca2+ effectors translating TRPML1-mediated Ca2+ release into an increase in AQP2-containining vesicle translocation to the apical membrane remain to be deciphered. However, TRPML1-dependent AQP2 translocation and actin depolymerization were inhibited by blocking the Ca2+-dependent phosphatase calcineurin (CaN) with cyclosporine A (Scorza et al., 2023). Intriguingly, CaN is selectively engaged by TRPML1-mediated lysosomal Ca2+ release to drive the nuclear translocation of TFEB (Medina et al., 2015), the master regulator of lysosomal function and autophagy (Di Paola et al., 2018; Medina, 2021) (Figure 1). CaN activation tightly bridges lysosomal Ca2+ signaling events and water reabsorption by directly dephosphorylating cytoskeletal organizing proteins (cofilin, WAVE-1 and synaptopodin) or eliciting long-lasting transcriptional effects mediated by NFAT (Descazeaud et al., 2012). Therefore, it is reasonable to assume that TRPML1 can regulate water balance by influencing the polymerization state of the actin cytoskeleton thus facilitating the fusion of AQP2-harboring vesicles with the apical plasma membrane (Figure 1). Noteworthy, TRPML1 induced Ca2+ events have been associated with fusion of gastric tubulovesicles carrying the H+/K+-ATPase that pumps H+ into the gastric lumen (Sahoo et al., 2017).
Mitochondrial enzymes and F0F1 ATP synthase require Ca2+ for activation and maintenance of bioenergetic activity and production of ATP. Mitochondria uptake Ca2+ with a high affinity directly from juxtaposed InsP3Rs located in mitochondria-associated ER membranes (MAMs) (Rizzuto et al., 1993). The morpho-functional complex that holds together interacting ER and mitochondria is referred to as mitochondria-ER contact sites (MERCS) (Herrera-Cruz and Simmen, 2017). Ca2+ transfer at MERCS occurs through a complex composed of InsP3Rs, voltage-dependent anion channel 1 (VDAC1) and the associated protein Grp75, and then, into mitochondrial matrix, via a low affinity mitochondrial Ca2+ uniporter. Besides Ca2+ fluxes, MERCS are responsible for a number of key cellular processes, such as lipid and steroid biogenesis, mitochondrial fission and dynamics, autophagosome formation, apoptosis induction, and others (Barazzuol et al., 2021). Disruption of MERCS has been observed in several neurodegenerative diseases, including Parkinson’s disease, amyotrophic lateral sclerosis and Alzheimer’s disease (AD) (Paillusson et al., 2016; Area-Gomez and Schon, 2017; Lim et al., 2021a; Leal and Martins, 2021). In AD, a strengthening of the interaction between ER and mitochondria has been found in human brains and in animal and cellular AD models (Lim et al., 2021a). Although such increase has been associated with mitochondrial dysfunction and with aberrant processing of amyloid precursor protein (APP), mechanistic aspects MERCS alterations and cause-effect relationships with AD-related cellular pathology remain poorly understood (Lim et al., 2021a; Lim et al., 2023).
AD, a major, yet uncurable, age-related neurological disorder, has a long-lasting pathogenesis with poorly characterized preclinical and prodromal phases. Cellular dysfunctions, such as alterations of protein synthesis and degradation with associated accumulation of misfolded/aggregated proteins, mitochondrial dysfunction with concomitant bioenergetic deficit and oxidative stress, and derangement of Ca2+ homeostasis and signalling, represent early signs of AD pathology (De Strooper and Karran, 2016). Yet, these dysfunctions have mainly been studied and interpreted from the point of view of neuronal pathology, while alterations in glial cells, specifically in astrocytes, have been largely overlooked (Verkhratsky et al., 2019; Merlo et al., 2021). Astrocytes are homeostatic and supportive cells in the central nervous system (CNS), which warrant correct development, function and adaptation of neurons and other cells in the CNS to activity and stress (Verkhratsky and Nedergaard, 2016; Santello et al., 2019; Tapella et al., 2020). They participate in formation of morpho-functional units in the brain, such as blood-brain barrier (BBB) and neurovascular unit (Schaeffer and Iadecola, 2021), and are responsible for metabolic, structural and functional support to neurons. In AD pathogenesis, astrocytes undergo complex biphasic alterations, first becoming asthenic and atrophic, to turn to hypertrophy and reactivity at later AD stages in concomitance with the development of senile plaques and neurofibrillary tangles accompanied by remodelling of astrocytic Ca2+ signalling (Lim et al., 2014; Lim et al., 2016b; Verkhratsky et al., 2019). Reactive astrocytes, in association with microglial cells, participate in the development of neuroinflammatory reaction. During these transformations, astrocytes lose their homeostatic and defensive functions and leave neurons to suffer damage, lose synaptic connectivity and die. Little is known about astrocytic cell pathology during early AD pathogenesis.
Unexpectedly, recent findings suggest that the alterations of MERCS and ER-mitochondrial Ca2+ transport may be responsible for a number of cellular dysfunctions, which may explain the loss of homeostatic function by AD astrocytes. These studies took advantage of a novel model of immortalized hippocampal astrocytes from 3xTg mouse model of AD, which faithfully reproduce transcriptional and functional alterations of primary AD astrocytes (Ruffinatti et al., 2018; Rocchio et al., 2019). Moreover, their produce and release β-amyloid peptide and have impaired autophagic and proteasomal protein degradation, which are signs of early cellular dysfunction in AD (Gong et al., 2023). Immortalized WT and 3xTg-AD astrocytes, referred to as WT-iAstro and 3Tg-iAstro, represent versatile and easy-to-handle astrocytic AD model, well suited for comprehensive investigation from single cell imaging and transfection to omics analyses and sub-cellular fractionation requiring large amount of material (Tapella et al., 2023). First, it was assessed whether 3Tg-iAstro present mitochondrial alterations characteristic for AD cells. 3Tg-iAstro cells have a lower basal mitochondrial respiration and severely impaired respiratory reserve, significantly lower mitochondrial ATP production and significantly higher mitochondrial ROS. Glycolytic activity was also impaired in 3Tg-iAstro compared with WT-iAstro cells. This was in line with recent reports on AD-derived human iPSC-differentiated astrocytes (Oksanen et al., 2017; Oksanen et al., 2019). Proteomics analysis on isolated mitochondria and associated ER membranes were also conducted. Surprisingly, differentially expressed proteins were found to be mainly responsible for ER functions and ribosomal proteins synthesis (Dematteis et al., 2020). Validation of these results showed that 3Tg-iAstro cells presented a lower rate of basal protein synthesis and low-grade chronic ER stress accompanied by an increased phosphorylation of eukaryotic initiation factor 2α (p-eIF2α). Gong et al. (2023) found that proteasomal and autophagic activities are impaired in 3Tg-iAstro cells. Moreover, 3Tg-iAstro, but not WT-iAstro cells, were unable to promote the formation of the bidimensional tubular network, which is the in vitro surrogate of in vivo blood vessel formation (Balbi et al., 2019; Balducci et al., 2021), in an in vitro astrocyte/pericyte/endothelial 3D co-culture due to a loss of secreted factors, thereby suggesting the impairment of key homeostatic functions (Tapella et al., 2022). These alterations were also found in hippocampus of 3xTg-AD mice in vivo (Tapella et al., 2022).
Next, it was investigated if 3Tg-iAstro presented alterations of Ca2+ homeostasis (Lim et al., 2021b). A significant increase of steady-state ER Ca2+ level and higher ATP-induced Ca2+ signals in the cytosolic compartment, indicating a higher Ca2+ ER load and higher InsP3R-mediated Ca2+ release, were reported. This was in accord with previous reports (Grolla et al., 2013a; Grolla et al., 2013b; Lim et al., 2013; Ronco et al., 2014). However, unexpectedly, ATP-induced Ca2+ transients, measured in mitochondrial matrix, were significantly lower in 3Tg-iAstro compared with WT-iAstro cells, indicating on the alterations with ER-mitochondrial Ca2+ transport. This was in line with the increased ER-mitochondrial interaction at a distance of 8–10 nm, which we have demonstrated using a split-GFP ER-mitochondrial contact site sensor (SPLICS) (Cieri et al., 2018; Dematteis et al., 2020). To investigate if the increased ER-mitochondrial interaction and the impaired mitochondrial Ca2+ signals were responsible for alterations of cellular proteostasis, an artificial linker that fixes the ER and the outer mitochondrial membrane at a short distance of about 10 nm was overexpressed in WT-iAstro cells, thereby reproducing MERCS and Ca2+ alterations found in 3Tg-iAstro cells. Strikingly, fixing MERCS at 10 nm reproduced the impairment of ribosomal protein synthesis and increased p-eIF2α levels. Moreover, as reported for 3Tg-iAstro cells, WT-iAstro cells overexpressing 10 nm linker were unable to support tubulogenesis in vitro in 3D co-culture with pericytes and endothelial cells (Tapella et al., 2022).
Taken together, these results provide proof of principle that the shortening of ER-mitochondrial distance, observed in AD, may be causative for a number of cellular AD-related alterations. Furthermore, our results suggest that the altered MERCS function in AD astrocytes may result in impairment of CNS homeostasis, BBB and neuronal dysfunction (Figure 2). Further experiments are necessary to elucidate molecular mechanisms of MERCS dysfunction and dissect the role of impaired ER-mitochondrial Ca2+ transfer in AD pathogenesis.
FIGURE 2. Proposed scheme relationships between AD-related mutations, mitochondrial-ER interaction, mitochondrial and ER Ca2+ signaling, and cellular dysfunctions in astrocytes. Altered ER-mitochondrial interaction impairs ER-mitochondrial Ca2+ transfer, resulting in mitochondrial bioenergetic deficit and increased production of ROS, induction of a low-grade chronic ER stress and derangement of proteins synthesis and degradation. Cellular dysproteostasis results in an impaired secretion of factors including adhesion molecules, components of extracellular matrix, pro-neurogenic and neuroprotective molecules. Altogether, this impairs homeostatic and signaling activity of AD astrocytes eventually leading to impairment of synaptic functions, blood-brain barrier integrity and to development of neurodegeneration.
TRPM8 is a member of the TRP family primarily known for its classical cold receptor function in sensory neurons required for cold thermal transduction and response as well as pain sensation in mammals (McKemy et al., 2002; Madrid et al., 2006; Dhaka et al., 2008; Knowlton et al., 2013). The first identified “full-length” isoform of TRPM8 consists of a homotetrameric protein of 1,104 amino acid (128 kDa) organized into six hydrophobic transmembrane α-helices (S1-S6) with a transmembrane loop between S5 and S6, and cytosolic tetrameric coiled-coil COOH-terminal domain (C-term) and a large hydrophilic NH2-terminal domain (N-term) containing ‘TRPM homology regions’ (MHR) involved in channel assembly and trafficking (Kraft and Harteneck, 2005; Fujiwara and Minor, 2008; Yin et al., 2018). The voltage sensor-like domain (VSLD) is defined by the first 4 TM helices (S1-S4) and also contains the binding sites for menthol and icilin at the cavity formed with the TRP domain (Bandell et al., 2006; Yin et al., 2019). The pore module of TRPM8 is, instead, formed by the last 2 TM helices (S5-S6) and it is characterized by a highly conserved hydrophobic region and a conserved aspartate residue, responsible for ion selectivity (PCa/PNa = 3.3) (Zholos et al., 2011). Interestingly, this full length TRPM8 is mainly localized in the plasma membrane but is also partly present at the ER level where it functions by releasing Ca2+ form the store (Chinigo et al., 2022).
Beside this well know role in thermal transduction, the human TRPM8 gene was first identified and cloned from prostate tissues and described as a new prostate-specific gene due to the peculiar expression pattern shown during prostate cancer (PCa) progression (Tsavaler et al., 2001). In particular, TRPM8 is upregulated in benign hyperplasia (BPH) and during the early androgen-dependent stages of PCa, and then downregulated in the more advanced androgen-independent metastatic stages of the tumor. Consistent with its unique deregulation during PCa progression, alterations in TRPM8 channel activity have been linked to several cancer hallmarks, including tumor cell proliferation and survival, cell migration, and angiogenesis (Alaimo et al., 2020; Grolez et al., 2022).
However, the impact of TRPM8 in the development and progression of PCa is subject to complex modulation mechanisms that also underlie the expression of different isoforms with distinct subcellular localization and activity depending on tumor stage and androgen sensitivity. Indeed, the expression of the full-length isoform of TRPM8 located on the plasma membrane (TRPM8PM) is highly subject to androgen regulation and thus is significantly downregulated in androgen deprivation and androgen receptor (AR) loss during the late androgen-independent phase of PCa (Zhang and Barritt, 2004; Bidaux et al., 2005; Grolez et al., 2019). This regulation occurs through both genomic and non-genomic mechanisms involving the AR (Figure 3) (Bidaux et al., 2005; Grolez et al., 2019). As regarding in particular the non-genomic action, the role of AR-TRPM8 interaction is tightly regulated by testosterone in a dose-dependent manner: low doses of testosterone (10 nM) are associated with AR-TRPM8 localization at the level of lipid rafts and a significant inhibition of TRPM8 activity which in turn lead to an increase in cell motility as compared with the absence of testosterone; on the other hand, high doses of testosterone (100 nM) lead to a decrease of TRPM8-AR interaction thus reverting the inhibitory effect of AR on TRPM8 activity (Grolez et al., 2019). This loss of interaction and delocalization of TRPM8 outside of lipid rafts, significantly increases prostate cancer cell motility (Grolez et al., 2019).
FIGURE 3. Schematic representation of TRPM8 subcellular localization and activity in cancer cells. TRPM8 Full length isoform localizes at the plasma membrane and is subjected to androgen regulation. Smaller isoforms typically localize in the ER and mediate Ca2+ release in the cytosol or Ca2+ transfer in the mitochondria. TRPM8 also act independently from its channel activity as an inhibitor of the small GTPase Rap1A thus inhibiting cell adhesion and migration. Created with BioRender.com (agreement number: AT259UYHZ3).
Beside the role of the full-length TRPM, during the transition from androgen-dependent to androgen-independent phases of PCa, through an alternative splicing mechanism, the “full-length” isoform of TRPM8 gives way to a shorter isoform with typical ER localization, known as TRPM8ER (Bidaux et al., 2007). The TRPM8ER isoform, being able to directly release ER Ca2+ and thereby activate SOCE on the plasma membrane, is mainly involved in the control of Ca2+-dependent pro-apoptotic mechanisms (Figure 3) (Thebault et al., 2005; Prevarskaya et al., 2007). Interestingly, the pro-apoptotic role of TRPM8 has also been confirmed in PCa cells treated with sub-lethal doses of radio, hormonal, or chemo therapies (Alaimo et al., 2020; Genovesi et al., 2022). Furthermore, other isoforms of the channel have been identified to date in the prostate. A functional TRPM8ER characterized by only 4 rather than 7 transmembrane domains (TMDs) has been identified and characterized as a mediator of the Ca2+ transfer from the ER to the mitochondria in PCa epithelial cells (Figure 2) (Bidaux et al., 2018), while short non-channel TRPM8 isoforms (sM8s) with ubiquitous cytosolic localization in PCa were found to exert antagonist functions towards the full-length isoform (Peng et al., 2015; Bidaux et al., 2016). sM8s are a first example of non-channel function of TRPM8 that influences cell behavior independently of pore function and Ca2+ mobilization (Fernandez et al., 2012). Therefore, the growth of primary PCa as a result of the equilibrium between proliferation and apoptosis may depend on the relative expression levels of the different TRPM8 isoforms with channel and non-channel functioning.
In addition, TRPM8 regulates cell migration through both Ca2+-dependent and Ca2+-independent mechanisms. TRPM8-mediated Ca2+ signals induce an increase in the expression and activity of some proteins that are crucial in the epithelial-to-mesenchymal transition (EMT), in focal adhesion dynamics and consequently in the control of cell adhesion and migration (Noren et al., 2000; Millar et al., 2017). In particular, Cdc42, Rac1, ERK, and FAK are stimulated in a Ca2+-dependent manner by TRPM8 activity in PCa cells (Yang et al., 2009; Zhu et al., 2011; Wang et al., 2012; Grolez et al., 2022). On the other hand, the involvement of TRPM8 in the migratory machinery goes beyond its channel function. Indeed, a novel facet of TRPM8 as an inhibitor of the small GTPase Rap1A that is completely independent of its cation channel activity has recently been unveiled (Figure 3) (Genova et al., 2017; Chinigo et al., 2022). More specifically, a direct physical interaction between TRPM8 and Rap1A has been characterized in both PCa-derived endothelial cells and epithelial PCa cells (Genova et al., 2017; Chinigo et al., 2022). The interaction site is located on the NH2-terminus of the channel and involves the glutamate 207 and the tyrosine 240, which directly interact with some residues (including tyrosine 32) located within the switch I region of Rap1A, responsible for the transition from the inactive to the active form of the small GTPase (Chinigo et al., 2022). Indeed, Rap1A, as a small GTPase, co-exists in two different forms: an active form when bound to GTP and an inactive form when bound to the GDP (Vetter and Wittinghofer, 2001). Specific guanine exchange factors (GEFs) catalyze the exchange between GDP and GTP thereby inducing small GTPase activation, which normally results in the promotion of cell adhesion through the activation of the β1-integrin signaling at the plasma membrane (Chrzanowska-Wodnicka et al., 2008; Boettner and Van Aelst, 2009; Carmona et al., 2009; Cherfils and Zeghouf, 2013). Recent work demonstrated that TRPM8 intracellularly binds Rap1A mainly at the ER in its inactive form, thus hindering its translocation to the plasma membrane and its subsequent activation (Genova et al., 2017; Chinigo et al., 2022). This mechanism results in the inhibition of cell adhesion and migration in PCa-derived endothelial cells and in epithelial PCa cells, thus making TRPM8 an appealing candidate to block both tumor invasiveness and angiogenesis (Genova et al., 2017; Chinigo et al., 2022). Although TRPM8 expression is sufficient to exert these functional effects, stimulation with TRPM8 agonists, such as icilin and WS12, further potentiates these effects not only by recruiting Ca2+-dependent pathways, such as Cdc42, Rac1, ERK, and FAK, but also by probably promoting TRPM8-Rap1 interaction. This could be explained by global conformational rearrangements triggered by agonist binding in the TRPM8 TMDs that are propagated to the cytosolic domain where interaction with Rap1A occurs (Yin et al., 2018; Yin et al., 2019). Rap1A is not the only GTPase involved in the TRPM8 interactome. Indeed, TRPM8 was found to interact with the inactive form of the G-protein subunit Gαq, which leads to the inhibition of TRPM8 gating and, in turn, may be subject to TRPM8-mediated metabotropic regulation (Klasen et al., 2012; Zhang et al., 2012). These data fit into the broader context of a bidirectional close interplay between TRP channels and small GTPases at all stages of the metastatic cascade through both Ca2+-dependent and Ca2+-independent pathways (Chinigo et al., 2020).
All these recent mechanistic findings on TRPM8 provide new insights for the development of innovative and effective tools targeting TRPM8 to block PCa progression and improve the prognosis of the currently incurable metastatic castration-resistant prostate cancer (mCRPC) phenotypes. In addition to supporting a potential use of TRPM8 in anti-tumor therapy as a dual target to simultaneously counteract metastatic dissemination and angiogenesis, they also shed new light on the possibility of using TRP channels as targets for the development of peptidomimetics in cancer therapy. In fact, the administration of therapeutic peptide mimicking the channel or part of its structure would further reduce any side effects associated with the wide tissue distribution of TRP channels and the multitude of intracellular signalling pathways regulated by them, directly targeting a specific protein-protein interaction and consequently impairing only its associated cellular pathways (Mabonga and Kappo, 2019; Tsagareli and Nozadze, 2020). As to TRPM8-Rap1A interaction, the applicability of a peptide that reproduces the N-terminus of the channel in patients in androgen-independent late stages of PCa seems to be further supported by the fact that none of the residues involved in this interaction were mutated in the analyzed patient cohorts (Chinigo et al., 2022). Of note, validation of TRPM8-Rap1A interaction in more than 1 cell line (Genova et al., 2017; Chinigo et al., 2022), including prostate, breast, and cervical cancer cells as well as endothelial cells, suggests a broader spectrum of action of TRPM8 as an inhibitor of Rap1, albeit with a different impact in terms of control of cell adhesion and migration according to the cell type. Therefore, this protein-protein interaction could prove to be an interesting target in the treatment of a much wider range of pathologies.
The idea to use light to trigger specific biological pathways, including Ca2+ signalling, represents one of the most fascinating insights in modern science (Lodola and Moccia, 2022). In recent years, photostimulation of cells and living systems has received great interest from the scientific community due to several unique advantages. Indeed, light is a minimally invasive biophysical tool that can overcome the limitations of more conventional stimulation approaches based on electrical, chemical, mechanical, or magnetic cues (i.e., limited spatial and temporal resolution) (Di Maria et al., 2018). The potential revolutionary role of light has been originally suggested by Sir Francis Crick. The Nobel Prize for Physiology or Medicine, discussing the need to achieve a selective control of individual neurons to understand the complexity of the brain, asserted that “The ideal signal would be light, probably at an infrared wavelength to allow the light to penetrate far enough. This seems rather farfetched, but it is conceivable that molecular biologists could engineer a particular cell type to be sensitive to light in this way” (Crick, 1999). This revolutionary concept become reality few years later with the implementation of Optogenetics, which consists in the expression of light-sensitive ion channels into the cellular plasma membrane to control the activity of neurons or other cell types with light (Deisseroth, 2011). However, the standard method to deliver the light-sensitive sensors-actuators to the target cells membrane impinges on viral constructs and this, combined with the fact that the exogenous proteins are isolated from very distant species (i.e., bacteria, algae, or unicellular fungi), open a series of issues in the therapeutic translatability of the approach.
An alternative strategy to still preserve the advantages of optical stimulation, but avoiding genetic modification, relies on the use of photosensitive transducers (Di Maria et al., 2018; Hopkins et al., 2019). The foundation of this approach is built on the convergence of various cutting-edge expertise ranging from biology, material science and photonics. In recent years, both inorganic and organic semiconductors have been used with excellent results (Di Maria et al., 2018; Hopkins et al., 2019). In particular, the organic one has aroused considerable interest within the scientific community due to their unique characteristics. In fact, these materials support both ionic and charge transfer, are soft and conformable, cost-effective and solution processable, but most importantly their absorption range is in the visible region, and they present a high biocompatibility, thus proving capable of interfacing with living matter to transduce light into a biological signal. Regioregular polymer poly(3-hexylthiophene-2,5-diyl), referred as P3HT, is probably the workhorse material among the organic semiconductors and the widely studied for biological purposes (Antognazza et al., 2015; Di Maria et al., 2018; Moccia et al., 2020).
The main photophysical mechanisms that occur at the polymer/cell interface could be capacitive, electrochemical, or thermally mediated. These phenomena in turn generate different cellular response. For example, at the cellular level, planar P3HT has been proven effective in the modulation of the membrane potential of non-excitable cells (i.e., HEK-293 cells and astrocytes) up to the optical stimulation/silencing of neuronal firing (Ghezzi et al., 2011; Benfenati et al., 2014; Antognazza et al., 2015; Feyen et al., 2016; Di Maria et al., 2018). Notably, its efficacy is not limited to in vitro applications. Indeed P3HT-based hybrid interfaces (Ghezzi et al., 2013; Antognazza et al., 2016; Maya-Vetencourt et al., 2017), and more recently also nanoparticles (Maya-Vetencourt et al., 2020), were also shown to restore light-sensitivity and visual acuity in animal models of retinal degeneration evidencing novel potential biomedical implications of conjugated polymers.
The modulation of cellular fate via electrochemical and/or thermal signals could be achieved by modulation of [Ca2+]i (Bossio et al., 2018; Moccia et al., 2022). Recently, it has been demonstrated that P3HT photoexcitation led to the activation of the non-selective cation channel Transient Receptor Potential Vanilloid 1 (TRPV1) channel (Lodola et al., 2017b; Moccia et al., 2020; Moccia et al., 2022). TRPV1 is a non-selective cation channel that can integrate a variety of extracellular cues (Moccia et al., 2020; Moccia et al., 2022), including an increase in ROS (Guarini et al., 2012), an increase in temperature >40 °C (Caterina et al., 1997), and by a reduction in extracellular pH (Jordt et al., 2000). In accord, P3HT photoexcitation can stimulate TRPV1-mediated membrane depolarization via the local increase in temperature and ROS concentration at the interface between PH3T thin films and cell membrane (Lodola et al., 2017b). Further studies showed that optical excitation of P3HT thin films induced intracellular Ca2+ oscillations in human circulating endothelial colony forming cells ECFCs) (Negri et al., 2022a), a truly endothelial progenitor population that is mobilized in peripheral circulation upon an ischemic insult to regenerate the damaged vascular networks (Moccia et al., 2018). TRPV1-mediated Ca2+ signals were mainly elicited by local ROS generation and were supported by InsP3-induced ER Ca2+ release and SOCE (Negri et al., 2022a). Of note, light-induced intracellular Ca2+ oscillations were reminiscent of the repetitive Ca2+ spikes whereby vascular endothelial growth factor (Dragoni et al., 2011; Dragoni et al., 2015; Lodola et al., 2017a) and the human amniotic fluid stem cell secretome (Balducci et al., 2021) induce the nuclear translocation of NF-κB to stimulate ECFC proliferation and tube formation. In agreement with these observations, optical excitation of P3HT thin films was found to boost ECFC pro-angiogenic activity by activating TRPV1 and thereby promoting a NF-κB-dependent gene expression program (Figure 4A) (Lodola et al., 2019a). These findings pave the way towards the use of these materials as a reliable tool for precise and reversible optically-driven modulation of ECFC physiological activity (Zhang et al., 2014; Lodola et al., 2019a; Moccia et al., 2020; Moccia et al., 2022).
FIGURE 4. Geneless light stimulation of Ca2+ signals in cardiac cells. (A) Polymer-mediated optical excitation induces a robust enhancement of proliferation and bidimensional tube formation in ECFCs seeded on top of P3HT thin films (λ = 520 nm). ECFC modulation in ECFCs requires TRPV1 activation on the plasma membrane, which in turn mediates extracellular Ca2+ entry to engage a NF-kB-dependent gene expression program. (B) Ziapin2 internalizes into the plasma membrane of human induced pluripotent stem cell-derived cardiomyocytes (hiPSC-CMs). Upon photoexcitation (λ = 470 nm) the molecule isomerizes, changing hiPSC-CMs membrane capacitance. This triggers action potential generation and consequently modulates the “excitation-contraction coupling” process at a whole extent opening a new way towards hybrid soft robotics and heart disease therapies. Adapted from (Vurro et al., 2023a). Created with BioRender.com (agreement number: RO259UYLP6).
The same interface has been applied also to optical increase the contractile activity of human induced pluripotent stem cell-derived cardiomyocytes (hiPSC-CMs), a process where Ca2+ is the actual coupling between excitation occurring in the sarcolemma and the onset of mechanical contraction (Bers and Guo, 2005). Although in this experimental setting the physical process was photothermal, P3HT still presents advantages over more traditional stimulation methods, thereby opening interesting perspectives for the control of cardiac pacing (Lodola et al., 2019b).
Within this context, an alternative approach involves photochromic compounds (Wang and Li, 2018). These organic molecules undergo reversible transformation between two metastable states following the absorption of an electromagnetic radiation and provide a conceptually simple and convenient way to control cellular activity. Indeed, photoswitches can bind covalently to ion channels/receptors or be targeted directly to the plasma membrane bilayer, thus modifying, upon photoisomerization, the ion channel dynamics and/or the electrical properties of the membrane (Gorostiza and Isacoff, 2008; Izquierdo-Serra et al., 2016; Leippe et al., 2017). Recently, a newly synthetized amphiphilic azobenzene-based photo-transducer (Ziapin2), successfully tested in bacteria, HEK-293 cells and neurons (Paterno et al., 2020a; Paterno et al., 2020b; DiFrancesco et al., 2020; Magni et al., 2022), has been used as a non-invasive optical tool to trigger hiPSC-CMs contraction behavior (Vurro et al., 2023a). Thanks to its peculiar chemical properties Ziapin2 has the capability to dwell within the hiPSC-CMs sarcolemma. In this environment the molecule photoisomerization induces a heatless mechanical perturbation upon millisecond pulse of visible light that leads to a dynamic modulation of membrane capacitance. This change in the passive electrical property of the cell results in a transient hyperpolarization followed by a delayed depolarization able to elicit an action potential. The electrical activity correlates with changes in Ca2+ dynamics and ultimately with an increase in the contraction rate (Figure 4B). The photopacing efficacy of the approach has been further extended to a cardiac microphysiological model that mimics the cellular organization and substrate mechanical properties of native cardiac tissue (Vurro et al., 2023b), thus proving that Ziapin2 could be a viable tool for the modulation of the excitation-contraction coupling with a precise spatial and temporal punctuality.
The Symposium “Ca2+ signalling: unexpected new roles for the usual suspect” has been one of the most attended events of the 72nd Annual Meeting of the Italian Society of Physiology. In our opinion, this was not only due to the widespread function of the Ca2+ handling machinery, which plays fundamental and diversified roles in human physiology that could of course gather vast interest by the audience. We believe that the Symposium gathered such a large audience since it aimed at a presenting one of the oldest signalling messengers known, i.e., Ca2+, from a novel perspective. It is now clear that the Ca2+ handling machinery is no longer limited, to quote a few paradigmatic examples, to intracellular Ca2+ stores that exclusively located in the ER or to voltage-gated Ca2+ channels and ligand-gated channels on the plasma membrane. Lysosomes and mitochondria are also crucial to shape the physiological Ca2+ response to extracellular cues by, respectively, amplifying, or modulating ER Ca2+ release. Altering this delicate balance of inter-organellar Ca2+ fluxes can lead to life-threatening disorders, such as AD, cancer, and lysosomal storage disorders, and many more are likely to be discovered in the next future. The non-canonical function of ion channels, exemplified by TRPM8-Rap1A interaction, represents another revolutionary field of research showing that classical omics technologies, such as single-cell RNA sequencing or mass spectrometry, need to be integrated by a physiological approach to truly understand the signalling mode of a channel transcript/protein. These emerging pieces of information on the heterogeneity and versatility of the Ca2+ handling machinery can be exploited to design alternative strategies to selectively rescue the function of diseased cells by combining novel nanotechnologies with a proper knowledge of molecular physiology. Due to its polymodal nature, TRPV1 is certainly the best molecular switch to translate optical stimulation of photosensitive conjugated polymers into a biologically relevant signal. But other candidates presenting similar sensitivity to heat and ROS, such as TRP Ankyrin 1, are likely to be rapidly integrated in the arsenal of Ca2+-permeable channels that could be probed for their therapeutic potential. In conclusion, this Symposium, which also engendered may fruitful discussions and opened the way to new collaborations among the participants (including many foreigner guests), confirmed that Italian Physiology is at the forefront of research in Ca2+ signalling, as also proven by many other oral and poster presentations of the meeting (Martinotti et al., 2019; Nesher et al., 2019; Badone et al., 2021; Lazzarini et al., 2022; Michelucci et al., 2022; Sforna et al., 2022; Arici et al., 2023; Lia et al., 2023).
All authors listed have made a substantial, direct, and intellectual contribution to the work and approved it for publication.
The authors gratefully acknowledge financial support from: Fondo Ricerca Giovani from the University of Pavia (FM); #NEXTGENERATIONEU (NGEU) and funded by the Ministry of University and Research (MUR), National Recovery and Resilience Plan (NRRP), project MNESYS (PE0000006)—A Multiscale integrated approach to the study of the nervous system in health and disease (DN. 1553 11.10.2022) (FM); EU Horizon 2020 FETOPEN-2018-2020 Programme “LION-HEARTED”, grant agreement n. 828984 (FM and FL); PRIN-2017 “Lioness” project (#GA 754345) from Italian Ministry for Education, University and Research (MIUR) (AF); FAR-2019 from the Università del Piemonte Orientale (DL); Fondo di Ateneo (2020-ATE-0044) from the University of Milano-Bicocca (FL).
The authors gratefully acknowledge all their coworkers for the enthusiasm and incredible commitment to their research projects on Ca2+ signalling. The Article Processing Charge has been paid by the Italian Society of Physiology, which we gratefully acknowledge.
The authors declare that the research was conducted in the absence of any commercial or financial relationships that could be construed as a potential conflict of interest.
The reviewer DG declared a past collaboration with the author AFP to the handling editor.
All claims expressed in this article are solely those of the authors and do not necessarily represent those of their affiliated organizations, or those of the publisher, the editors and the reviewers. Any product that may be evaluated in this article, or claim that may be made by its manufacturer, is not guaranteed or endorsed by the publisher.
Abdullaev, I. F., Bisaillon, J. M., Potier, M., Gonzalez, J. C., Motiani, R. K., and Trebak, M. (2008). Stim1 and Orai1 mediate CRAC currents and store-operated calcium entry important for endothelial cell proliferation. Circ. Res. 103, 1289–1299. doi:10.1161/01.RES.0000338496.95579.56
Alaimo, A., Lorenzoni, M., Ambrosino, P., Bertossi, A., Bisio, A., Macchia, A., et al. (2020). Calcium cytotoxicity sensitizes prostate cancer cells to standard-of-care treatments for locally advanced tumors. Cell Death Dis. 11, 1039. doi:10.1038/s41419-020-03256-5
Aley, P. K., Singh, N., Brailoiu, G. C., Brailoiu, E., and Churchill, G. C. (2013). Nicotinic acid adenine dinucleotide phosphate (NAADP) is a second messenger in muscarinic receptor-induced contraction of Guinea pig trachea. J. Biol. Chem. 288, 10986–10993. doi:10.1074/jbc.M113.458620
Antognazza, M. R., Di Paolo, M., Ghezzi, D., Mete, M., Di Marco, S., Maya-Vetencourt, J. F., et al. (2016). Characterization of a polymer-based, fully organic prosthesis for implantation into the subretinal space of the rat. Adv. Healthc. Mater 5, 2271–2282. doi:10.1002/adhm.201600318
Antognazza, M. R., Martino, N., Ghezzi, D., Feyen, P., Colombo, E., Endeman, D., et al. (2015). Shedding light on living cells. Adv. Mater 27, 7662–7669. doi:10.1002/adma.201403513
Arcangeli, A., Duranti, C., Iorio, J., and Lastraioli, E. (2023). The role of potassium channels in tumours of the gastrointestinal tract: A focus on the human ether-a-go-go related gene 1 channels. J. Physiol. 601, 1597–1610. doi:10.1113/JP282310
Area-Gomez, E., and Schon, E. A. (2017). On the pathogenesis of alzheimer's disease: The MAM hypothesis. FASEB J. 31, 864–867. doi:10.1096/fj.201601309
Arici, M., Ferrandi, M., Barassi, P., Hsu, S. C., Torre, E., Luraghi, A., et al. (2023). Istaroxime metabolite PST3093 selectively stimulates SERCA2a and reverses disease-induced changes in cardiac function. J. Pharmacol. Exp. Ther. 384, 231–244. doi:10.1124/jpet.122.001335
Astesana, V., Faris, P., Ferrari, B., Siciliani, S., Lim, D., Biggiogera, M., et al. (2021). [Pt(O,O'-acac)(γ-acac)(DMS)]: Alternative strategies to overcome cisplatin-induced side effects and resistance in T98G glioma cells. Cell Mol. Neurobiol. 41, 563–587. doi:10.1007/s10571-020-00873-8
Atakpa, P., Thillaiappan, N. B., Mataragka, S., Prole, D. L., and Taylor, C. W. (2018). IP3 receptors preferentially associate with ER-lysosome contact sites and selectively deliver Ca(2+) to lysosomes. Cell Rep. 25, 3180–3193.e7. doi:10.1016/j.celrep.2018.11.064
Bading, H. (2013). Nuclear calcium signalling in the regulation of brain function. Nat. Rev. Neurosci. 14, 593–608. doi:10.1038/nrn3531
Badone, B., Ronchi, C., Lodola, F., Knaust, A. E., Hansen, A., Eschenhagen, T., et al. (2021). Characterization of the PLN p.Arg14del Mutation in Human Induced Pluripotent Stem Cell-Derived Cardiomyocytes. Int. J. Mol. Sci. 22, 13500. doi:10.3390/ijms222413500
Bagur, R., and Hajnoczky, G. (2017). Intracellular Ca(2+) sensing: Its role in calcium homeostasis and signaling. Mol. Cell 66, 780–788. doi:10.1016/j.molcel.2017.05.028
Balbi, C., Lodder, K., Costa, A., Moimas, S., Moccia, F., Van Herwaarden, T., et al. (2019). Reactivating endogenous mechanisms of cardiac regeneration via paracrine boosting using the human amniotic fluid stem cell secretome. Int. J. Cardiol. 287, 87–95. doi:10.1016/j.ijcard.2019.04.011
Balducci, V., Faris, P., Balbi, C., Costa, A., Negri, S., Rosti, V., et al. (2021). The human amniotic fluid stem cell secretome triggers intracellular Ca2+ oscillations, NF-κB nuclear translocation and tube formation in human endothelial colony-forming cells. J. Cell Mol. Med. 25, 8074–8086. doi:10.1111/jcmm.16739
Bandell, M., Dubin, A. E., Petrus, M. J., Orth, A., Mathur, J., Hwang, S. W., et al. (2006). High-throughput random mutagenesis screen reveals TRPM8 residues specifically required for activation by menthol. Nat. Neurosci. 9, 493–500. doi:10.1038/nn1665
Barak, P., and Parekh, A. B. (2020). Signaling through Ca(2+) microdomains from store-operated CRAC channels. Cold Spring Harb. Perspect. Biol. 12, a035097. doi:10.1101/cshperspect.a035097
Barazzuol, L., Giamogante, F., and Cali, T. (2021). Mitochondria associated membranes (MAMs): Architecture and physiopathological role. Cell Calcium 94, 102343. doi:10.1016/j.ceca.2020.102343
Bartok, A., Weaver, D., Golenar, T., Nichtova, Z., Katona, M., Bansaghi, S., et al. (2019). IP(3) receptor isoforms differently regulate ER-mitochondrial contacts and local calcium transfer. Nat. Commun. 10, 3726. doi:10.1038/s41467-019-11646-3
Benfenati, V., Martino, N., Antognazza, M. R., Pistone, A., Toffanin, S., Ferroni, S., et al. (2014). Photostimulation of whole-cell conductance in primary rat neocortical astrocytes mediated by organic semiconducting thin films. Adv. Healthc. Mater 3, 392–399. doi:10.1002/adhm.201300179
Bernardini, M., Brossa, A., Chinigo, G., Grolez, G. P., Trimaglio, G., Allart, L., et al. (2019). Transient receptor potential channel expression signatures in tumor-derived endothelial cells: Functional roles in prostate cancer angiogenesis. Cancers (Basel) 11, E956. doi:10.3390/cancers11070956
Berra-Romani, R., Brunetti, V., Pellavio, G., Soda, T., Laforenza, U., Scarpellino, G., et al. (2023). Allyl isothiocianate induces Ca(2+) signals and nitric oxide release by inducing reactive oxygen species production in the human cerebrovascular endothelial cell line hCMEC/D3. Cells 12, 1732. doi:10.3390/cells12131732
Berra-Romani, R., Guerra, G., and Moccia, F. (2021). Editorial: Advances and current challenges in calcium signaling within the cardiovascular system. Front. Physiol. 12, 696315. doi:10.3389/fphys.2021.696315
Berridge, M. J., Bootman, M. D., and Roderick, H. L. (2003). Calcium signalling: Dynamics, homeostasis and remodelling. Nat. Rev. Mol. Cell Biol. 4, 517–529. doi:10.1038/nrm1155
Bers, D. M. (2008). Calcium cycling and signaling in cardiac myocytes. Annu. Rev. Physiol. 70, 23–49. doi:10.1146/annurev.physiol.70.113006.100455
Bers, D. M., and Guo, T. (2005). Calcium signaling in cardiac ventricular myocytes. Ann. N. Y. Acad. Sci. 1047, 86–98. doi:10.1196/annals.1341.008
Bidaux, G., Borowiec, A. S., Dubois, C., Delcourt, P., Schulz, C., Vanden Abeele, F., et al. (2016). Targeting of short TRPM8 isoforms induces 4TM-TRPM8-dependent apoptosis in prostate cancer cells. Oncotarget 7, 29063–29080. doi:10.18632/oncotarget.8666
Bidaux, G., Flourakis, M., Thebault, S., Zholos, A., Beck, B., Gkika, D., et al. (2007). Prostate cell differentiation status determines transient receptor potential melastatin member 8 channel subcellular localization and function. J. Clin. Invest. 117, 1647–1657. doi:10.1172/JCI30168
Bidaux, G., Gordienko, D., Shapovalov, G., Farfariello, V., Borowiec, A. S., Iamshanova, O., et al. (2018). 4TM-TRPM8 channels are new gatekeepers of the ER-mitochondria Ca(2+) transfer. Biochim. Biophys. Acta Mol. Cell Res. 1865, 981–994. doi:10.1016/j.bbamcr.2018.04.007
Bidaux, G., Roudbaraki, M., Merle, C., Crepin, A., Delcourt, P., Slomianny, C., et al. (2005). Evidence for specific TRPM8 expression in human prostate secretory epithelial cells: Functional androgen receptor requirement. Endocr. Relat. Cancer 12, 367–382. doi:10.1677/erc.1.00969
Boettner, B., and Van Aelst, L. (2009). Control of cell adhesion dynamics by Rap1 signaling. Curr. Opin. Cell Biol. 21, 684–693. doi:10.1016/j.ceb.2009.06.004
Bonora, M., Giorgi, C., and Pinton, P. (2022). Molecular mechanisms and consequences of mitochondrial permeability transition. Nat. Rev. Mol. Cell Biol. 23, 266–285. doi:10.1038/s41580-021-00433-y
Bootman, M. D., Berridge, M. J., and Lipp, P. (1997). Cooking with calcium: The recipes for composing global signals from elementary events. Cell 91, 367–373. doi:10.1016/s0092-8674(00)80420-1
Borowiec, A. S., Bidaux, G., Tacine, R., Dubar, P., Pigat, N., Delcourt, P., et al. (2014). Are Orai1 and Orai3 channels more important than calcium influx for cell proliferation? Biochim. Biophys. Acta 1843, 464–472. doi:10.1016/j.bbamcr.2013.11.023
Bossio, C., Abdel Aziz, I., Tullii, G., Zucchetti, E., Debellis, D., Zangoli, M., et al. (2018). Photocatalytic activity of polymer nanoparticles modulates intracellular calcium dynamics and reactive oxygen species in HEK-293 cells. Front. Bioeng. Biotechnol. 6, 114. doi:10.3389/fbioe.2018.00114
Brailoiu, G. C., Brailoiu, E., Parkesh, R., Galione, A., Churchill, G. C., Patel, S., et al. (2009). NAADP-mediated channel 'chatter' in neurons of the rat medulla oblongata. Biochem. J. 419 (91-97), 91–97. 92 p following 97. doi:10.1042/BJ20081138
Cai, N., Lou, L., Al-Saadi, N., Tetteh, S., and Runnels, L. W. (2018). The kinase activity of the channel-kinase protein TRPM7 regulates stability and localization of the TRPM7 channel in polarized epithelial cells. J. Biol. Chem. 293, 11491–11504. doi:10.1074/jbc.RA118.001925
Carmona, G., Gottig, S., Orlandi, A., Scheele, J., Bauerle, T., Jugold, M., et al. (2009). Role of the small GTPase Rap1 for integrin activity regulation in endothelial cells and angiogenesis. Blood 113, 488–497. doi:10.1182/blood-2008-02-138438
Caterina, M. J., Schumacher, M. A., Tominaga, M., Rosen, T. A., Levine, J. D., and Julius, D. (1997). The capsaicin receptor: A heat-activated ion channel in the pain pathway. Nature 389, 816–824. doi:10.1038/39807
Cherfils, J., and Zeghouf, M. (2013). Regulation of small GTPases by GEFs, GAPs, and GDIs. Physiol. Rev. 93, 269–309. doi:10.1152/physrev.00003.2012
Chinigo, G., Fiorio Pla, A., and Gkika, D. (2020). TRP channels and small GTPases interplay in the main hallmarks of metastatic cancer. Front. Pharmacol. 11, 581455. doi:10.3389/fphar.2020.581455
Chinigo, G., Grolez, G. P., Audero, M., Bokhobza, A., Bernardini, M., Cicero, J., et al. (2022). TRPM8-Rap1A interaction sites as critical determinants for adhesion and migration of prostate and other epithelial cancer cells. Cancers (Basel) 14, 2261. doi:10.3390/cancers14092261
Chou, C. L., Yip, K. P., Michea, L., Kador, K., Ferraris, J. D., Wade, J. B., et al. (2000). Regulation of aquaporin-2 trafficking by vasopressin in the renal collecting duct. Roles of ryanodine-sensitive Ca2+ stores and calmodulin. J. Biol. Chem. 275, 36839–36846. doi:10.1074/jbc.M005552200
Christensen, K. A., Myers, J. T., and Swanson, J. A. (2002). pH-dependent regulation of lysosomal calcium in macrophages. J. Cell Sci. 115, 599–607. doi:10.1242/jcs.115.3.599
Chrzanowska-Wodnicka, M., Kraus, A. E., Gale, D., White, G. C., 2nd, , and Vansluys, J. (2008). Defective angiogenesis, endothelial migration, proliferation, and MAPK signaling in Rap1b-deficient mice. Blood 111, 2647–2656. doi:10.1182/blood-2007-08-109710
Cieri, D., Vicario, M., Giacomello, M., Vallese, F., Filadi, R., Wagner, T., et al. (2018). Splics: A split green fluorescent protein-based contact site sensor for narrow and wide heterotypic organelle juxtaposition. Cell Death Differ. 25, 1131–1145. doi:10.1038/s41418-017-0033-z
Crick, F. (1999). The impact of molecular biology on neuroscience. Philos. Trans. R. Soc. Lond B Biol. Sci. 354, 2021–2025. doi:10.1098/rstb.1999.0541
Csordas, G., Varnai, P., Golenar, T., Roy, S., Purkins, G., Schneider, T. G., et al. (2010). Imaging interorganelle contacts and local calcium dynamics at the ER-mitochondrial interface. Mol. Cell 39, 121–132. doi:10.1016/j.molcel.2010.06.029
Csordas, G., Weaver, D., and Hajnoczky, G. (2018). Endoplasmic reticulum-mitochondrial contactology: Structure and signaling functions. Trends Cell Biol. 28, 523–540. doi:10.1016/j.tcb.2018.02.009
Curcic, S., Schober, R., Schindl, R., and Groschner, K. (2019). TRPC-mediated Ca(2+) signaling and control of cellular functions. Semin. Cell Dev. Biol. 94, 28–39. doi:10.1016/j.semcdb.2019.02.001
De Strooper, B., and Karran, E. (2016). The cellular phase of alzheimer's disease. Cell 164, 603–615. doi:10.1016/j.cell.2015.12.056
Dematteis, G., Vydmantaite, G., Ruffinatti, F. A., Chahin, M., Farruggio, S., Barberis, E., et al. (2020). Proteomic analysis links alterations of bioenergetics, mitochondria-ER interactions and proteostasis in hippocampal astrocytes from 3xTg-AD mice. Cell Death Dis. 11, 645. doi:10.1038/s41419-020-02911-1
Desai, B. N., Krapivinsky, G., Navarro, B., Krapivinsky, L., Carter, B. C., Febvay, S., et al. (2012). Cleavage of TRPM7 releases the kinase domain from the ion channel and regulates its participation in Fas-induced apoptosis. Dev. Cell 22, 1149–1162. doi:10.1016/j.devcel.2012.04.006
Descazeaud, V., Mestre, E., Marquet, P., and Essig, M. (2012). Calcineurin regulation of cytoskeleton organization: A new paradigm to analyse the effects of calcineurin inhibitors on the kidney. J. Cell Mol. Med. 16, 218–227. doi:10.1111/j.1582-4934.2011.01398.x
Dhaka, A., Earley, T. J., Watson, J., and Patapoutian, A. (2008). Visualizing cold spots: TRPM8-expressing sensory neurons and their projections. J. Neurosci. 28, 566–575. doi:10.1523/JNEUROSCI.3976-07.2008
Di Maria, F., Lodola, F., Zucchetti, E., Benfenati, F., and Lanzani, G. (2018). The evolution of artificial light actuators in living systems: From planar to nanostructured interfaces. Chem. Soc. Rev. 47, 4757–4780. doi:10.1039/c7cs00860k
Di Paola, S., Scotto-Rosato, A., and Medina, D. L. (2018). TRPML1: The Ca((2+))retaker of the lysosome. Cell Calcium 69, 112–121. doi:10.1016/j.ceca.2017.06.006
Difrancesco, M. L., Lodola, F., Colombo, E., Maragliano, L., Bramini, M., Paterno, G. M., et al. (2020). Neuronal firing modulation by a membrane-targeted photoswitch. Nat. Nanotechnol. 15, 296–306. doi:10.1038/s41565-019-0632-6
Diver, M. M., Lin King, J. V., Julius, D., and Cheng, Y. (2022). Sensory TRP channels in three dimensions. Annu. Rev. Biochem. 91, 629–649. doi:10.1146/annurev-biochem-032620-105738
Dragoni, S., Laforenza, U., Bonetti, E., Lodola, F., Bottino, C., Berra-Romani, R., et al. (2011). Vascular endothelial growth factor stimulates endothelial colony forming cells proliferation and tubulogenesis by inducing oscillations in intracellular Ca2+ concentration. Stem Cells 29, 1898–1907. doi:10.1002/stem.734
Dragoni, S., Reforgiato, M., Zuccolo, E., Poletto, V., Lodola, F., Ruffinatti, F. A., et al. (2015). Dysregulation of VEGF-induced proangiogenic Ca2+ oscillations in primary myelofibrosis-derived endothelial colony-forming cells. Exp. Hematol. 43, 1019–1030.e3. doi:10.1016/j.exphem.2015.09.002
Eisner, D. A., Caldwell, J. L., Kistamas, K., and Trafford, A. W. (2017). Calcium and excitation-contraction coupling in the heart. Circ. Res. 121, 181–195. doi:10.1161/CIRCRESAHA.117.310230
Ezra-Nevo, G., Prestori, F., Locatelli, F., Soda, T., Ten Brinke, M. M., Engel, M., et al. (2018). Cerebellar learning properties are modulated by the CRF receptor. J. Neurosci. 38, 6751–6765. doi:10.1523/JNEUROSCI.3106-15.2018
Faouzi, M., Kilch, T., Horgen, F. D., Fleig, A., and Penner, R. (2017). The TRPM7 channel kinase regulates store-operated calcium entry. J. Physiol. 595, 3165–3180. doi:10.1113/JP274006
Faris, P., Casali, C., Negri, S., Iengo, L., Biggiogera, M., Maione, A. S., et al. (2022). Nicotinic acid adenine dinucleotide phosphate induces intracellular Ca(2+) signalling and stimulates proliferation in human cardiac mesenchymal stromal cells. Front. Cell Dev. Biol. 10, 874043. doi:10.3389/fcell.2022.874043
Faris, P., Pellavio, G., Ferulli, F., Di Nezza, F., Shekha, M., Lim, D., et al. (2019). Nicotinic acid adenine dinucleotide phosphate (NAADP) induces intracellular Ca(2+) release through the two-pore channel TPC1 in metastatic colorectal cancer cells. Cancers (Basel) 11, 542. doi:10.3390/cancers11040542
Faris, P., Rumolo, A., Pellavio, G., Tanzi, M., Vismara, M., Berra-Romani, R., et al. (2023). Transient receptor potential ankyrin 1 (TRPA1) mediates reactive oxygen species-induced Ca(2+) entry, mitochondrial dysfunction, and caspase-3/7 activation in primary cultures of metastatic colorectal carcinoma cells. Cell Death Discov. 9, 213. doi:10.1038/s41420-023-01530-x
Faris, P., Shekha, M., Montagna, D., Guerra, G., and Moccia, F. (2018). Endolysosomal Ca(2+) signalling and cancer hallmarks: Two-pore channels on the move, TRPML1 lags behind. Cancers (Basel) 11, 27. doi:10.3390/cancers11010027
Fernandez, J. A., Skryma, R., Bidaux, G., Magleby, K. L., Scholfield, C. N., Mcgeown, J. G., et al. (2012). Short isoforms of the cold receptor TRPM8 inhibit channel gating by mimicking heat action rather than chemical inhibitors. J. Biol. Chem. 287, 2963–2970. doi:10.1074/jbc.M111.272823
Feyen, P., Colombo, E., Endeman, D., Nova, M., Laudato, L., Martino, N., et al. (2016). Light-evoked hyperpolarization and silencing of neurons by conjugated polymers. Sci. Rep. 6, 22718. doi:10.1038/srep22718
Fiorio Pla, A., Ong, H. L., Cheng, K. T., Brossa, A., Bussolati, B., Lockwich, T., et al. (2012). TRPV4 mediates tumor-derived endothelial cell migration via arachidonic acid-activated actin remodeling. Oncogene 31, 200–212. doi:10.1038/onc.2011.231
Foster, W. J., Taylor, H. B. C., Padamsey, Z., Jeans, A. F., Galione, A., and Emptage, N. J. (2018). Hippocampal mGluR1-dependent long-term potentiation requires NAADP-mediated acidic store Ca(2+) signaling. Sci. Signal 11, eaat9093. doi:10.1126/scisignal.aat9093
Fujiwara, Y., and Minor, D. L. (2008). X-ray crystal structure of a TRPM assembly domain reveals an antiparallel four-stranded coiled-coil. J. Mol. Biol. 383, 854–870. doi:10.1016/j.jmb.2008.08.059
Galione, A., Davis, L. C., Martucci, L. L., and Morgan, A. J. (2023). NAADP-mediated Ca(2+) signalling. Handb. Exp. Pharmacol. 278, 3–34. doi:10.1007/164_2022_607
Galione, A. (2019). NAADP receptors. Cold Spring Harb. Perspect. Biol. 11, a035071. doi:10.1101/cshperspect.a035071
Gan, N., Han, Y., Zeng, W., Wang, Y., Xue, J., and Jiang, Y. (2022). Structural mechanism of allosteric activation of TRPML1 by PI(3,5)P(2) and rapamycin. Proc. Natl. Acad. Sci. U. S. A. 119, e2120404119. doi:10.1073/pnas.2120404119
Genova, T., Grolez, G. P., Camillo, C., Bernardini, M., Bokhobza, A., Richard, E., et al. (2017). TRPM8 inhibits endothelial cell migration via a non-channel function by trapping the small GTPase Rap1. J. Cell Biol. 216, 2107–2130. doi:10.1083/jcb.201506024
Genovesi, S., Moro, R., Vignoli, B., De Felice, D., Canossa, M., Montironi, R., et al. (2022). Trpm8 expression in human and mouse castration resistant prostate adenocarcinoma paves the way for the preclinical development of TRPM8-based targeted therapies. Biomolecules 12, 193. doi:10.3390/biom12020193
Gerasimenko, J. V., Tepikin, A. V., Petersen, O. H., and Gerasimenko, O. V. (1998). Calcium uptake via endocytosis with rapid release from acidifying endosomes. Curr. Biol. 8, 1335–1338. doi:10.1016/s0960-9822(07)00565-9
Gerndt, S., Chen, C. C., Chao, Y. K., Yuan, Y., Burgstaller, S., Scotto Rosato, A., et al. (2020). Agonist-mediated switching of ion selectivity in TPC2 differentially promotes lysosomal function. Elife 9, e54712. doi:10.7554/eLife.54712
Gherardi, G., Monticelli, H., Rizzuto, R., and Mammucari, C. (2020). The mitochondrial Ca(2+) uptake and the fine-tuning of aerobic metabolism. Front. Physiol. 11, 554904. doi:10.3389/fphys.2020.554904
Ghezzi, D., Antognazza, M. R., Dal Maschio, M., Lanzarini, E., Benfenati, F., and Lanzani, G. (2011). A hybrid bioorganic interface for neuronal photoactivation. Nat. Commun. 2, 166. doi:10.1038/ncomms1164
Ghezzi, D., Antognazza, M. R., Maccarone, R., Bellani, S., Lanzarini, E., Martino, N., et al. (2013). A polymer optoelectronic interface restores light sensitivity in blind rat retinas. Nat. Photonics 7, 400–406. doi:10.1038/nphoton.2013.34
Gomez-Ospina, N., Tsuruta, F., Barreto-Chang, O., Hu, L., and Dolmetsch, R. (2006). The C terminus of the L-type voltage-gated calcium channel Ca(V)1.2 encodes a transcription factor. Cell 127, 591–606. doi:10.1016/j.cell.2006.10.017
Gong, C., Bonfili, L., Zheng, Y., Cecarini, V., Cuccioloni, M., Angeletti, M., et al. (2023). Immortalized alzheimer's disease astrocytes: Characterization of their proteolytic systems. Mol. Neurobiol. 60, 2787–2800. doi:10.1007/s12035-023-03231-z
Gorostiza, P., and Isacoff, E. Y. (2008). Optical switches for remote and noninvasive control of cell signaling. Science 322, 395–399. doi:10.1126/science.1166022
Grolez, G. P., Chinigo, G., Barras, A., Hammadi, M., Noyer, L., Kondratska, K., et al. (2022). TRPM8 as an anti-tumoral target in prostate cancer growth and metastasis dissemination. Int. J. Mol. Sci. 23, 6672. doi:10.3390/ijms23126672
Grolez, G. P., Gordiendko, D. V., Clarisse, M., Hammadi, M., Desruelles, E., Fromont, G., et al. (2019). TRPM8-androgen receptor association within lipid rafts promotes prostate cancer cell migration. Cell Death Dis. 10, 652. doi:10.1038/s41419-019-1891-8
Grolla, A. A., Fakhfouri, G., Balzaretti, G., Marcello, E., Gardoni, F., Canonico, P. L., et al. (2013a). Aβ leads to Ca²⁺ signaling alterations and transcriptional changes in glial cells. Neurobiol. Aging 34, 511–522. doi:10.1016/j.neurobiolaging.2012.05.005
Grolla, A. A., Sim, J. A., Lim, D., Rodriguez, J. J., Genazzani, A. A., and Verkhratsky, A. (2013b). Amyloid-beta and Alzheimer's disease type pathology differentially affects the calcium signalling toolkit in astrocytes from different brain regions. Cell Death Dis. 4, e623. doi:10.1038/cddis.2013.145
Guarini, G., Ohanyan, V. A., Kmetz, J. G., Dellostritto, D. J., Thoppil, R. J., Thodeti, C. K., et al. (2012). Disruption of TRPV1-mediated coupling of coronary blood flow to cardiac metabolism in diabetic mice: Role of nitric oxide and BK channels. Am. J. Physiol. Heart Circ. Physiol. 303, H216–H223. doi:10.1152/ajpheart.00011.2012
He, L., Wang, L., Zeng, H., Tan, P., Ma, G., Zheng, S., et al. (2021). Engineering of a bona fide light-operated calcium channel. Nat. Commun. 12, 164. doi:10.1038/s41467-020-20425-4
Herrera-Cruz, M. S., and Simmen, T. (2017). Over six decades of discovery and characterization of the architecture at mitochondria-associated membranes (MAMs). Adv. Exp. Med. Biol. 997, 13–31. doi:10.1007/978-981-10-4567-7_2
Hibino, H., Pironkova, R., Onwumere, O., Rousset, M., Charnet, P., Hudspeth, A. J., et al. (2003). Direct interaction with a nuclear protein and regulation of gene silencing by a variant of the Ca2+-channel beta 4 subunit. Proc. Natl. Acad. Sci. U. S. A. 100, 307–312. doi:10.1073/pnas.0136791100
Hirano, T. (2013). Long-term depression and other synaptic plasticity in the cerebellum. Proc. Jpn. Acad. Ser. B Phys. Biol. Sci. 89, 183–195. doi:10.2183/pjab.89.183
Hopkins, J., Travaglini, R., Lauto, A., Cramer, T., Fraboni, B., Seidel, J., et al. (2019). Photoactive organic substrates for cell stimulation: Progress and perspectives. Adv. Mat. Technol. 4, 1800744. doi:10.1002/admt.201800744
Izquierdo-Serra, M., Bautista-Barrufet, A., Trapero, A., Garrido-Charles, A., Diaz-Tahoces, A., Camarero, N., et al. (2016). Optical control of endogenous receptors and cellular excitability using targeted covalent photoswitches. Nat. Commun. 7, 12221. doi:10.1038/ncomms12221
Jordt, S. E., Tominaga, M., and Julius, D. (2000). Acid potentiation of the capsaicin receptor determined by a key extracellular site. Proc. Natl. Acad. Sci. U. S. A. 97, 8134–8139. doi:10.1073/pnas.100129497
Katona, M., Bartok, A., Nichtova, Z., Csordas, G., Berezhnaya, E., Weaver, D., et al. (2022). Capture at the ER-mitochondrial contacts licenses IP(3) receptors to stimulate local Ca(2+) transfer and oxidative metabolism. Nat. Commun. 13, 6779. doi:10.1038/s41467-022-34365-8
Keebler, M. V., and Taylor, C. W. (2017). Endogenous signalling pathways and caged IP3 evoke Ca(2+) puffs at the same abundant immobile intracellular sites. J. Cell Sci. 130, 3728–3739. doi:10.1242/jcs.208520
Kilpatrick, B. S., Eden, E. R., Hockey, L. N., Yates, E., Futter, C. E., and Patel, S. (2017). An endosomal NAADP-sensitive two-pore Ca(2+) channel regulates ER-endosome membrane contact sites to control growth factor signaling. Cell Rep. 18, 1636–1645. doi:10.1016/j.celrep.2017.01.052
Kilpatrick, B. S., Eden, E. R., Schapira, A. H., Futter, C. E., and Patel, S. (2013). Direct mobilisation of lysosomal Ca2+ triggers complex Ca2+ signals. J. Cell Sci. 126, 60–66. doi:10.1242/jcs.118836
Kilpatrick, B. S., Yates, E., Grimm, C., Schapira, A. H., and Patel, S. (2016). Endo-lysosomal TRP mucolipin-1 channels trigger global ER Ca2+ release and Ca2+ influx. J. Cell Sci. 129, 3859–3867. doi:10.1242/jcs.190322
Kiselyov, K., Yamaguchi, S., Lyons, C. W., and Muallem, S. (2010). Aberrant Ca2+ handling in lysosomal storage disorders. Cell Calcium 47, 103–111. doi:10.1016/j.ceca.2009.12.007
Klasen, K., Hollatz, D., Zielke, S., Gisselmann, G., Hatt, H., and Wetzel, C. H. (2012). The TRPM8 ion channel comprises direct Gq protein-activating capacity. Pflugers Arch. 463, 779–797. doi:10.1007/s00424-012-1098-7
Knepper, M. A., Kwon, T. H., and Nielsen, S. (2015). Molecular physiology of water balance. N. Engl. J. Med. 372, 1349–1358. doi:10.1056/NEJMra1404726
Knot, H. J., and Nelson, M. T. (1998). Regulation of arterial diameter and wall [Ca2+] in cerebral arteries of rat by membrane potential and intravascular pressure. J. Physiol. 508 (Pt 1), 199–209. doi:10.1111/j.1469-7793.1998.199br.x
Knowlton, W. M., Palkar, R., Lippoldt, E. K., Mccoy, D. D., Baluch, F., Chen, J., et al. (2013). A sensory-labeled line for cold: TRPM8-expressing sensory neurons define the cellular basis for cold, cold pain, and cooling-mediated analgesia. J. Neurosci. 33, 2837–2848. doi:10.1523/JNEUROSCI.1943-12.2013
Kordasiewicz, H. B., Thompson, R. M., Clark, H. B., and Gomez, C. M. (2006). C-termini of P/Q-type Ca2+ channel alpha1A subunits translocate to nuclei and promote polyglutamine-mediated toxicity. Hum. Mol. Genet. 15, 1587–1599. doi:10.1093/hmg/ddl080
Kraft, R., and Harteneck, C. (2005). The mammalian melastatin-related transient receptor potential cation channels: An overview. Pflugers Arch. 451, 204–211. doi:10.1007/s00424-005-1428-0
Landstrom, A. P., Dobrev, D., and Wehrens, X. H. T. (2017). Calcium signaling and cardiac arrhythmias. Circ. Res. 120, 1969–1993. doi:10.1161/CIRCRESAHA.117.310083
Lazzarini, E., Lodrini, A. M., Arici, M., Bolis, S., Vagni, S., Panella, S., et al. (2022). Stress-induced premature senescence is associated with a prolonged QT interval and recapitulates features of cardiac aging. Theranostics 12, 5237–5257. doi:10.7150/thno.70884
Leal, N. S., and Martins, L. M. (2021). Mind the gap: Mitochondria and the endoplasmic reticulum in neurodegenerative diseases. Biomedicines 9, 227. doi:10.3390/biomedicines9020227
Leippe, P., Koehler Leman, J., and Trauner, D. (2017). Specificity and speed: Tethered photopharmacology. Biochemistry 56, 5214–5220. doi:10.1021/acs.biochem.7b00687
Leoni, L., Tonelli, F., Besio, R., Gioia, R., Moccia, F., Rossi, A., et al. (2021). Knocking out TMEM38B in human foetal osteoblasts hFOB 1.19 by CRISPR/cas9: A model for recessive OI type xiv. PLoS One 16, e0257254. doi:10.1371/journal.pone.0257254
Lewis, R. S. (2020). Store-operated calcium channels: From function to structure and back again. Cold Spring Harb. Perspect. Biol. 12, a035055. doi:10.1101/cshperspect.a035055
Lia, A., Sansevero, G., Chiavegato, A., Sbrissa, M., Pendin, D., Mariotti, L., et al. (2023). Rescue of astrocyte activity by the calcium sensor STIM1 restores long-term synaptic plasticity in female mice modelling Alzheimer's disease. Nat. Commun. 14, 1590. doi:10.1038/s41467-023-37240-2
Lim, D., Bertoli, A., Sorgato, M. C., and Moccia, F. (2016a). Generation and usage of aequorin lentiviral vectors for Ca(2+) measurement in sub-cellular compartments of hard-to-transfect cells. Cell Calcium 59, 228–239. doi:10.1016/j.ceca.2016.03.001
Lim, D., Dematteis, G., Tapella, L., Genazzani, A. A., Cali, T., Brini, M., et al. (2021a). Ca(2+) handling at the mitochondria-ER contact sites in neurodegeneration. Cell Calcium 98, 102453. doi:10.1016/j.ceca.2021.102453
Lim, D., Ercolano, E., Kyozuka, K., Nusco, G. A., Moccia, F., Lange, K., et al. (2003). The M-phase-promoting factor modulates the sensitivity of the Ca2+ stores to inositol 1,4,5-trisphosphate via the actin cytoskeleton. J. Biol. Chem. 278, 42505–42514. doi:10.1074/jbc.M301851200
Lim, D., Iyer, A., Ronco, V., Grolla, A. A., Canonico, P. L., Aronica, E., et al. (2013). Amyloid beta deregulates astroglial mGluR5-mediated calcium signaling via calcineurin and Nf-kB. Glia 61, 1134–1145. doi:10.1002/glia.22502
Lim, D., Rodriguez-Arellano, J. J., Parpura, V., Zorec, R., Zeidan-Chulia, F., Genazzani, A. A., et al. (2016b). Calcium signalling toolkits in astrocytes and spatio-temporal progression of Alzheimer's disease. Curr. Alzheimer Res. 13, 359–369. doi:10.2174/1567205013666151116130104
Lim, D., Ronco, V., Grolla, A. A., Verkhratsky, A., and Genazzani, A. A. (2014). Glial calcium signalling in Alzheimer's disease. Rev. Physiol. Biochem. Pharmacol. 167, 45–65. doi:10.1007/112_2014_19
Lim, D., Semyanov, A., Genazzani, A., and Verkhratsky, A. (2021b). Calcium signaling in neuroglia. Int. Rev. Cell Mol. Biol. 362, 1–53. doi:10.1016/bs.ircmb.2021.01.003
Lim, D., Tapella, L., Dematteis, G., Genazzani, A. A., Corazzari, M., and Verkhratsky, A. (2023). The endoplasmic reticulum stress and unfolded protein response in alzheimer's disease: A calcium dyshomeostasis perspective. Ageing Res. Rev. 87, 101914. doi:10.1016/j.arr.2023.101914
Lloyd-Evans, E., Waller-Evans, H., Peterneva, K., and Platt, F. M. (2010). Endolysosomal calcium regulation and disease. Biochem. Soc. Trans. 38, 1458–1464. doi:10.1042/BST0381458
Locatelli, F., Soda, T., Montagna, I., Tritto, S., Botta, L., Prestori, F., et al. (2021). Calcium Channel-dependent induction of long-term synaptic plasticity at excitatory golgi cell synapses of cerebellum. J. Neurosci. 41, 3307–3319. doi:10.1523/JNEUROSCI.3013-19.2020
Lodola, F., Laforenza, U., Cattaneo, F., Ruffinatti, F. A., Poletto, V., Massa, M., et al. (2017a). VEGF-induced intracellular Ca2+ oscillations are down-regulated and do not stimulate angiogenesis in breast cancer-derived endothelial colony forming cells. Oncotarget 8, 95223–95246. doi:10.18632/oncotarget.20255
Lodola, F., Martino, N., Tullii, G., Lanzani, G., and Antognazza, M. R. (2017b). Conjugated polymers mediate effective activation of the mammalian ion channel Transient receptor potential vanilloid 1. Sci. Rep. 7, 8477. doi:10.1038/s41598-017-08541-6
Lodola, F., and Moccia, F. (2022). Fiat lux - light at the service of cardiac physiology. pH 2, 39–46.
Lodola, F., Rosti, V., Tullii, G., Desii, A., Tapella, L., Catarsi, P., et al. (2019a). Conjugated polymers optically regulate the fate of endothelial colony-forming cells. Sci. Adv. 5, eaav4620. doi:10.1126/sciadv.aav4620
Lodola, F., Vurro, V., Crasto, S., Di Pasquale, E., and Lanzani, G. (2019b). Optical pacing of human-induced pluripotent stem cell-derived cardiomyocytes mediated by a conjugated polymer interface. Adv. Healthc. Mater 8, e1900198. doi:10.1002/adhm.201900198
Loncke, J., Kaasik, A., Bezprozvanny, I., Parys, J. B., Kerkhofs, M., and Bultynck, G. (2021). Balancing ER-mitochondrial Ca(2+) fluxes in health and disease. Trends Cell Biol. 31, 598–612. doi:10.1016/j.tcb.2021.02.003
Longden, T. A., Mughal, A., Hennig, G. W., Harraz, O. F., Shui, B., Lee, F. K., et al. (2021). Local IP3 receptor-mediated Ca(2+) signals compound to direct blood flow in brain capillaries. Sci. Adv. 7, eabh0101. doi:10.1126/sciadv.abh0101
Mabonga, L., and Kappo, A. P. (2019). Protein-protein interaction modulators: Advances, successes and remaining challenges. Biophys. Rev. 11, 559–581. doi:10.1007/s12551-019-00570-x
Macgregor, A., Yamasaki, M., Rakovic, S., Sanders, L., Parkesh, R., Churchill, G. C., et al. (2007). NAADP controls cross-talk between distinct Ca2+ stores in the heart. J. Biol. Chem. 282, 15302–15311. doi:10.1074/jbc.M611167200
Madrid, R., Donovan-Rodriguez, T., Meseguer, V., Acosta, M. C., Belmonte, C., and Viana, F. (2006). Contribution of TRPM8 channels to cold transduction in primary sensory neurons and peripheral nerve terminals. J. Neurosci. 26, 12512–12525. doi:10.1523/JNEUROSCI.3752-06.2006
Magni, A., Bondelli, G., Paterno, G. M., Sardar, S., Sesti, V., D'andrea, C., et al. (2022). Azobenzene photoisomerization probes cell membrane viscosity. Phys. Chem. Chem. Phys. 24, 8716–8723. doi:10.1039/d1cp05881a
Maione, A. S., Faris, P., Iengo, L., Catto, V., Bisonni, L., Lodola, F., et al. (2022). Ca(2+) dysregulation in cardiac stromal cells sustains fibro-adipose remodeling in Arrhythmogenic Cardiomyopathy and can be modulated by flecainide. J. Transl. Med. 20, 522. doi:10.1186/s12967-022-03742-8
Mamenko, M., Dhande, I., Tomilin, V., Zaika, O., Boukelmoune, N., Zhu, Y., et al. (2016). Defective store-operated calcium entry causes partial nephrogenic diabetes insipidus. J. Am. Soc. Nephrol. 27, 2035–2048. doi:10.1681/ASN.2014121200
Marta, K., Booth, D., Csordas, G., and Hajnoczky, G. (2022). Fluorescent protein transgenic mice for the study of Ca(2+) and redox signaling. Free Radic. Biol. Med. 181, 241–250. doi:10.1016/j.freeradbiomed.2022.02.011
Martinotti, S., Laforenza, U., Patrone, M., Moccia, F., and Ranzato, E. (2019). Honey-mediated wound healing: H₂O₂ entry through AQP3 determines extracellular Ca2+ influx. Int. J. Mol. Sci. 20, 764. doi:10.3390/ijms20030764
Maya-Vetencourt, J. F., Ghezzi, D., Antognazza, M. R., Colombo, E., Mete, M., Feyen, P., et al. (2017). A fully organic retinal prosthesis restores vision in a rat model of degenerative blindness. Nat. Mater 16, 681–689. doi:10.1038/nmat4874
Maya-Vetencourt, J. F., Manfredi, G., Mete, M., Colombo, E., Bramini, M., Di Marco, S., et al. (2020). Subretinally injected semiconducting polymer nanoparticles rescue vision in a rat model of retinal dystrophy. Nat. Nanotechnol. 15, 698–708. doi:10.1038/s41565-020-0696-3
Mckemy, D. D., Neuhausser, W. M., and Julius, D. (2002). Identification of a cold receptor reveals a general role for TRP channels in thermosensation. Nature 416, 52–58. doi:10.1038/nature719
Medina, D. L., Di Paola, S., Peluso, I., Armani, A., De Stefani, D., Venditti, R., et al. (2015). Lysosomal calcium signalling regulates autophagy through calcineurin and TFEB. Nat. Cell Biol. 17, 288–299. doi:10.1038/ncb3114
Medina, D. L. (2021). Lysosomal calcium and autophagy. Int. Rev. Cell Mol. Biol. 362, 141–170. doi:10.1016/bs.ircmb.2021.03.002
Melchionda, M., Pittman, J. K., Mayor, R., and Patel, S. (2016). Ca2+/H+ exchange by acidic organelles regulates cell migration in vivo. J. Cell Biol. 212, 803–813. doi:10.1083/jcb.201510019
Menigoz, A., Ahmed, T., Sabanov, V., Philippaert, K., Pinto, S., Kerselaers, S., et al. (2016). TRPM4-dependent post-synaptic depolarization is essential for the induction of NMDA receptor-dependent LTP in CA1 hippocampal neurons. Pflugers Arch. 468, 593–607. doi:10.1007/s00424-015-1764-7
Merlo, S., Spampinato, S. F., and Lim, D. (2021). Molecular aspects of cellular dysfunction in alzheimer's disease: The need for a holistic view of the early pathogenesis. Biomolecules 11, 1807. doi:10.3390/biom11121807
Michelucci, A., Pietrangelo, L., Rastelli, G., Protasi, F., Dirksen, R. T., and Boncompagni, S. (2022). Constitutive assembly of Ca2+ entry units in soleus muscle from calsequestrin knockout mice. J. Gen. Physiol. 154, e202213114. doi:10.1085/jgp.202213114
Millar, F. R., Janes, S. M., and Giangreco, A. (2017). Epithelial cell migration as a potential therapeutic target in early lung cancer. Eur. Respir. Rev. 26, 160069. doi:10.1183/16000617.0069-2016
Moccia, F., Antognazza, M. R., and Lodola, F. (2020). Towards novel geneless approaches for therapeutic angiogenesis. Front. Physiol. 11, 616189. doi:10.3389/fphys.2020.616189
Moccia, F., Berra-Romani, R., Baruffi, S., Spaggiari, S., Signorelli, S., Castelli, L., et al. (2002). Ca2+ uptake by the endoplasmic reticulum Ca2+-ATPase in rat microvascular endothelial cells. Biochem. J. 364, 235–244. doi:10.1042/bj3640235
Moccia, F., Bonetti, E., Dragoni, S., Fontana, J., Lodola, F., Romani, R. B., et al. (2012). Hematopoietic progenitor and stem cells circulate by surfing on intracellular Ca2+ waves: A novel target for cell-based therapy and anti-cancer treatment? Curr. Signal Transd T 7, 161–176. doi:10.2174/157436212800376672
Moccia, F., Brunetti, V., Perna, A., Guerra, G., Soda, T., and Berra-Romani, R. (2023). The molecular heterogeneity of store-operated Ca(2+) entry in vascular endothelial cells: The different roles of Orai1 and TRPC1/TRPC4 channels in the transition from Ca(2+)-selective to non-selective cation currents. Int. J. Mol. Sci. 24, 3259. doi:10.3390/ijms24043259
Moccia, F., Dragoni, S., Cinelli, M., Montagnani, S., Amato, B., Rosti, V., et al. (2013). How to utilize Ca2+ signals to rejuvenate the repairative phenotype of senescent endothelial progenitor cells in elderly patients affected by cardiovascular diseases: A useful therapeutic support of surgical approach? BMC Surg. 13 (Suppl. 2), S46. doi:10.1186/1471-2482-13-S2-S46
Moccia, F. (2018). Endothelial Ca(2+) signaling and the resistance to anticancer treatments: Partners in crime. Int. J. Mol. Sci. 19, 217. doi:10.3390/ijms19010217
Moccia, F., Lodola, F., Stadiotti, I., Pilato, C. A., Bellin, M., Carugo, S., et al. (2019a). Calcium as a key player in arrhythmogenic cardiomyopathy: Adhesion disorder or intracellular alteration? Int. J. Mol. Sci. 20, 3986. doi:10.3390/ijms20163986
Moccia, F., Lucariello, A., and Guerra, G. (2018). TRPC3-mediated Ca(2+) signals as a promising strategy to boost therapeutic angiogenesis in failing hearts: The role of autologous endothelial colony forming cells. J. Cell Physiol. 233, 3901–3917. doi:10.1002/jcp.26152
Moccia, F., Negri, S., Faris, P., Perna, A., De Luca, A., Soda, T., et al. (2021a). Targeting endolysosomal two-pore channels to treat cardiovascular disorders in the novel COronaVIrus disease 2019. Front. Physiol. 12, 629119. doi:10.3389/fphys.2021.629119
Moccia, F., Negri, S., Faris, P., Ronchi, C., and Lodola, F. (2022). Optical excitation of organic semiconductors as a highly selective strategy to induce vascular regeneration and tissue repair. Vasc. Pharmacol. 144, 106998. doi:10.1016/j.vph.2022.106998
Moccia, F., Negri, S., Shekha, M., Faris, P., and Guerra, G. (2019b). Endothelial Ca(2+) signaling, angiogenesis and vasculogenesis: Just what it takes to make a blood vessel. Int. J. Mol. Sci. 20, 3962. doi:10.3390/ijms20163962
Moccia, F., Nusco, G. A., Lim, D., Kyozuka, K., and Santella, L. (2006). NAADP and InsP3 play distinct roles at fertilization in starfish oocytes. Dev. Biol. 294, 24–38. doi:10.1016/j.ydbio.2006.02.011
Moccia, F., Zuccolo, E., Di Nezza, F., Pellavio, G., Faris, P. S., Negri, S., et al. (2021b). Nicotinic acid adenine dinucleotide phosphate activates two-pore channel TPC1 to mediate lysosomal Ca(2+) release in endothelial colony-forming cells. J. Cell Physiol. 236, 688–705. doi:10.1002/jcp.29896
Montes De Oca Balderas, P., and Aguilera, P. (2015). A metabotropic-like flux-independent NMDA receptor regulates Ca2+ exit from endoplasmic reticulum and mitochondrial membrane potential in cultured astrocytes. PLoS One 10, e0126314. doi:10.1371/journal.pone.0126314
Montes De Oca Balderas, P. (2022). Meeting report: Flux-independent signaling by ionotropic receptors: Unforeseen roles, complexities, and challenges. J. Biol. Chem. 298, 102330. doi:10.1016/j.jbc.2022.102330
Morgan, A. J., Davis, L. C., and Galione, A. (2015). Imaging approaches to measuring lysosomal calcium. Methods Cell Biol. 126, 159–195. doi:10.1016/bs.mcb.2014.10.031
Morgan, A. J., Platt, F. M., Lloyd-Evans, E., and Galione, A. (2011). Molecular mechanisms of endolysosomal Ca2+ signalling in health and disease. Biochem. J. 439, 349–374. doi:10.1042/BJ20110949
Negri, S., Faris, P., Maniezzi, C., Pellavio, G., Spaiardi, P., Botta, L., et al. (2021a). NMDA receptors elicit flux-independent intracellular Ca(2+) signals via metabotropic glutamate receptors and flux-dependent nitric oxide release in human brain microvascular endothelial cells. Cell Calcium 99, 102454. doi:10.1016/j.ceca.2021.102454
Negri, S., Faris, P., and Moccia, F. (2021b). Endolysosomal Ca(2+) signaling in cardiovascular health and disease. Int. Rev. Cell Mol. Biol. 363, 203–269. doi:10.1016/bs.ircmb.2021.03.001
Negri, S., Faris, P., Soda, T., and Moccia, F. (2021c). Endothelial signaling at the core of neurovascular coupling: The emerging role of endothelial inward-rectifier K(+) (Kir2.1) channels and N-methyl-d-aspartate receptors in the regulation of cerebral blood flow. Int. J. Biochem. Cell Biol. 135, 105983. doi:10.1016/j.biocel.2021.105983
Negri, S., Faris, P., Tullii, G., Vismara, M., Pellegata, A. F., Lodola, F., et al. (2022a). Conjugated polymers mediate intracellular Ca(2+) signals in circulating endothelial colony forming cells through the reactive oxygen species-dependent activation of Transient Receptor Potential Vanilloid 1 (TRPV1). Cell Calcium 101, 102502. doi:10.1016/j.ceca.2021.102502
Negri, S., Scolari, F., Vismara, M., Brunetti, V., Faris, P., Terribile, G., et al. (2022b). GABA(A) and GABA(B) receptors mediate GABA-induced intracellular Ca(2+) signals in human brain microvascular endothelial cells. Cells 11, 3860. doi:10.3390/cells11233860
Nelson, M. T., Cheng, H., Rubart, M., Santana, L. F., Bonev, A. D., Knot, H. J., et al. (1995). Relaxation of arterial smooth muscle by calcium sparks. Science 270, 633–637. doi:10.1126/science.270.5236.633
Nesher, N., Maiole, F., Shomrat, T., Hochner, B., and Zullo, L. (2019). From synaptic input to muscle contraction: Arm muscle cells of Octopus vulgaris show unique neuromuscular junction and excitation-contraction coupling properties. Proc. Biol. Sci. 286, 20191278. doi:10.1098/rspb.2019.1278
Noren, N. K., Liu, B. P., Burridge, K., and Kreft, B. (2000). p120 catenin regulates the actin cytoskeleton via Rho family GTPases. J. Cell Biol. 150, 567–580. doi:10.1083/jcb.150.3.567
Oksanen, M., Lehtonen, S., Jaronen, M., Goldsteins, G., Hamalainen, R. H., and Koistinaho, J. (2019). Astrocyte alterations in neurodegenerative pathologies and their modeling in human induced pluripotent stem cell platforms. Cell Mol. Life Sci. 76, 2739–2760. doi:10.1007/s00018-019-03111-7
Oksanen, M., Petersen, A. J., Naumenko, N., Puttonen, K., Lehtonen, S., Gubert Olive, M., et al. (2017). PSEN1 mutant iPSC-derived model reveals severe astrocyte pathology in alzheimer's disease. Stem Cell Rep. 9, 1885–1897. doi:10.1016/j.stemcr.2017.10.016
Ong, H. L., Subedi, K. P., Son, G. Y., Liu, X., and Ambudkar, I. S. (2019). Tuning store-operated calcium entry to modulate Ca(2+)-dependent physiological processes. Biochim. Biophys. Acta Mol. Cell Res. 1866, 1037–1045. doi:10.1016/j.bbamcr.2018.11.018
Paillusson, S., Stoica, R., Gomez-Suaga, P., Lau, D. H. W., Mueller, S., Miller, T., et al. (2016). There's something wrong with my MAM; the ER-mitochondria Axis and neurodegenerative diseases. Trends Neurosci. 39, 146–157. doi:10.1016/j.tins.2016.01.008
Patel, S., and Cai, X. (2015). Evolution of acidic Ca²⁺ stores and their resident Ca²⁺-permeable channels. Cell Calcium 57, 222–230. doi:10.1016/j.ceca.2014.12.005
Patel, S. (2015). Function and dysfunction of two-pore channels. Sci. Signal 8, re7. doi:10.1126/scisignal.aab3314
Patella, F., Schug, Z. T., Persi, E., Neilson, L. J., Erami, Z., Avanzato, D., et al. (2015). Proteomics-based metabolic modeling reveals that fatty acid oxidation (FAO) controls endothelial cell (EC) permeability. Mol. Cell Proteomics 14, 621–634. doi:10.1074/mcp.M114.045575
Paterno, G. M., Bondelli, G., Sakai, V. G., Sesti, V., Bertarelli, C., and Lanzani, G. (2020a). The effect of an intramembrane light-actuator on the dynamics of phospholipids in model membranes and intact cells. Langmuir 36, 11517–11527. doi:10.1021/acs.langmuir.0c01846
Paterno, G. M., Colombo, E., Vurro, V., Lodola, F., Cimo, S., Sesti, V., et al. (2020b). Membrane environment enables ultrafast isomerization of amphiphilic azobenzene. Adv. Sci. (Weinh) 7, 1903241. doi:10.1002/advs.201903241
Peng, M., Wang, Z., Yang, Z., Tao, L., Liu, Q., Yi, L. U., et al. (2015). Overexpression of short TRPM8 variant α promotes cell migration and invasion, and decreases starvation-induced apoptosis in prostate cancer LNCaP cells. Oncol. Lett. 10, 1378–1384. doi:10.3892/ol.2015.3373
Pressey, J. C., and Woodin, M. A. (2021). Kainate receptor regulation of synaptic inhibition in the hippocampus. J. Physiol. 599, 485–492. doi:10.1113/JP279645
Prevarskaya, N., Flourakis, M., Bidaux, G., Thebault, S., and Skryma, R. (2007). Differential role of TRP channels in prostate cancer. Biochem. Soc. Trans. 35, 133–135. doi:10.1042/BST0350133
Prevarskaya, N., Skryma, R., and Shuba, Y. (2018). Ion channels in cancer: Are cancer hallmarks oncochannelopathies? Physiol. Rev. 98, 559–621. doi:10.1152/physrev.00044.2016
Procino, G., Gerbino, A., Milano, S., Nicoletti, M. C., Mastrofrancesco, L., Carmosino, M., et al. (2015). Rosiglitazone promotes AQP2 plasma membrane expression in renal cells via a Ca-dependent/cAMP-independent mechanism. Cell Physiol. Biochem. 35, 1070–1085. doi:10.1159/000373933
Prole, D. L., and Taylor, C. W. (2019). Structure and function of IP3 receptors. Cold Spring Harb. Perspect. Biol. 11, a035063. doi:10.1101/cshperspect.a035063
Rizzuto, R., Brini, M., Murgia, M., and Pozzan, T. (1993). Microdomains with high Ca2+ close to IP3-sensitive channels that are sensed by neighboring mitochondria. Science 262, 744–747. doi:10.1126/science.8235595
Rocchio, F., Tapella, L., Manfredi, M., Chisari, M., Ronco, F., Ruffinatti, F. A., et al. (2019). Gene expression, proteome and calcium signaling alterations in immortalized hippocampal astrocytes from an Alzheimer's disease mouse model. Cell Death Dis. 10, 24. doi:10.1038/s41419-018-1264-8
Ronco, V., Grolla, A. A., Glasnov, T. N., Canonico, P. L., Verkhratsky, A., Genazzani, A. A., et al. (2014). Differential deregulation of astrocytic calcium signalling by amyloid-β, TNFα, IL-1β and LPS. Cell Calcium 55, 219–229. doi:10.1016/j.ceca.2014.02.016
Ronco, V., Potenza, D. M., Denti, F., Vullo, S., Gagliano, G., Tognolina, M., et al. (2015). A novel Ca²⁺-mediated cross-talk between endoplasmic reticulum and acidic organelles: Implications for NAADP-dependent Ca²⁺ signalling. Cell Calcium 57, 89–100. doi:10.1016/j.ceca.2015.01.001
Ruffinatti, F., Tapella, L., Gregnanin, I., Stevano, A., Chiorino, G., Canonico, P. L., et al. (2018). Transcriptional remodeling in primary hippocampal astrocytes from an alzheimer's disease mouse model. Curr. Alzheimer Res. 15, 986–1004. doi:10.2174/1567205015666180613113924
Sahoo, N., Gu, M., Zhang, X., Raval, N., Yang, J., Bekier, M., et al. (2017). Gastric acid secretion from parietal cells is mediated by a Ca(2+) efflux channel in the tubulovesicle. Dev. Cell 41, 262–273.e6. doi:10.1016/j.devcel.2017.04.003
Sanchez-Vazquez, V. H., Martinez-Martinez, E., Gallegos-Gomez, M. L., Arias, J. M., Pallafacchina, G., Rizzuto, R., et al. (2023). Heterogeneity of the endoplasmic reticulum Ca(2+) store determines colocalization with mitochondria. Cell Calcium 109, 102688. doi:10.1016/j.ceca.2022.102688
Santello, M., Toni, N., and Volterra, A. (2019). Astrocyte function from information processing to cognition and cognitive impairment. Nat. Neurosci. 22, 154–166. doi:10.1038/s41593-018-0325-8
Santulli, G., Lewis, D., Des Georges, A., Marks, A. R., and Frank, J. (2018). Ryanodine receptor structure and function in health and disease. Subcell. Biochem. 87, 329–352. doi:10.1007/978-981-10-7757-9_11
Sbano, L., Bonora, M., Marchi, S., Baldassari, F., Medina, D. L., Ballabio, A., et al. (2017). TFEB-mediated increase in peripheral lysosomes regulates store-operated calcium entry. Sci. Rep. 7, 40797. doi:10.1038/srep40797
Scarpellino, G., Munaron, L., Cantelmo, A. R., and Fiorio Pla, A. (2020). Calcium-permeable channels in tumor vascularization: Peculiar sensors of microenvironmental chemical and physical cues. Rev. Physiol. Biochem. Pharmacol. 182, 111–137. doi:10.1007/112_2020_32
Schaeffer, S., and Iadecola, C. (2021). Revisiting the neurovascular unit. Nat. Neurosci. 24, 1198–1209. doi:10.1038/s41593-021-00904-7
Scorza, S. I., Milano, S., Saponara, I., Certini, M., De Zio, R., Mola, M. G., et al. (2023). TRPML1-Induced lysosomal Ca(2+) signals activate AQP2 translocation and water flux in renal collecting duct cells. Int. J. Mol. Sci. 24, 1647. doi:10.3390/ijms24021647
Sforna, L., Michelucci, A., Morena, F., Argentati, C., Franciolini, F., Vassalli, M., et al. (2022). Piezo1 controls cell volume and migration by modulating swelling-activated chloride current through Ca(2+) influx. J. Cell Physiol. 237, 1857–1870. doi:10.1002/jcp.30656
Soda, T., Brunetti, V., Berra-Romani, R., and Moccia, F. (2023). The emerging role of N-Methyl-D-Aspartate (NMDA) receptors in the cardiovascular system: Physiological implications, pathological consequences, and therapeutic perspectives. Int. J. Mol. Sci. 24, 3914. doi:10.3390/ijms24043914
Soda, T., Mapelli, L., Locatelli, F., Botta, L., Goldfarb, M., Prestori, F., et al. (2019). Hyperexcitability and hyperplasticity disrupt cerebellar signal transfer in the IB2 KO mouse model of autism. J. Neurosci. 39, 2383–2397. doi:10.1523/JNEUROSCI.1985-18.2019
Tapella, L., Dematteis, G., Genazzani, A. A., De Paola, M., and Lim, D. (2023). Immortalized hippocampal astrocytes from 3xTg-AD mice, a new model to study disease-related astrocytic dysfunction: A comparative review. Neural Regen. Res. 18, 1672–1678. doi:10.4103/1673-5374.363192
Tapella, L., Dematteis, G., Moro, M., Pistolato, B., Tonelli, E., Vanella, V. V., et al. (2022). Protein synthesis inhibition and loss of homeostatic functions in astrocytes from an alzheimer's disease mouse model: A role for ER-mitochondria interaction. Cell Death Dis. 13, 878. doi:10.1038/s41419-022-05324-4
Tapella, L., Soda, T., Mapelli, L., Bortolotto, V., Bondi, H., Ruffinatti, F. A., et al. (2020). Deletion of calcineurin from GFAP-expressing astrocytes impairs excitability of cerebellar and hippocampal neurons through astroglial Na(+)/K(+) ATPase. Glia 68, 543–560. doi:10.1002/glia.23737
Thakore, P., Pritchard, H. a. T., Griffin, C. S., Yamasaki, E., Drumm, B. T., Lane, C., et al. (2020). TRPML1 channels initiate Ca(2+) sparks in vascular smooth muscle cells. Sci. Signal 13, eaba1015. doi:10.1126/scisignal.aba1015
Thebault, S., Lemonnier, L., Bidaux, G., Flourakis, M., Bavencoffe, A., Gordienko, D., et al. (2005). Novel role of cold/menthol-sensitive transient receptor potential melastatine family member 8 (TRPM8) in the activation of store-operated channels in LNCaP human prostate cancer epithelial cells. J. Biol. Chem. 280, 39423–39435. doi:10.1074/jbc.M503544200
Thillaiappan, N. B., Chavda, A. P., Tovey, S. C., Prole, D. L., and Taylor, C. W. (2017). Ca(2+) signals initiate at immobile IP3 receptors adjacent to ER-plasma membrane junctions. Nat. Commun. 8, 1505. doi:10.1038/s41467-017-01644-8
Tiwari, M. N., Mohan, S., Biala, Y., and Yaari, Y. (2018). Differential contributions of Ca(2+) -activated K(+) channels and Na(+)/K(+) -ATPases to the generation of the slow afterhyperpolarization in CA1 pyramidal cells. Hippocampus 28, 338–357. doi:10.1002/hipo.22836
Tomilin, V. N., Mamenko, M., Zaika, O., Ren, G., Marrelli, S. P., Birnbaumer, L., et al. (2019). TRPC3 determines osmosensitive [Ca2+]i signaling in the collecting duct and contributes to urinary concentration. PLoS One 14, e0226381. doi:10.1371/journal.pone.0226381
Tsagareli, M. G., and Nozadze, I. (2020). An overview on transient receptor potential channels superfamily. Behav. Pharmacol. 31, 413–434. doi:10.1097/FBP.0000000000000524
Tsavaler, L., Shapero, M. H., Morkowski, S., and Laus, R. (2001). Trp-p8, a novel prostate-specific gene, is up-regulated in prostate cancer and other malignancies and shares high homology with transient receptor potential calcium channel proteins. Cancer Res. 61, 3760–3769.
Vaeth, M., Kahlfuss, S., and Feske, S. (2020). CRAC channels and calcium signaling in T cell-mediated immunity. Trends Immunol. 41, 878–901. doi:10.1016/j.it.2020.06.012
Vangeel, L., and Voets, T. (2019). Transient receptor potential channels and calcium signaling. Cold Spring Harb. Perspect. Biol. 11, a035048. doi:10.1101/cshperspect.a035048
Venetucci, L., Denegri, M., Napolitano, C., and Priori, S. G. (2012). Inherited calcium channelopathies in the pathophysiology of arrhythmias. Nat. Rev. Cardiol. 9, 561–575. doi:10.1038/nrcardio.2012.93
Verkhratsky, A., and Nedergaard, M. (2016). The homeostatic astroglia emerges from evolutionary specialization of neural cells. Philos. Trans. R. Soc. Lond B Biol. Sci. 371, 20150428. doi:10.1098/rstb.2015.0428
Verkhratsky, A., Parpura, V., Rodriguez-Arellano, J. J., and Zorec, R. (2019). Astroglia in alzheimer's disease. Adv. Exp. Med. Biol. 1175, 273–324. doi:10.1007/978-981-13-9913-8_11
Vetter, I. R., and Wittinghofer, A. (2001). The guanine nucleotide-binding switch in three dimensions. Science 294, 1299–1304. doi:10.1126/science.1062023
Vrenken, K. S., Jalink, K., Van Leeuwen, F. N., and Middelbeek, J. (2016). Beyond ion-conduction: Channel-dependent and -independent roles of TRP channels during development and tissue homeostasis. Biochim. Biophys. Acta 1863, 1436–1446. doi:10.1016/j.bbamcr.2015.11.008
Vurro, V., Federici, B., Ronchi, C., Florindi, C., Sesti, V., Crasto, S., et al. (2023a). Optical modulation of excitation-contraction coupling in human-induced pluripotent stem cell-derived cardiomyocytes. iScience 26, 106121. doi:10.1016/j.isci.2023.106121
Vurro, V., Shani, K., Ardona, H. a. M., Zimmerman, J. F., Sesti, V., Lee, K. Y., et al. (2023b). Light-triggered cardiac microphysiological model. Apl. Bioeng. 7, 026108. doi:10.1063/5.0143409
Wang, L., and Li, Q. (2018). Photochromism into nanosystems: Towards lighting up the future nanoworld. Chem. Soc. Rev. 47, 1044–1097. doi:10.1039/c7cs00630f
Wang, Y., Wang, X., Yang, Z., Zhu, G., Chen, D., and Meng, Z. (2012). Menthol inhibits the proliferation and motility of prostate cancer DU145 cells. Pathol. Oncol. Res. 18, 903–910. doi:10.1007/s12253-012-9520-1
Woudenberg-Vrenken, T. E., Bindels, R. J., and Hoenderop, J. G. (2009). The role of transient receptor potential channels in kidney disease. Nat. Rev. Nephrol. 5, 441–449. doi:10.1038/nrneph.2009.100
Xu, H., and Ren, D. (2015). Lysosomal physiology. Annu. Rev. Physiol. 77, 57–80. doi:10.1146/annurev-physiol-021014-071649
Yang, J., Zhao, Z., Gu, M., Feng, X., and Xu, H. (2019). Release and uptake mechanisms of vesicular Ca(2+) stores. Protein Cell 10, 8–19. doi:10.1007/s13238-018-0523-x
Yang, Z. H., Wang, X. H., Wang, H. P., and Hu, L. Q. (2009). Effects of TRPM8 on the proliferation and motility of prostate cancer PC-3 cells. Asian J. Androl. 11, 157–165. doi:10.1038/aja.2009.1
Yin, Y., Le, S. C., Hsu, A. L., Borgnia, M. J., Yang, H., and Lee, S. Y. (2019). Structural basis of cooling agent and lipid sensing by the cold-activated TRPM8 channel. Science 363, eaav9334. doi:10.1126/science.aav9334
Yin, Y., Wu, M., Zubcevic, L., Borschel, W. F., Lander, G. C., and Lee, S. Y. (2018). Structure of the cold- and menthol-sensing ion channel TRPM8. Science 359, 237–241. doi:10.1126/science.aan4325
Zhang, J., Wang, J., and Tian, H. (2014). Taking orders from light: Progress in photochromic bio-materials. Mater. Horizons 1, 169–184. doi:10.1039/c3mh00031a
Zhang, L., and Barritt, G. J. (2004). Evidence that TRPM8 is an androgen-dependent Ca2+ channel required for the survival of prostate cancer cells. Cancer Res. 64, 8365–8373. doi:10.1158/0008-5472.CAN-04-2146
Zhang, X., Cheng, X., Yu, L., Yang, J., Calvo, R., Patnaik, S., et al. (2016). MCOLN1 is a ROS sensor in lysosomes that regulates autophagy. Nat. Commun. 7, 12109. doi:10.1038/ncomms12109
Zhang, X., Mak, S., Li, L., Parra, A., Denlinger, B., Belmonte, C., et al. (2012). Direct inhibition of the cold-activated TRPM8 ion channel by Gαq. Nat. Cell Biol. 14, 851–858. doi:10.1038/ncb2529
Zhao, X., Liang, B., Li, C., and Wang, W. (2023). Expression regulation and trafficking of aquaporins. Adv. Exp. Med. Biol. 1398, 39–51. doi:10.1007/978-981-19-7415-1_3
Zholos, A., Johnson, C., Burdyga, T., and Melanaphy, D. (2011). TRPM channels in the vasculature. Adv. Exp. Med. Biol. 704, 707–729. doi:10.1007/978-94-007-0265-3_37
Zhu, G., Wang, X., Yang, Z., Cao, H., Meng, Z., Wang, Y., et al. (2011). Effects of TRPM8 on the proliferation and angiogenesis of prostate cancer PC-3 cells in vivo. Oncol. Lett. 2, 1213–1217. doi:10.3892/ol.2011.410
Zuccolo, E., Di Buduo, C., Lodola, F., Orecchioni, S., Scarpellino, G., Kheder, D. A., et al. (2018a). Stromal cell-derived factor-1α promotes endothelial colony-forming cell migration through the Ca2+-dependent activation of the extracellular signal-regulated kinase 1/2 and phosphoinositide 3-kinase/AKT pathways. Stem Cells Dev. 27, 23–34. doi:10.1089/scd.2017.0114
Zuccolo, E., Kheder, D. A., Lim, D., Perna, A., Nezza, F. D., Botta, L., et al. (2019). Glutamate triggers intracellular Ca(2+) oscillations and nitric oxide release by inducing NAADP- and InsP3 -dependent Ca(2+) release in mouse brain endothelial cells. J. Cell Physiol. 234, 3538–3554. doi:10.1002/jcp.26953
Zuccolo, E., Laforenza, U., Ferulli, F., Pellavio, G., Scarpellino, G., Tanzi, M., et al. (2018b). Stim and Orai mediate constitutive Ca(2+) entry and control endoplasmic reticulum Ca(2+) refilling in primary cultures of colorectal carcinoma cells. Oncotarget 9, 31098–31119. doi:10.18632/oncotarget.25785
Keywords: Ca2+ signalling, lysosomal Ca2+, mitochondria-ER contact sites, TRP channels, non-canonical signalling, optical stimulation
Citation: Moccia F, Fiorio Pla A, Lim D, Lodola F and Gerbino A (2023) Intracellular Ca2+ signalling: unexpected new roles for the usual suspect. Front. Physiol. 14:1210085. doi: 10.3389/fphys.2023.1210085
Received: 21 April 2023; Accepted: 18 July 2023;
Published: 27 July 2023.
Edited by:
Fátima Regina Mena Barreto Silva, Federal University of Santa Catarina, BrazilReviewed by:
Roberta Gualdani, Université catholique de Louvain, BelgiumCopyright © 2023 Moccia, Fiorio Pla, Lim, Lodola and Gerbino. This is an open-access article distributed under the terms of the Creative Commons Attribution License (CC BY). The use, distribution or reproduction in other forums is permitted, provided the original author(s) and the copyright owner(s) are credited and that the original publication in this journal is cited, in accordance with accepted academic practice. No use, distribution or reproduction is permitted which does not comply with these terms.
*Correspondence: Andrea Gerbino, YW5kcmVhLmdlcmJpbm9AdW5pYmEuaXQ=
†These authors have contributed equally to this work and share last authorship
Disclaimer: All claims expressed in this article are solely those of the authors and do not necessarily represent those of their affiliated organizations, or those of the publisher, the editors and the reviewers. Any product that may be evaluated in this article or claim that may be made by its manufacturer is not guaranteed or endorsed by the publisher.
Research integrity at Frontiers
Learn more about the work of our research integrity team to safeguard the quality of each article we publish.