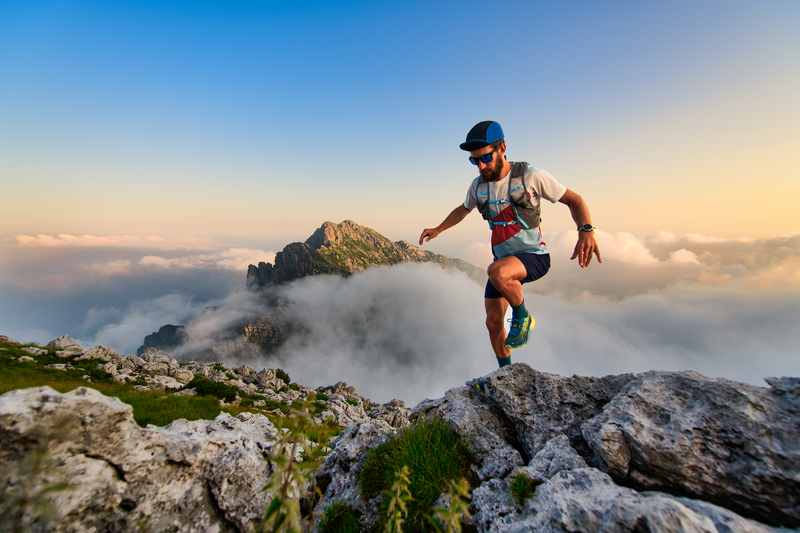
95% of researchers rate our articles as excellent or good
Learn more about the work of our research integrity team to safeguard the quality of each article we publish.
Find out more
ORIGINAL RESEARCH article
Front. Physiol. , 20 November 2023
Sec. Mitochondrial Research
Volume 14 - 2023 | https://doi.org/10.3389/fphys.2023.1207567
This article is part of the Research Topic Mitochondrial function and dysfunction in pathogenic fungi View all 6 articles
Aims: Farnesol was identified 20 years ago in a search for Candida albicans quorum sensing molecules (QSM), but there is still uncertainty regarding many aspects of its mode of action including whether it employs farnesol transport mechanisms other than diffusion. Based on the structural similarity between farnesol and the farnesylated portion of the MTLa pheromone, we explored the effects of ploidy and mating type locus (MTL) on the antifungal activity of exogenous farnesol.
Methods and results: We approached this question by examining five MTLa and five MTLα haploid strains with regard to their farnesol sensitivity in comparison to six heterozygous MTLa/α diploids. We examined the haploid and diploid strains for percent cell death after exposure of exponentially growing cells to 0–200 µM farnesol. The heterozygous (MTLa/α) diploids were tolerant of exogenous farnesol whereas the MTLa and MTLα haploids were on average 2- and 4-times more sensitive, respectively. In the critical range from 10–40 µM farnesol their cell death values were in the ratio of 1:2:4. Very similar results were obtained with two matched sets of MATa, MATα, and MATa/α Saccharomyces cerevisiae strains.
Conclusion: We propose that the observed MTL dependence of farnesol is based on differentially regulated mechanisms of entry and efflux which determine the actual cellular concentration of farnesol. The mechanisms by which pathogens such as C. albicans tolerate the otherwise lethal effects of farnesol embrace a wide range of physiological functions, including MTL type, ubiquinone type (UQ6-UQ9), energy availability, and aerobic/anaerobic status.
The dimorphic fungus C. albicans is an important human pathogen. Yeast-hyphal interconversion is essential for its pathogenicity and in 2001 we identified the sesquiterpene E,E-farnesol as a quorum sensing molecule (QSM) able to block the yeast to hypha conversion in a cell density dependent manner (Hornby et al., 2001) and to inhibit biofilm formation (Ramage et al., 2002). Later we found that excreted farnesol also acted as a virulence factor for pathogenicity in mice (Navarathna et al., 2007; Hargarten et al., 2015) and it induced apoptosis in numerous fungi including Aspergillus nidulans and Aspergillus fumigatus (Semighini et al., 2006; Shirtliff et al., 2009). Thus, farnesol is a highly bioactive molecule (Nickerson et al., 2006). The potential role of farnesol and related molecules (i.e. 2, 3-dihydrofarnesol) as useful antifungal drugs has regained interest. For instance, Brasch et al. (2014) detailed their effectiveness versus dermatophytes while Katragkou et al. (2015) showed their synergistic effectiveness with fluconazole, amphotericin B, and micafungin versus C. albicans biofilms. More recently, Nagy et al. (2020) reported that farnesol inhibited growth and biofilm forming ability by C. auris, Dekkerova et al. (2022) confirmed a synergistic effect between farnesol and fluconazole in C. auris, and Nikoomanesh et al. (2023) confirmed a synergism between farnesol and either fluconazole or itraconazole which restored the original sensitivity of azole-resistant C. albicans and Candida parapsilosis. The idea that C. albicans secretes antifungal molecules as a mechanism of competition with other fungi is longstanding. Pure cultures of C. albicans were sometimes isolated from clinical lesions (Lewis and Hooper, 1943) while the dermatophyte Trichophyton rubrum did not grow when cocultivated with either C. albicans or spent medium from C. albicans (Jillson and Nickerson, 1948). The idea that this antifungal molecule was farnesol and that the target was actively respiring mitochondria was inherent in the observations of Machida and Tanaka (1999) and Machida et al. (1998), Machida et al. (1999). They showed a dose-dependent growth inhibition and ROS production for farnesol but not for other closely related molecules. Farnesol (3 isoprenes, C15) inhibited the growth of S. cerevisiae at 12–25 µM whereas geraniol (2 isoprenes, C10), geranylgeraniol (4 isoprenes, C20), farnesyl acetate, linalool, and squalene were not inhibitory at concentrations up to 200 or 400 µM. The mitochondrial involvement was clearly shown by the toxicity of 20–30 µM farnesol to S. cerevisiae, while petite mutants of S. cerevisiae (Machida et al., 1998; Fairn et al., 2007; Pathirana et al., 2020) and anaerobically grown C. albicans (Dumitru et al., 2004) were farnesol resistant.
An intriguing unanswered question concerns how 20–25 µM farnesol can kill or lyse potentially competing yeasts (Machida et al., 1998) and fungi (Semighini et al., 2006) as well as the opaque form of C. albicans (Dumitru et al., 2007) whereas the white form of C. albicans can tolerate 250–300 µM farnesol (Hornby et al., 2001; Ramage et al., 2002; Polke et al., 2017). What mechanisms does C. albicans use to protect itself from farnesol? This question applies to both exogenous farnesol and intracellular farnesol (Boone et al., 2022). We also know that the sensitivity of C. albicans to exogenous farnesol depends on the stage of growth at which the cells were stressed; cultures inoculated with stationary-phase cells tolerated up to 300 µM farnesol whereas those inoculated with exponential-phase cells were inhibited by 40 μM farnesol and killed by 100–300 µM farnesol (Uppuluri et al., 2007; Langford et al., 2010). At least three possible answers to this question have been tested. The first concerns the need for an energy source as was nicely shown by Shirtliff et al. (2009) wherein C. albicans cells underwent apoptosis after treatment with 40 µM farnesol if they had first been incubated for 24 h in phosphate-buffered saline (PBS). Both exponential- and stationary-phase cells were 2–10 times more likely to be lysed by farnesol when incubated in PBS than when incubated in growth media (Shirtliff et al., 2009; Langford et al., 2010). This need for an energy source to tolerate otherwise lethal detergent-like molecules duplicates our prior observation with Enterobacter cloacae growing in 1%–10% sodium dodecyl sulfate (SDS). Bacterial cells which entered stationary phase owing to carbon limitation were rapidly lysed by the SDS present whereas those that had entered owing to nitrogen or phosphorous limitation were not (Aspedon and Nickerson, 1993). An attractive model for how this energy dependence operates in C. albicans was presented by Liu et al. (2018) wherein farnesol activated the Tac1 and Znc1 transcription factors, thus inducing expression of the Cdr1, an ABC transporter and multidrug efflux pump, known to export phospholipids and possibly farnesol as well. The second idea concerns the types of ubiquinones synthesized by the microbes. Ubiquinones consist of a benzoquinone redox active ring system attached to a side chain with variable isoprenoid units. S. cerevisiae has six isoprenoid units in its side chain (UQ6), while most Candida sp. have seven (UQ7), and C. albicans and C. dubliniensis have nine (UQ9). Previously, we showed that S. cerevisiae engineered to produce UQ9 were ca. 5X less sensitive to exogenous farnesol than their parent S. cerevisiae and produced ca. 10X less ROS in response to a given level of farnesol (Pathirana et al., 2020). We then suggested that C. albicans and C. dubliniensis evolved to use UQ9 rather than UQ7 in part to protect themselves from their secreted farnesol (Pathirana et al., 2020). The third idea, and the one tested in this paper, is closely tied to the unanswered question of whether the lipophilic farnesol enters a cell via diffusion, facilitated diffusion, or active transport (Nickerson and Atkin, 2016). Both farnesol and the homoserine lactones used by Gram-negative bacteria were first identified following extraction of the active molecules into ethyl acetate (Eberhard et al., 1981; Hornby et al., 2001) and thus they both should have some capacity to enter via diffusion.
A variable capacity for diffusion was implicit in the studies of Polke et al. (2017) who identified eed1∆/∆ as the first farnesol hypersensitive mutant of C. albicans. This mutant was 50-fold more sensitive to exogenous farnesol as a QSM and it also secreted ×10 more farnesol than its parent. Also, while able to form hyphae, eed1∆/∆ could not maintain those hyphae (Polke et al., 2017). Then, in a MicroCommentary on the mechanistic implications of eed1∆/∆, we (Nickerson and Atkin, 2016) suggested that C. albicans has a regulated farnesol transporter which might be related to using a farnesylated peptide as a mating pheromone (Bennett et al., 2003; Dignard et al., 2007). The a-factor is a farnesylated peptide secreted by mating type a cells via an ABC transporter called Ste6p or Hst6p and then recognized and bound by mating type α cells via an a-factor receptor called Ste3p. Ste6p is in the plasma membrane of MTLa cells only and Ste3p is in the plasma membrane of MTLα cells only. We further suggested that Ste6p or Ste3p might transport free farnesol as well as the farnesylated peptide. A key point is that in MTLa/α heterozygotes, both a-specfic, α-specific, and haploid specific gene expression is turned off, at least in S. cerevisiae, suggesting that the evolutionary pressure for C. albicans to become diploid derived in part from its use of farnesol as a QSM and a virulence factor (Nickerson and Atkin, 2016); the secreted farnesol would otherwise have been toxic to one or both of the haploid cell types.
The present paper examines the farnesol sensitivity for six diploid and ten haploid strains of C. albicans. The haploid strains, five MTLa and five MTLα, were described by Hickman et al. (2013). Transport of exogenous farnesol by a haploid specific mechanism was corroborated in that the heterozygous diploid strains of C. albicans were 2.4 times more resistant to 20–40 µM farnesol than the MATa haploid cells, and 4.6 times more resistant than the MATα haploid cells. Furthermore, the farnesol sensitivities of two MTLα/α and two MTLa/a cell lines matched those of the α haploid and a haploid cells, respectively. These results provide a partial explanation for why C. albicans is primarily diploid and why heterozygous diploid strains of C. albicans are more virulent than homozygous diploids (Lockhart et al., 2005).
A complete list of the yeast strains is in Table 1. The haploid C. albicans cell lines were previously described and obtained from the Judith Berman laboratory (Hickman et al., 2013). The BY4741-BY4743 S. cerevisiae series was purchased from EUROSCARF, Oberusel, Germany. The W303 series was obtained from Susan Wente, Vanderbilt University, Nashville, Tennessee. Cultures were grown in YPD [1% (w/v) yeast extract, 2% peptone (w/v) and 2% dextrose (w/v)] media. A 3 mL overnight culture grown from a single colony was used to inoculate 50 mL YPD in 250 mL Erlenmeyer flask at an OD600 of 0.1. The 50 mL cultures were grown for 4–6 h at 30°C on a rotary shaker set at 270 RPM until they had reached ca. 2 × 107 cells mL−1 (OD600 = 0.5) whereupon they were subdivided into six 25 mL Erlenmeyer flasks (5 mL) containing 0–200 µM farnesol in methanol and incubated as above for an additional 30 min. The negative control without farnesol received 50 µL methanol (1%), equivalent to the methanol added for 200 µM farnesol. The E, E-farnesol stock solution (Sigma product # 277541) was sealed under nitrogen and stored at −20°C. Note that farnesol has a maximum water solubility of only 1–1.2 mM (Knobloch et al., 1988).
Cell vitality was assessed as shown in Figures 1A–D using FungaLight Yeast Vitality Kit (Life Technologies) combined with FACS (Boone et al., 2017). The kit contains the cell permeable, non-specific esterase substrate 5-carboxyfluorescein diacetate, acetoxymethyl ester (CFDA,AM) to assess esterase housekeeping protein activity, and the cell impermeable, DNA intercalating agent, Propidium Iodide (PI) to assess membrane integrity. Probe fluorescence indicative of cell death of individual cells to varying concentrations of farnesol exposure was measured on a BDFACS Canto II (BD Biosciences, San Jose CA, United States) instrument interfaced with FACS Diva v6.11 software (Becton, Dickinson and Co., Franklin Lakes NJ, United States) and analyzed using Flowjo v10.2 software (TreeStar Inc., Ashland OR, United States). Instrument setup, acquisition, and data analysis using non-stained and single-stained controls was performed as suggested by ISAC, the International Society for Analytical Cytometry (Lee et al., 2008). Following the 30-min incubation with farnesol, 1 mL aliquots were harvested by centrifugation (10,000 g at 4°C), washed once with an equal volume of PBS, diluted 10-fold (to 106 cells mL−1) in PBS with 0.01% Tween 20, and incubated with 2 µM CFDA, AM and 9 µM PI probes for 20 min in the dark, and analyzed by FACS. Non-stained, single probe stained, and double probe stained controls were used to ensure that a correct number of cells (106 cells mL−1) were used to prevent non-stained cells biasing results (non-stained population <5%). PE channel (PI stained or non-stained populations) histograms were used to assess the percentage of dead cells in the cultures, see Figure 1D. Thus, each run generates a live/dead histogram and the values reported in Figures 2–6 are the mean ± SEM of three histograms.
FIGURE 1. Experimental setup for percent cell viability using flow cytometry (A). Representative side scatter/forward scatter (SSC/FSC) plot gated to include whole cells (the P1 population) but exclude sample debris from analysis (B). Cell death histogram for a non-stained sample (PI negative control) and heat killed sample (PI positive control) (C). Four controls showing how the gates were defined. Live cells, no stain control; heat killed cells, single PI probe; heat killed cells, single CFDA-AM probe; live cells, double probe. For PI staining, the gate is the horizontal line, cells above the line are dead. For CFDA-AM staining, four vertical lines are the gates, cells to the right of the line are viable. These controls determine the correct number of cells to use, to avoid having non-stained cells biasing the results (D). Sample histograms show the progression from live to dead cells following treatment with increasing concentrations of farnesol.
FIGURE 2. Candida albicans wild-type diploid farnesol sensitivity at increasing farnesol concentrations. Mean ± SEM across strains were calculated for strains SC5314, A72, MEN, 10261, and 3153A only. RM 1000 is an auxotrophic mutant derived from SC5314. Statistical analysis by 2-way ANOVA with Tukey’s post hoc correction. *p < 0.05, **p < 0.01.
S. cerevisiae cells were grown at 30°C in 500 mL flasks with 100 mL liquid GPP (Hornby et al., 2001). To fulfill their respective auxotrophies, the BY471, BY4742, and BY4743 strains had added Leu, Lys, Met, His, and uracil and the W303 series had added Trp, Leu, His and adenine (all at 40 μg/mL). After ca. 10 h growth the mid-log cultures (OD600 = 2.0–3.5) were diluted with fresh media to OD600 = 2.0 and then subdivided to compare their farnesol tolerance. For each strain, 10 mL of culture was aliquoted into each of a series of 50 mL flasks containing 0, 10, 20, 40, 80, and 200 µM farnesol and shaken at 220 RPM for 1 h at 30°C. Then 500 µL of the farnesol-stressed culture was mixed with 500 µL of propidium iodide (12 μg/mL) for a final PI concentration of 6 μg/mL and incubated for 10 min in the dark (Davey and Hexley, 2011). The PI stock (MP Biochemicals, Solon, Ohio) was 3 mg/mL in methanol, subsequently diluted by 40 µL in 10 mL water (12 μg/mL). In no case did the methanol exceed 1%. After their incubation with PI, the cultures were diluted 1:10 in water so that their cell densities better fit the dynamic range of the Countess II FL automated cell counter (Life Technologies, Carlsbad, CA). Ten µL of culture was loaded into each chamber of the dual chamber cell counting slides (Invitrogen), thus providing technical duplicate values. Bright field intensity was set at 30 and red fluorescence (RFP) at 61. These values were chosen based on control cultures with less than 5% PI positive cells. Small debris was size excluded prior to analysis. Dead cells exhibited red fluorescence. Percent dead cells was measured in technical duplicate and biological triplicate. Data are biological triplicate means ± SD (Figure 7) with live/dead ratios based on the percent of total cells which are positive (red); they are not dependent on cell size or the PI intensity per cell.
Statistical analyses were performed using Microsoft Excel (Version 16.52) and GraphPad Prism Software (Version 9.1.2). All data are represented as mean ± SEM unless otherwise stated. Normal distribution was accessed by visual inspection of Q-Q plots. Differences between two or more groups were accessed by Two-way ANOVA with Tukey’s post hoc test if differences were significant. Differences were considered significant at p < 0.05. Significance is denoted as *p < 0.05, **p < 0.01, ***p < 0.001.
Previous studies on the toxicity of exogenous farnesol to fungi have ignored the question of how farnesol enters the responding cell (Machida et al., 1998; Semighini et al., 2006). One explanation for why clinical isolates of C. albicans are so tolerant of exogenous farnesol invokes entry assisted by transport systems active in haploid cells but turned off or minimized in MTLa/α heterozygous diploid cells. Step one in testing this hypothesis is establishing a baseline for diploid C. albicans, i.e., not just strain SC5314.
Figure 2 shows the % cell death values for six diploid strains of C. albicans which had been stressed with 0–200 µM farnesol, strains SC5314, A72, MEN, 10261, 3153A, and RM1000. The negative control (0 µM farnesol) showed that 1% methanol was not harmful to any of the strains tested. The percent cell death values were very similar for all strains; they were effectively resistant to ≤20 µM exogenous farnesol and then exhibited 10, 20, and 44% cell death at 40, 80, and 200 µM farnesol, respectively (Figure 2). The appearance of significant cell death at 80 and 200 µM farnesol (Figure 2) does not contradict prior statements that C. albicans is resistant to 250–300 µM farnesol (Hornby et al., 2001; Ramage et al., 2002) because of differences in the experimental design. In Figure 2 farnesol was added to actively growing, exponential phase cells, which are more sensitive to farnesol than nongrowing cells exposed to farnesol from time zero (Uppuluri et al., 2007; Langford et al., 2010). For strain SC5314, the % death values with increasing farnesol were confirmed by CFU values on YPD plates (data not shown) and all subsequent experiments (Figures 2–4, 6) included SC5314 as a positive control.
Next, ten haploid strains, five MTLa (Figure 3) and five MTLα (Figure 4), were analyzed by FACS for their farnesol sensitivities, whereupon their average % cell death values were compared to those previously found for the diploids (Figure 5). The MTLa (Figure 3) and MTLα (Figure 4) haploids gave tight clusters with low standard deviation; they exhibited significant cell death even at 10 µM farnesol, the lowest concentration tested. For farnesol concentrations from 10 to 80 μM, the average % cell death values for the α haploids were roughly 2-times that for the a haploids (Figure 5) and for all concentrations, the cell death values were in the order: diploid < a haploid < α haploid (Figure 5). For the concentrations which we deem most physiologically significant (20–40 µM), the relative cell death values were ca. 1:2:4 (Figure 5).
FIGURE 3. Candida albicans MTLa farnesol sensitivity at increasing farnesol concentrations. Mean ± SEM across strains were calculated. For each farnesol concentration, the cluster of 5 bars reflect YJB 12814, 12864, 12868, 12870, and 12881 reading from left to right. Statistical analysis by 2-way ANOVA with Tukey’s post hoc correction. *p < 0.05, **p < 0.01, ***p < 0.001.
FIGURE 4. Candida albicans MTLα farnesol sensitivity at increasing farnesol concentrations. Mean ± SEM across strains were calculated. For each farnesol concentration, the cluster of 5 bars reflect YJB 12804, 12812, 12818, 12875, and 12880 reading from left to right. Statistical analysis by 2-way ANOVA with Tukey’s post hoc correction. *p < 0.05, **p < 0.01, ***p < 0.001.
FIGURE 5. Candida albicans wild-type diploid versus MTLa versus MTLα haploid farnesol sensitivity at increasing farnesol concentrations. Mean ± SEM signified by errors bars are from Figures 3, 4, this figure, respectively. The average fold differences are reported below [F], with the diploid averages assigned a value of 1. At 0 μM farnesol fold difference is not significant because total cell death was miniscule. Statistical analysis by 2-way ANOVA with Tukey’s post hoc correction. *p < 0.05, **p < 0.01, ***p < 0.001.
We also examined the farnesol sensitivity of four MTL homozygous diploids previously used to demonstrate anaerobic mating in C. albicans (Dumitru et al., 2007). As seen in Figure 6, the homozygous diploids exhibited % cell death values comparable with those observed for the corresponding haploid strains. The two MTLα/α strains (3,315 and 3,740) matched the high cell death rates of the MTLα haploids (Figure 4) while the two MTLa/a strains (3,294 and 3,745) matched those of the MTLa haploids (Figure 3). These data suggest that it is the combination of MTLa/α in the heterozygous diploids which confers farnesol resistance rather than the duplicated DNA content.
FIGURE 6. Candida albicans homozygous diploid farnesol sensitivity. Strains 3,315 and 3,740 = MTLα/α; strains 3,294 and 3,745 = MTLa/a. Statistical analysis by 2-way ANOVA with Tukey’s post hoc correction. *p < 0.05, **p < 0.01, ***p < 0.001.
The rationale for why diploid cells of C. albicans might be more farnesol tolerant than haploid cells applies equally to the model yeast S. cerevisiae because it too employs a farnesylated peptide as the a-pheromone (Michaelis and Barrowman, 2012). Two matched sets of S. cerevisiae diploid/a haploid/α haploid strains were examined in a PI based live/dead assay (Figure 7). All the negative control cultures (without added farnesol) had <5% PI positive cells. The results for the BY (Figure 7A) and W303 (Figure 7B) series were almost identical. As expected, the % PI positive cells were 20%–30% greater for S. cerevisiae (Figure 7) than for C. albicans (Figure 5) and the cell death values for all farnesol concentrations were in the same order: diploid < a haploid < α haploid (Figure 7). We conclude that the underlying mechanisms for the greater farnesol resistance exhibited by diploid yeasts are likely the same.
FIGURE 7. Saccharomyces cerevisiae diploid versus MATa versus MATα haploid farnesol sensitivity at increasing farnesol concentrations (A). BY4741-4743 series (B). W303 series. Mean ± SD (n = 3) with statistical analysis by 2-way ANOVA with Tukey’s post hoc correction. **p < 0.01, ***p < 0.001.
The adaptations made by a potential pathogen to become a successful pathogen are important and intriguing. C. albicans employs farnesol as one of its several virulence factors (Navarathna et al., 2007; Hargarten et al., 2015) and farnesol is a highly bioactive molecule. It is toxic to many fungi (Semighini et al., 2006) and its mode of action is thought to include interaction with fungal mitochondria leading to lethal levels of ROS production (Machida et al., 1998) and apoptosis (Semighini et al., 2006; Shirtliff et al., 2009). Any organism producing an antibiotic or other bioactive, biocidal molecule must perforce evolve mechanisms to protect itself from that molecule. We previously suggested that C. albicans and C. dubliniensis evolved to use UQ9 rather than UQ7 in part to protect themselves from their secreted farnesol (Pathirana et al., 2020). We now suggest that the push towards diploidization may have served a similar purpose. We have taken advantage of the collection of a and α haploid isolates of C. albicans (Hickman et al., 2013) to examine their sensitivity to exogenous farnesol. Marked differences were observed. With regard to farnesol sensitivity, all strains showed increasing percent cell death by flow cytometry as the farnesol concentration increased from 0 to 200 µM. However, the farnesol cell death values for the a/α diploid strains were always far less than for the haploid strains, with the % cell death values for diploids, a haploids, and α haploids being in the ratio of 1:2:4 (Figure 5). Thus, the higher farnesol sensitivity of α cells presents the opportunity for one haploid cell type to kill the other. These data are consistent with the suggestion that the secretion of farnesol as a QSM and as a virulence factor provided an evolutionary driving force for the diploidization of C. albicans (Nickerson and Atkin, 2016).
These results show the correlation of mating type with farnesol sensitivity (entry) and they are consistent with most of the predictions made by Nickerson and Atkin (2016). These predictions were based on both S. cerevisiae (Michaelis and Barrowman, 2012) and C. albicans (Dignard et al., 2007) using farnesylated peptide pheromones secreted by MTLa strains and then bound by MTLα strains. These functions are accomplished by Ste6p in MTLa and Ste3p in MTLα, respectively. Because both of these proteins are able to distinguish the farnesylated and non-farnesylated peptides, we hypothesized that they or related proteins would also be able to transport farnesol, or allow passage of farnesol, possibly at a reduced efficiency. The data presented in this paper are consistent with the idea that the haploid strains are more sensitive to exogenous farnesol because they have and express a set of genes that are unique to the MTLa and MTLα strains, or any other gene which is transcribed in a ploidy-specific or mating type specific manner.
As an intriguing possibility, Srikantha et al. (2012) showed that the MTL locus of C. albicans contained the mating type genes and also three apparently unrelated “nonsex” genes, the essential phosphatidyl inositol kinase gene, PIK, the poly(A) polymerase gene, PAP, and the nonessential oxysterol binding protein gene, OBP. The MTLa and MTLα loci both contain all three nonsex genes. Importantly, the DNA homologues of the a and α copies of PIK, PAP, and OBP were 58, 66, and 66% while the identity of the deduced a and α proteins encoded by PIK, PAP, and OBP were 81, 89, and 91%, respectively (Srikantha et al., 2012). These numbers are in marked contrast to the DNA homology (99.5%) and protein identity (99.6%) values for 50 genes neighboring the MTL locus (Srikantha et al., 2012).
The potential importance of these nonsex genes is evident in prior work from the Soll laboratory (Yi et al., 2011) when they showed that the biofilms produced by C. albicans MTLa/α cells differed dramatically from those produced by MTLa/a or MTLα/α cells. The biofilms were similar morphologically, but they were structurally and functionally distinct. In particular, biofilms formed by MTLa/α cells were impermeable to molecules in the size range of 140–300 Da and resistant to many antifungals whereas the MTLa/a and MTLα/α biofilms were permeable to molecules in this size range and susceptible to those antifungals (Yi et al., 2011). Farnesol has a molecular weight of 222, Srikantha et al. (2012) showed that OBP was an essential gene for biofilm impermeability and fluconazole resistance. Since the biofilms are morphologically indistinguishable, this difference in susceptibility suggests critical differences at the individual cell level.
Currently, our data show the importance of ploidy and mating type (a vs. α); they do not show anything further regarding an actual mechanism. Another possibility is that the ploidy state and/or mating type may determine more or less active farnesol metabolism in the cell, hence more or less resistance to growth inhibition or killing by farnesol. In this regard, neither C. albicans nor S. cerevisiae appears to have the two-step farnesol salvage pathway (Crick et al., 1997) whereby farnesol is converted to FPP (Verdaguer et al., 2022). This pathway is present in plants, animals, and bacteria but the relevant genes (VTE5 and VTE6 from Arabidopsis thaliana) have no homologs in either the Candida or Saccharomyces Genome Databases. Other regulated mechanisms for farnesol metabolism are still possible. In Boone et al. (2022) we followed the intracellular farnesol levels (Fi) for C. albicans SC5314 over 3 days of growth. The per cell Fi increased continuously during exponential growth but then decreased during stationary phase until they returned to their pre-growth levels. Such a decrease in Fi could have many explanations. However, a subsequent study of 164 transcription regulator knockout mutants in the Homann collection (Homann et al., 2009) identified two high-accumulating mutants that did not exhibit the decay in farnesol levels during stationary phase, suggesting that an otherwise uncharacterized farnesol modification/degradation mechanism is absent in these mutants (Gutzmann et al., 2023). Clearly there is an abundance of possible mechanisms for the ploidy and mating type specific differences we have observed.
The original contributions presented in the study are included in the article/Supplementary material, further inquiries can be directed to the corresponding author.
CB: conception and design, methodology, data collection; KP: data collection; DG: visualization, data analysis; AA: resources, supervision, writing, editing; KN: conception and design, resources, funding, writing. All authors contributed to the article and approved the submitted version.
This work was supported by Ann L. Kelsall and the Farnesol and C. albicans Research Fund, University of Nebraska Foundation.
We thank Judy Berman for providing the haploid isolates of C. albicans.
The authors declare that the research was conducted in the absence of any commercial or financial relationships that could be construed as a potential conflict of interest.
All claims expressed in this article are solely those of the authors and do not necessarily represent those of their affiliated organizations, or those of the publisher, the editors and the reviewers. Any product that may be evaluated in this article, or claim that may be made by its manufacturer, is not guaranteed or endorsed by the publisher.
Aspedon A., Nickerson K. W. (1993). A two-part energy burden imposed by growth of Enterobacter cloacae and Escherichia coli in sodium dodecyl sulfate. Can. J. Microbiol. 39, 555–561. doi:10.1139/m93-080
Bennett R. J., Uhl M. A., Miller A. G., Johnson A. D. (2003). Identification and characterization of a Candida albicans mating pheromone. Mol. Cell. Biol. 23 (22), 8189–8201. doi:10.1128/mcb.23.22.8189-8201.2003
Boone C. H. T., Grove R. A., Adamcova D., Seravalli J., Adamec J. (2017). Oxidative stress, metabolomics profiling, and mechanism of local anesthetic induced cell death in yeast. Redox Biol. 12, 139–149. doi:10.1016/j.redox.2017.01.025
Boone C. H. T., Gutzmann D. J., Kramer J. J., Atkin A. L., Nickerson K. W. (2022). Quantitative assay for farnesol and the aromatic fusel alcohols from the fungus Candida albicans. Appl. Microbiol. Biotech. 106, 6759–6773. doi:10.1007/s00253-022-12165-w
Brachmann C. B., Davies A., Cost G. J., Caputo E., Li J., Hieter P., et al. (1998). Designer deletion strains derived from Saccharomyces cerevisiae S288C: a useful set of strains and plasmids for PCR-mediated gene disruption and other applications. Yeast 14, 115–132. doi:10.1002/(SICI)1097-0061(19980130)14:2<115::AID-YEA204>3.0.CO;2-2
Brasch J., Horter F., Fritsch D., Beck-Jendroschek V., Tröger A., Francke W. (2014). Acyclic sesquiterpenes released by Candida albicans inhibit growth of dermatophytes. Med. Mycol. 52, 46–55. doi:10.3109/13693786.2013.814174
Crick D. C., Andres D. A., Waechter C. J. (1997). Novel salvage pathway utilizing farnesol and geranylgeraniol for protein isoprenylation. Biochem. Biophys. Res. Commun. 237 (3), 483–487. doi:10.1006/bbrc.1997.7145
Davey H. M., Hexley P. (2011). Red but not dead? Membranes of stressed Saccharomyces cerevisiae are permeable to propidium iodide. Environ. Microbiol. 13, 163–171. doi:10.1111/j.1462-2920.2010.02317.x
Dekkerova J., Cernakova L., Kendra S., Borghi E., Ottaviano E., Willinger B., et al. (2022). Farnesol boosts the antifungal effect of fluconazole and modulates resistance in Candida auris through regulation of the CDR1 and ERG11 genes. J. Fungi 8, 783. doi:10.3390/jof8080783
Dignard D., El-Naggar A. L., Logue M. E., Butler G., Whiteway M. (2007). Identification and characterization of MFA1, the gene encoding Candida albicansa-factor pheromone. Euk. Cell 6, 487–494. doi:10.1128/EC.00387-06
Dumitru R., Hornby J. M., Nickerson K. W. (2004). Defined anaerobic growth medium for studying Candida albicans basic biology and resistance to eight antifungal drugs. Antimicro. Agents Chemother. 48, 2350–2354. doi:10.1128/AAC.48.7.2350-2354.2004
Dumitru R., Navarathna D. H., Semighini C. P., Elowsky C. G., Dumitru R. V., Dignard D., et al. (2007). In vivo and in vitro anaerobic mating in Candida albicans. Eukaryot. Cell 6, 465–472. doi:10.1128/EC.00316-06
Eberhard A., Burlingame A. L., Eberhard C., Kenyon G. L., Nealson K. H., Oppenheimer N. J. (1981). Structural identification of autoinducer of Photobacterium fischeri luciferase. Biochemistry 20, 2444–2449. doi:10.1021/bi00512a013
Fairn G. D., MacDonald K., McMaster C. R. (2007). A chemogenomic screen in Saccharomyces cerevisiae uncovers a primary role for the mitochondria in farnesol toxicity and its regulation by the Pĸc1 pathway. J. Biol. Chem. 282, 4868–4874. doi:10.1074/jbc.M610575200
Gutzmann D. J., Kramer J. J., Toomey B. M., Boone C. H. T., Atkin A. L., Nickerson K. W. (2023). Transcriptional regulation of the synthesis and secretion of farnesol in the fungus Candida albicans: examination of the Homann transcription regulator knockout collection. G3 Genes, Genomes, Genet. 13, jkad172. doi:10.1093/g3journal/jkad172
Hargarten J. C., Moore T. C., Petro T. M., Nickerson K. W., Atkin A. L. (2015). Candida albicans quorum sensing molecules stimulate mouse macrophage migration. Infect. Immun. 83, 3857–3864. doi:10.1128/IAI.00886-15
Hickman M. A., Zeng G., Forche A., Hirakawa M. P., Abbey D., Harrison B. D., et al. (2013). The 'obligate diploid' Candida albicans forms mating-competent haploids. Nature 494, 55–59. doi:10.1038/nature11865
Homann O. R., Dea J., Noble S. M., Johnson A. D. (2009). A phenotypic profile of the Candida albicans regulatory network. PLoS Genet. 5 (12), e1000783. doi:10.1371/journal.pgen.1000783
Hornby J. M., Jensen E. C., Lisec A. D., Tasto J. J., Jahnke B., Shoemaker R., et al. (2001). Quorum sensing in the dimorphic fungus Candida albicans is mediated by farnesol. Appl. Environ. Microbiol. 67, 2982–2992. doi:10.1128/AEM.67.7.2982-2992.2001
Jillson O. F., Nickerson W. J. (1948). Mutual antagonism between pathogenic fungi. Inhibition of dimorphism in Candida albicans. Mycologia 40, 369–385. doi:10.2307/3755039
Katragkou A., McCarthy M., Alexander E. L., Antachopoulos C., Meletiadis J., Jabra-Rizk M. A., et al. (2015). In vitro interactions between farnesol and fluconazole, amphotericin B, or microfungin against Candida albicans biofilms. J. Antimicrob. Chemother. 70, 470–478. doi:10.1093/jac/dku374
Knobloch K., Pauli A., Iberl B., Weis N., Weigand H. (1988). “Mode of action of essential oil components on whole cells of bacteria and fungi in plate tests,” in Bioflavour ’87. Editor P. Schrier (Berlin, Germany: Walter de Gruyter), 287–299.
Langford M. L., Hasim S., Nickerson K. W., Atkin A. L. (2010). Activity and toxicity of farnesol towards Candida albicans are dependent on growth conditions. Antimicrob. Agents Chemother. 54, 940–942. doi:10.1128/AAC.01214-09
Lee J. A., Spidlen J., Boyce K., Cai J., Crosbie N., Dalphin M., et al. (2008). MIFlowCyt: the minimum information about a flow cytometry experiment. Cytometry 73 (10), 926–930. doi:10.1002/cyto.a.20623
Lewis G. M., Hooper M. E. (1943). Concurrent, combined, and consecutive fungus infections of the skin. Arch. Dermat. Syph. 47, 27–35. doi:10.1001/archderm.1943.01500190030003
Liu Z., Rossi J. M., Myers L. S. (2018). Candida albicans Zn cluster transcription factors Tac1 and Znc1 are activated by farnesol to upregulate a transcriptional program including the multidrug efflux pump CDR1. Antimicrob. Agents Chemother. 62 (11), 00968–e1018. doi:10.1128/AAC.00968-18
Lockhart S. R., Wu W., Radke J. B., Zhao R., Soll D. R. (2005). Increased virulence and competitive advantage of a/alpha over a/a or alpha/alpha offspring conserves the mating system of Candida albicans. Genetics 169, 1883–1890. doi:10.1534/genetics.104.038737
Machida K., Tanaka T. (1999). Farnesol-induced generation of reactive oxygen species dependent on mitochondrial trans-membrane potential hyperpolarization mediated by F0 F1 – ATPase in yeast. FEBS Lett. 462, 108–112. doi:10.1016/s0014-5793(99)01506-9
Machida K., Tanaka T., Fujita K., Taniguchi M. (1998). Farnesol-induced generation of reactive oxygen species via indirect inhibition of the mitochondrial electron transport chain in the yeast Saccharomyces cerevisiae. J. Bacteriol. 180, 4460–4465. doi:10.1128/JB.180.17.4460-4465.1998
Machida K., Tanaka T., Yano Y., Otani S., Taniguchi M. (1999). Farnesol-induced growth inhibition in Saccharomyces cerevisiae by a cell cycle mechanism. Microbiology 145, 293–299. doi:10.1099/13500872-145-2-293
Michaelis S., Barrowman J. (2012). Biogenesis of the Saccharomyces cerevisiae pheromone a-factor, from yeast mating to human disease. Microbiol. Mol. Biol. Rev. 76, 626–651. doi:10.1128/MMBR.00010-12
Nagy F., Vitalis E., Jakab A., Borman A. M., Forgacs L., Toth Z., et al. (2020). In vitro and in vivo effect of exogenous farnesol exposure against Candida auris. Front. Microbiol. 11, 957. doi:10.3389/fmicb.2020.00957
Navarathna D. H., Hornby J. M., Krishnan N., Parkhurst A., Duhamel G. E., Nickerson K. W. (2007). Effect of farnesol on a mouse model of systemic candidiasis, determined by use of a DPP3 knockout mutant of Candida albicans. Infect. Immun. 75, 1609–1618. doi:10.1128/IAI.01182-06
Nickerson K. W., Atkin A. L. (2016). Deciphering fungal dimorphism: farnesol’s unanswered questions. Mol. Microbiol. 103, 567–575. doi:10.1111/mmi.13601
Nickerson K. W., Atkin A. L., Hornby J. M. (2006). Quorum sensing in dimorphic fungi: farnesol and beyond. Appl. Environ. Microbiol. 72, 3805–3813. doi:10.1128/AEM.02765-05
Nikoomanesh F., Falahatinejad M., Cernakova L., dos Santos A. L. S., Mohammadi S. R., Rafiee M., et al. (2023). Combination of farnesol with common antifungal drugs: inhibitory effect against Candida species isolated from women with RVVC. Medicina 59, 743. doi:10.3390/medicina59040743
Pathirana R. U., Boone C., Nickerson K. W. (2020). Longer ubiquinone side chains contribute to enhanced farnesol resistance in yeasts. Micororganisms 8 (11), 1641. doi:10.3390/microorganisms8111641
Polke M., Sprenger M., Scherlach K., Albán-Proaño M. C., Martin R., Hertweck C., et al. (2017). A functional link between hyphal maintenance and quorum sensing in Candida albicans. Mol. Microbiol. 103, 595–617. doi:10.1111/mmi.13526
Ramage G., Saville S. P., Wickes B. L., Lopez-Ribot J. L. (2002). Inhibition of Candida albicans biofilm formation by farnesol, a quorum-sensing molecule. Appl. Environ. Microbiol. 68, 5459–5463. doi:10.1128/aem.68.11.5459-5463.2002
Semighini C. P., Hornby J. M., Dumitru R., Nickerson K. W., Harris S. D. (2006). Farnesol-induced apoptosis in Aspergillus nidulans reveals a possible mechanism for antagonistic interactions between fungi. Mol. Microbiol. 59, 753–764. doi:10.1111/j.1365-2958.2005.04976.x
Shirtliff M. E., Krom B. P., Meijering R. A., Peters B. M., Zhu J., Scheper M. A., et al. (2009). Farnesol-induced apoptosis in Candida albicans. Antimicrob. Agents Chemother. 53, 2392–2401. doi:10.1128/AAC.01551-08
Srikantha T., Daniels K. J., Pujol C., Sahni N., Yi S., Soll D. R. (2012). Nonsex genes in the mating type locus of Candida albicans play roles in a/α biofilm formation, including impermeability and fluconazole resistance. PLoS Pathog. 8 (1), 1002476. doi:10.1371/journal.ppat.1002476
Uppuluri P., Mekala S., Chaffin W. L. (2007). Farnesol-mediated inhibition of Candida albicans yeast growth and rescue by a diacylglycerol analogue. Yeast 24, 681–693. doi:10.1002/yea.1501
Verdaguer I. B., Crispim M., Hernández A., Katzin A. M. (2022). The biomedical importance of the missing pathway for farnesol and geranylgeraniol salvage. Molecules 27 (24), 8691. doi:10.3390/molecules27248691
Wente S. R., Blobel G. (1993). A temperature-sensitive NUP116 null mutant forms a nuclear envelope seal over the yeast nuclear pore complex thereby blocking nucleocytoplasmic traffic. J. Cell Biol. 123, 275–284. doi:10.1083/jcb.123.2.275
Keywords: farnesol sensitivity, haploid specific genes, mating type, Candida albicans, Saccharomyces cerevisiae
Citation: Boone CHT, Parker KA, Gutzmann DJ, Atkin AL and Nickerson KW (2023) Farnesol as an antifungal agent: comparisons among MTLa and MTLα haploid and diploid Candida albicans and Saccharomyces cerevisiae. Front. Physiol. 14:1207567. doi: 10.3389/fphys.2023.1207567
Received: 17 April 2023; Accepted: 09 November 2023;
Published: 20 November 2023.
Edited by:
Rosana Alves, University of Minho, PortugalReviewed by:
Ilse Denise Jacobsen, Leibniz Institute for Natural Product Research and Infection Biology, GermanyCopyright © 2023 Boone, Parker, Gutzmann, Atkin and Nickerson. This is an open-access article distributed under the terms of the Creative Commons Attribution License (CC BY). The use, distribution or reproduction in other forums is permitted, provided the original author(s) and the copyright owner(s) are credited and that the original publication in this journal is cited, in accordance with accepted academic practice. No use, distribution or reproduction is permitted which does not comply with these terms.
*Correspondence: Kenneth W. Nickerson, a25pY2tlcnNvbjFAdW5sLmVkdQ==
Disclaimer: All claims expressed in this article are solely those of the authors and do not necessarily represent those of their affiliated organizations, or those of the publisher, the editors and the reviewers. Any product that may be evaluated in this article or claim that may be made by its manufacturer is not guaranteed or endorsed by the publisher.
Research integrity at Frontiers
Learn more about the work of our research integrity team to safeguard the quality of each article we publish.