- Institute of Integrated Traditional Chinese and Western Medicine, Tongji Hospital of Tongji Medical College, Huazhong University of Science and Technology, Wuhan, China
The follicle is the functional unit of the ovary, whereby ovarian development is largely dependent on the development of the follicles themselves. The activation, growth, and progression of follicles are modulated by a diverse range of factors, including reproductive endocrine system and multiple signaling pathways. The Hippo pathway exhibits a high degree of evolutionary conservation between both Drosophila and mammalian systems, and is recognized for its pivotal role in regulating cellular proliferation, control of organ size, and embryonic development. During the process of follicle development, the components of the Hippo pathway show temporal and spatial variations. Recent clinical studies have shown that ovarian fragmentation can activate follicles. The mechanism is that the mechanical signal of cutting triggers actin polymerization. This process leads to the disruption of the Hippo pathway and subsequently induces the upregulation of downstream CCN and apoptosis inhibitors, thereby promoting follicle development. Thus, the Hippo pathway plays a crucial role in both the activation and development of follicles. In this article, we focused on the development and atresia of follicles and the function of Hippo pathway in these processes. Additionally, the physiological effects of Hippo pathway in follicle activation are also explored.
1 Introduction
The ovary is a complex, dynamic structure that undergoes extensive tissue remodeling throughout each reproductive cycle. This periodic remodeling includes follicular formation and atresia, ovulation, formation and regression of the corpus luteum (CL), and accompanying changes in the vascular system and microenvironment, which are essential factors for maintaining normal reproductive function (Brown and Russell, 2014; Vanselow et al., 2020). The ovarian principal role is the periodic generation and release of oocytes, alongside the secretion of steroid hormones (Gougeon, 1996). Within the ovary, the follicle represents the fundamental functional unit, characterized by different types and numbers of cells, which provide the appropriate microenvironment for oocyte development (Gougeon, 1996).
Ovarian reserve refers to the quantity and quality of remaining oocytes in the ovary, which is the basic indicator of female fertility (Practice Committee of the American Society for Reproductive Medicine Azziz et al., 2020). Human follicle development begins at the fourth month of fetal life, peaking at the fifth month of gestation (Markstrom et al., 2002). During the latter stages of embryonic development, the depletion of primordial follicles occurs rapidly through apoptosis. At birth, the total number of primordial follicles ranges between 500,000 and 1,000,000, of which only about 400 will develop into primary oocytes, ovulate and fertilize during the fertile period of the woman, and the majority of the remaining follicles are destined to atresia (Hansen et al., 2008; Findlay et al., 2015).
Although the endocrine system (consisting of the hypothalamus, pituitary and ovary) exerts a critical influence over follicular development and atresia, more and more pathways are also found to be involved in this regulation (Klionsky, 2008). The intricate interplay of signaling pathways plays a crucial role in the coordinated regulation of ovarian follicular development and subsequent ovulatory processes (Li et al., 2021a). Relevant research has identified several signaling that regulate follicular development, growth, and atresia, including adenylate cyclase (AC)/CAMP/protein kinase A (PKA), phospholipase C (PLC)/calcium/protein kinase C (PKC) (Liu and Ge, 2013), PI3K/Akt/FOXO3 (Zeng et al., 2020), Notch (Xie et al., 2017), Hedgehog (Hh) (Wijgerde et al., 2005; Ren et al., 2012)and Wnt (Castanon et al., 2012; Wang et al., 2013), members of the transforming growth factor β (TGF-β) superfamily (Monsivais et al., 2017), receptor tyrosine kinases (RTKs) (Fan et al., 2009; Chaves et al., 2010) and nuclear receptor (Hughes and Murphy, 2021). In recent years, there has been growing evidence to suggest that the Hippo pathway also plays a critical role in regulating ovarian development (Ma et al., 2019; Dey et al., 2020) (Figure 1). In this article, we explore the role of the Hippo pathway in the complex process of ovarian development.
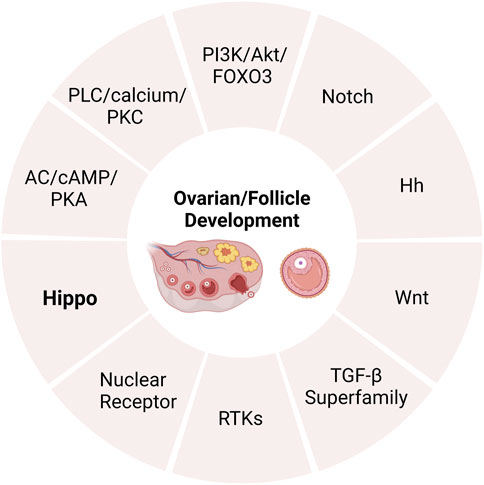
FIGURE 1. The pathways of regulating ovarian development. AC, adenylate cyclase; PKA, protein kinase A; PLC, phospholipase C; PKC, protein kinase C; TGF-β, transforming growth factor β; RTKs, receptor tyrosine kinases.
2 Ovarian development
2.1 Follicular development
Mammalian oocytes mature in the follicles, which serve as the fundamental units of the ovary. Consequently, ovarian development is fundamentally dependent on follicular development (Li et al., 2021a). Ovarian development begins in the embryonic period, during which it undergoes massive colonization of the ovaries, production of primordial germ cells (PGCs), migration of primordial germ cells to genital ridge, colonization of gonads by PGCs, gonadal sex differentiation, as well as germ cell mitosis and apoptosis (Skinner, 2005; Matsuda-Minehata et al., 2006). After differentiation of PGCs, oocytes begin to develop in the embryo.
First, proliferating PGCs migrate to the nascent genital ridge, differentiate into oogonia, which are encapsulated by a single layer of follicular epithelium or granular cells (GCs), and subsequently enter meiosis to become primary oocytes (McLaughlin and McIver, 2009). The primordial follicle is the most basic follicular structure, composed of an oocyte enclosed by a few flattened granulosa cells and basal layer (Hirshfield, 1991). With the regulation of intraovarian growth factors in the ovary and secretion of pituitary gonadotropins, follicles grow a gonadotropin-independent phase to a gonadotropin-dependent phase (Hsueh et al., 2015; Gershon and Dekel, 2020). The transformation of follicles from primordial to primary stage is accompanied by a rapid increase in the cytoplasmic and nuclear volume of oocytes, and changes in the morphology and proliferation of GCs. As follicles develop from primary to secondary stages, GCs around oocytes continue to proliferate and increase in size, and theca cells (TCs) layers begin to form (Hirshfield, 1991). TCs proliferates from undifferentiated mesenchymal cells in ovarian stroma and is a layer of cells close to the basal layer of the follicle (Richards et al., 2018). It is composed of the inner membrane, which contains endocrine cells, and the outer membrane, which is a fibrous connective tissue layer composed of fibroblast-like cells. TCs are the critical component of the ovarian follicle, serving as the source of androgens, Which are subsequently converted into estrogens by GCs (Magoffin, 2005). The TCs layer also contains vascular tissue and immune cells (Young and McNeilly, 2010). As the secondary follicles develop into preantral follicles, the sinus cavity begins to form, the preantral follicles are characterized by multi-layered cube GCs, which undergo continuous proliferation during follicular development, and the TCs layer provides a self-contained blood supply to the growing follicles. In the preantral follicles, TCs exhibit a response to luteinizing hormone (LH), while the differentiated GCs express follicle stimulating hormone (FSH) receptors that facilitate the production and secretion of estradiol (E2) (Edson et al., 2009). GCs of growing antral follicles competitively bind to FSH and grow into dominant follicles. E2 reaches its peak in mature follicles to lead a surge in LH levels, which are necessary for ovulation to occur (de Ziegler et al., 2007). After ovulation, the residual GCs and TCs of the collapsed follicle undergo luteinization and angiogenesis, which forming CL and promoting the secretion of progesterone and other hormones, thereby establishing pregnancy and maintaining early embryonic development (Figure 2).
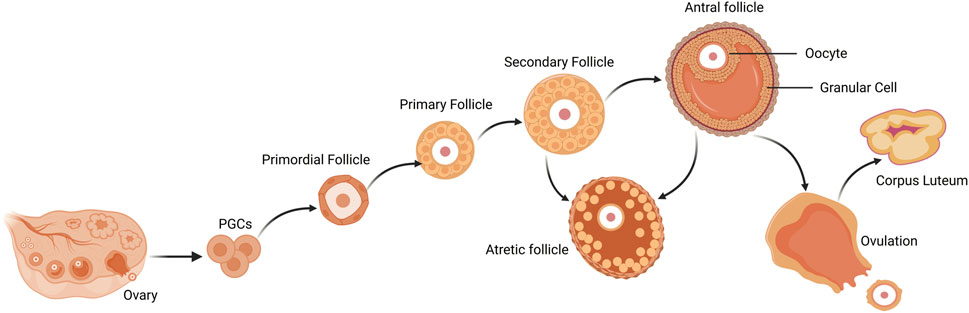
FIGURE 2. Follicle development and atresia, ovulation of the mammalian ovary. Primordial follicles are activated to become primary follicles after colonization and differentiation of PGCs, which further develop into secondary follicles. Secondary follicles continue to grow into antral follicles. After ovulation, mature follicles degenerate into corpus luteum. During follicular growth and development, most follicles undergo a degenerative process of atresia. PGCs, primordial germ cells.
2.2 Generation of oocytes
During follicular growth, the volume of oocytes can increase by 100–300 times and stop at the preantral stage. Furthermore, it has been observed that the quantity of granulosa cells surrounding oocytes exhibits a gradual increase (Keefe et al., 2015), and at this stage, oocytes commence the accumulation of macromolecules such as glycogen, lipid droplets, proteins, and mRNA, in anticipation of their later developmental processes (Sturmey et al., 2009). The oocytes exhibit a high level of synthetic activity, which induces structural changes and the redistribution of the endoplasmic reticulum and Golgi complex. These changes result in the increased number of vesicles and ribosomes and the formation of cortical granules. Additionally, the zona pellucida initiates the secretion of glycoproteins (Picton et al., 1998). Mitochondria are abundant in oocytes and are essential for oocyte maturation, fertilization and subsequent embryogenesis (Kirillova et al., 2021). Mitochondria are unique in that they possess own genetic information except the nucleus. The genetic material in the mitochondria is called mitochondrial deoxyribonucleic acid (mtDNA) (Kukat et al., 2011). Unlike mitochondria in differentiated somatic cells that are mature and form a highly organized network, mitochondria in oocytes display unstructured cristae and limited capacity to generate energy (Trebichalska et al., 2021). During oocyte maturation, the number of mtDNA copy increased significantly. There are two turning points in the rapid increase of mtDNA copies: the initial transition from primordial to primary follicles, and the second transition from the germinal vesicle (GV) stage to the oocyte metaphase II (MII) in antral follicle development. PGCs contain about 200 mtDNA copies, while mature oocytes have about 4 million copies (Santos et al., 2006). At the second turning point of rapid mtDNA copy, a significant change in the distribution of mitochondria occurs, as they move from the center of the ooplasm to the pericortical region and are distributed throughout the ooplasm (Trebichalska et al., 2021). ATP levels also increase significantly as oogenesis progresses (Kirillova et al., 2021). The maturation of oocytes is reliant on the generation of ATP, which is essential for subsequent transcription and translation. Insufficient levels of ATP can cause meiosis errors and result in alterations in chromosome number, culminating in genetic disorders (Dalton and Carroll, 2013).
The maturation of oocytes includes cytoplasmic and nuclear maturation, which must be completed to enable gametes to obtain developmental ability (Eppig, 1996). The nuclear maturation of oocytes involves the following steps: nuclear changes associated with the restoration of meiosis I and the extrusion of the first polar body before MII stops, decondensation of sperm chromatin, formation of the pronucleus, and provision of support for the first division of the embryo. In addition, cytoplasmic maturation during oocyte growth is critical for mRNA post-transcriptional modification, protein synthesis, and regulation of post-translational modification. In the late stage of oocyte development, LH surge promotes the selection and final growth of ovulation follicles, and triggers the expansion of cumulus-oocyte complexes and the loss of gap junctions, eventually leading to oocyte maturation and ovulation. The gradual acquisition of developmental ability during oocyte growth has been well established (Lonergan and Fair, 2016).
It is well known that oocytes not only provide genetic information to developing embryos, but also provide them with energy, nutrients, and mtDNA sets (Kopeika et al., 2015). The differences in embryonic developmental competence can be attributed to changes in mtDNA content, oocytes with low mtDNA copy numbers showing lower developmental potential and thus affecting embryonic viability (Reynier et al., 2001; Van Blerkom, 2011; Ge et al., 2012). Compared with nuclear DNA (nDNA), mtDNA has some special characteristics. It is highly susceptible to the deleterious effects of reactive oxygen species (ROS) and is particularly easy to mutations that lead to functional degradation (Eichenlaub-Ritter et al., 2011). In recent years, oocyte cryopreservation has been widely used in assisted reproductive technology (ART), which can store excess oocytes and preserve fertility for women (Stigliani et al., 2015). At present, slow freezing and vitrification are most commonly used in oocyte cryopreservation (Gardner et al., 2007; Smith et al., 2010). However, slow freezing leads to excessive production of ROS, which affects some key oocyte organelles (such as mitochondria), and mtDNA is damaged by ROS produced within the mitochondrial respiratory chain, further affecting oocyte development and embryo implantation (Ebert et al., 1988; Abedelahi et al., 2008; Kagawa et al., 2009; McCarthy et al., 2010; Farzollahi et al., 2016; May-Panloup et al., 2016). Monzo et al. (2012) examined gene expression profiles in slow-frozen and vitrified oocytes, slow freezing is more detrimental to oocyte developmental competence than vitrification, and it may be the cause of the reduced implantation and pregnancy rates of human oocytes. The indications and frequency of oocyte vitrification in the field of ART are expanding globally (Barberet et al., 2022). It has been demonstrated that the addition of vitrification raises the survival and pregnancy rates of embryo transfer during ART (Cobo et al., 2019). Potdar et al. (2014) found that fresh and vitrified oocytes both responded admirably in terms of fertilization rates, ongoing pregnancy rates, and clinical pregnancy rates. Nottola et al. (2009) further showed that vitrified oocytes generally maintained mitochondrial fine structure after rewarming, with round or oval mitochondria ranging from 0.5 to 0.8 µm in diameter. Regarding the effect of vitrification on genetic integrity and mtDNA carried by oocytes, Ana Arnanz et al. (2020) showed that vitrification of oocytes had no discernible impact on the mtDNA content of trophectoderm biopsy specimens and there was no noticeable distinction in the total rate and mtDNA content between fresh and vitrified blastocysts. It has been reported that the smaller the volume of cryopreservation carrier, the faster the cooling rate, and the higher the cryoprotectant agent concentrations (CPAs), the higher the success rate of vitrification of oocytes (Arav, 2014; Best, 2015). Cobo et al. (2019) found no difference in terms of fertilization rate, day 2 and day 3 cleavage, and blastocyst formation between vitrified and fresh oocytes using the Cryotop method, and the embryo quality was similar on days 3 and 5–6 in vitrified and fresh oocyte groups. Wu et al. (2017) found that LHe vitrification improved the survival of immature bovine oocytes by lessening the deleterious effects of cryodamage on the ultrastructure of certain organelles and the expression of some associated genes as compared to LN vitrification. Zhang et al. (2020) further showed that low vitrification temperatures (VTs) and CPAs could increase blastocyst rate by altering mRNA levels of apoptotic and mitochondrial genes. In conclusion, optimizing the vitrification procedure, setting the appropriate VTs and CPAs, vitrification can better maintain the integrity of oocytes and mtDNA content, which provides a good technical guarantee for the preservation of germ cells during ART.
2.3 Atresia of follicles
During follicular development, most follicles undergo a degenerative process of atresia, which makes the primordial follicles continuously depleted (Manabe et al., 2008). Follicular atresia is inhibited by FSH, which is conducive to follicular development. Follicular maturation promotes the secretion of estrogen, which in turn inhibits the secretion of FSH. Follicular atresia is an apoptotic process regulated by threshold-dependent hormones, and the most important part is the apoptosis of GCs (Kaipia and Hsueh, 1997). In the early atretic follicles, apoptotic granulosa cells begin to appear and their number gradually increases. In the progressing atretic follicle, most granulosa cells undergo apoptosis and the granular layer shows severe destruction (Matsuda et al., 2012). Deprivation of critical pro-survival factors or exposure of death ligands are the major causes of apoptosis, both of which lead to the activation of caspase-3, and then participate in the apoptosis of GCs (Matsuda et al., 2012). Follicular atresia is a complex phenomenon involving multiple ligand systems associated with apoptosis, such as FAS ligands, tumor necrosis factor-alpha (TNF-α), and TNF-α-related apoptosis-inducing ligands (Matsuda-Minehata et al., 2006; Quirk et al., 2006; Jaaskelainen et al., 2009). In addition, BCL2 family members regulate germ cell and somatic cell apoptosis by regulating mitochondrial-mediated apoptosis. It plays an important role in follicular atresia (Hsu et al., 1996).
3 Hippo pathway and its function
The Hippo pathway, a serine/threonine kinase signaling cascade, is an evolutionary conserved pathway first discovered through screening and identification in Drosophila melanogaster (Ma et al., 2019). In mammals, Hippo pathway comprises a set of core components (Avruch et al., 2012), including Mammalian STE20-like kinase 1/2 (MST1/2), Salvador protein 1 (SAV1), Large tumor suppressor kinase 1/2 (LATS1/2), Mps one binder 1A/B (MOB1A/B), yes-associated protein (YAP), transcriptional co-activator with PDZ-binding motif (TAZ) and the transcriptional enhanced associate domain (TEAD) family (Hong et al., 2018).
The activation of the Hippo kinase cascade can be modulated directly or indirectly by multiple upstream signals, including cell polarity, density, stress signals, and soluble factors (Stein et al., 2015). Mitogen activated protein 4 kinase (MAP4K) regulates the activation of MST1/2 and LATS1/2 for phosphorylation and inhibition of YAP/TAZ (Chen et al., 2019). Mechanical stress, such as the stiffness of the extracellular matrix (ECM), are also effective regulatory factors for YAP/TAZ, as the attachment of cells to the tough ECM activates the Rho-GTP enzyme to inhibit the phosphorylation of LATS dependent YAP (Chang et al., 2018). The regulation of the Hippo pathway involves multiple mechanisms, one of which is the activation of G protein-coupled receptors (GPCRs). GPCRs that are coupled to Gα12/13 and Gαq/11, such as lysophosphatidic acid (LPA), sphingosine 1-phosphate (S1P), induce the activation of Rho GTPase, and subsequently increase the levels of filamentous actin (F-actin), which promote the proliferation and migration of epithelial cells on the ovarian surface (Luo and Yu, 2019). Integrin signaling is a crucial factor in regulating the activity of YAP/TAZ in 3D, and it has been found to be associated with differentiation of cultured skeletal stem cells and proliferation of malignant cells (Aragona et al., 2013; Tang et al., 2013). The Hippo pathway is susceptible to inhibition by growth factors such as insulin-like growth factor 1 (IGF1) and epidermal growth factor (EGF) ligand families, which activate RTKs leading to the activation of PI3K/Akt signaling pathway, which further inactivates hippo kinase complex, resulting in increased YAP expression level (Zhou et al., 2020).
The activation of the Hippo pathway initiates with the activation of MST1/2 (Chen et al., 2019). MST1/2 forms a heterodimer with SAV1 via its C-terminal SARAH domain, promoting the phosphorylation of SAV1, MOB1 and LATS1/2, as well as the association between MOB1 and LATS1/2 (Karchugina et al., 2021). The phosphorylation of YAP and TAZ at several sites by LATS1/2 is a direct inhibition mechanism for their nuclear translocation (Qi et al., 2022). Further phosphorylation of YAP/TAZ by casein kinase 1 can lead to β-trcp-mediated ubiquitination and proteasome degradation, thereby inhibiting tissue growth and cell proliferation (Liu et al., 2010; Plouffe et al., 2016). Upon the disruption of the Hippo pathway, YAP/TAZ dephosphorylates and translocates into the nucleus to function as a transcription co-activator (Li et al., 2021b; Ito et al., 2021). The binding of YAP/TAZ with transcription factor TEAD facilitates the expression of CCN2/connective tissue growth factor (CTGF) and baculoviral inhibitors of apoptosis repeat containing (BIRC), and then organ size is regulated by cell proliferation, apoptosis and self-renewal of stem cells (Avruch et al., 2012; Clark et al., 2022) (Figure 3).
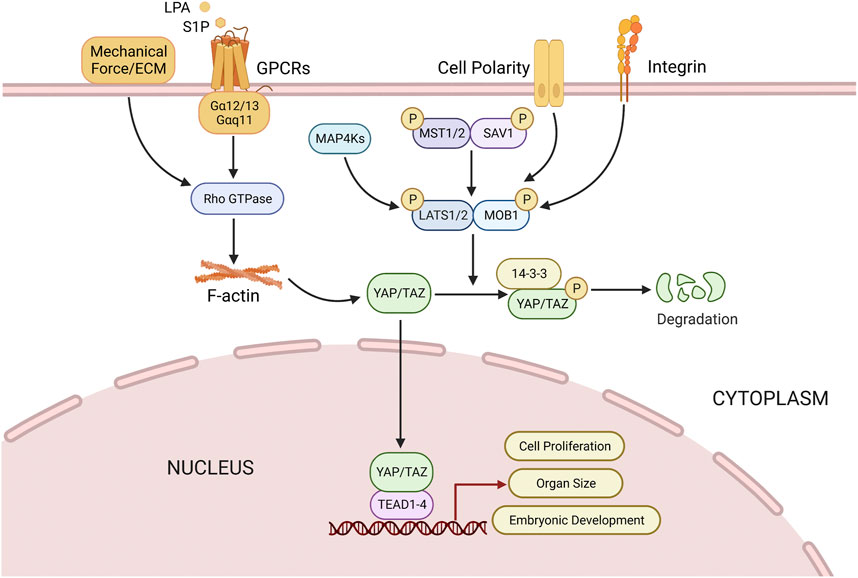
FIGURE 3. Regulation of Hippo pathway. Multiple factors (Mechanical force, Small molecule soluble substances (LPA, S1P), cell polarity, integrins) can directly or indirectly modulate Hippo pathway, thus regulating cell proliferation, organ size, and embryonic development, etc. See text for details. LPA, lysophosphatidic acid; S1P, sphingosine 1-phosphate.
Hippo pathway is involved in a wide range of biological processes that are mainly mediated by the activity of YAP (Russell and Camargo, 2022). These processes include cell proliferation and differentiation, organ growth, embryonic development, and tissue regeneration (Yu et al., 2015). Disorder of the Hippo pathway has been implicated in various diseases, ranging from cancer to ophthalmic, heart, lung, kidney, liver and ovarian diseases, as well as immune dysfunction (Wang et al., 2020). Therefore, Hippo pathway and YAP protein activity may be the key to the treatment of various diseases (Misra and Irvine, 2018).
4 Hippo pathway in ovarian development
The Hippo pathway is involved in regulating essential biological processes like cell proliferation, migration, and differentiation. It also has a critical role in follicle growth and activation, as well as steroidogenesis (Clark et al., 2022). The disorder of Hippo pathway will lead to the loss of follicular homeostasis and reproductive disorders, resulting in a variety of diseases, such as polycystic ovary syndrome (PCOS), premature ovarian failure (POF) and ovarian cancer (Hu et al., 2019a; Siegel et al., 2020; Chang and Dunaif, 2021).
4.1 The Hippo pathway regulates follicular development and oogenesis
In mouse and human ovaries, key hippo pathway components (YAP, TAZ, MST1/2, SAV1, and LATS1/2) are expressed in follicles at different stages (Kawamura et al., 2013). Correlational studies have shown that the key components of Hippo pathway, MST1/2, LATS1/2, YAP and phosphorylated YAP (p-YAP), are expressed in oocytes, GCs and TCs from primary to sinus follicular stages, as well as in atretic follicles and corpus luteum (Kawamura et al., 2013; Hu et al., 2019b; Sun et al., 2021). During follicular development, the expression of these molecules in oocytes changes dynamically. De Roo et al. (2020) showed that MST1 progressively translocated from oocyte cytoplasm of primordial and primary follicles to the oocyte nucleus of secondary follicles. In addition, Lv et al. (2020) showed that YAP was located in the nucleus of primary to preovulation follicular granulosa cells, and after ovulation, it is mainly located in the cytoplasm of differentiated corpus luteum cells. However, Sun and Diaz (2019) found that YAP1 was mainly localized in the nucleus in the co-culture of GC and oocytes (Table 1).
The upstream negative regulators of the Hippo pathway, including MST1/2, SAV1 and LATS1/2, have been shown to regulate the growth, development and function of the mammalian ovary (Tsoi et al., 2019). At present, the role of Hippo pathway component MST1/2 in follicular development is less studied (Lyu et al., 2016). Studies utilizing avian follicles as models have demonstrated that mRNA expression levels of SAV1 are highest in small follicles (<1 mm), and decreased with the development of follicles. Silencing the Sav1 gene by small interfering RNA (siRNA) can disrupt the Hippo pathway, leading to elevated YAP activity and subsequently promote proliferation of granulosa cells (Lyu et al., 2016). LATS1/2 is an important regulatory factor of the Hippo pathway. Sun et al. (2021) observed the regulatory role of LATS2 in the development of chicken ovary and found that LATS2 was mainly located in oocytes and undifferentiated GC of pre-layered follicles of chicken ovary, and the expression of Lats2 mRNA in smaller follicles and GC was significantly higher than that in larger follicles. High expression of LATS2 inhibits GC proliferation and differentiation, follicle selection, and mRNA and protein expression of biomarker related genes for steroid-production, including FSH receptor (FSHR), steroidogenic acute regulatory protein (STAR), estrogen receptor (ESR), etc. (Sun et al., 2021). At the same time, Silencing the Lats gene by siRNA can lead to enhanced proliferation of granulosa cells and increased expressions of FSHR, STAR and ESR (Vanselow et al., 2020).
YAP is an effector molecule downstream of Hippo pathway and plays a pivotal role in ovarian development (De Roo et al., 2017). Hu et al. (2019b) found that the expression of the core components of Hippo pathway changed with follicle development by observing the expression of the major components of Hippo pathway in the ovaries of mice at 3 days, 1, 5, and 16 months postnatally. The expression of MST1 and LATS2 decreased significantly with increasing age of the mice, while the expression levels of YAP1 and p-YAP showed the opposite trend (Hu et al., 2019b). It has been observed that the expression of YAP in mouse ovarian germ cells during embryonic development is negligible at 13.5 days of gestation but increases gradually in pups at 15.5, 18.5, and 2 days of gestation (Abbassi et al., 2016). All these results suggested that the expression of YAP in mouse ovary increases with the growth of follicles (Hu et al., 2019b; Abbassi et al., 2016). In the cultured mouse ovary in vitro, the low expression of yap inhibited follicle growth, whereas overexpression of Yap resulted in follicle activation as indicated by decreased primordial follicle number and increased secondary follicle number (Hu et al., 2019b; Xiang et al., 2015; Lv et al., 2019). These studies have shown that YAP1 mainly induces the transition of follicles from primordial to primary stages (Gershon and Dekel, 2020). A recent investigation demonstrated that the conditional knockout of the Yap gene in granulosa cells of mice resulted in abnormal ovarian follicle development, decreased ovarian volume, augmented follicular atresia, as well as reduced litter size and number of offspring (Lv et al., 2019). The overexpression of Yap also increased the thickness of ovarian surface epithelium in chemotherapy-induced infertile mice, promoted follicular recruitment and increased the live birth rate (Devos et al., 2020). Therefore, the Hippo pathway effector YAP plays an important role in follicular development and function.
The appropriate growth and maturation of oocytes is essential for successful reproduction in mammals, and these processes are dynamically coupled (Shah et al., 2018). YAP1 has been discovered in mature oocytes using single-cell transcriptome analysis of oocytes taken from in vitro fertilization patients (Yan et al., 2013). YAP1 is not intrinsically involved in mouse oogenesis (Abbassi et al., 2016). Oocyte-specific deletion of Yap1 had no effect on folliculogenesis, oocyte maturation, spindle organization or fertilization (Yu et al., 2016). Sun and Diaz (2019) found that prior to ovulation, oocytes inhibit the Hippo pathway to activate YAP1 and increase granulosa cell survival and proliferation, while inhibiting cell differentiation, and these effects are reversed by ovulation signaling, which first inhibits YAP1 and then leads to YAP1 degradation, thereby promoting cell differentiation. Nuclear YAP has no significant physiological role during mammalian oocyte development (Abbassi et al., 2016). Multiple mechanisms synergistically act to prevent YAP accumulation in the oocyte nucleus (Basu et al., 2003). PKA can phosphorylate LATS kinase and promote S112 to phosphorylate YAP to anchor it in the cytoplasm (Abbassi et al., 2016). It is clear that the Hippo signaling pathway controls the development of follicles and the maturation of oocytes in ovarian somatic cells as opposed to germ cells (Clark et al., 2022). However, oocyte Yap1 is essential for embryonic development after fertilization (Sha et al., 2020). The abundance of F-actin bundles in the trophectoderm of early mammalian embryos is linked to higher YAP activity and cell proliferation (Slager et al., 1992).
During oogenesis, the oocyte is responsible for establishing the polarity of the egg and embryo along the anterior-posterior (AP) and dorsal-ventral axes, and follicular cell differentiation along the AP axis is a critical step in the normal development of the egg chamber and the establishment of oocyte polarity (Wong et al., 2015). Yu et al. (2008) showed that the posterior follicle cell (PFC) of Drosophila require the Hippo pathway and its downstream target Yorkie (Yki) to form AP axis polarity of oocyte compartment. Several signaling pathways in the polar follicle cells (PFCs) are essential for the establishment of dorsal-ventral polarity during mid-oogenesis. These pathways include JAK/STAT, EGFR, Notch, and Hippo (Wong et al., 2015). One of the fundamental events during oocyte maturation and meiosis is cell polarization (Pennarossa et al., 2021). In non-polarized cells, angiomotin (AMOT) is phosphorylated and activates NF2 and LATS1/2 kinases, promoting YAP/TAZ phosphorylation and inducing their cytoplasmic retention (Zhao et al., 2011; Li et al., 2015a). In contrast, in polarized cells, AMOT is sequestered by the PAR-aPKC system, leading to dephosphorylation of YAP/TAZ, with consequent nuclear accumulation (Hirate and Sasaki, 2014).
4.2 Effect of hippo pathway on ovarian endocrine secretion
The abnormality of Hippo pathway leads to the abnormal secretion of ovarian gonadotropin levels and other hormones. Related studies revealed that deletion of Lats1 in female mice led to increased ovarian weight, loss of granulosa cell function, and reduced serum levels of LH, prolactin, and progesterone, while FSH levels were not affected (Tsoi et al., 2019; Menard et al., 2020). Hormone-dependent E2 production is critical for the proper functioning of granulosa cell and contributes to the regulation of the reproductive cycle (Chauvin et al., 2022). A study utilizing siRNA to silence the Yap1 gene in bovine granulosa cells in vitro has demonstrated a marked decrease in FSH-induced E2 production, with an 80% reduction observed in comparison to the control group (Plewes et al., 2019). Similarly, the stimulation with testosterone and E2 was observed to elevate the expression and function of YAP (Ji et al., 2017).
LH and FSH are heterodimeric glycoproteins that share a common α-subunit and differ in their respective β-subunits [LHβ (Lhb) and FSHβ (Fshb)]. The transcription of Fshb is mainly regulated by TGFβ superfamily ligands, the most prominent of which is activin (Kumar, 2018). Activin binding to FSHR promotes intracellular phosphorylation of SMAD2 and SMAD3 proteins, which form a complex with SMAD4 and translocate to the nucleus. In combination with forkhead box L2, the SMAD2/3/4 complex functions as a transcription factor to promote the transcription of Fshb (Li et al., 2017). Related studies showed that the Hippo pathway interacted with the function of TGFβ/activin of receptor-regulated SMAD, and in epithelial cells, high cell density promoted the phosphorylation of YAP/TAZ and binding to the TGFβ-induced SMAD2/3/4 complex, which led to its cytoplasmic segregation and inhibition of TGFβ signaling (Grannas et al., 2015). Furthermore, the SMAD complex can regulate the transcription of Lhb, Ariane et al. showed that YAP/TAZ act as inhibitors of basal gonadotropin secretion (Lalonde-Larue et al., 2022). YAP/TAZ play a vital role in the regulation of Fshb and Lhb transcription.
The Hippo and ERK pathways may potentially converge in the regulation of ovarian function (Fan et al., 2009). Ji et al. (2017) observed an increase in the expression of phosphorylated MST and a decrease in the expression of YAP1 in granulosa cells of ERK1/2-deficient superovulatory mouse model, while no significant changes in the expression of YAP1 were observed. In various cell types, MAPKs co-regulate LATS1/2 with MST1/2 (Meng et al., 2015). Pre-ovulatory LH surge leads to activation of the RAS-ERK1/2 signaling cascade in the ovary. RAS signaling is critical for the inactivation of the Hippo pathway, and it has been shown that EGFR-RAS-RAF-MEK-ERK-mediated interactions can regulate the Hippo pathway (Zinatizadeh et al., 2021). In vitro treatment of bovine granulosa cells with vetiporfin, a YAP1/TEAD inhibitor, the downregulation of CTGF downstream of YAP1 and a decrease in epidermal growth factor receptor mRNA abundance were observed (Dos Santos et al., 2022). The Hippo pathway plays a critical role in regulating cell differentiation and thus regulates the onset of gonadotropin-dependent ovulatory response (Clark et al., 2022).
4.3 Application of disrupting hippo pathway in follicular activation
Mechanical signals from ECM, cell adhesion sites and shape, and actin cytoskeleton can regulate Hippo pathway, thereby regulating cell proliferation and differentiation (Dasgupta and McCollum, 2019) (Figure 4). Cultivating mesenchymal stem cells (MSCs) in a high hardness ECM can increase the activity of YAP and promote bone formation, while cultivating in a soft matrix can reduce the activity of YAP and promote adipogenesis (Mohri et al., 2017). Recent clinical studies have shown that ovarian cortical tissue fragmentation followed by autologous transplantation can activate follicles, increase serum E2 levels in patients with decreased ovarian reserve function, and thereby improve fertility (Fabregues et al., 2018; Lunding et al., 2019). In the ovary, mechanical stimuli such as fragmentation, incision, drilling, or wedge resection lead to actin polymerization, thereby blocking Hippo signal transduction. Studies have shown that F-actin formation in stress fibers is necessary for Hippo pathway blockade and YAP nuclear localization (Wada et al., 2011). After follicular fragmentation, YAP is predominantly expressed in the nuclei of oocytes and GCs in ovarian wedge sections. The nuclear YAP interacts with TEAD transcription factors, leading to increased expression of downstream CTGF and BIRC, which ultimately promotes the proliferation of granulosa and membrane cells, thus facilitating follicular growth (Hsueh and Kawamura, 2020). In addition, coculture of broken ovaries with protein kinase B (PKB/AKT) activators can promote the growth of secondary and sinus follicles. In contrast, the use of AKT inhibitor MK2206 can inhibit follicular growth, and overexpression of Yap1 can partially offset the inhibition of follicular growth caused by MK2206 (Hu et al., 2019b).
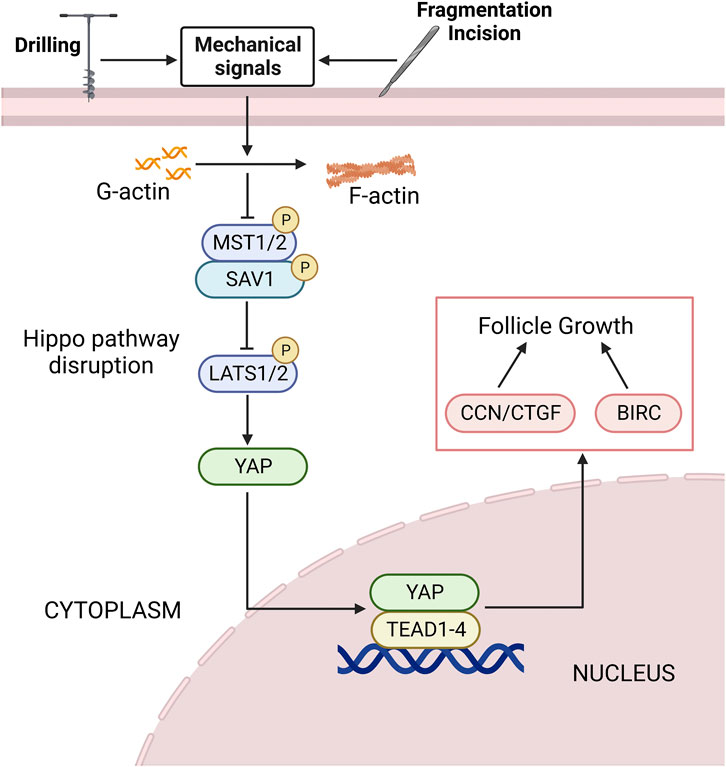
FIGURE 4. The Hippo pathway is disrupted by mechanical signaling. Mechanical signals such as ovarian fragmentation and incision, drilling can promote actin polymerization, thereby disrupting the Hippo pathway. The expression level of upstream negative regulators of the Hippo pathway is decreased, and the nuclear localization of YAP is increased. It binds to the TEAD1-4 to promote the expression of downstream CCN/CTGF and BIRC, thereby promoting follicle growth and development. See text for details. YAP, yes-associated protein; TEAD, transcriptional enhanced associate domain; CTGF, connective tissue growth factor; BIRC, baculoviral inhibitors of apoptosis repeat containing; G-actin, global-actin; F-actin, filamentous actin.
Extensive studies have shown that disrupting the Hippo pathway by mechanical signaling can activate follicles (Table 2). Cheng et al. (2015) treated mouse ovaries with actin polymerization agent S1P, and after treatment, it was found that F-actin expression was increased, and the expression of YAP and CTGF in Hippo pathway nucleus was increased, and short-term treatment and retransplantation of ovaries with S1P in vitro can promote follicular growth, Pors et al. (2020) confirmed this result later. Hu et al. (2019b) cultured the ovaries of mouse pups in vitro and detected the changes in the components of Hippo pathway during culture. The findings demonstrated that Hippo-YAP1 signaling pathway played a crucial role in the activation of primordial follicles in mice. Devos et al. (2020) cut and cultured ovaries and found that ovarian fragmentation disrupted the Hippo pathway and promoted YAP recruitment in oocytes and GCs nuclei. Kawamura et al. (2013) conducted allogeneic transplantation after ovarian rupture in mice, and found that the expression of F-actin increased, resulting in the blocking of Hippo pathway. They observed a decrease in phosphorylated YAP, an increase in nuclear localization of YAP, and an increase in downstream growth factors and apoptosis inhibitors, thereby promoting follicular growth and oocyte maturation. Grosbois and Demeestere (2018); Masciangelo et al. (2020) further cultured frozen-thawed ovarian cortical fragments from clinical patients in vitro and found that ovarian fragmentation resulted in the translocation of Hippo effector YAP to the granulosa nucleus, promoted the upregulation of downstream targets BIRC1 and CCN2, and led to increased follicle growth near the incision site. De Roo C et al. presented evidence of Hippo pathway involvement in primordial follicle activation in vitro, specifically during the period from day 0 to day 4 (De Roo et al., 2020). Therefore, blocking the Hippo pathway is an important link in follicular activation, and ovarian tissue rupture and retransplantation can activate follicles. This discovery provides ideas for the clinical treatment of early-onset ovarian insufficiency and POF (Lunding et al., 2019). However, Lunding et al. (2020) found that actin polymerization, the expression of YAP and downstream CCN2 were not increased after in vitro fragmentation of frozen ovarian cortex, suggesting that fragmentation is likely to be ineffective to activate follicle growth in human ovarian cortex. This indicated that the effect of Hippo pathway in the process of follicle activation may be spatiotemporal and thus difficult to detect its changes with fewer investigation timepoints. More studies are needed to verify the regulatory role of F-actin and Hippo pathway in human ovarian tissue fragmentation.
4.4 Hippo regulates ovarian development by influencing other pathways
It has been reported that several coordinated signaling pathways are interconnected to regulate ovarian folliculogenesis, starting with the dormant primordial follicle and ending with the fully mature and normally formed oocyte ready for fertilization (Jones and Shikanov, 2019). As the main effectors of the Hippo pathway, YAP1/TAZ are crucial for granulosa cell proliferation and follicle development, and the specific loss of YAP in GCs increases cell apoptosis and leads to subfertility (Hsueh and Kawamura, 2020). YAP1/TAZ are involved in the regulation of several signaling pathways including PI3K/Akt, Wnt, TGFβ, Hh, Notch, and IGF, and act in concert to control follicular development (Vanuytsel et al., 2013).
PI3K/Akt/mTOR is a pathway regulated by YAP that regulates cell size, tissue growth and proliferation (John et al., 2008). Activation of the PI3K/AKT pathway can lead to FOXO3 phosphorylation and nuclear export, further inducing primordial follicle activation, which results in rapid depletion of the follicle pool and subfertility (Reddy et al., 2010; Adhikari et al., 2012). In mammalian ovary, FOXO3 is located in GCs at all stages of follicular development and plays a designated role in proliferation, apoptosis and differentiation of them, as well as oocyte maturation, maintaining primordial follicle quiescence, thereby inhibiting follicle activation to increase ovarian reserve (Castrillon et al., 2003; Cunningham et al., 2004). Devos et al. (2020) confirmed that in vitro follicle activation is mainly involved in PI3K/AKT/mTOR and Hippo pathways, and both can be regulated by mTORC1 inhibitors, indicating a link between the two pathways. Loss of Phosphatase and tensin homolog (PTEN) in mice promotes the expression of Akt and/or mTOR (Tanaka et al., 2012). In human gastric cancer cell lines, PTEN knockdown increased the nuclear localization of YAP (Xu et al., 2018). The ability of Everolimus acting downstream of AKT to prevent 4-hydroperoxycyclophosphamide-induced Ccn2 expression suggests an alternative connection between the two pathways, and the specific mechanism is not clear but it has been shown that mTORC2 may directly activate Rho protein, a small G protein that regulates cell shape by stimulating actin polymerization into filaments and ultimately disrupting the Hippo pathway (Makker et al., 2014). In addition, Borreguero-Munoz et al. (2019) suggested that follicle cells of drosophila must receive an insulin/IGF-1 signal that activates PI3K-PDK1-Akt to inhibit Hippo kinase, possibly through Akt phosphorylation or other mechanisms to be elucidated. LNK is an important regulator of insulin signaling pathway and can promote granulosa cell apoptosis in PCOS by negatively regulating the insulin-stimulated PI3K/AKT/FOXO3 pathway (Luo, 2017). Pre-follicular development is significantly initiated when PTEN inhibitors or PI3K activators are added to human ovarian tissue (McLaughlin et al., 2014; Novella-Maestre et al., 2015). The interaction of Hippo and Akt has been used for in vitro activation (IVA) of follicles, ovarian fragmentation (Hippo signaling disruption) followed by IVA drug treatment (AKT stimulation). Successful pregnancies have been reported after transplantation of small ovarian fragments exposed to PI3K/Akt activators into patients diagnosed with POF (Kawamura et al., 2013; Suzuki et al., 2015).
The canonical Wnt/β-catenin signaling pathway is a cellular communication system that plays a pivotal role during embryogenesis (Steinhart and Angers, 2018). TGF-β cytokine family, such as TGF-β, bone morphogenetic protein (BMP), and activin, which can regulate a variety of biological activities in various cell types and at different developmental stages (Luo, 2017). Hippo signaling can interect with Wnt/β-catenin and TGF-β signaling to control cell growth, and its regulators YAP/TAZ locate at the crosstalk between Wnt/β-catenin and TGF-β/Smads, which provides the transcriptional response, and these responses converge on the transcription of target genes that function in the nucleus (Attisano and Wrana, 2013). YAP/TAZ is trapped in the cytoplasm while the Hippo pathway is active, which prevents Wnt/β-catenin and TGF signals (Attisano and Wrana, 2013) (Figure 5). Similarly, Varelas et al. (2010) also showed that cytosolic TAZ is a negative regulator of the Wnt pathway, and elimination of LATS expression leads to nuclear accumulation of TAZ, reduced TAZ-Dishevelled (DVL) interaction, enhanced DVL phosphorylation, and promotes Wnt pathway activation in cells, ultimately leading to elevated β-catenin levels. β-catenin is a crucial molecule in the Wnt pathway (Li et al., 2021a). In the mammalian intestinal canal, an increase expression of YAP1 induces nuclear β-catenin accumulation, thereby activating the Wnt signal and maintaining crypt stem cell proliferation and stemness (Camargo et al., 2007; Zhou et al., 2011). Previous studies have also shown that β-catenin activation can promote FSH-mediated effects in ovarian follicle cells (Wang et al., 2010; Hernandez Gifford, 2015). Wang et al. (2013) showed that WNT2 regulates DNA synthesis in mouse granule cells via β-catenin (Wang et al., 2013). In addition, overexpression of Wnt2 in GCs promoted cell proliferation and increased β-catenin levels in the cytoplasm and nucleus (Wang et al., 2010). YAP/TAZ can also interact with TGF-β regulated Smads, interfering with hippo activity of the cell results in concomitant nuclear accumulation of YAP/TAZ and Smads (Varelas et al., 2008). Subsequently, TAZ/YAP cooperates with Smads to promote the activation of specific target genes that control the maintenance of stem cell pluripotency or the induction of differentiation (Varelas et al., 2008). In addition, YAP/TAZ-TEAD and SMAD may act synergistically to promote follicle growth by disrupting the Hippo pathway in vitro (Grosbois and Demeestere, 2018). GDF9 and BMP15, the members of the TGFβ superfamily, which are expressed in oocytes during most of folliculogenesis. They are participated in specific functions of GCs and cumulus cells (McGrath et al., 1995; Laitinen et al., 1998; Gilchrist et al., 2008). During follicular growth, they activate the canonical TGF-β signaling cascade in the GCs of growing follicles where CCN2 mRNA is abundantly expressed (Harlow and Hillier, 2002). Alarcon et al. (2009) found that in embryonic stem cells of mouse, phosphorylated SMAD1-SMAD4 complexes recruit YAP1 to the enhancer regions of BMP-responsive genes, leading to BMP-induced gene expression and further induction of cell differentiation. In conclusion, the reduction of cytosolic YAP/TAZ after Hippo pathway disrupted could activate WNT and TGFβ signaling pathways to jointly promote granulosa cell proliferation and follicular development.
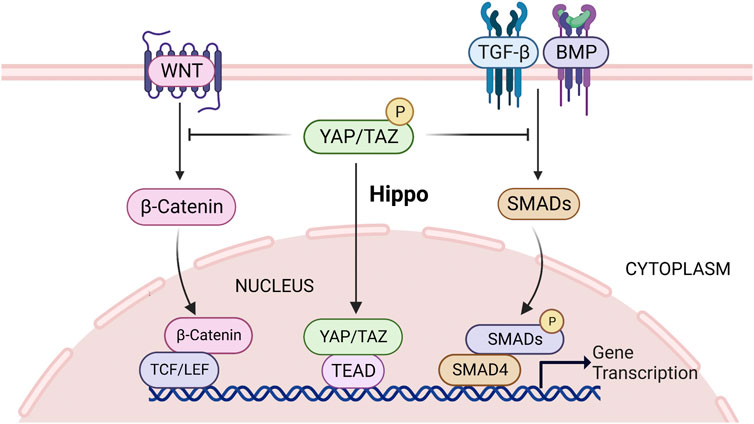
FIGURE 5. Hippo signaling can interect with Wnt/β-catenin and TGF-β signaling, and its regulators YAP/TAZ locate at the crosstalk between Wnt/β-catenin and TGF-β/Smads. When the Hippo pathway is activated, YAP/TAZ is sequestered in the cytoplasm, thereby inhibiting Wnt/β-catenin and TGF-β activity and subsequent gene transcription. See text for details. TGF-β, transforming growth factor β; YAP, yes-associated protein; TAZ, transcriptional co-activator with PDZ-binding motif.
There are few studies investigating the mechanism of Hippo and Hh interaction in the human ovary, and there is evidence for an association between the Hippo and Hh pathways in medulloblastoma (Hong et al., 2016). Activation of Sonic hedgehog of cerebellar granule neuron precursors upregulates YAP1 expresssion, and immediate binding between YAP1 and insulin receptor substrate 1 (IRS1), leads to the nuclear accumulation of YAP1 (Fernandez et al., 2009). Hh signaling in Drosophila melanogaster ovarian follicle stem cells (FSCs) increases the activity of Yki. Both Hh signaling and Yki positively regulate the rate of FSC proliferation (Huang and Kalderon, 2014). Li et al. (2015b) further demonstrated in Drosophila ovaries that Cubitus interruptus (Hh signaling effector) inhibits Hippo activity, which impairs the formation of the Hpo-Wts signaling complex, thus increasing the expression of Yki in nucleus.
Regarding the association of Hippo and Notch pathway, related studies have found that in the intestinal canal, activated YAP1 transcription upregulates Notch receptor expression, leading to an increase of Notch signaling activity (Hong et al., 2016). Several members of the Notch pathway, such as Notch1 and Notch2, were upregulated in hepatocytes with Yap1 overexpression, and Notch2, identified as the targeted gene of YAP1-TEAD complex, was expressed at high levels in the cell membrane and GCs of ovarian follicles (Yimlamai et al., 2014; Jing et al., 2017). Meignin et al. (2007) showed that the Salvador-Warts-Hippo (SWH) Pathway is required for Notch signaling in Drosophila ovaries and that the SWH signal is involved in Notch-dependent maturation of the PFC.
The mechanism of Hippo pathway and the crosstalk of several pathways to regulate ovarian development is complex and diverse, so a large number of experimental studies are still needed to confirm.
5 Conclusion
Hippo pathway and its downstream transcription effector YAP/TAZ have emerged as key regulatory players in ovarian development and function, regulating the proliferation and migration of ovarian germ cells and somatic cells, follicular activation and development, and cell apoptosis. This article elucidates the process of ovarian development and the function and molecular mechanism of Hippo pathway in it. However, ovarian development and regulatory mechanisms are complex, and Hippo pathway intersects with other pathways that regulate follicular development, and is affected by ovarian endocrine and metabolic regulation. Therefore, further experimental research is still needed to clarify the localization and regulatory mechanism of Hippo pathway molecules at various stages of follicular development, which is of great significance for clinical treatment of various ovarian diseases.
Author contributions
MZ performed literature review and the writing of the manuscript. MX, JZ and CZ contributed to draft modification. All authors contributed to manuscript and approved the submitted version.
Funding
This study was supported by grants from National Natural Science Foundation of China (No. 81202757, No. 81874388).
Conflict of interest
The authors declare that the research was conducted in the absence of any commercial or financial relationships that could be construed as a potential conflict of interest.
Publisher’s note
All claims expressed in this article are solely those of the authors and do not necessarily represent those of their affiliated organizations, or those of the publisher, the editors and the reviewers. Any product that may be evaluated in this article, or claim that may be made by its manufacturer, is not guaranteed or endorsed by the publisher.
References
Abbassi, L., Malki, S., Cockburn, K., Macaulay, A., Robert, C., Rossant, J., et al. (2016). Multiple mechanisms cooperate to constitutively exclude the transcriptional Co-activator YAP from the nucleus during murine oogenesis. Biol. Reprod. 94 (5), 102. doi:10.1095/biolreprod.115.137968
Abedelahi, A., Salehnia, M., and Allameh, A. A. (2008). The effects of different concentrations of sodium selenite on the in vitro maturation of preantral follicles in serum-free and serum supplemented media. J. Assist. Reprod. Genet. 25 (9-10), 483–488. doi:10.1007/s10815-008-9252-z
Adhikari, D., Gorre, N., Risal, S., Zhao, Z., Zhang, H., Shen, Y., et al. (2012). The safe use of a PTEN inhibitor for the activation of dormant mouse primordial follicles and generation of fertilizable eggs. PLoS One 7 (6), e39034. doi:10.1371/journal.pone.0039034
Alarcon, C., Zaromytidou, A. I., Xi, Q., Gao, S., Yu, J., Fujisawa, S., et al. (2009). Nuclear CDKs drive Smad transcriptional activation and turnover in BMP and TGF-beta pathways. Cell 139 (4), 757–769. doi:10.1016/j.cell.2009.09.035
Aragona, M., Panciera, T., Manfrin, A., Giulitti, S., Michielin, F., Elvassore, N., et al. (2013). A mechanical checkpoint controls multicellular growth through YAP/TAZ regulation by actin-processing factors. Cell 154 (5), 1047–1059. doi:10.1016/j.cell.2013.07.042
Arav, A. (2014). Cryopreservation of oocytes and embryos. Theriogenology 81 (1), 96–102. doi:10.1016/j.theriogenology.2013.09.011
Arnanz, A., De Munck, N., Bayram, A., El-Damen, A., Abdalla, A., ElKhatib, I., et al. (2020). Blastocyst mitochondrial DNA (mtDNA) is not affected by oocyte vitrification: A sibling oocyte study. J. Assist. Reprod. Genet. 37 (6), 1387–1397. doi:10.1007/s10815-020-01795-6
Attisano, L., and Wrana, J. L. (2013). Signal integration in TGF-beta, WNT, and Hippo pathways. F1000Prime Rep. 5, 17. doi:10.12703/P5-17
Avruch, J., Zhou, D., Fitamant, J., Bardeesy, N., Mou, F., and Barrufet, L. R. (2012). Protein kinases of the hippo pathway: Regulation and substrates. Semin. Cell Dev. Biol. 23 (7), 770–784. doi:10.1016/j.semcdb.2012.07.002
Practice Committee of the American Society for Reproductive Medicine Penzias, A., Azziz, R., Bendikson, K., Falcone, T., Hansen, K., Hill, M., et al. (2020). Testing and interpreting measures of ovarian reserve: A committee opinion. Fertil. Steril. 114 (6), 1151–1157. doi:10.1016/j.fertnstert.2020.09.134
Barberet, J., Ducreux, B., Bruno, C., Guilleman, M., Simonot, R., Lieury, N., et al. (2022). Comparison of oocyte vitrification using a semi-automated or a manual closed system in human siblings: Survival and transcriptomic analyses. J. Ovarian Res. 15 (1), 128. doi:10.1186/s13048-022-01064-3
Basu, S., Totty, N. F., Irwin, M. S., Sudol, M., and Downward, J. (2003). Akt phosphorylates the Yes-associated protein, YAP, to induce interaction with 14-3-3 and attenuation of p73-mediated apoptosis. Mol. Cell 11 (1), 11–23. doi:10.1016/s1097-2765(02)00776-1
Best, B. P. (2015). Cryoprotectant toxicity: Facts, issues, and questions. Rejuvenation Res. 18 (5), 422–436. doi:10.1089/rej.2014.1656
Borreguero-Munoz, N., Fletcher, G. C., Aguilar-Aragon, M., Elbediwy, A., Vincent-Mistiaen, Z. I., and Thompson, B. J. (2019). The Hippo pathway integrates PI3K-Akt signals with mechanical and polarity cues to control tissue growth. PLoS Biol. 17 (10), e3000509. doi:10.1371/journal.pbio.3000509
Brown, H. M., and Russell, D. L. (2014). Blood and lymphatic vasculature in the ovary: Development, function and disease. Hum. Reprod. Update 20 (1), 29–39. doi:10.1093/humupd/dmt049
Camargo, F. D., Gokhale, S., Johnnidis, J. B., Fu, D., Bell, G. W., Jaenisch, R., et al. (2007). YAP1 increases organ size and expands undifferentiated progenitor cells. Curr. Biol. 17 (23), 2054–2060. doi:10.1016/j.cub.2007.10.039
Castanon, B. I., Stapp, A. D., Gifford, C. A., Spicer, L. J., Hallford, D. M., and Hernandez Gifford, J. A. (2012). Follicle-stimulating hormone regulation of estradiol production: Possible involvement of WNT2 and beta-catenin in bovine granulosa cells. J. Anim. Sci. 90 (11), 3789–3797. doi:10.2527/jas.2011-4696
Castrillon, D. H., Miao, L., Kollipara, R., Horner, J. W., and DePinho, R. A. (2003). Suppression of ovarian follicle activation in mice by the transcription factor Foxo3a. Science 301 (5630), 215–218. doi:10.1126/science.1086336
Chang, L., Azzolin, L., Di Biagio, D., Zanconato, F., Battilana, G., Lucon Xiccato, R., et al. (2018). The SWI/SNF complex is a mechanoregulated inhibitor of YAP and TAZ. Nature 563 (7730), 265–269. doi:10.1038/s41586-018-0658-1
Chang, S., and Dunaif, A. (2021). Diagnosis of polycystic ovary syndrome: Which criteria to use and when? Endocrinol. Metab. Clin. North Am. 50 (1), 11–23. doi:10.1016/j.ecl.2020.10.002
Chauvin, S., Cohen-Tannoudji, J., and Guigon, C. J. (2022). Estradiol signaling at the heart of folliculogenesis: Its potential deregulation in human ovarian pathologies. Int. J. Mol. Sci. 23 (1), 512. doi:10.3390/ijms23010512
Chaves, R. N., Lima-Verde, I. B., Celestino, J. J., Duarte, A. B., Alves, A. M., Matos, M. H., et al. (2010). Fibroblast growth factor-10 maintains the survival and promotes the growth of cultured goat preantral follicles. Domest. Anim. Endocrinol. 39 (4), 249–258. doi:10.1016/j.domaniend.2010.06.006
Chen, R., Xie, R., Meng, Z., Ma, S., and Guan, K. L. (2019). STRIPAK integrates upstream signals to initiate the Hippo kinase cascade. Nat. Cell Biol. 21 (12), 1565–1577. doi:10.1038/s41556-019-0426-y
Cheng, Y., Feng, Y., Jansson, L., Sato, Y., Deguchi, M., Kawamura, K., et al. (2015). Actin polymerization-enhancing drugs promote ovarian follicle growth mediated by the Hippo signaling effector YAP. FASEB J. 29 (6), 2423–2430. doi:10.1096/fj.14-267856
Clark, K. L., George, J. W., Przygrodzka, E., Plewes, M. R., Hua, G., Wang, C., et al. (2022). Hippo signaling in the ovary: Emerging roles in development, fertility, and disease. Endocr. Rev. 43 (6), 1074–1096. doi:10.1210/endrev/bnac013
Cobo, A., Kuwayama, M., Perez, S., Ruiz, A., Pellicer, A., and Remohi, J. (2019). Reprint of: Comparison of concomitant outcome achieved with fresh and cryopreserved donor oocytes vitrified by the Cryotop method. Fertil. Steril. 112, e85–e92. doi:10.1016/j.fertnstert.2019.08.078
Cunningham, M. A., Zhu, Q., and Hammond, J. M. (2004). FoxO1a can alter cell cycle progression by regulating the nuclear localization of p27kip in granulosa cells. Mol. Endocrinol. 18 (7), 1756–1767. doi:10.1210/me.2004-0071
Dalton, C. M., and Carroll, J. (2013). Biased inheritance of mitochondria during asymmetric cell division in the mouse oocyte. J. Cell Sci. 126, 2955–2964. doi:10.1242/jcs.128744
Dasgupta, I., and McCollum, D. (2019). Control of cellular responses to mechanical cues through YAP/TAZ regulation. J. Biol. Chem. 294 (46), 17693–17706. doi:10.1074/jbc.REV119.007963
De Roo, C., Lierman, S., Tilleman, K., and De Sutter, P. (2020). In-vitro fragmentation of ovarian tissue activates primordial follicles through the Hippo pathway. Hum. Reprod. Open 2020 (4), hoaa048. doi:10.1093/hropen/hoaa048
De Roo, C., Lierman, S., Tilleman, K., Peynshaert, K., Braeckmans, K., Caanen, M., et al. (2017). Ovarian tissue cryopreservation in female-to-male transgender people: Insights into ovarian histology and physiology after prolonged androgen treatment. Reprod. Biomed. Online 34 (6), 557–566. doi:10.1016/j.rbmo.2017.03.008
de Ziegler, D., Fraisse, T., de Candolle, G., Vulliemoz, N., Bellavia, M., and Colamaria, S. (2007). Outlook: Roles of FSH and LH during the follicular phase: Insight into natural cycle IVF. Reprod. Biomed. Online 15 (5), 507–513. doi:10.1016/s1472-6483(10)60381-1
Devos, M., Grosbois, J., and Demeestere, I. (2020). Interaction between PI3K/AKT and Hippo pathways during in vitro follicular activation and response to fragmentation and chemotherapy exposure using a mouse immature ovary model. Biol. Reprod. 102 (3), 717–729. doi:10.1093/biolre/ioz215
Dey, A., Varelas, X., and Guan, K. L. (2020). Targeting the Hippo pathway in cancer, fibrosis, wound healing and regenerative medicine. Nat. Rev. Drug Discov. 19 (7), 480–494. doi:10.1038/s41573-020-0070-z
Dos Santos, E. C., Lalonde-Larue, A., Antoniazzi, A. Q., Barreta, M. H., Price, C. A., Dias Goncalves, P. B., et al. (2022). YAP signaling in preovulatory granulosa cells is critical for the functioning of the EGF network during ovulation. Mol. Cell Endocrinol. 541, 111524. doi:10.1016/j.mce.2021.111524
Ebert, K. M., Liem, H., and Hecht, N. B. (1988). Mitochondrial DNA in the mouse preimplantation embryo. J. Reprod. Fertil. 82 (1), 145–149. doi:10.1530/jrf.0.0820145
Edson, M. A., Nagaraja, A. K., and Matzuk, M. M. (2009). The mammalian ovary from Genesis to revelation. Endocr. Rev. 30 (6), 624–712. doi:10.1210/er.2009-0012
Eichenlaub-Ritter, U., Wieczorek, M., Luke, S., and Seidel, T. (2011). Age related changes in mitochondrial function and new approaches to study redox regulation in mammalian oocytes in response to age or maturation conditions. Mitochondrion 11 (5), 783–796. doi:10.1016/j.mito.2010.08.011
Eppig, J. J. (1996). Coordination of nuclear and cytoplasmic oocyte maturation in eutherian mammals. Reprod. Fertil. Dev. 8 (4), 485–489. doi:10.1071/rd9960485
Fabregues, F., Ferreri, J., Calafell, J. M., Moreno, V., Borras, A., Manau, D., et al. (2018). Pregnancy after drug-free in vitro activation of follicles and fresh tissue autotransplantation in primary ovarian insufficiency patient: A case report and literature review. J. Ovarian Res. 11 (1), 76. doi:10.1186/s13048-018-0447-3
Fan, H. Y., Liu, Z., Shimada, M., Sterneck, E., Johnson, P. F., Hedrick, S. M., et al. (2009). MAPK3/1 (ERK1/2) in ovarian granulosa cells are essential for female fertility. Science 324 (5929), 938–941. doi:10.1126/science.1171396
Farzollahi, M., Tayefi-Nasrabadi, H., Mohammadnejad, D., and Abedelahi, A. (2016). Supplementation of culture media with vitamin E improves mouse antral follicle maturation and embryo development from vitrified ovarian tissue. J. Obstet. Gynaecol. Res. 42 (5), 526–535. doi:10.1111/jog.12933
Fernandez, L. A., Northcott, P. A., Dalton, J., Fraga, C., Ellison, D., Angers, S., et al. (2009). YAP1 is amplified and up-regulated in hedgehog-associated medulloblastomas and mediates Sonic hedgehog-driven neural precursor proliferation. Genes Dev. 23 (23), 2729–2741. doi:10.1101/gad.1824509
Findlay, J. K., Hutt, K. J., Hickey, M., and Anderson, R. A. (2015). How is the number of primordial follicles in the ovarian reserve established? Biol. Reprod. 93 (5), 111. doi:10.1095/biolreprod.115.133652
Gardner, D. K., Sheehan, C. B., Rienzi, L., Katz-Jaffe, M., and Larman, M. G. (2007). Analysis of oocyte physiology to improve cryopreservation procedures. Theriogenology 67 (1), 64–72. doi:10.1016/j.theriogenology.2006.09.012
Ge, H., Tollner, T. L., Hu, Z., Dai, M., Li, X., Guan, H., et al. (2012). The importance of mitochondrial metabolic activity and mitochondrial DNA replication during oocyte maturation in vitro on oocyte quality and subsequent embryo developmental competence. Mol. Reprod. Dev. 79 (6), 392–401. doi:10.1002/mrd.22042
Gershon, E., and Dekel, N. (2020). Newly identified regulators of ovarian folliculogenesis and ovulation. Int. J. Mol. Sci. 21 (12), 4565. doi:10.3390/ijms21124565
Gilchrist, R. B., Lane, M., and Thompson, J. G. (2008). Oocyte-secreted factors: Regulators of cumulus cell function and oocyte quality. Hum. Reprod. Update 14 (2), 159–177. doi:10.1093/humupd/dmm040
Gougeon, A. (1996). Regulation of ovarian follicular development in primates: Facts and hypotheses. Endocr. Rev. 17 (2), 121–155. doi:10.1210/edrv-17-2-121
Grannas, K., Arngarden, L., Lonn, P., Mazurkiewicz, M., Blokzijl, A., Zieba, A., et al. (2015). Crosstalk between hippo and TGFβ: Subcellular localization of YAP/TAZ/smad complexes. J. Mol. Biol. 427 (21), 3407–3415. doi:10.1016/j.jmb.2015.04.015
Grosbois, J., and Demeestere, I. (2018). Dynamics of PI3K and Hippo signaling pathways during in vitro human follicle activation. Hum. Reprod. 33 (9), 1705–1714. doi:10.1093/humrep/dey250
Hansen, K. R., Knowlton, N. S., Thyer, A. C., Charleston, J. S., Soules, M. R., and Klein, N. A. (2008). A new model of reproductive aging: The decline in ovarian non-growing follicle number from birth to menopause. Hum. Reprod. 23 (3), 699–708. doi:10.1093/humrep/dem408
Harlow, C. R., and Hillier, S. G. (2002). Connective tissue growth factor in the ovarian paracrine system. Mol. Cell Endocrinol. 187 (1-2), 23–27. doi:10.1016/s0303-7207(01)00702-x
Hernandez Gifford, J. A. (2015). The role of WNT signaling in adult ovarian folliculogenesis. Reproduction 150 (4), R137–R148. doi:10.1530/REP-14-0685
Hirate, Y., and Sasaki, H. (2014). The role of angiomotin phosphorylation in the Hippo pathway during preimplantation mouse development. Tissue Barriers 2 (1), e28127. doi:10.4161/tisb.28127
Hirshfield, A. N. (1991). Development of follicles in the mammalian ovary. Int. Rev. Cytol. 124, 43–101. doi:10.1016/s0074-7696(08)61524-7
Hong, A. W., Meng, Z., and Guan, K. L. (2016). The Hippo pathway in intestinal regeneration and disease. Nat. Rev. Gastroenterol. Hepatol. 13 (6), 324–337. doi:10.1038/nrgastro.2016.59
Hong, L., Li, X., Zhou, D., Geng, J., and Chen, L. (2018). Role of Hippo signaling in regulating immunity. Cell Mol. Immunol. 15 (12), 1003–1009. doi:10.1038/s41423-018-0007-1
Hsu, S. Y., Lai, R. J., Finegold, M., and Hsueh, A. J. (1996). Targeted overexpression of Bcl-2 in ovaries of transgenic mice leads to decreased follicle apoptosis, enhanced folliculogenesis, and increased germ cell tumorigenesis. Endocrinology 137 (11), 4837–4843. doi:10.1210/endo.137.11.8895354
Hsueh, A. J., Kawamura, K., Cheng, Y., and Fauser, B. C. (2015). Intraovarian control of early folliculogenesis. Endocr. Rev. 36 (1), 1–24. doi:10.1210/er.2014-1020
Hsueh, A. J. W., and Kawamura, K. (2020). Hippo signaling disruption and ovarian follicle activation in infertile patients. Fertil. Steril. 114 (3), 458–464. doi:10.1016/j.fertnstert.2020.07.031
Hu, L. L., Chang, H. M., Yi, Y., Liu, Y., Taylor, E. L., Zheng, L. P., et al. (2019a). CCN2 mediates S1P-induced upregulation of COX2 expression in human granulosa-lutein cells. Cells 8 (11), 1445. doi:10.3390/cells8111445
Hu, L. L., Su, T., Luo, R. C., Zheng, Y. H., Huang, J., Zhong, Z. S., et al. (2019b). Hippo pathway functions as a downstream effector of AKT signaling to regulate the activation of primordial follicles in mice. J. Cell Physiol. 234 (2), 1578–1587. doi:10.1002/jcp.27024
Huang, J., and Kalderon, D. (2014). Coupling of Hedgehog and Hippo pathways promotes stem cell maintenance by stimulating proliferation. J. Cell Biol. 205 (3), 325–338. doi:10.1083/jcb.201309141
Hughes, C. H. K., and Murphy, B. D. (2021). Nuclear receptors: Key regulators of somatic cell functions in the ovulatory process. Mol. Asp. Med. 78, 100937. doi:10.1016/j.mam.2020.100937
Ito, M., Yoshino, O., Ono, Y., Yamaki-Ushijima, A., Tanaka, T., Shima, T., et al. (2021). Bone morphogenetic protein-2 enhances gonadotropin-independent follicular development via sphingosine kinase 1. Am. J. Reprod. Immunol. 85 (5), e13374. doi:10.1111/aji.13374
Jaaskelainen, M., Kyronlahti, A., Anttonen, M., Nishi, Y., Yanase, T., Secchiero, P., et al. (2009). TRAIL pathway components and their putative role in granulosa cell apoptosis in the human ovary. Differentiation 77 (4), 369–376. doi:10.1016/j.diff.2008.12.001
Ji, S. Y., Liu, X. M., Li, B. T., Zhang, Y. L., Liu, H. B., Zhang, Y. C., et al. (2017). The polycystic ovary syndrome-associated gene Yap1 is regulated by gonadotropins and sex steroid hormones in hyperandrogenism-induced oligo-ovulation in mouse. Mol. Hum. Reprod. 23 (10), 698–707. doi:10.1093/molehr/gax046
Jing, J., Jiang, X., Chen, J., Yao, X., Zhao, M., Li, P., et al. (2017). Notch signaling pathway promotes the development of ovine ovarian follicular granulosa cells. Anim. Reprod. Sci. 181, 69–78. doi:10.1016/j.anireprosci.2017.03.017
John, G. B., Gallardo, T. D., Shirley, L. J., and Castrillon, D. H. (2008). Foxo3 is a PI3K-dependent molecular switch controlling the initiation of oocyte growth. Dev. Biol. 321 (1), 197–204. doi:10.1016/j.ydbio.2008.06.017
Jones, A. S. K., and Shikanov, A. (2019). Follicle development as an orchestrated signaling network in a 3D organoid. J. Biol. Eng. 13, 2. doi:10.1186/s13036-018-0134-3
Kagawa, N., Silber, S., and Kuwayama, M. (2009). Successful vitrification of bovine and human ovarian tissue. Reprod. Biomed. Online 18 (4), 568–577. doi:10.1016/s1472-6483(10)60136-8
Kaipia, A., and Hsueh, A. J. (1997). Regulation of ovarian follicle atresia. Annu. Rev. Physiol. 59, 349–363. doi:10.1146/annurev.physiol.59.1.349
Karchugina, S., Benton, D., and Chernoff, J. (2021). Regulation of MST complexes and activity via SARAH domain modifications. Biochem. Soc. Trans. 49 (2), 675–683. doi:10.1042/BST20200559
Kawamura, K., Cheng, Y., Suzuki, N., Deguchi, M., Sato, Y., Takae, S., et al. (2013). Hippo signaling disruption and Akt stimulation of ovarian follicles for infertility treatment. Proc. Natl. Acad. Sci. U. S. A. 110 (43), 17474–17479. doi:10.1073/pnas.1312830110
Keefe, D., Kumar, M., and Kalmbach, K. (2015). Oocyte competency is the key to embryo potential. Fertil. Steril. 103 (2), 317–322. doi:10.1016/j.fertnstert.2014.12.115
Kirillova, A., Smitz, J. E. J., Sukhikh, G. T., and Mazunin, I. (2021). The role of mitochondria in oocyte maturation. Cells 10 (9), 2484. doi:10.3390/cells10092484
Klionsky, D. J. (2008). Autophagy revisited: A conversation with christian de Duve. Autophagy 4 (6), 740–743. doi:10.4161/auto.6398
Kopeika, J., Thornhill, A., and Khalaf, Y. (2015). The effect of cryopreservation on the genome of gametes and embryos: Principles of cryobiology and critical appraisal of the evidence. Hum. Reprod. Update 21 (2), 209–227. doi:10.1093/humupd/dmu063
Kukat, C., Wurm, C. A., Spahr, H., Falkenberg, M., Larsson, N. G., and Jakobs, S. (2011). Super-resolution microscopy reveals that mammalian mitochondrial nucleoids have a uniform size and frequently contain a single copy of mtDNA. Proc. Natl. Acad. Sci. U. S. A. 108 (33), 13534–13539. doi:10.1073/pnas.1109263108
Kumar, T. R. (2018). Fshb knockout mouse model, two decades later and into the future. Endocrinology 159 (5), 1941–1949. doi:10.1210/en.2018-00072
Laitinen, M., Vuojolainen, K., Jaatinen, R., Ketola, I., Aaltonen, J., Lehtonen, E., et al. (1998). A novel growth differentiation factor-9 (GDF-9) related factor is co-expressed with GDF-9 in mouse oocytes during folliculogenesis. Mech. Dev. 78 (1-2), 135–140. doi:10.1016/s0925-4773(98)00161-0
Lalonde-Larue, A., Boyer, A., Dos Santos, E. C., Boerboom, D., Bernard, D. J., and Zamberlam, G. (2022). The hippo pathway effectors YAP and TAZ regulate LH release by pituitary gonadotrope cells in mice. Endocrinology 163 (1), bqab238. doi:10.1210/endocr/bqab238
Li, C., Kan, L., Chen, Y., Zheng, X., Li, W., Zhang, W., et al. (2015). Ci antagonizes Hippo signaling in the somatic cells of the ovary to drive germline stem cell differentiation. Cell Res. 25 (10), 1152–1170. doi:10.1038/cr.2015.114
Li, L., Shi, X., Shi, Y., and Wang, Z. (2021). The signaling pathways involved in ovarian follicle development. Front. Physiol. 12, 730196. doi:10.3389/fphys.2021.730196
Li, Q., Wang, M., Hu, Y., Zhao, E., Li, J., Ren, L., et al. (2021). MYBL2 disrupts the Hippo-YAP pathway and confers castration resistance and metastatic potential in prostate cancer. Theranostics 11 (12), 5794–5812. doi:10.7150/thno.56604
Li, Y., Schang, G., Boehm, U., Deng, C. X., Graff, J., and Bernard, D. J. (2017). SMAD3 regulates follicle-stimulating hormone synthesis by pituitary gonadotrope cells in vivo. J. Biol. Chem. 292 (6), 2301–2314. doi:10.1074/jbc.M116.759167
Li, Y., Zhou, H., Li, F., Chan, S. W., Lin, Z., Wei, Z., et al. (2015). Angiomotin binding-induced activation of Merlin/NF2 in the Hippo pathway. Cell Res. 25 (7), 801–817. doi:10.1038/cr.2015.69
Liu, C. Y., Zha, Z. Y., Zhou, X., Zhang, H., Huang, W., Zhao, D., et al. (2010). The hippo tumor pathway promotes TAZ degradation by phosphorylating a phosphodegron and recruiting the SCF{beta}-TrCP E3 ligase. J. Biol. Chem. 285 (48), 37159–37169. doi:10.1074/jbc.M110.152942
Liu, K. C., and Ge, W. (2013). Evidence for gating roles of protein kinase A and protein kinase C in estradiol-induced luteinizing hormone receptor (lhcgr) expression in zebrafish ovarian follicle cells. PLoS One 8 (5), e62524. doi:10.1371/journal.pone.0062524
Lonergan, P., and Fair, T. (2016). Maturation of oocytes in vitro. Annu. Rev. Anim. Biosci. 4, 255–268. doi:10.1146/annurev-animal-022114-110822
Lunding, S. A., Andersen, A. N., Hardardottir, L., Olesen, H. O., Kristensen, S. G., Andersen, C. Y., et al. (2020). Hippo signaling, actin polymerization, and follicle activation in fragmented human ovarian cortex. Mol. Reprod. Dev. 87 (6), 711–719. doi:10.1002/mrd.23353
Lunding, S. A., Pors, S. E., Kristensen, S. G., Landersoe, S. K., Jeppesen, J. V., Flachs, E. M., et al. (2019). Biopsying, fragmentation and autotransplantation of fresh ovarian cortical tissue in infertile women with diminished ovarian reserve. Hum. Reprod. 34 (10), 1924–1936. doi:10.1093/humrep/dez152
Luo, J., and Yu, F. X. (2019). GPCR-hippo signaling in cancer. Cells 8 (5), 426. doi:10.3390/cells8050426
Luo, K. (2017). Signaling cross talk between TGF-β/smad and other signaling pathways. Cold Spring Harb. Perspect. Biol. 9 (1), a022137. doi:10.1101/cshperspect.a022137
Lv, X., He, C., Huang, C., Hua, G., Chen, X., Timm, B. K., et al. (2020). Reprogramming of ovarian granulosa cells by YAP1 leads to development of high-grade cancer with mesenchymal lineage and serous features. Sci. Bull. (Beijing) 65 (15), 1281–1296. doi:10.1016/j.scib.2020.03.040
Lv, X., He, C., Huang, C., Wang, H., Hua, G., Wang, Z., et al. (2019). Timely expression and activation of YAP1 in granulosa cells is essential for ovarian follicle development. FASEB J. 33 (9), 10049–10064. doi:10.1096/fj.201900179RR
Lyu, Z., Qin, N., Tyasi, T. L., Zhu, H., Liu, D., Yuan, S., et al. (2016). The hippo/MST pathway member SAV1 plays a suppressive role in development of the prehierarchical follicles in hen ovary. PLoS One 11 (8), e0160896. doi:10.1371/journal.pone.0160896
Ma, S., Meng, Z., Chen, R., and Guan, K. L. (2019). The hippo pathway: Biology and pathophysiology. Annu. Rev. Biochem. 88, 577–604. doi:10.1146/annurev-biochem-013118-111829
Magoffin, D. A. (2005). Ovarian theca cell. Int. J. Biochem. Cell Biol. 37 (7), 1344–1349. doi:10.1016/j.biocel.2005.01.016
Makker, A., Goel, M. M., and Mahdi, A. A. (2014). PI3K/PTEN/Akt and TSC/mTOR signaling pathways, ovarian dysfunction, and infertility: An update. J. Mol. Endocrinol. 53 (3), R103–R118. doi:10.1530/JME-14-0220
Manabe, N., Matsuda-Minehata, F., Goto, Y., Maeda, A., Cheng, Y., Nakagawa, S., et al. (2008). Role of cell death ligand and receptor system on regulation of follicular atresia in pig ovaries. Reprod. Domest. Anim. 43, 268–272. doi:10.1111/j.1439-0531.2008.01172.x
Markstrom, E., Svensson, E., Shao, R., Svanberg, B., and Billig, H. (2002). Survival factors regulating ovarian apoptosis - dependence on follicle differentiation. Reproduction 123 (1), 23–30. doi:10.1530/rep.0.1230023
Masciangelo, R., Hossay, C., Chiti, M. C., Manavella, D. D., Amorim, C. A., Donnez, J., et al. (2020). Role of the PI3K and Hippo pathways in follicle activation after grafting of human ovarian tissue. J. Assist. Reprod. Genet. 37 (1), 101–108. doi:10.1007/s10815-019-01628-1
Matsuda, F., Inoue, N., Manabe, N., and Ohkura, S. (2012). Follicular growth and atresia in mammalian ovaries: Regulation by survival and death of granulosa cells. J. Reprod. Dev. 58 (1), 44–50. doi:10.1262/jrd.2011-012
Matsuda-Minehata, F., Inoue, N., Goto, Y., and Manabe, N. (2006). The regulation of ovarian granulosa cell death by pro- and anti-apoptotic molecules. J. Reprod. Dev. 52 (6), 695–705. doi:10.1262/jrd.18069
May-Panloup, P., Boucret, L., Chao de la Barca, J. M., Desquiret-Dumas, V., Ferre-L'Hotellier, V., Moriniere, C., et al. (2016). Ovarian ageing: The role of mitochondria in oocytes and follicles. Hum. Reprod. Update 22 (6), 725–743. doi:10.1093/humupd/dmw028
McCarthy, M. J., Baumber, J., Kass, P. H., and Meyers, S. A. (2010). Osmotic stress induces oxidative cell damage to rhesus macaque spermatozoa. Biol. Reprod. 82 (3), 644–651. doi:10.1095/biolreprod.109.080507
McGrath, S. A., Esquela, A. F., and Lee, S. J. (1995). Oocyte-specific expression of growth/differentiation factor-9. Mol. Endocrinol. 9 (1), 131–136. doi:10.1210/mend.9.1.7760846
McLaughlin, E. A., and McIver, S. C. (2009). Awakening the oocyte: Controlling primordial follicle development. Reproduction 137 (1), 1–11. doi:10.1530/REP-08-0118
McLaughlin, M., Kinnell, H. L., Anderson, R. A., and Telfer, E. E. (2014). Inhibition of phosphatase and tensin homologue (PTEN) in human ovary in vitro results in increased activation of primordial follicles but compromises development of growing follicles. Mol. Hum. Reprod. 20 (8), 736–744. doi:10.1093/molehr/gau037
Meignin, C., Alvarez-Garcia, I., Davis, I., and Palacios, I. M. (2007). The salvador-warts-hippo pathway is required for epithelial proliferation and axis specification in Drosophila. Curr. Biol. 17 (21), 1871–1878. doi:10.1016/j.cub.2007.09.062
Menard, A., Abou Nader, N., Levasseur, A., St-Jean, G., Le Roy, M. L. G., Boerboom, D., et al. (2020). Targeted disruption of Lats1 and Lats2 in mice impairs adrenal cortex development and alters adrenocortical cell fate. Endocrinology 161 (5), bqaa052. doi:10.1210/endocr/bqaa052
Meng, Z., Moroishi, T., Mottier-Pavie, V., Plouffe, S. W., Hansen, C. G., Hong, A. W., et al. (2015). MAP4K family kinases act in parallel to MST1/2 to activate LATS1/2 in the Hippo pathway. Nat. Commun. 6, 8357. doi:10.1038/ncomms9357
Misra, J. R., and Irvine, K. D. (2018). The hippo signaling network and its biological functions. Annu. Rev. Genet. 52, 65–87. doi:10.1146/annurev-genet-120417-031621
Mohri, Z., Del Rio Hernandez, A., and Krams, R. (2017). The emerging role of YAP/TAZ in mechanotransduction. J. Thorac. Dis. 9 (5), E507–E509. doi:10.21037/jtd.2017.03.179
Monsivais, D., Matzuk, M. M., and Pangas, S. A. (2017). The TGF-beta family in the reproductive tract. Cold Spring Harb. Perspect. Biol. 9 (10), a022251. doi:10.1101/cshperspect.a022251
Monzo, C., Haouzi, D., Roman, K., Assou, S., Dechaud, H., and Hamamah, S. (2012). Slow freezing and vitrification differentially modify the gene expression profile of human metaphase II oocytes. Hum. Reprod. 27 (7), 2160–2168. doi:10.1093/humrep/des153
Nottola, S. A., Coticchio, G., Sciajno, R., Gambardella, A., Maione, M., Scaravelli, G., et al. (2009). Ultrastructural markers of quality in human mature oocytes vitrified using cryoleaf and cryoloop. Reprod. Biomed. Online 19, 17–27. doi:10.1016/s1472-6483(10)60280-5
Novella-Maestre, E., Herraiz, S., Rodriguez-Iglesias, B., Diaz-Garcia, C., and Pellicer, A. (2015). Short-term PTEN inhibition improves in vitro activation of primordial follicles, preserves follicular viability, and restores AMH levels in cryopreserved ovarian tissue from cancer patients. PLoS One 10 (5), e0127786. doi:10.1371/journal.pone.0127786
Pennarossa, G., Gandolfi, F., and Brevini, T. A. L. (2021). Biomechanical signaling in oocytes and parthenogenetic cells. Front. Cell Dev. Biol. 9, 646945. doi:10.3389/fcell.2021.646945
Picton, H., Briggs, D., and Gosden, R. (1998). The molecular basis of oocyte growth and development. Mol. Cell Endocrinol. 145 (1-2), 27–37. doi:10.1016/s0303-7207(98)00166-x
Plewes, M. R., Hou, X., Zhang, P., Liang, A., Hua, G., Wood, J. R., et al. (2019). Yes-associated protein 1 is required for proliferation and function of bovine granulosa cells in vitro†. Biol. Reprod. 101 (5), 1001–1017. doi:10.1093/biolre/ioz139
Plouffe, S. W., Meng, Z., Lin, K. C., Lin, B., Hong, A. W., Chun, J. V., et al. (2016). Characterization of hippo pathway components by gene inactivation. Mol. Cell 64 (5), 993–1008. doi:10.1016/j.molcel.2016.10.034
Pors, S. E., Harethardottir, L., Olesen, H. O., Riis, M. L., Jensen, L. B., Andersen, A. S., et al. (2020). Effect of sphingosine-1-phosphate on activation of dormant follicles in murine and human ovarian tissue. Mol. Hum. Reprod. 26 (5), 301–311. doi:10.1093/molehr/gaaa022
Potdar, N., Gelbaya, T. A., and Nardo, L. G. (2014). Oocyte vitrification in the 21st century and post-warming fertility outcomes: A systematic review and meta-analysis. Reprod. Biomed. Online 29 (2), 159–176. doi:10.1016/j.rbmo.2014.03.024
Qi, S., Zhu, Y., Liu, X., Li, P., Wang, Y., Zeng, Y., et al. (2022). WWC proteins mediate LATS1/2 activation by Hippo kinases and imply a tumor suppression strategy. Mol. Cell 82 (10), 1850–1864.e7. doi:10.1016/j.molcel.2022.03.027
Quirk, S. M., Cowan, R. G., and Harman, R. M. (2006). The susceptibility of granulosa cells to apoptosis is influenced by oestradiol and the cell cycle. J. Endocrinol. 189 (3), 441–453. doi:10.1677/joe.1.06549
Reddy, P., Zheng, W., and Liu, K. (2010). Mechanisms maintaining the dormancy and survival of mammalian primordial follicles. Trends Endocrinol. Metab. 21 (2), 96–103. doi:10.1016/j.tem.2009.10.001
Ren, Y., Cowan, R. G., Migone, F. F., and Quirk, S. M. (2012). Overactivation of hedgehog signaling alters development of the ovarian vasculature in mice. Biol. Reprod. 86 (6), 174. doi:10.1095/biolreprod.112.099176
Reynier, P., May-Panloup, P., Chretien, M. F., Morgan, C. J., Jean, M., Savagner, F., et al. (2001). Mitochondrial DNA content affects the fertilizability of human oocytes. Mol. Hum. Reprod. 7 (5), 425–429. doi:10.1093/molehr/7.5.425
Richards, J. S., Ren, Y. A., Candelaria, N., Adams, J. E., and Rajkovic, A. (2018). Ovarian follicular theca cell recruitment, differentiation, and impact on fertility: 2017 update. Endocr. Rev. 39 (1), 1–20. doi:10.1210/er.2017-00164
Russell, J. O., and Camargo, F. D. (2022). Hippo signalling in the liver: Role in development, regeneration and disease. Nat. Rev. Gastroenterol. Hepatol. 19 (5), 297–312. doi:10.1038/s41575-021-00571-w
Santos, T. A., El Shourbagy, S., and St John, J. C. (2006). Mitochondrial content reflects oocyte variability and fertilization outcome. Fertil. Steril. 85 (3), 584–591. doi:10.1016/j.fertnstert.2005.09.017
Sha, Q. Q., Zheng, W., Wu, Y. W., Li, S., Guo, L., Zhang, S., et al. (2020). Dynamics and clinical relevance of maternal mRNA clearance during the oocyte-to-embryo transition in humans. Nat. Commun. 11 (1), 4917. doi:10.1038/s41467-020-18680-6
Shah, J. S., Sabouni, R., Cayton Vaught, K. C., Owen, C. M., Albertini, D. F., and Segars, J. H. (2018). Biomechanics and mechanical signaling in the ovary: A systematic review. J. Assist. Reprod. Genet. 35 (7), 1135–1148. doi:10.1007/s10815-018-1180-y
Siegel, R. L., Miller, K. D., and Jemal, A. (2020). Cancer statistics, 2020. CA Cancer J. Clin. 70 (1), 7–30. doi:10.3322/caac.21590
Skinner, M. K. (2005). Regulation of primordial follicle assembly and development. Hum. Reprod. Update 11 (5), 461–471. doi:10.1093/humupd/dmi020
Slager, H. G., Good, M. J., Schaart, G., Groenewoud, J. S., and Mummery, C. L. (1992). Organization of non-muscle myosin during early murine embryonic differentiation. Differentiation 50 (1), 47–56. doi:10.1111/j.1432-0436.1992.tb00485.x
Smith, G. D., Serafini, P. C., Fioravanti, J., Yadid, I., Coslovsky, M., Hassun, P., et al. (2010). Prospective randomized comparison of human oocyte cryopreservation with slow-rate freezing or vitrification. Fertil. Steril. 94 (6), 2088–2095. doi:10.1016/j.fertnstert.2009.12.065
Stein, C., Bardet, A. F., Roma, G., Bergling, S., Clay, I., Ruchti, A., et al. (2015). YAP1 exerts its transcriptional control via TEAD-mediated activation of enhancers. PLoS Genet. 11 (8), e1005465. doi:10.1371/journal.pgen.1005465
Steinhart, Z., and Angers, S. (2018). Wnt signaling in development and tissue homeostasis. Development 145 (11), dev146589. doi:10.1242/dev.146589
Stigliani, S., Moretti, S., Anserini, P., Casciano, I., Venturini, P. L., and Scaruffi, P. (2015). Storage time does not modify the gene expression profile of cryopreserved human metaphase II oocytes. Hum. Reprod. 30 (11), 2519–2526. doi:10.1093/humrep/dev232
Sturmey, R. G., Reis, A., Leese, H. J., and McEvoy, T. G. (2009). Role of fatty acids in energy provision during oocyte maturation and early embryo development. Reprod. Domest. Anim. 44, 50–58. doi:10.1111/j.1439-0531.2009.01402.x
Sun, T., and Diaz, F. J. (2019). Ovulatory signals alter granulosa cell behavior through YAP1 signaling. Reprod. Biol. Endocrinol. 17 (1), 113. doi:10.1186/s12958-019-0552-1
Sun, X., Niu, X., Qin, N., Shan, X., Zhao, J., Ma, C., et al. (2021). Novel insights into the regulation of LATS2 kinase in prehierarchical follicle development via the Hippo pathway in hen ovary. Poult. Sci. 100 (12), 101454. doi:10.1016/j.psj.2021.101454
Suzuki, N., Yoshioka, N., Takae, S., Sugishita, Y., Tamura, M., Hashimoto, S., et al. (2015). Successful fertility preservation following ovarian tissue vitrification in patients with primary ovarian insufficiency. Hum. Reprod. 30 (3), 608–615. doi:10.1093/humrep/deu353
Tanaka, Y., Park, J. H., Tanwar, P. S., Kaneko-Tarui, T., Mittal, S., Lee, H. J., et al. (2012). Deletion of tuberous sclerosis 1 in somatic cells of the murine reproductive tract causes female infertility. Endocrinology 153 (1), 404–416. doi:10.1210/en.2011-1191
Tang, Y., Rowe, R. G., Botvinick, E. L., Kurup, A., Putnam, A. J., Seiki, M., et al. (2013). MT1-MMP-dependent control of skeletal stem cell commitment via a β1-integrin/YAP/TAZ signaling axis. Dev. Cell 25 (4), 402–416. doi:10.1016/j.devcel.2013.04.011
Trebichalska, Z., Kyjovska, D., Kloudova, S., Otevrel, P., Hampl, A., and Holubcova, Z. (2021). Cytoplasmic maturation in human oocytes: An ultrastructural study †. Biol. Reprod. 104 (1), 106–116. doi:10.1093/biolre/ioaa174
Tsoi, M., Morin, M., Rico, C., Johnson, R. L., Paquet, M., Gevry, N., et al. (2019). Lats1 and Lats2 are required for ovarian granulosa cell fate maintenance. FASEB J. 33 (10), 10819–10832. doi:10.1096/fj.201900609R
Van Blerkom, J. (2011). Mitochondrial function in the human oocyte and embryo and their role in developmental competence. Mitochondrion 11 (5), 797–813. doi:10.1016/j.mito.2010.09.012
Vanselow, J., Christenson, L. K., and Pate, J. L. (2020). Editorial: Regulation of dynamic changes and remodeling events during the formation, rescue and regression of the corpus luteum. Front. Endocrinol. (Lausanne) 11, 244. doi:10.3389/fendo.2020.00244
Vanuytsel, T., Senger, S., Fasano, A., and Shea-Donohue, T. (2013). Major signaling pathways in intestinal stem cells. Biochim. Biophys. Acta 1830 (2), 2410–2426. doi:10.1016/j.bbagen.2012.08.006
Varelas, X., Miller, B. W., Sopko, R., Song, S., Gregorieff, A., Fellouse, F. A., et al. (2010). The Hippo pathway regulates Wnt/beta-catenin signaling. Dev. Cell 18 (4), 579–591. doi:10.1016/j.devcel.2010.03.007
Varelas, X., Sakuma, R., Samavarchi-Tehrani, P., Peerani, R., Rao, B. M., Dembowy, J., et al. (2008). TAZ controls Smad nucleocytoplasmic shuttling and regulates human embryonic stem-cell self-renewal. Nat. Cell Biol. 10 (7), 837–848. doi:10.1038/ncb1748
Wada, K., Itoga, K., Okano, T., Yonemura, S., and Sasaki, H. (2011). Hippo pathway regulation by cell morphology and stress fibers. Development 138 (18), 3907–3914. doi:10.1242/dev.070987
Wang, H. X., Gillio-Meina, C., Chen, S., Gong, X. Q., Li, T. Y., Bai, D., et al. (2013). The canonical WNT2 pathway and FSH interact to regulate gap junction assembly in mouse granulosa cells. Biol. Reprod. 89 (2), 39. doi:10.1095/biolreprod.113.109801
Wang, H. X., Li, T. Y., and Kidder, G. M. (2010). WNT2 regulates DNA synthesis in mouse granulosa cells through beta-catenin. Biol. Reprod. 82 (5), 865–875. doi:10.1095/biolreprod.109.080903
Wang, S., Zhou, L., Ling, L., Meng, X., Chu, F., Zhang, S., et al. (2020). The crosstalk between hippo-YAP pathway and innate immunity. Front. Immunol. 11, 323. doi:10.3389/fimmu.2020.00323
Wijgerde, M., Ooms, M., Hoogerbrugge, J. W., and Grootegoed, J. A. (2005). Hedgehog signaling in mouse ovary: Indian hedgehog and desert hedgehog from granulosa cells induce target gene expression in developing theca cells. Endocrinology 146 (8), 3558–3566. doi:10.1210/en.2005-0311
Wong, K. K., Li, W., An, Y., Duan, Y., Li, Z., Kang, Y., et al. (2015). β-Spectrin regulates the hippo signaling pathway and modulates the basal actin network. J. Biol. Chem. 290 (10), 6397–6407. doi:10.1074/jbc.M114.629493
Wu, H., Yu, X. L., Guo, X. F., Zhang, F., Pei, X. Z., Li, X. X., et al. (2017). Effect of liquid helium vitrification on the ultrastructure and related gene expression of mature bovine oocytes after vitrifying at immature stage. Theriogenology 87, 91–99. doi:10.1016/j.theriogenology.2016.08.010
Xiang, C., Li, J., Hu, L., Huang, J., Luo, T., Zhong, Z., et al. (2015). Hippo signaling pathway reveals a spatio-temporal correlation with the size of primordial follicle pool in mice. Cell Physiol. Biochem. 35 (3), 957–968. doi:10.1159/000369752
Xie, Q., Cheng, Z., Chen, X., Lobe, C. G., and Liu, J. (2017). The role of Notch signalling in ovarian angiogenesis. J. Ovarian Res. 10 (1), 13. doi:10.1186/s13048-017-0308-5
Xu, W., Yang, Z., Xie, C., Zhu, Y., Shu, X., Zhang, Z., et al. (2018). PTEN lipid phosphatase inactivation links the hippo and PI3K/Akt pathways to induce gastric tumorigenesis. J. Exp. Clin. Cancer Res. 37 (1), 198. doi:10.1186/s13046-018-0795-2
Yan, L., Yang, M., Guo, H., Yang, L., Wu, J., Li, R., et al. (2013). Single-cell RNA-Seq profiling of human preimplantation embryos and embryonic stem cells. Nat. Struct. Mol. Biol. 20 (9), 1131–1139. doi:10.1038/nsmb.2660
Yimlamai, D., Christodoulou, C., Galli, G. G., Yanger, K., Pepe-Mooney, B., Gurung, B., et al. (2014). Hippo pathway activity influences liver cell fate. Cell 157 (6), 1324–1338. doi:10.1016/j.cell.2014.03.060
Young, J. M., and McNeilly, A. S. (2010). Theca: The forgotten cell of the ovarian follicle. Reproduction 140 (4), 489–504. doi:10.1530/REP-10-0094
Yu, C., Ji, S. Y., Dang, Y. J., Sha, Q. Q., Yuan, Y. F., Zhou, J. J., et al. (2016). Oocyte-expressed yes-associated protein is a key activator of the early zygotic genome in mouse. Cell Res. 26 (3), 275–287. doi:10.1038/cr.2016.20
Yu, F. X., Zhao, B., and Guan, K. L. (2015). Hippo pathway in organ size control, tissue homeostasis, and cancer. Cell 163 (4), 811–828. doi:10.1016/j.cell.2015.10.044
Yu, J., Poulton, J., Huang, Y. C., and Deng, W. M. (2008). The hippo pathway promotes Notch signaling in regulation of cell differentiation, proliferation, and oocyte polarity. PLoS One 3 (3), e1761. doi:10.1371/journal.pone.0001761
Zeng, J., Sun, Y., Li, X., Zhu, J., Zhang, W., Lu, W., et al. (2020). 2,5-Hexanedione influences primordial follicular development in cultured neonatal mouse ovaries by interfering with the PI3K signaling pathway via miR-214-3p. Toxicol. Appl. Pharmacol. 409, 115335. doi:10.1016/j.taap.2020.115335
Zhang, Z. Y., Yu, X. L., Cai, M. D., Liu, Y. H., Liu, J. Q., Zhao, S. Y., et al. (2020). Relationship between bovine oocytes developmental competence and mRNA expression of apoptotic and mitochondrial genes following the change of vitrification temperatures and cryoprotectant concentrations. Cryobiology 97, 110–122. doi:10.1016/j.cryobiol.2020.09.009
Zhao, B., Li, L., Lu, Q., Wang, L. H., Liu, C. Y., Lei, Q., et al. (2011). Angiomotin is a novel Hippo pathway component that inhibits YAP oncoprotein. Genes Dev. 25 (1), 51–63. doi:10.1101/gad.2000111
Zhou, D., Zhang, Y., Wu, H., Barry, E., Yin, Y., Lawrence, E., et al. (2011). Mst1 and Mst2 protein kinases restrain intestinal stem cell proliferation and colonic tumorigenesis by inhibition of Yes-associated protein (Yap) overabundance. Proc. Natl. Acad. Sci. U. S. A. 108 (49), E1312–E1320. doi:10.1073/pnas.1110428108
Zhou, X., Chen, N., Xu, H., Zhou, X., Wang, J., Fang, X., et al. (2020). Regulation of Hippo-YAP signaling by insulin-like growth factor-1 receptor in the tumorigenesis of diffuse large B-cell lymphoma. J. Hematol. Oncol. 13 (1), 77. doi:10.1186/s13045-020-00906-1
Keywords: ovarian development, follicle, Hippo pathway, YAP, follicle activation
Citation: Zhu M, Xu M, Zhang J and Zheng C (2023) The role of Hippo pathway in ovarian development. Front. Physiol. 14:1198873. doi: 10.3389/fphys.2023.1198873
Received: 02 April 2023; Accepted: 22 May 2023;
Published: 02 June 2023.
Edited by:
Michelle M. Collins, University of Saskatchewan, CanadaReviewed by:
Linjun Hong, South China Agricultural University, ChinaMartin Stimpfel, University Medical Centre Ljubljana, Slovenia
Copyright © 2023 Zhu, Xu, Zhang and Zheng. This is an open-access article distributed under the terms of the Creative Commons Attribution License (CC BY). The use, distribution or reproduction in other forums is permitted, provided the original author(s) and the copyright owner(s) are credited and that the original publication in this journal is cited, in accordance with accepted academic practice. No use, distribution or reproduction is permitted which does not comply with these terms.
*Correspondence: Cuihong Zheng, Y2h6aGVuZ0B0amgudGptdS5lZHUuY24=