- Department of Animal and Poultry Science, Faculty of Agricultural Technology, College of Agriculture and Natural Resources, University of Tehran, Tehran, Iran
Supplement of ω-3 fatty acids can decrease the harmful effects of stress. However, the potential molecular mechanisms that are modulated by dietary ω-3 fatty acids in laying hens under stress remain unknown. Hence, RNA-sequencing (RNA-Seq) technology was used to gain new insights into different gene expression profiles and potential pathways involved in response to stress in the liver of 35-week-old Lohmann LSL-Lite laying hens supplemented with ω-3. Three groups including control (non-stress), stress, and stress_ω-3 fatty acids (three layers per each group) were applied. A total of 1,321 genes were detected as differentially expressed genes of which 701, 1,049, and 86 DEGs belonged to stress vs. control, stress_ω-3 vs. control, and stress vs. stress_ω-3 pairwise comparisons, respectively. Gene ontology and KEGG pathway analysis indicated that the DEGs were enriched in particular regulation of steroid and cholesterol biosynthetic process, fatty acid degradation, AMPK signaling pathway, fatty acid biosynthesis, and immune response. Our data represented a promising approach regarding the importance of ω-3 as anxiolytic and anti-stress. In this context, UNC13B and ADRA1B genes were downregulated in the stress_ω-3 group compared to the stress group, which are associated with decreased activity of glutamatergic stimulatory neurons and probably play important role in facilitating the response to stress. This study extends the current understanding of the liver transcriptome response to physiological stress, and provides new insights into the molecular responses to stress in laying hens fed a diet supplemented with ω-3 fatty acids.
1 Introduction
Laying-type birds reared under commercial conditions are introduced to various stressors such as high density, climate changes, nutritional constraints, fear, infection, and metabolic diseases (Thaxton et al., 2006; Delezie et al., 2007). In addition, domestication and genetic selection for higher egg production and better feed conversion ratio have made birds susceptible to a variety of stresses (Surai and Fisinin, 2016). In the face of stressors, glucocorticoid (GCs) hormones are released from the hypothalamic-pituitary-adrenal (HPA) axis. GCs cause metabolic changes such as the stimulation of hepatic gluconeogenesis, inhibition of glucose uptake by peripheral tissues and suppression of insulin (Romero et al., 2009), mobilization of energy stores, increased lipogenesis and lipid storage (Yuan et al., 2008), inhibition of reproductive activity, and increase in anxiety (Love et al., 2004). GCs can regulate lipid metabolism in adipose tissue, liver, and other organs (Roldan and Herzig, 2015). Genome-wide analysis of glucocorticoid-regulated target genes has shown that the glucocorticoid receptor controls some aspects of the hepatic energy metabolism (Le et al., 2005). It has been reported that more than 50 genes are directly affected by the regulatory actions of GCs (Roldan and Herzig, 2015). In birds, the liver is a central metabolic organ that functions to control the metabolism of glucose and lipid. It is well understood that the liver is a place of some metabolic changes such as gluconeogenesis, changes in lipid composition, and hepatic steatosis which all can be stimulated by GCs. GCs have strong effects on the energy balance of the body, and they are counter-regulatory hormones with broad effects on lipid metabolism. It has been reported that chronic stress reduces docosahexaenoic acid (as an ω-3 fatty acid) content in the phospholipid membranes of glutamatergic neurons at the amygdala, while it increases arachidonic acid content, which in turn inhibits the release of gamma-aminobutyric acid (GABA) from presynaptic neurons (Pérez et al., 2013). Given that, GABA is considered an inhibitory neurotransmitter in the brain. Inhibition of GABA release may increase excitatory neuronal activity in the amygdala, and enhance anxiety. Previous studies reported that ω-3 supplementation increases serotonin levels in the brain of stressed rats (Gross et al., 2002). Serotonin has a vital role in the regulation of anxiety-like behaviors. Studies in humans and mice have revealed the modulating effects of ω-3 fatty acids on the HPA axis and the reduction of glucocorticoid concentrations (Ferraz et al., 2011; Barbadoro et al., 2013). In addition to the anti-stress effects of ω-3 fatty acids in the brain, it has been reported that these compounds reduce the accumulation of triglycerides in the liver through increased and decreased lipid oxidation and triglyceride biosynthesis, respectively (Clarke, 2001). Most of the studies concerning stress in laying hens have been mainly focused on animal performance and biometric traits evaluation and the molecular mechanisms underlying these processes are still unclear. On the other hand, to the author’s best knowledge, the use of ω-3 fatty acids supplementation in stressed birds has never been evaluated for modulating stress. Previous studies have employed reverse transcription polymerase chain reaction (RT-PCR) to explore differentially expressed genes related to the mechanism of action of glucocorticoids and the effects of stress in poultry. In this regard, Wang et al. (2013) suggested that corticosterone decreased the availability of circulating yolk precursors by decreased apolipoprotein synthesis in the liver of laying hens. They also reported the upregulated expression of occludin and claudin in granulosa cells, preventing yolk deposition into oocytes. In another study, Duan et al. (2014) showed that chronic corticosterone administration causes cholesterol accumulation in the pectoralis major muscle of broiler chickens by up-regulating the HMGCR mRNA expression. Recently, transcriptome analysis of liver tissue among three distinct genetic chicken lines (heat-susceptible broiler line, heat-resistant Fayoumi line, and their advanced intercross line) suggested that angiopoietin-like 4 is a candidate gene for improvement of heat tolerance in chickens (Lan et al., 2016). Previous studies have evaluated the effects of ω-3 fatty acid on the rodent brain and identified genes involved in neuronal function, stress reactivity, and energy metabolism (Le-Niculescu et al., 2011; Begg et al., 2012). Transcriptome analysis is a powerful approach in functional genomics for a deeper understanding of complicated physiological pathways, such as the lipid metabolism (Bakhtiarizadeh and Salami, 2019). Recently, next-generation sequencing (NGS) has been revolutionizing the field of comprehensive gene expression profiling. In this regard, RNA sequencing (RNA-Seq) is currently the method of choice for performing genome-wide transcriptome analysis. This technology can provide new insights to understand the molecular basis of different biological processes (Bakhtiarizadeh et al., 2019).
To date, little is known about the exact molecular regulatory mechanisms of stress in lipid metabolism. The comprehension of the underlying molecular mechanism is potentially critical to understand the effect of ω-3 fatty acid on stress in birds. Toward this goal, laying hens were used as a model for the study of physiological stress. Then, the effects of physiological stress in laying hens fed by a diet supplemented with ω-3 were assessed to identify differentially expressed genes and explore the underlying molecular regulatory mechanisms. This study can be helpful in identifying candidate genes and molecular pathways involved in response to stress in laying hens.
2 Materials and methods
2.1 Ethics approval
All methods were carried out in accordance with relevant guidelines and regulations. All experimental protocols were approved by a research council of the University of Tehran.
2.2 Trial design
A total of forty-eight 35-week-old Lohmann LSL-Lite laying hens were used in this experiment. Layers were randomly assigned to three trial groups: 1) control (non-stress, no ω-3), 2) stress, birds supplemented by dexamethasone (DEX) (1.5 mg/kg diet), 3) stress_ω-3 fatty acids, stressed birds supplemented with ω-3 (0.48% of diet). Four replicates and four birds per each replicate (16 birds) were considered per each group. DEX is a potent synthetic glucocorticoid and has been suggested in the previous study due to its capability to simulate the effects of the glucocorticoid (Foucaud et al., 1998). Therefore, in the present study, DEX in experimental diets was applied to build a stress model. DEX tablets (Iran Hormone Co., Iran) was dissolved in oil and mixed with a mash diet. Salomega (Agritech, Ireland) was used as an ω-3 fatty acids source to supply the fatty acids desired in the experiment. Salomega is prepared using salmon oil, and corn cob is used as a carrier in this product. It contains 52% fat and about 17% total omega 3 fatty acids. From age of 35–41 weeks, birds were fed by a basal diet supplemented with ω-3 fatty acids. At the beginning of the age of 41 weeks, the stressed birds were continuously fed diets containing DEX (1.5 mg/kg diet) for 1 week (end of 41 weeks). The birds were allocated in 12 cages equipped with 1 drinker and 1 feeder. Cages were 40 × 50 × 35 cm and contained four hens. All birds had ad libitum access to water and a basal diet that met all nutritional recommendations of laying hens (Table 1, Lohmann laying hen’s manual). The hens were maintained under similar conditions with (a controlled photoperiod regimen 16:8 h light: dark cycle, temperature 22°C–23°C and relative humidity of 50%–60%). At the end of 41 weeks of age, nine birds (n = 3 birds/group) were randomly chosen and were weighed and slaughtered by cervical dislocation. Liver tissue samples were immediately collected, snap-frozen in liquid nitrogen, and stored at 80°C for RNA extraction and RNA-sequencing.
2.3 Performance
The feed intake, egg number and egg weight were recorded during the stress period, and then the egg production, egg mass, daily feed intake, and feed conversion ratio (FCR) (feed/egg mass) were calculated. All the means of experimental treatments were analyzed by analysis of variance using General Linear Models procedure of SAS (version 9.4). Differences among treatment means were tested using the Tukey test and statistical differences declared at p < 0.05.
2.4 RNA extraction and transcriptome sequencing
Total RNA was isolated from nine liver samples using TRIzol reagent (Invitrogen, Carlsbad, CA) according to the manufacturer’s protocol. The RNA quality was determined using the Agilent Bioanalyzer 2100 system. The samples were sent to BGI company (Shenzhen, China) to construct a cDNA library and RNA sequencing. Only samples with an RNA integrity number (RIN) greater than 8.5 were used for cDNA library construction. The sequencing was performed on an Illumina HiSeq 2500 platform by paired-end strategy (read length 150 bp).
2.5 Quality control and read trimming
The quality of the raw reads was assessed using FastQC (v0.11.5) program to detect common issues in RNA-Seq data. Adapters and low-quality bases were trimmed with Trimmomatic using the options: TRAILING:20, MAXINFO:120:0.9, MINLEN:120. The quality of the reads was re-assessed with FastQC after trimming to confirm quality improvements.
2.6 Mapping to genome and identification of differentially expressed genes (DEGs)
Two important steps in RNA-Seq studies are mapping of cleaned reads to the reference genome and normalization and statistical analysis to identify DEGs. In the present study, to increase the accuracy of the identified DEGs our previous pipeline was applied (Bakhtiarizadeh et al., 2019), as the results of two alignment tools (Hisat2 and STAR) and two statistical methods (edgeR and DESeq2) were combined. In this regard, four different combinations including 1) Hisat2 + edgeR, 2) Hisat2 + DESeq2, 3) STAR + edgeR and 4) STAR + DESeq2 were assessed. These tools were selected since they are reliable and robust and also widely used in transcriptome analysis-related studies (Baruzzo et al., 2017; Everaert et al., 2017). Only those genes were considered for further analysis that was identified as DEGs by the four approaches. To do this end, the clean reads were mapped to the reference chicken genome (GRCg6a) using STAR (version 2.5.3a) (Dobin et al., 2013) and Hisat2 software (version 2.1.0) based on the default parameters (Kim et al., 2015). Additionally, HTSeq-count (Python package HTSeq, python v 2.7.3) software was used to count the reads numbers mapped to each gene using the reference annotation file (version 96). Gene expression was quantified as the total number of reads for each sample that uniquely aligned to the reference genome. Differential expression analyses between stress birds versus control (S/C), stress_ω-3 versus control (S3/C), and stress_ω-3 versus stress (S3/S) were performed using two count-based methods in R packages including edgeR (version 3.18.1) (Robinson et al., 2010) and DESeq2 (version 1.16.1). Using a generalized linear model based on the negative binomial distribution. A principal component analysis (PCA) was also employed to assess sample relationships based on voom-transformed values of raw read counts (Law et al., 2014), calculated by the Hisat2+DESEq2 approach.
2.7 Gene ontology (GO) and pathway analysis
The DEG lists were submitted to Enrichr web-based tool (Kuleshov et al., 2016) for functional enrichment analysis. This analysis was performed to identify the biological processes and Kyoto Encyclopedia of Genes and Genomes (KEGG) pathways significantly enriched in DEGs. Adjusted p-values less than 0.05 were considered as significantly enriched terms.
2.8 Real-time PCR analysis
The expression levels of eight DEGs were evaluated by RT-PCR to validate the accuracy of RNA-Seq results, with the RNA samples used for RNA-Seq. Three biological replicates along with two technical replicates were used for each gene. First-strand cDNA synthesis was performed by first-strand cDNA synthesis kit (Thermo Fisher, Co., United States) according to the manufacturer’s guideline. RT-PCR was carried out by Rotor-Gene 6,000 instrument (Corbett Research, Australia) with the Ampliqon Kit (Denmark), according to the manufacturer’s protocol. RT-PCR thermocycling conditions were as follows: an initial 1 cycle at 95°C for 900 s, 40 cycles at 95°C for 15 s, 60°C for 20 s, 72 °C for 20, and a final elongation at 72°C for 1,450 s. All the primer sequences were designed using Primer3Plus software and were blasted against the chicken genome using the national center for biotechnology information (NCBI) Primer-BLAST software to guarantee unique production. The genes and related primer sequences are listed in Table 2. CT-method was applied to quantify changes in gene expression and GAPDH and 18s rRNA genes were used as reference genes for the normalization of gene expression levels. The relative gene expression values were calculated using the Delta-Delta Ct method and calculated fold changes were compared with RNA-Seq results.
3 Results
3.1 Laying performance
The effects of stress and dietary ω-3 fatty acids supplementation on the performance of laying hens are shown in Table 3. Body weight were not affected (p > 0.05) by the trial treatments. However, it tended to be decreased in response to stress.
Induction physiological stress reduced feed intake, egg production and egg mass (p < 0.05). Stressed birds that fed on diet containing ω-3 fatty acids had lower feed intake, egg production and egg mass compared to the other groups. Trial tretments had no significant effect on FCR (p > 0.05).
3.2 Alignment and mapping of the chicken genome
To investigate the molecular mechanisms involved in response to the physiological stress of laying hen fed by ω-3 fatty acids, three treatment groups including control, stress, and stress_ω-3 were considered and cDNA libraries from nine chicken liver tissues (three per treatment group) were constructed for high throughput RNA sequencing. RNA-Seq generated around 219,189,680 paired-end reads ranging from 24,023,561 to 24,614,348, with an average of 24,273,029 in control, 24,411,997 in stress and 24,378,201 in stress_ω-3 treatment group. For control, stress, and stress_ω-3 groups, an average of 24,272,851, 24,411,859, and 24,378,088 reads per sample passed the quality control. On average, 85% and 79% of all the clean reads were aligned to the chicken genome by Hisat2 and STAR tools, respectively. Detailed information on raw and clean reads along with mapping results are presented in Table 4.
3.3 Differentially expressed genes
Across all samples, a total of 16,595 genes were identified to be expressed (read counts >0), accounting for 68% of the total annotated genes. PCA analysis was performed to check the level of similarity/dissimilarity in the gene expression profiles of the three treatments. This analysis shows if samples from the same treatment cluster together or not. Results of this analysis based on the first two principal components showed that all the samples can be divided into three clusters according to their treatments (Figure 1). Hence, the differences in the transcriptome profiles can be appropriate for detecting putative candidate genes explaining the known differences that exist among the treatments.
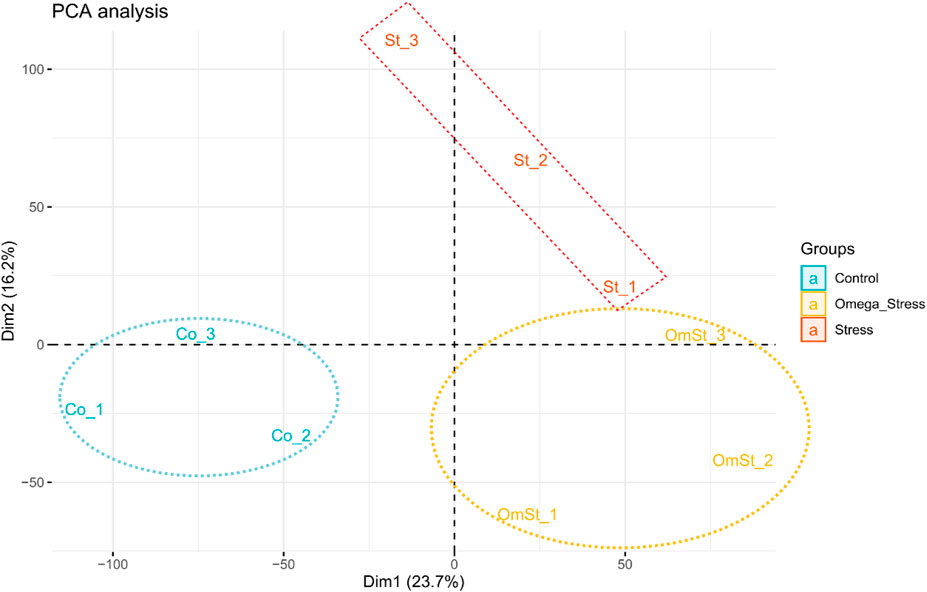
FIGURE 1. PCA plot of gene expression profiles of the treatments. This plot shows the variance of the three biological replicates of each of the three treatments. The percentages on each axis represent the percentages of variation explained by the principal components.
To explore the transcriptional changes of the genes affected by the applied treatments, DEGs were identified by three pairwise comparisons using two well-established statistical analysis methods including DESeq2 and edgeR. Therefore, four approaches were used to identify DEGs: Hisat2 + edgeR, Hisat2 + DESeq2, STAR + edgeR and STAR + DESeq2. A total of 1,321 DEGs were identified among the three comparisons; the majority of DEGs were generated in S/C and S3/C comparisons. The comparative profile of the DEGs in all the comparisons revealed that more numbers of DEGs were downregulated. A total of 701 DEGs were detected in S/C, with 302 upregulated and 399 downregulated genes in the stress group. A total of 1,049 DEGs were identified in S3/C, with 428 upregulated and 621 downregulated genes in the stress ω-3 group. Only 86 DEGs were observed in S3/S, with 31 upregulated and 55 downregulated genes in the stressed ω-3 group. The shared DEGs among pairwise comparisons are depicted as Venn Diagrams in Figure 2. There was a significant increase in DEGs detected in both S/C and S3/C comparisons than S/S3. Furthermore, the highest number of common DEGs were detected in the comparison of the control group against under-stress treatments (453 genes). Only five genes were commonly identified as DEGs in all comparisons including calcium-regulated heat stable protein 1 (CARHSP1), discoidin CUB and LCCL domain containing 1 (DCBLD1), acetyl-CoA carboxylase alpha (ACACA), macrophage stimulating 1 receptor (MST1R) and cytochrome P450, family 1, subfamily A, polypeptide 2 (CYP1A2).
3.4 Functional enrichment analysis
To explore the potential functions of stress-responsive genes, DEGs from each of the pairwise comparisons were subjected to functional enrichment analysis, as upregulated and downregulated DEGs were treated separately. Comparing the stress and control groups of genes with different expressions, it was observed that 302 upregulated DEGs were significantly enriched in 117 biological processes (BP) terms and 22 KEGG pathways, while 399 downregulated DEGs were significantly enriched in 212 BP terms and 38 KEGG pathways (Supplementary File S1). The most important enriched BP terms and KEGG pathways are shown in Figure 3. When comparing the stress_ω-3 and control groups, 428 upregulated and 621 downregulated DEGs were enriched significantly in 149 and 223 BP terms and 11 and 30 KEGG pathways, respectively (Supplementary File S2). Figure 4 indicates the most notably enriched BP terms and KEGG pathways. Finally, 31 upregulated and 55 downregulated DEGs were enriched in102 and 136 BP terms as well as 13 and 12 KEGG pathways, respectively (p < 0.5, Supplementary File S3), according to the comparison between stress_ω-3 and stress groups. The considerably enriched BP terms and KEGG pathways are shown in Figure 5. Most of the significant terms or pathways in control against under stress groups comparisons were related to lipid metabolism such as fatty acid oxidation, cholesterol biosynthetic process, biosynthesis of unsaturated fatty acids, steroid biosynthesis, fatty acid biosynthesis, reverse cholesterol transport, acyl-CoA biosynthetic process, and cholesterol efflux. Other important GO terms and KEGG pathways enriched in insulin resistance, AMPK signaling pathway, cortisol synthesis and secretion, steroid hormone biosynthesis, cellular response to oxidative stress, bile secretion, and peroxisome included. The functional analysis of S/S3 indicated that most of the upregulated DEGs were significantly enriched in immune response-related biological processes or pathways, while most downregulated DEGs were enriched in lipid and glucose metabolism as well as neurotransmission biological processes or pathways. Some important enriched terms related to immune response and nervous system were lymphocyte proliferation, negative regulation of interleukin-6 production, negative regulation of interleukin-6 production, peripheral nervous system development and synaptic transmission, and glutamatergic. Taken together of all functional enrichment analysis results, the following biological processes and KEGG pathways were the most affected: cholesterol metabolism, ATP-binding cassette (ABC) transporters, insulin resistance, AMPK signaling pathway, fatty acid degradation, and sterol metabolic process.
3.5 Validation of differentially expressed genes by RT-PCR
To confirm the results obtained from RNA-Seq, eight DEGs were randomly selected from both upregulated (SQLE, SCD, CYP51A1, and MSMO1) and downregulated (APOC3, ACOX1, PPARGC1A, and CPT1A) genes. Although fold changes varied between the two methods, however, all the genes showed a similar expression pattern between RNA-Seq and RT-PCR results, except MSMO1 and CYP51A1 in the S/C comparison (Figure 6). Therefore, RT-PCR and RNA-Seq results were in good agreement, indicating the reliability of the RNA-Seq data.
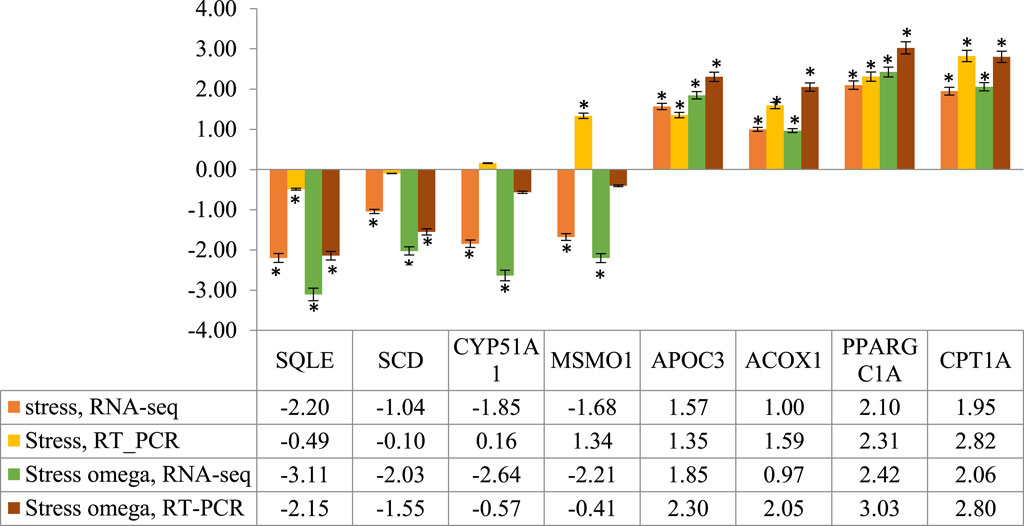
FIGURE 6. RT-PCR validation of the eight randomly selected genes identified by RNA-Seq analysis in stress and stress-ω-3 groups. *The differences between experimental groups are significant at p < 0.05.
4 Discussion
The combination of ω-3 fatty acids and stress reduced feed intake in stressed birds. Decreased feed intake and performance in laying hens and quails has been reported previously following the treatment with corticosterone and dexamethasone (Liu et al., 2012; Berenjian et al., 2018). Activation of the HPA axis as a result of stressors can causes the secretion of the corticotropin-releasing factor (CRF), which is a powerful anorexigenic peptide in birds (Richardson et al., 2000). Gray et al. (2013) reported that ω-3 fatty acids increase leptin levels and reduce appetite in obese patients. On the other hand, Houseknecht et al. (1998) stated that cortisol hormone stimulates the levels of leptin expression.
The feed intake in birds under stress can be diminished by the increased level of leptin as a satiety hormone that is created by the use of ω-3 fatty acids. In general, the synergistic effects of stress and ω-3 fatty acids reduce feed intake of birds. The adverse effects of stress on laying performance investigating by the present paper, are in agreement with other researches (Wang et al., 2013). The reduced productive performance in this study can primarily be due to the reduced feed intake. While reducing feed intake, the energy required to produce yolk precursors in the liver is not provided; resulting in the suppressed development of follicles and ovulation by reducing the availability of yolk precursors. The cumulative effect of stress and omega-3 fatty acids could reduce the feed intake, and the use of omega-3 fatty acids could not improve the negative effects of stress on laying performance as tested at dosage in this research.
The action of GCs involves a high diversity of metabolic effects, such as the stimulation of hepatic gluconeogenesis, proteolysis, and changes in lipid metabolism. High metabolic rate due to GCs has been hypothesized to result in elevated oxidative stress (Lin et al., 2004; Caro et al., 2007). In this study, genes related to lipid and glucose metabolism in the liver were significantly activated by stress induced by DEX. It shows that GCs play an important role in energy metabolism by regulating the genes involved in the metabolic pathways, including the lipid metabolism (Roldan and Herzig, 2015; Hu et al., 2019).
Here, several pathways and biological processes involved in lipid metabolism were enriched in the liver tissue of laying hens under stress. These pathways included cholesterol and steroid biosynthesis, cholesterol metabolism, peroxisome pathway, fatty acid degradation, AMPK signalling pathway, insulin resistance, ABC transporters, and glucagon signalling pathway. Most of the significantly enriched terms were observed in S/C and S3/C comparisons than in S3/S, indicating larger differences in the stress-related treatments transcriptome profiles compared with control conditions, which was supported by the more DEGs in control against the two other treatments.
Cholesterol is an important factor in the biosynthesis of steroids and steroid hormones and is closely related to lipid metabolism. Seven genes were associated with the regulation of cholesterol and steroid biosynthetic process including sterol regulatory element-binding proteins 1/2 (SREBP1/2), 3-hydroxy 3-methyl glutaryl-CoA reductase (HMGCR), fatty acid synthase (FASN), squalene epoxidase (SQLE), stearoyl-CoA desaturase (SCD) and cytochrome P450 family 51subfamily A member 1 (CYP51A1), which were downregulated in stressed birds (stress and stress_ω-3 groups) compared to control birds. SREBPs are located in the center of cholesterol homeostasis. They are a family of transcription factors anchored in the endoplasmic reticulum membrane. SREBPs are responsible for maintaining cholesterol homeostasis, acting on synthesis and uptake in response to the cholesterol depletion (Shimano et al., 1996). SREBP1 plays a key role in the regulation of lipogenesis in birds (Assaf et al., 2003). SREBP2 increases the expression of the HMGCR gene, which is a rate-limiting enzyme in the cholesterol synthesis (Maxfield and Tabas, 2005). SCD is a microsomal enzyme that catalyzes the synthesis of monounsaturated fatty acids (MUFAs) from saturated fatty Acyl-CoAs in the liver tissue (Lefevre et al., 1999). SQLE is an enzyme located in the endoplasmatic reticulum catalyzing the epoxidation of squalene and producing 2,3-oxidosqualene. SQLE is one of the rate-limiting enzymes in the cholesterol biosynthesis (Ono and Imai, 1985). According to the results of this study, decreased expression of SREBPs as transcription factors could reduce the expression of genes involved in lipid and cholesterol synthesis.
CYP51A1 catalyzes the methylation of lanosterol to dimethyl colostrum, the main pathway in the biosynthesis of sterols (Lewińska et al., 2013). In the present study, physiological stress and ω-3 fatty acids reduced the synthesis of cholesterol, steroids, and fatty acids by reducing the expression of the above genes. It was shown that the expression of FASN is regulated by the glucocorticoids (Xu et al., 2009). As shown above, feed intake was reduced in stressed hens and they were exposed to decrease accesses of energy. Reduce energy intake leads to the breakdown of body reserves such as lipids and proteins to produce ATP, while processes like lipid synthesis are limited. However, contrary to this result, the expression of genes involved in cholesterol biosynthesis and fatty acids such as SREBPs, SCD, FASN, HMGCR, and CYP51A1 in laying hens and broilers increased by GCs (Cai et al., 2011; Roldan and Herzig, 2015; Hu et al., 2019). Similar to the results of this study, a reduction in lipid synthesis and accumulation in mice fed with a diet supplemented with ω-3 fatty acids has been previously reported (Valenzuela et al., 2012). ω-3 fatty acids downregulate the expression of transcription factor SREBP-1 with inhibition of the transcription of lipogenic genes such as FASN, acetyl-CoA carboxylase, stearoyl-CoA desaturase-1, which can be led to a decrease in de novo lipogenesis (Valenzuela et al., 2012). Based on the findings of this study, both of stress and ω-3 fatty acids factors reduced lipid synthesis and accumulation in the liver by decreasing the expression of SREBP as the main regulator of lipogenesis in birds.
The next important enriched pathway was cholesterol metabolism. Some important genes in this pathway were cholesteryl ester transfer protein (CETP), apolipoprotein A1 (APOA1), and apolipoprotein C3 (APOC3), which were all upregulated in under stress treatments (S and S3 groups) compared with the control group. These genes play a role in the reverse transport and removal of cholesterol from tissues to the liver (Bolanos-Garcia and Miguel, 2003). In line with these results, the expression of the APOA1 gene was increased in glucocorticoid treatment, which is the main protein involved in HDL particles (Taylor et al., 1996). In fact, increasing the reverse transport of cholesterol to the liver is a response to the decrease in cholesterol synthesis in stressed birds.
The next pathway was fatty acid degradation-related genes such as carnitine palmitoyl transferase 1A (CPT1A), acyl CoA oxidase 1 (ACOX1), and acetyl CoA acyltransferase 1 (ACAA1), which were all upregulated in the stressed groups compared to control group. CPT1A is a rate-limiting enzyme associated with β-oxidation of fatty acids in mitochondria and has a dominant role in the triglyceride metabolism (Zammit, 2008; Akkaoui et al., 2009). CPT1A upregulation increases the oxidation of fatty acids in hepatocytes and reduces the lipid accumulation (Akkaoui et al., 2009). ACOX1 is a flavoenzyme that catalyzes the initial and rate-determining reaction of β-oxidation of fatty acids in the peroxisome, ACOX1 catalyzes the desaturation of acyl-CoAs to 2-trans-enoyl-CoAs (Zeng et al., 2017). ACAA1 encodes an enzyme that cleaves 3-ketoacyl CoA to give acetyl-CoA and acyl-CoA during the fatty acid β-oxidation in the peroxisome (Li et al., 2019). Activation of fatty acid oxidation in stressed birds, shift the function of the liver toward increasing energy supply and reducing anabolism. Dietary supplementation of ω-3 fatty acids in stressed birds also increased oxidation of fatty acids and decreased lipid accumulation in tissue. In line with these views, enhanced fatty acid oxidation was observed in the liver of mice supplemented with ω-3 fatty acids (Breuner and Hahn, 2003).
ABC transporters such as ATP binding cassette subfamily B member 1 (ABCB1), ATP binding cassette subfamily A member 12 (ABCA12), and ATP binding cassette subfamily G member 2 (ABCG2) were upregulated in under stress groups than the control (p < 0.05). The ABC transporters are efflux transporters and play an important role in the absorption, distribution, excretion, and toxicity of the xenobiotics (Tlemsani et al., 2015). Moreover, several genes involved in the AMPK signaling pathway and insulin resistance such as CPT1A, PPARG coactivator 1 alpha (PPARGC1A), glucose-6-phosphatase catalytic subunit (G6PC), and glycogen synthase 2 (GYS2) were upregulated, while SREBP1, Acetyl-CoA carboxylase alpha (ACACA), insulin receptor substrate 1 (IRS1), SCD, HMGCR and FASN in AMPK signaling pathway were downregulated in the under-stress birds compared with the control group. G6PC is involved in the final stages of hepatic glucose production. GYS2 is the inactive form of glycogen synthase, which reduces glycogen synthesis. AMPK is the main sensor of cellular energy status that is activated in response to stress to restore energy balance by inhibiting ATP-consuming pathways and expanding ATP-producing processes. In this regard, SREBP1 a transcriptional regulator of the genes involved in lipid synthesis, biosynthesis, and uptake of cholesterol, is inhibited by AMPK (Li et al., 2011). Similar to our results, it was observed that activation of the AMPK pathway in mammals stimulated hepatic fatty acid oxidation and decreased cholesterol synthesis and De novo lipogenesis (Winder and Hardie, 1999). Also, in rats, the administration of dexamethasone promoted acetyl-CoA carboxylase phosphorylation and increased oxidation of fatty acids, likely by activating the AMPK (Qi et al., 2006).
Insulin resistance was the other activated pathway in birds under stress. Rare mutations of the insulin receptor gene (Baynes et al., 1997) and IRS1 protein (Yoshimura et al., 1997) lead to insulin resistance. It has been shown that high concentrations of hormones such as cortisol, glucagon, and catecholamines are associated with impaired insulin action (Reynolds and Walker, 2003). Insulin resistance induced by GCs can result from an alteration in the molecules involved in the insulin signaling cascade. Glucose intolerance, high blood pressure, and insulin resistance are common in people with high blood cortisol levels (Reynolds and Walker, 2003). Decreased expression of the IRS1 gene in this study, could indicate insulin resistance in laying hens under physiological stress. Consistent with these results, chronic treatment with corticosterone decrease IRS-1 and insulin receptor and evokes insulin resistance in chickens (Dupont et al., 1999). Based on the results of this study, it can be stated that activation of AMPK and insulin resistance pathways, as a result of stress, as well as, the act of ω-3 fatty acids as signaling molecules regulating hepatic lipid metabolism, prevented lipogenesis in liver tissue, whereas, increased oxidation of fatty acids.
These results are in contrast to various experiments and increased lipogenesis, cholesterol synthesis, and hepatic steatosis as a result of the use of GCs or a variety of stresses in different birds. The difference in results can be explained by the fact that the AMPK modulates glucocorticoid action in the target tissues. On the other hand, insulin resistance induced by dexamethasone is associated with several changes along the insulin signaling cascade. Because insulin signaling is required for lipogenic activity of the glucocorticoid in the liver, activation of AMPK and insulin resistance pathways prevented lipogenesis and hepatic steatosis in laying hens under stress.
Cytochrome P450 family 17 subfamily A member 1 (CYP17A1) and phosphodiesterase 8A (PDE8A) are involved in cortisol synthesis and secretion. These genes were upregulated in the liver of stressed birds compared to that in the liver of control birds (S/C comparison). Stress leads to the secretion of stress hormone and subsequently challenge the patterns of physiological response. The CYP17A1 is an important enzyme in the pathway of steroid biosynthesis and converts pregnenolone to 17-hydroxy pregnenolone (Zhang et al., 2019). PDE8A modulates corticosterone secretion in the adrenal gland (Tsai and Beavo, 2011). These findings are in good agreement with previous works as increased synthesis of corticosterone in the birds under stress is reported (Post et al., 2003; Xie et al., 2015).
Genes of acetyl-CoA carboxylase alpha (ACACA) and GYS2 were enriched in the KEGG pathways of AMPK, insulin, and glucagon signaling. These genes were downregulated in a stress ω-3 group compared to the stress group. The fatty acid biosynthesis pathway with one gene (ACACA) was also downregulated in this comparison. ACACA converts acetyl-CoA to malonyl-CoA in fatty acid biosynthesis. These results are similar to the results of comparisons of stressed birds versus control that were previously stated. These findings confirm the role of ω-3 fatty acids in reducing lipid biosynthesis.
Furthermore, we observed increased gene expression of neurofascin (NFASC) and decreased genes expression of unc-13 homolog B (UNC13B) and adrenoceptor alpha 1B (ADRA1B) in the stress_ω-3 group compared to the stress group. Neurofascin is a transmembrane protein that plays an essential role in nervous system development and function of the axon initial, encoded by NFASC gene (Monfrini et al., 2019). ADRA1B is involved in the adrenergic receptor signaling pathway and plays an important role in facilitating the response to the HPA axis during stress. Stimulation of ADRA1B is mainly associated with decreased sodium and potassium conduction and slow depolarization (Bergles et al., 1996). In agreement with these results, the use of dexamethasone in cultured smooth muscle cells increased the expression of the ADRA1B gene (Sakaue and Hoffman, 1991). UNC13B is a presynaptic protein that promotes the priming of synaptic vesicles by acting through syntaxin (Söllner, 2003). UNC13B is involved in the biological term of synaptic transmission, glutamatergic. UNC13B downregulation is associated with decreased activity of glutamatergic stimulatory neurons. Glutamate is a stimulant neurotransmitter in the hippocampus. Increased release of glutamate from pre-synapse neurons has been reported in rodents under chronic stress (Fontella et al., 2004). Chronic corticosterone administration in rats also increased the release of glutamate in the synaptic space (Wang and Wang, 2009). The use of ω-3 fatty acids in this experiment reduced the expression of the genes beta-adrenergic receptors and consequence the glutamatergic signaling pathway decreased. In this regard, it was reported that supplementation of diet with ω-3 fatty acids decreases neurotransmitter release by presynaptic neurons and glutamate receptors in both rodents and humans subjected to stressful situations (Hennebelle et al., 2014). Latour et al. (2013) showed that the supplementation of ω-3 fatty acids improves the uptake of glutamate by astroglia, and in this way, prevents damage and death of neurons due to the accumulation of glutamate in synapses. The present findings suggest that ω-3 fatty acid supplementation decreases glutamatergic activity by reduction of glutamate receptor genes expression. Previous studies have reported that ω-3 fatty acids have anti-stress effects and reduce anxiety and depression in humans and rat (Takeuchi et al., 2003; Pérez et al., 2013). Therefore, according to these results, the use of ω-3 fatty acids in laying hens might have anti-stress effects on this animal.
5 Conclusion
RNA-Seq was applied to identify DEGs, significantly enriched biological processes, and KEGG pathways in laying hens under physiological stress, which were fed a diet supplemented with ω-3 fatty acids. Our data demonstrated a marked effect of stress on the regulation of genes and pathways involved in hepatic lipid metabolism. The DEGs between stress and stress_ω-3 groups versus control were enriched for genes involved in the peroxisome, fatty acid degradation, insulin resistance, AMPK signaling pathway, cholesterol metabolism, and steroid biosynthesis. Activation of the above routes in particular AMPK, insulin resistance pathways, and oxidation of fatty acids indicate a decrease in lipogenesis and lipid accumulation in liver tissue. In other words, both factors, namely, DEX-induced stress in stressed groups and the use of ω-3 fatty acids in the stress_ω-3 group could reduce the synthesis of lipid and increase fatty acid oxidation. Furthermore, the NFASC, UNC13B, and ADRA1B involved in the adrenergic receptor signaling pathway were differentially expressed in the stress_ω-3 group compared to the stress group. It appears that ω-3 fatty acid supplementation may ameliorate dexamethasone-induced stress by reducing activity in the glutamatergic pathway. Further research needs to be conducted to provide a better understanding of the beneficial effects of omega-3 to alleviate the adverse effects of stress on poultry.
Data availability statement
The datasets presented in this study can be found in online repositories. The names of the repository/repositories and accession number(s) can be found in the article/Supplementary Material.
Ethics statement
The animal study was reviewed and approved by Research council of the University of Tehran.
Author contributions
AB, SS, MB, and AM-S conceived and designed the study. AB collected the data. MB analysed all the data. AB and MB contributed to the interpretation of the results. AB and MB wrote the first draft of the manuscript. All authors contributed to the article and approved the submitted version.
Acknowledgments
The authors thank the support of Office of Research Affairs of the University of Tehran.
Conflict of interest
The authors declare that the research was conducted in the absence of any commercial or financial relationships that could be construed as a potential conflict of interest.
Publisher’s note
All claims expressed in this article are solely those of the authors and do not necessarily represent those of their affiliated organizations, or those of the publisher, the editors and the reviewers. Any product that may be evaluated in this article, or claim that may be made by its manufacturer, is not guaranteed or endorsed by the publisher.
Supplementary material
The Supplementary Material for this article can be found online at: https://www.frontiersin.org/articles/10.3389/fphys.2023.1198247/full#supplementary-material
References
Akkaoui, M., Cohen, I., Esnous, C., Lenoir, V., Sournac, M., Girard, J., et al. (2009). Modulation of the hepatic malonyl-CoA–carnitine palmitoyltransferase 1A partnership creates a metabolic switch allowing oxidation of de novo fatty acids. Biochem. J. 420 (3), 429–438. doi:10.1042/BJ20081932
Assaf, S., Hazard, D., Pitel, F., Morisson, M., Alizadeh, M., Gondret, F., et al. (2003). Cloning of cDNA encoding the nuclear form of chicken sterol response element binding protein-2 (SREBP-2), chromosomal localization, and tissue expression of chicken SREBP-1 and-2 genes. Poult. Sci. 82 (1), 54–61. doi:10.1093/ps/82.1.54
Bakhtiarizadeh, M. R., and Salami, S. A. (2019). Identification and expression analysis of long noncoding RNAs in fat-tail of sheep breeds. G3 Genes., Genomes, Genet. 9 (4), 1263–1276. doi:10.1534/g3.118.201014
Bakhtiarizadeh, M. R., Salehi, A., Alamouti, A. A., Abdollahi-Arpanahi, R., and Salami, S. A. (2019). Deep transcriptome analysis using RNA-Seq suggests novel insights into molecular aspects of fat-tail metabolism in sheep. Sci. Rep. 9 (1), 9203–9214. doi:10.1038/s41598-019-45665-3
Barbadoro, P., Annino, I., Ponzio, E., Romanelli, R. M., D'Errico, M. M., Prospero, E., et al. (2013). Fish oil supplementation reduces cortisol basal levels and perceived stress: A randomized, placebo-controlled trial in abstinent alcoholics. Mol. Nutr. Food Res. 57 (6), 1110–1114. doi:10.1002/mnfr.201200676
Baruzzo, G., Hayer, K. E., Kim, E. J., Di Camillo, B., FitzGerald, G. A., and Grant, G. R. (2017). Simulation-based comprehensive benchmarking of RNA-seq aligners. Nat. Methods. 14 (2), 135–139. doi:10.1038/nmeth.4106
Baynes, K., Whitehead, J., Krook, A., and O'Rahilly, S. (1997). Molecular mechanisms of inherited insulin resistance. QJM - Mon. J. Assoc. Physicians . 90 (9), 557–562. doi:10.1093/qjmed/90.9.557
Begg, D. P., Puskás, L. G., Kitajka, K., Ménesi, D., Allen, A. M., Li, D., et al. (2012). Hypothalamic gene expression in ω-3 PUFA-deficient male rats before, and following, development of hypertension. Hypertens. Res. 35 (4), 381–387. doi:10.1038/hr.2011.194
Berenjian, A., Sharifi, S. D., Mohammadi-Sangcheshmeh, A., and Ghazanfari, S. (2018). Effect of chromium nanoparticles on physiological stress induced by exogenous dexamethasone in Japanese quails. Biol. Trace Elem. Res. 1-8, 474–481. doi:10.1007/s12011-017-1192-y
Bergles, D. E., Doze, V. A., Madison, D. V., and Smith, S. J. (1996). Excitatory actions of norepinephrine on multiple classes of hippocampal CA1 interneurons. J. Neurosci. 16 (2), 572–585. doi:10.1523/JNEUROSCI.16-02-00572.1996
Bolanos-Garcia, V. M., and Miguel, R. N. (2003). On the structure and function of apolipoproteins: More than a family of lipid-binding proteins. Prog. Biophys. Mol. Biol. 83 (1), 47–68. doi:10.1016/S0079-6107(03)00028-2
Breuner, C. W., and Hahn, T. P. (2003). Integrating stress physiology, environmental change, and behavior in free-living sparrows. Horm. Behav. 43 (1), 115–123. doi:10.1016/S0018-506X(02)00020-X
Cai, Y., Song, Z., Wang, X., Jiao, H., and Lin, H. (2011). Dexamethasone-induced hepatic lipogenesis is insulin dependent in chickens (Gallus gallus domesticus). Stress 14 (3), 273–281. doi:10.3109/10253890.2010.543444
Caro, P., Gómez, J., Sanz, A., Portero-Otín, M., Pamplona, R., and Barja, G. (2007). Effect of graded corticosterone treatment on aging-related markers of oxidative stress in rat liver mitochondria. Biogerontology 8 (1), 1–11. doi:10.1007/s10522-006-9026-x
Clarke, S. D. (2001). Nonalcoholic steatosis and steatohepatitis. I. Molecular mechanism for polyunsaturated fatty acid regulation of gene transcription. Am. J. Physiol. Gastrointest. Liver Physiol. 281 (4), G865–G869. doi:10.1152/ajpgi.2001.281.4.G865
Delezie, E., Swennen, Q., Buyse, J., and Decuypere, E. (2007). The effect of feed withdrawal and crating density in transit on metabolism and meat quality of broilers at slaughter weight. Poult. Sci. 86 (7), 1414–1423. doi:10.1093/ps/86.7.1414
Dobin, A., Davis, C. A., Schlesinger, F., Drenkow, J., Zaleski, C., Jha, S., et al. (2013). Star: Ultrafast universal RNA-seq aligner. Bioinform 29 (1), 15–21. doi:10.1093/bioinformatics/bts635
Duan, Y., Fu, W., Wang, S., Ni, Y., and Zhao, R. (2014). Cholesterol deregulation induced by chronic corticosterone (CORT) stress in pectoralis major of broiler chickens. Comp. Biochem. Physiol. A Mol. Integr. Physiol. 176, 59–64. doi:10.1016/j.cbpa.2014.07.010
Dupont, J., Derouet, M., Simon, J., and Taouis, M. (1999). Corticosterone alters insulin signaling in chicken muscle and liver at different steps. J. Endocrinol. 162 (1), 67–76. doi:10.1677/joe.0.1620067
Everaert, C., Luypaert, M., Maag, J. L., Cheng, Q. X., Dinger, M. E., Hellemans, J., et al. (2017). Benchmarking of RNA-sequencing analysis workflows using whole-transcriptome RT-qPCR expression data. Sci. Rep. 7 (1), 1559–1611. doi:10.1038/s41598-017-01617-3
Ferraz, A. C., Delattre, A. M., Almendra, R. G., Sonagli, M., Borges, C., Araujo, P., et al. (2011). Chronic ω-3 fatty acids supplementation promotes beneficial effects on anxiety, cognitive and depressive-like behaviors in rats subjected to a restraint stress protocol. Behav. Brain Res. 219 (1), 116–122. doi:10.1016/j.bbr.2010.12.028
Fontella, F. U., Vendite, D. A., Tabajara, A. S., Porciúncula, L. O., da Silva Torres, I. L., Jardim, F. M., et al. (2004). Repeated restraint stress alters hippocampal glutamate uptake and release in the rat. Neurochem. Res. 29 (9), 1703–1709. doi:10.1023/b:nere.0000035805.46592.6c
Foucaud, L., Niot, I., Kanda, T., and Besnard, P. (1998). Indirect dexamethasone down-regulation of the liver fatty acid-binding protein expression in rat liver. Biochim Biophys Acta -Lipids Lipid Metabolism 1391 (2), 204–212. doi:10.1016/S0005-2760(97)00213-0
Gray, B., Steyn, F., Davies, P., and Vitetta, L. (2013). Omega-3 fatty acids: A review of the effects on adiponectin and leptin and potential implications for obesity management. Eur. J. Clin. Nutr. 67 (12), 1234–1242. doi:10.1038/ejcn.2013.197
Gross, C., Zhuang, X., Stark, K., Ramboz, S., Oosting, R., Kirby, L., et al. (2002). Serotonin 1A receptor acts during development to establish normal anxiety-like behaviour in the adult. Nature 416 (6879), 396–400. doi:10.1038/416396a
Hennebelle, M., Champeil-Potokar, G., Lavialle, M., Vancassel, S., and Denis, I. (2014). Omega-3 polyunsaturated fatty acids and chronic stress-induced modulations of glutamatergic neurotransmission in the hippocampus. Nutr. Rev. 72 (2), 99–112. doi:10.1111/nure.12088
Houseknecht, K. L., Baile, C. A., Matteri, R. L., and Spurlock, M. E. (1998). The biology of leptin: A review. J. Anim. Sci. 76 (5), 1405–1420. doi:10.2527/1998.7651405x
Hu, X., Wang, Y., Sheikhahmadi, A., Li, X., Buyse, J., Lin, H., et al. (2019). Effects of glucocorticoids on lipid metabolism and AMPK in broiler chickens' liver. Comp. Biochem. Physiol. B Biochem. Mol. Biol.C 232, 23–30. doi:10.1016/j.cbpb.2019.02.001
Kim, D., Langmead, B., and Salzberg, S. L. (2015). Hisat: A fast spliced aligner with low memory requirements. Nat. Methods. 12 (4), 357–360. doi:10.1038/nmeth.3317
Kuleshov, M. V., Jones, M. R., Rouillard, A. D., Fernandez, N. F., Duan, Q., Wang, Z., et al. (2016). Enrichr: A comprehensive gene set enrichment analysis web server 2016 update. Nucleic Acids Res. 44 (W1), W90–W97. doi:10.1093/nar/gkw377
Lan, X., Hsieh, J. C., Schmidt, C. J., Zhu, Q., and Lamont, S. J. (2016). Liver transcriptome response to hyperthermic stress in three distinct chicken lines. BMC Genet. 17 (1), 955. doi:10.1186/s12864-016-3291-0
Latour, A., Grintal, B., Champeil-Potokar, G., Hennebelle, M., Lavialle, M., Dutar, P., et al. (2013). Omega-3 fatty acids deficiency aggravates glutamatergic synapse and astroglial aging in the rat hippocampal CA 1. Aging Cell. 12 (1), 76–84. doi:10.1111/acel.12026
Law, C. W., Chen, Y., Shi, W., and Smyth, G. K. (2014). voom: Precision weights unlock linear model analysis tools for RNA-seq read counts. Genome Biol. 15 (2), R29. doi:10.1186/gb-2014-15-2-r29
Le, P. P., Friedman, J. R., Schug, J., Brestelli, J. E., Parker, J. B., Bochkis, I. M., et al. (2005). Glucocorticoid receptor-dependent gene regulatory networks. PLoS Genet. 1 (2), e16. doi:10.1371/journal.pgen.0010016
Le-Niculescu, H., Case, N., Hulvershorn, L., Patel, S., Bowker, D., Gupta, J., et al. (2011). Convergent functional genomic studies of omega-3 fatty acids in stress reactivity, bipolar disorder and alcoholism. Transl. Psychiatry 1 (4), e4. doi:10.1038/tp.2011.1
Lefevre, P., Diot, C., Legrand, P., and Douaire, M. (1999). Hormonal regulation of stearoyl coenzyme-A desaturase 1 activity and gene expression in primary cultures of chicken hepatocytes. Arch. Biochem. Biophys. 368 (2), 329–337. doi:10.1006/abbi.1999.1315
Lewińska, M., Zelenko, U., Merzel, F., Grdadolnik, S. G., Murray, J. C., and Rozman, D. (2013). Polymorphisms of CYP51A1 from cholesterol synthesis: Associations with birth weight and maternal lipid levels and impact on CYP51 protein structure. PloS one 8 (12), e82554. doi:10.1371/journal.pone.0082554
Li, G., Fu, S., Chen, Y., Jin, W., Zhai, B., Li, Y., et al. (2019). MicroRNA-15a regulates the differentiation of intramuscular preadipocytes by targeting ACAA1, ACOX1 and SCP2 in chickens. Int. J. Mol. Sci. 20 (16), 4063. doi:10.3390/ijms20164063
Li, Y., Xu, S., Mihaylova, M. M., Zheng, B., Hou, X., Jiang, B., et al. (2011). AMPK phosphorylates and inhibits SREBP activity to attenuate hepatic steatosis and atherosclerosis in diet-induced insulin-resistant mice. Cell. Metab. 13 (4), 376–388. doi:10.1016/j.cmet.2011.03.009
Lin, H., Decuypere, E., and Buyse, J. (2004). Oxidative stress induced by corticosterone administration in broiler chickens (Gallus gallus domesticus): 2. Short-Term effect. Comp. Biochem. Physiol. B Biochem. Mol. Biol. 139 (4), 745–751. doi:10.1016/j.cbpc.2004.09.014
Liu, L., Song, Z., Sheikhahmadi, A., Jiao, H., and Lin, H. (2012). Effect of corticosterone on gene expression of feed intake regulatory peptides in laying hens. Comp. Biochem. Physiol. B, Biochem. Mol. Biol. 162 (4), 81–87. doi:10.1016/j.cbpb.2012.04.005
Love, O. P., Breuner, C. W., Vézina, F., and Williams, T. D. (2004). Mediation of a corticosterone-induced reproductive conflict. Horm. Behav. 46 (1), 59–65. doi:10.1016/j.yhbeh.2004.02.001
Maxfield, F. R., and Tabas, I. (2005). Role of cholesterol and lipid organization in disease. Nature 438 (7068), 612–621. doi:10.1038/nature04399
Monfrini, E., Straniero, L., Bonato, S., Compagnoni, G. M., Bordoni, A., Dilena, R., et al. (2019). Neurofascin (NFASC) gene mutation causes autosomal recessive ataxia with demyelinating neuropathy. Park. Relat. Disord. 63, 66–72. doi:10.1016/j.parkreldis.2019.02.045
Ono, T., and Imai, Y. (1985). Squalene epoxidase from rat liver microsomes. Meth. Enzymol. No. 110, 375–380. doi:10.1016/s0076-6879(85)10096-0
Pérez, M. Á., Terreros, G., and Dagnino-Subiabre, A. (2013). Long-term ω-3 fatty acid supplementation induces anti-stress effects and improves learning in rats. Behav. Brain Funct. 9 (1), 25. doi:10.1186/1744-9081-9-25
Post, J., Rebel, J., and Ter Huurne, A. (2003). Physiological effects of elevated plasma corticosterone concentrations in broiler chickens. An alternative means by which to assess the physiological effects of stress. Poult. Sci. 82 (8), 1313–1318. doi:10.1093/ps/82.8.1313
Qi, D., An, D., Kewalramani, G., Qi, Y., Pulinilkunnil, T., Abrahani, A., et al. (2006). Altered cardiac fatty acid composition and utilization following dexamethasone-induced insulin resistance. Am. J. Physiol. Endocrinol. Metab. 291 (2), E420–E427. doi:10.1152/ajpendo.00083.2006
Reynolds, R. M., and Walker, B. R. (2003). Human insulin resistance: The role of glucocorticoids. Diabetes Obes. Metab. 5 (1), 5–12. doi:10.1046/j.1463-1326.2003.00221.x
Richardson, R. D., Boswell, T., Woods, S. C., and Wingfield, J. C. (2000). Intracerebroventricular corticotropin-releasing factor decreases food intake in white-crowned sparrows. Physiol. Behav. 71 (1-2), 213–216. doi:10.1016/s0031-9384(00)00326-7
Robinson, M. D., McCarthy, D. J., and Smyth, G. K. (2010). edgeR: a Bioconductor package for differential expression analysis of digital gene expression data. Bioinform 26 (1), 139–140. doi:10.1093/bioinformatics/btp616
Roldan, M., and Herzig, S. (2015). How do glucocorticoids regulate lipid metabolism? Adv. Exp. Med. Biol. 872, 127–144. doi:10.1007/978-1-4939-2895-8_6
Romero, L. M., Dickens, M. J., and Cyr, N. E. (2009). The reactive scope model—A new model integrating homeostasis, allostasis, and stress. Horm. Behav. 55 (3), 375–389. doi:10.1016/j.yhbeh.2008.12.009
Sakaue, M., and Hoffman, B. B. (1991). Glucocorticoids induce transcription and expression of the alpha 1B adrenergic receptor gene in DTT1 MF-2 smooth muscle cells. J. Clin. Investig. 88 (2), 385–389. doi:10.1172/JCI115315
Shimano, H., Horton, J. D., Hammer, R. E., Shimomura, I., Brown, M. S., and Goldstein, J. L. (1996). Overproduction of cholesterol and fatty acids causes massive liver enlargement in transgenic mice expressing truncated SREBP-1a. J. Clin. Investig. 98 (7), 1575–1584. doi:10.1172/JCI118951
Söllner, T. H. (2003). Regulated exocytosis and SNARE function (Review). Mol. Membr. Biol. 20 (3), 209–220. doi:10.1080/0968768031000104953
Surai, P., and Fisinin, V. (2016). Vitagenes in poultry production: Part 1. Technological and environmental stresses. Worlds Poult. Sci. 72 (4), 721–734. doi:10.1017/s0043933916000714
Takeuchi, T., Iwanaga, M., and Harada, E. (2003). Possible regulatory mechanism of DHA-induced anti-stress reaction in rats. Brain Res. 964 (1), 136–143. doi:10.1016/S0006-8993(02)04113-6
Taylor, A. H., Raymond, J., Dionne, J. M., Romney, J., Chan, J., Lawless, D. E., et al. (1996). Glucocorticoid increases rat apolipoprotein AI promoter activity. J. Lipid Res. 37 (10), 2232–2243. doi:10.1016/s0022-2275(20)37304-1
Thaxton, J., Dozier, W., Branton, S., Morgan, G., Miles, D., Roush, W., et al. (2006). Stocking density and physiological adaptive responses of broilers. Poult. Sci. 85 (5), 819–824. doi:10.1093/ps/85.5.819
Tlemsani, C., Huillard, O., Arrondeau, J., Boudou-Rouquette, P., Cessot, A., Blanchet, B., et al. (2015). Effect of glucuronidation on transport and tissue accumulation of tyrosine kinase inhibitors: Consequences for the clinical management of sorafenib and regorafenib. Expert Opin. Drug Metab. Toxicol. 11 (5), 785–794. doi:10.1517/17425255.2015.1030392
Tsai, L. C. L., and Beavo, J. A. (2011). The roles of cyclic nucleotide phosphodiesterases (PDEs) in steroidogenesis. Curr. Opin. Pharmacol. 11 (6), 670–675. doi:10.1016/j.coph.2011.09.003
Valenzuela, R., Espinosa, A., González-Mañán, D., D'Espessailles, A., Fernández, V., Videla, L. A., et al. (2012). N-3 long-chain polyunsaturated fatty acid supplementation significantly reduces liver oxidative stress in high fat induced steatosis. PloS one 7 (10), e46400. doi:10.1371/journal.pone.0046400
Wang, C. C., and Wang, S. J. (2009). Modulation of presynaptic glucocorticoid receptors on glutamate release from rat hippocampal nerve terminals. Synapse 63 (9), 745–751. doi:10.1002/syn.20654
Wang, X. J., Li, Y., Song, Q. Q., Guo, Y. Y., Jiao, H. C., Song, Z. G., et al. (2013). Corticosterone regulation of ovarian follicular development is dependent on the energy status of laying hens. J. Lipid Res. 54 (7), 1860–1876. doi:10.1194/jlr.M036301
Winder, W. a., and Hardie, D. (1999). AMP-Activated protein kinase, a metabolic master switch: Possible roles in type 2 diabetes. Am. J. Physiol. Endocrinol. Metab. 277 (1), E1–E10. doi:10.1152/ajpendo.1999.277.1.E1
Xie, J., Tang, L., Lu, L., Zhang, L., Lin, X., Liu, H. C., et al. (2015). Effects of acute and chronic heat stress on plasma metabolites, hormones and oxidant status in restrictedly fed broiler breeders. Poult. Sci. 94 (7), 1635–1644. doi:10.3382/ps/pev105
Xu, C., He, J., Jiang, H., Zu, L., Zhai, W., Pu, S., et al. (2009). Direct effect of glucocorticoids on lipolysis in adipocytes. Mol. Endocrinol. 23 (8), 1161–1170. doi:10.1210/me.2008-0464
Yoshimura, R., Araki, E., Ura, S., Todaka, M., Tsuruzoe, K., Noboru, F., et al. (1997). Impact of natural IRS-1 mutations on insulin signals: Mutations of IRS-1 in the PTB domain and near SH2 protein binding sites result in impaired function at different steps of IRS-1 signaling. Diabetes 46 (6), 929–936. doi:10.2337/diab.46.6.929
Yuan, L., Lin, H., Jiang, K., Jiao, H., and Song, Z. (2008). Corticosterone administration and high-energy feed results in enhanced fat accumulation and insulin resistance in broiler chickens. Br. Poult. Sci. 49 (4), 487–495. doi:10.1080/00071660802251731
Zammit, V. A. (2008). Carnitine palmitoyltransferase 1: Central to cell function. IUBMB life 60 (5), 347–354. doi:10.1002/iub.78
Zeng, J., Deng, S., Wang, Y., Li, P., Tang, L., and Pang, Y. (2017). Specific inhibition of acyl-CoA oxidase-1 by an acetylenic acid improves hepatic lipid and reactive oxygen species (ROS) metabolism in rats fed a high fat diet. J. Biol. Chem. 292 (9), 3800–3809. doi:10.1074/jbc.M116.763532
Keywords: RNA-Seq, nutrigenomics, gene expression, lipid metabolism, stress
Citation: Berenjian A, Bakhtiarizadeh MR, Mohammadi-Sangcheshmeh A and Sharifi SD (2023) A nutrigenomics approach to study the effects of ω-3 fatty acids in laying hens under physiological stress. Front. Physiol. 14:1198247. doi: 10.3389/fphys.2023.1198247
Received: 05 April 2023; Accepted: 11 July 2023;
Published: 25 July 2023.
Edited by:
Gale Strasburg, Michigan State University, United StatesReviewed by:
Yanfa Sun, Longyan University, ChinaJiahui Xu, University of California, Irvine, United States
Copyright © 2023 Berenjian, Bakhtiarizadeh, Mohammadi-Sangcheshmeh and Sharifi. This is an open-access article distributed under the terms of the Creative Commons Attribution License (CC BY). The use, distribution or reproduction in other forums is permitted, provided the original author(s) and the copyright owner(s) are credited and that the original publication in this journal is cited, in accordance with accepted academic practice. No use, distribution or reproduction is permitted which does not comply with these terms.
*Correspondence: Seyed Davood Sharifi, c2RzaGFyaWZpQHV0LmFjLmly