- 1National Key Laboratory for Conservation and Utilization of Biological Resources in Yunnan, College of Plant Protection, Yunnan Agricultural University, Kunming, China
- 2Yunnan Yuntianhua Co., Ltd., Kunming, Yunnan, China
- 3College of Resources and Environment, Yunnan Agricultural University, Kunming, China
- 4Yunnan Urban Agricultural Engineering and Technological Research Center, College of Agronomy and Life Sciences, Kunming University, Kunming, China
Encarsia formosa is a natural enemy of the invasive pest Bemisia tabaci and is known to be a dominant parasitic. The frequency and magnitude of climate extremes, particularly temperature extremes, have increased, which has put insect populations at risk. However, the effects of temperature extremes on E. formosa are not well understood. To examine the impact of short-term extreme temperature exposure on the development and reproduction of E. formosa, eggs, larvae, pupae, and adults were exposed to high/low temperature treatments (HLT25, HLT50, LLT25, and LLT50). Our findings indicate that the pupal stage of E. formosa exhibited the strongest tolerance to both heat and cold, while adults exhibited a weaker tolerance. The shortest egg-to-adult development period of 12.65 days was observed in E. formosa exposed to HLT50 treatment during the egg-larval stage. The parasitism peak of the adult stage was delayed by 1–6 days after exposure to extreme temperatures during the egg-larval stage. Conversely, the parasitism peak was advanced by 1–3 days after exposure to extreme temperatures during the pupal and adult stages. The eclosion rate, total parasitism, eclosion rate of the F1 generation, and adult longevity of the F1 generation were lower in the treatment groups than in the control groups. The F1 generation’s development period was prolonged to 15.49 and 15.19 days after exposure to HLT25 and HLT50 treatments, respectively, during the egg-larval stage. The F1 generation’s development period was shortened to 13.33 days after exposure to LLT50 treatment during the pupal stage. Male individuals appeared in the F1 generation after exposure to HLT50 treatment during the pupal stage, with females accounting for only 56.38%. Our results demonstrate that short-term exposure to extreme temperatures has detrimental effects on the growth and reproduction of E. formosa. In field biocontrol against E. formosa, the release of E. formosa should be avoided as much as possible when the ambient temperature is higher than 35°C or lower than 0°C. During extreme temperature conditions, timely supplementation and release of E. formosa population, along with ventilation and cooling in greenhouse facilities during summer, are necessary for better pest control efficacy.
Highlights
1) E. formosa exhibits varied tolerance to temperature extremes depending on its developmental stage. The pupal stage of E. formosa demonstrated a high degree of tolerance to both heat and cold, whereas the adult stage displayed weaker tolerance. 2) Exposure to short-term temperature extremes had detrimental effects on the eclosion rate, total parasitism, eclosion rate of the F1 generation, and adult longevity of the F1 generation in E. formosa. 3) Notably, the emergence of male individuals in the F1 generation was observed only after exposing the pupal stage of E. formosa to HLT50 treatment.
1 Introduction
Bemisia tabaci (Gennadius) (Hemiptera: Aleyrodidae) is a highly polyphagous insect pest characterized by small individuals, diverse biotypes, and a global distribution excluding Antarctica (Kanakala and Ghanim, 2019; Pan et al., 2021; Isaac et al., 2023). This pest’s host plant range is vast, as it can damage over 900 host plant species, including Gossypium spp. (Malvales: Malvaceae), Solanum lycopersicum Miller (Tubiflorae: Solanaceae), and Phaseolus vulgaris L. (Rosales: Fabaceae) (Lee, 2020). Bemisia tabaci inflicts direct harm on crops by extracting plant sap and excreting honeydew, which leads to sooty mold and significantly impairs plant photosynthesis. Indirect damage also occurs through the transmission of various plant viruses (Li et al., 2022). The economic losses from indirect damage considerably exceed those caused by direct feeding (Zhang X M et al., 2020). At present, chemical control is the primary method for managing B. tabaci. However, the extended use of chemical pesticides heightens the likelihood of resistance development in B. tabaci populations (Basit, 2019). Consequently, the implementation of biological control as part of an integrated management strategy for B. tabaci is essential (Lenteren et al., 2018; Roda et al., 2020). Encarsia formosa Gahan (Hymenoptera: Aphelinidae) is a crucial parasitic of whitefly pests and one of the predominant parasitic species of B. tabaci, which may effectively control the population growth of B. tabaci (He et al., 2019; Deeksha et al., 2023). E. formosa undergoes complete metamorphosis, with the larval and pupal stages developing inside the body of B. tabaci (Wang et al., 2019). The adult E. formosa have reddish-brown ocelli and rod-shaped antennae, with males having yellow heads and black abdomens, distinguishing them from females (Li, 2017). E. formosa is a parthenogenetic parasitic wasp that tends to parasitize the 3rd-instar and 4th-instar nymphs of whitefly, with parasitized 3rd-instar nymphs of B. tabaci turning black after about a week (Dai et al., 2014; Yang et al., 2023). Commercial production of E. formosa has been established, and it has demonstrated considerable effectiveness in controlling whitefly pests in Hebei, Henan, Liaoning, Shanxi, and Beijing, China (Liu, 2017).
As ectothermic animals, insects are particularly susceptible to external factors such as temperature (Daniel et al., 2020). Temperature stress outside the optimal range may cause changes in insect metabolism, material composition, and hormone levels, negatively impacting their development, reproduction, and survival (Bennett and Moran, 2015). Temperature effects on insects can be classified into two types: long-term and short-term high or low temperature stress, which have distinct effects (Wang et al., 2010). The increasing occurrence of extreme temperature conditions due to global warming poses a significant threat to the survival and reproduction of insects, even for brief periods (Meehl and Tebaldi, 2004). Different developmental stages of insects have different levels of temperature tolerance. For example, in Propylaea japonica (Thunberg) (Coleoptera: Coccinellidae), the pupal stage exhibits the highest tolerance to high temperatures, followed by older and younger larvae stages (Pumhan et al., 2020). Cold resistance is the strongest in B. tabaci pupae, followed by eggs, nymphs, and adults, with increased mortality as low-temperature stress increases (Yang et al., 2021). Temperature also affects the parasitism of insects, a critical reference index for the control potential of natural pest enemies. High-temperature stress significantly reduces the fecundity of Trichogramma dendrolimi Matsumura (Hymenoptera: Trichogrammatidae) females and inhibits their parasitism (Liu et al., 2021), whereas low-temperature storage of adult Diadegma semiclausum (Hellén) (Hymenoptera: Ichneumonidae) reduces parasitism and emergence rates (Zhang et al., 2022). Short-term extremely high temperatures may lead to reduced individual fitness of parasitic wasps, causing delayed development, shorter lifespan, reduced fecundity, and decreased female ratio (Klockmann et al., 2017a; Klockmann et al., 2017b). The growth, development, and fecundity of Aphidius gifuensis Ashmead (Hymenoptera: Aphidiidae) are seriously impacted by decreasing temperatures (Izumi and Makoto, 2006). E. formosa grows optimally within the 15°C–30°C temperature range, and deviation from this range may negatively affect their development and reproduction, ultimately impacting their population growth (Chown, 2006; Yin et al., 2018). However, the effects of extreme temperature on different developmental stages of E. formosa remain poorly understood.
Employing E. formosa to manage B. tabaci populations has become a focal point of contemporary research. Factors such as high summer temperatures, elevated greenhouse temperatures, extremely low winter temperatures, and low-temperature storage technology during commercial production may potentially affect the survival and reproduction of E. formosa, thereby altering its efficacy in controlling B. tabaci. In this study, we investigated the impacts of short-term exposure to various extreme temperatures on the growth, development, reproductive capacity, and pest control ability of E. formosa across its different developmental stages. The insights gained from this research are anticipated to inform the strategic release of E. formosa in future agricultural practices and provide a valuable reference for preserving and predicting E. formosa’s control efficiency in extreme temperature environments.
2 Materials and methods
2.1 Insects, host plant, and temperature measurements
The study utilized E. formosa insects collected from P. vulgaris plants (102°40′E, 25°11′N) in the northern suburb of Kunming City, Yunnan Province, China. The MED cryptic species of B. tabaci was kindly provided by the Biotechnology and Germplasm Resources Institute, Yunnan Academy of Agricultural Sciences, and reared separately in insect cages (60 cm long × 60 cm wide × 60 cm deep) with P. vulgaris as the host plant for at least 20 generations in an insect-rearing room maintained at a temperature of 25°C ± 1°C and a humidity level of 75% ± 5%. To ensure the purity of the insect populations and minimize external factors, the tested B. tabaci and E. formosa populations were reared separately in different insect cages in a greenhouse without the use of insecticides for over 20 generations. When we want to use it, we take the appropriate age of insects to test. For the experiment, a single P. vulgaris plant was grown in a flowerpot (10 cm in diameter, 12 cm in height) inside an insect cage maintained at a temperature of 25°C ± 1°C and a humidity level of 75% ± 5% in the laboratory. The amount of P. vulgaris planted should ensure that the experiment is carried out properly. After the expansion of the first pair of primary leaves in the seedlings, fours pots of seedlings were used per cage, with 200 individuals of B. tabaci (male to female ratio of about 1:1) inoculated for oviposition on each cage. After 24 h, the bean seedlings were removed and placed inside an insect cage without the adults of B. tabaci for use in experiments after the B. tabaci nymphs on the back of the leaves reached the 3rd-instar stage.
For the short-term high-temperature stress treatment, the E. formosa insects were subjected to temperatures of 30°C, 34°C, 38°C, 42°C, 46°C, and 50°C. The short-term low-temperature stress treatments were conducted at temperatures of −12°C, −8°C, −4°C, 0°C, 4°C, and 8°C, and temperatures below 0 °C were achieved using a BCD-201STPA refrigerator, while the remaining temperatures were maintained inside a BIC-300 artificial climate chamber. The control-group temperature was 25°C. Each temperature treatment had three replicates, and each stress treatment lasted for 6 h. After exposure to stress, the E. formosa insects were immediately transferred to an artificial climate chamber maintained at a temperature of 25°C ± 1°C, a photoperiod of 14:10 (L:D) h, and a humidity level of 75% ± 5% for feeding.
The experimental equipment consisted of a BIC-300 artificial climate chamber (Shanghai Boxun Medical Equipment Factory), a BCD-201STPA refrigerator (Haier Group), and an OLYMPUS SZ51 stereomicroscope (Olympus, Japan).
The insect-rearing containers utilized in the experiments were custom-made. Cylindrical transparent plastic pots, measuring 9 cm in diameter and 5 cm in height, were procured from Hangzhou Wangyi E-commerce Co. Ltd. The detachable lid of the plastic container connects to the main body through a spiral jaw mechanism. The center of the lid was removed and replaced with a 120-mesh screen, which was affixed using hot melt adhesive to enable proper ventilation. These custom-made insect-rearing containers were employed for housing E. formosa at various developmental stages during treatment, and for the daily replacement of host plant leaves hosting 50 individual 3rd-instar stage nymphs of B. tabaci.
2.2 Determination of the mortality of Encarsia formosa at different developmental stages after short-term temperature treatment
Treatment of the different developmental stages of E. formosa:
Adult: E. formosa adults within 24 h of emergence;
Pupa: 3 days after the pupation of E. formosa;
Egg–larvae: The 3rd day after the 3rd-instar stage nymphs of B. tabaci were parasitized by E. formosa.
Thirty E. formosa specimens at various developmental phases (egg-larvae, pupa, adult) were placed in artificial climate chambers or refrigerators, depending on the required treatment, at distinct high/low-temperature stress treatments or 25°C (control), and subsequently transferred to artificial climate chambers. Each treatment had a duration of 6 h. To preserve leaf freshness, water was added to the petiole. Leaves were examined every 24 h using a stereoscope (OuYang et al., 2014). The number of deceased E. formosa adults after 6 h exposure to varying temperatures was documented. For the treatment involving E. formosa adults, they were gently touched with a fine brush, and unresponsive individuals were considered dead. The number of emerged and dead E. formosa pupae after exposure to different temperatures was recorded. Dead E. formosa pupae were identified by their loss of water and shriveled appearance. The number of E. formosa pupae, emerged B. tabaci adults, and dead B. tabaci nymphs after egg-larvae exposure to various temperatures was also noted. For the treatment on E. formosa egg-larvae, B. tabaci nymphs displaying water loss and withering, as well as the E. formosa egg-larvae, were deemed dead (Jiang et al., 2018). Each temperature treatment experiment was conducted three times. The corrected mortality rate of E. formosa at different developmental stages was calculated after 6 h of exposure to various temperatures. The findings revealed that extreme-high temperatures (HLT25 and HLT50) and extreme-low temperatures (LLT25 and LLT50) resulted in mortality rates of 25% and 50%, respectively (Zhou et al., 2011).
2.3 Effects of short-term exposure to extreme temperature on the growth and development of Encarsia formosa
Short-term extreme temperature treatment of adults:
In this study, 30 adult individuals of E. formosa were chosen within 24 h of emergence and exposed to extreme temperatures of HLT25, HLT50, LLT25, and LLT50 treatments for 6 h as outlined in Section 2.2. Subsequently, the insects were placed in an artificial climate chamber with ambient temperature for 30 min before being selected randomly and placed in a self-constructed insect-rearing jar and fed. The leaves of kidney bean plant with 50 individual 3rd-instar stage nymphs of B. tabaci were changed every 24 h. Survival days of E. formosa were recorded under different treatments, while the daily parasitism of B. tabaci nymphs was observed, and the number of parasitized nymphs was recorded. Each experiment was replicated three times.
Short-term extreme temperature treatment of the egg–larvae and pupae:
Additionally, 30 egg–larvae and 30 pupae of E. formosa were also exposed to extreme temperatures of HLT25, HLT50, LLT25, and LLT50 treatments for 6 h, as specified in Section 2.2. After treatment, the pupae were transferred to a self-constructed insect-rearing jar placed in an artificial climate chamber with ambient temperature for further development. The petiole was kept moist with water, and observations were made every 24 h. The eclosion date and the eclosion number of the emerged E. formosa were also recorded. Each experiment was repeated three times. A total of 30 adults within 24 h of emergence from each group were randomly selected and reared in the self-constructed insect-rearing jar in each group. The leaves of the kidney bean plant with 50 individual 3rd-instar stage nymphs of B. tabaci were replaced every 24 h. The survival days of E. formosa under the different treatments were recorded, the daily parasitism of the B. tabaci nymphs was observed, and the number of parasitized nymphs was recorded (Wang et al., 2018).
2.4 Effects of the short-term extreme temperature treatment on the growth and development of the F1 generation of Encarsia formosa
The leaves of B. tabaci nymphs were parasitized by E. formosa adults, and ten of these leaves were randomly selected for the respective temperature treatments outlined in Section 2.3. The emergence date and number of pupae that emerged were recorded every 24 h for the F1 generation of E. formosa. After pupation, each leaf was placed separately in a self-constructed insect-rearing jar, and the number of males and females and the emergence date of the F1 generation of E. formosa adults were recorded every 24 h. A total of 30 F1 generation E. formosa adults were randomly selected within 24 h of emergence and reared in the self-constructed insect-rearing jar. Leaves with 50 3rd-instar nymphs of B. tabaci were replaced every 24 h until the F1 generation E. formosa adults died, and the time and number of deaths were recorded (Wang et al., 2019).
2.5 Data analyses
The LT25 and LT50 values of E. formosa at various developmental stages, under short-term exposure to extreme temperature stress, along with associated experimental data on growth and development, were analyzed using one-way analysis of variance (one-way ANOVA) in SPSS 22.0. Tukey’s multiple-comparison test was utilized to identify significant differences in the relevant parameters of E. formosa at different developmental stages, following short-term exposure to different extreme temperatures. Survival rates were compared using the Kaplan-Meier procedure. Graphs representing the number of parasitism and survival rate were generated using Origin 2018.
3 Results
3.1 Corrected mortality of Encarsia formosa under short-term temperature stress
When subjected to short-term high-temperature stress for 6 h, E. formosa exhibited increasing corrected mortality at each developmental stage (egg-larvae, pupae, and adults) as the temperature increased, with no survival observed at 50°C. The corrected mortality of egg-larvae exceeded 40% when exposed to temperatures above 38°C, while adults could not survive at temperatures of 46 °C and above. In contrast, pupae demonstrated a strong ability to withstand high temperatures, surviving under short-term temperature stress ranging from 30°C to 46°C (egg-larvae: F5,12 = 241.67, p = 0.0001; pupae: F5,12 = 1180.90, p = 0.0001; adults: F5,12 = 289.93, p = 0.0001) (Table 1).
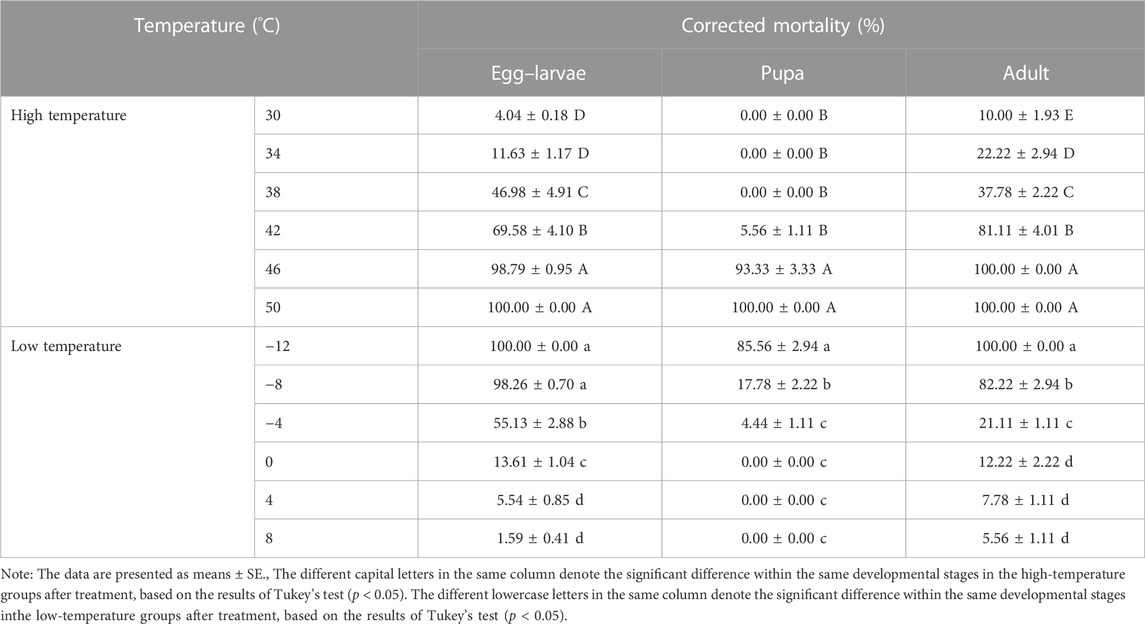
TABLE 1. Corrected mortality of the different developmental stages of Encarsia formosa (host: Bemisia tabaci) under short-term extreme temperature stress.
Conversely, when subjected to short-term low-temperature stress for 6 h, E. formosa exhibited increasing corrected mortality at each developmental stage as the temperature decreased. The corrected mortality of egg-larvae and adults exceeded 10% when exposed to temperatures at or below 0°C, with no survival recorded at temperatures below −12°C. Pupae exhibited higher tolerance to low temperatures than the other developmental stages, with survival recorded at temperatures ranging from −12°C to 8°C (egg-larvae: F5,12 = 1159.3, p = 0.0001; pupae: F5,12 = 463.28, p = 0.0001; adults: F5,12 = 605.09, p = 0.0001) (Table 1).
The HLT25 and HLT50 of the pupae were the highest at 42.87°C and 44.55°C, respectively, followed by the HLT25 and HLT50 of the egg–larvae at 35.77°C and 38.73°C, respectively. The HLT25 and HLT50 of the adults were the lowest at 33.96°C and 37.67°C, respectively. The LLT25 and LLT50 of the pupae were the lowest at −8.15°C and −9.78°C, respectively, followed by the LLT25 and LLT50 of the adults at −1.43°C and −4.78°C, respectively. The LLT25 and LLT50 of the egg–larvae were the highest at −0.45°C and −2.87°C, respectively (Table 2).
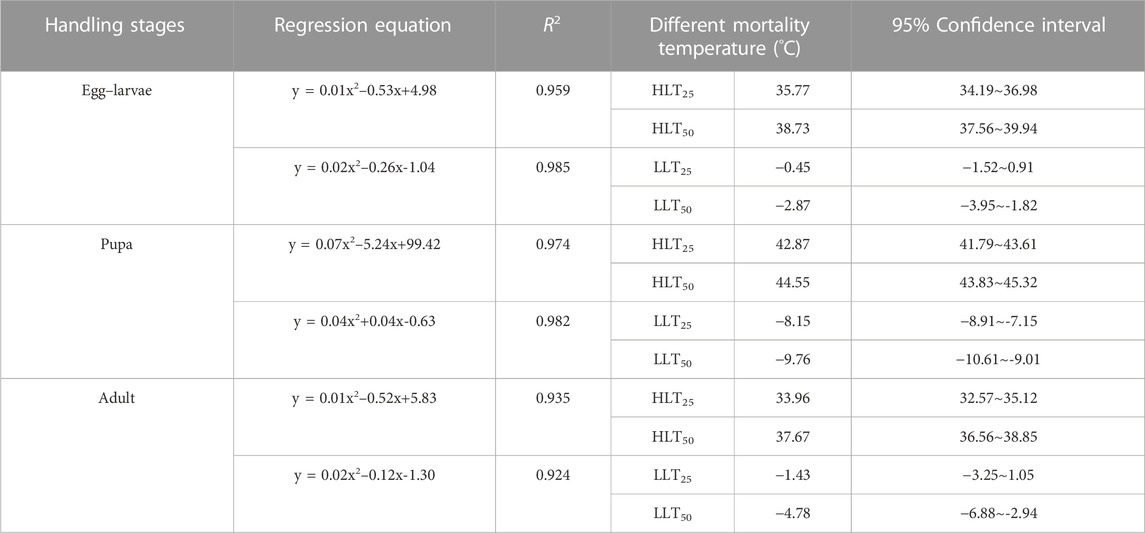
TABLE 2. HLT25, HLT50, LLT25, and LLT50 of the different developmental stages of Encarsia formosa (host: Bemisia tabaci) under short-term extreme temperature stress.
3.2 Lengths of the period of development of Encarsia formosa under short-term extreme temperature stress
Compared to other temperature treatments, the shortest duration for pupa-adult and egg–adult development (5.70 days and 12.65 days, respectively) was observed when egg–larvae of E. formosa were exposed to short-term treatment of HLT25. The duration of pupa-adult and egg-adult development increased when pupae were exposed to short-term treatment of HLT25 and HLT50, compared to the control (pupa–adult development at high temperature: F4,45 = 44.29, p = 0.0001; egg–adult development at high temperature: F4,45 = 33.07, p = 0.0001). Similarly, when egg-larvae and pupae were exposed to short-term treatments of LLT25 and LLT50, the duration of pupa–adult and egg-adult development was longer than that observed in the control (pupa-adult development at low temperature: F4,45 = 23.56, p = 0.0001; egg–adult development at low temperature: F4,45 = 16.39, p = 0.0001). The longest pupa–adult duration (8.24 days) was observed when egg-larvae were exposed to short-term treatment of LLT50, and the longest egg-adult duration (15.32 days) was observed when pupae were exposed to short-term treatment of LLT50 (Table 3).
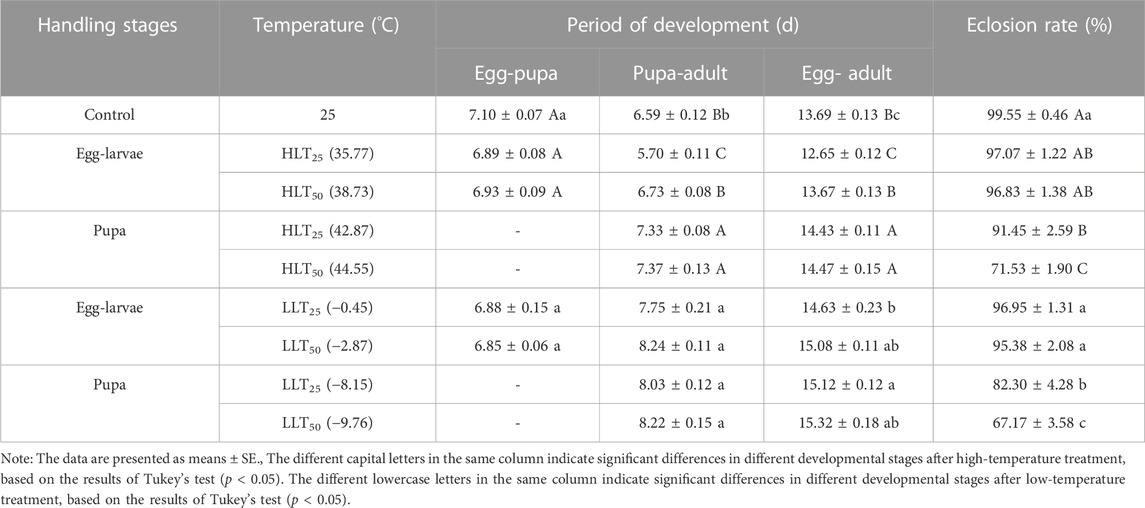
TABLE 3. The period of development of each developmental stage of Encarsia formosa (host: Bemisia tabaci) under short-term extreme temperature stress.
The eclosion rates of egg–larvae and pupae were lower after short-term exposure to extreme temperatures than the control. The eclosion rates decreased with an increase in extreme-high temperature and a decrease in extreme-low temperature. The eclosion rates for egg–larvae exposed to short-term treatments of HLT25, HLT50, LLT25, and LLT50 were 97.07%, 96.83%, 96.95%, and 95.38%, respectively, which were not significantly different from the control. However, the eclosion rates of pupae exposed to HLT25 and HLT50 treatments were 91.45% and 71.53%, respectively, which were significantly lower than the control (F4,45 = 44.93, p = 0.0001). The eclosion rates of pupae exposed to LLT25 and LLT50 treatments were 82.30% and 67.17%, respectively, which were significantly lower than the control (F4,45 = 24.52, p = 0.0001) (Table 3).
3.3 Adult longevity and parasitism of Encarsia formosa under short-term extreme temperature stress
The study employed Kaplan-Meier survival analysis to compare the survival rates of E. formosa at different developmental stages after short-term extreme temperature treatments. The results indicated that E. formosa adults experienced increased mortality between the 12th and 20th day (Figure 1). After exposure to HLT50, the adult longevity of E. formosa was significantly reduced to 13.07 days for egg-larvae and 13.27 days for pupae, compared to the control group (F6,203 = 3.29, p = 0.0042). However, the adult longevity was prolonged to 18.47 days for egg-larvae under the short-term treatment of LLT25 but shortened to 16.03 days under LLT50. The adult longevity was also reduced after exposure of pupae and adults to LLT25 and LLT50 treatments, with the shortest adult longevity (15.07 days) occurring after the adults were subjected to LLT50 (Table 4).
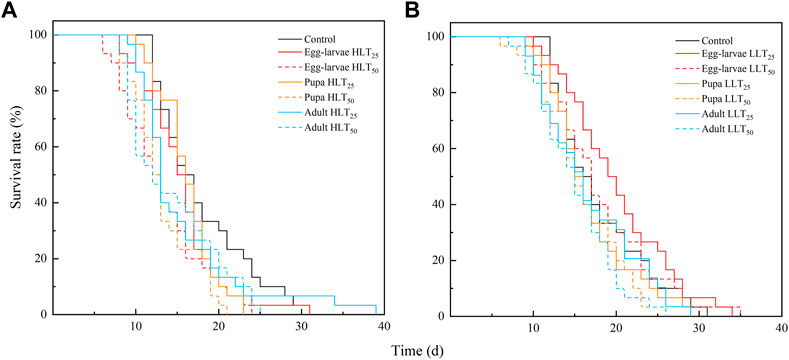
FIGURE 1. Survival rate of adult of Encarsia formosa (host: Bemisia tabaci) under short-term extreme temperature stress at each developmental stage. Note: Fig. (A) presents the survival rate of adult of Encarsia formosa under short-term high-temperature stress at each developmental stage. Fig. (B) presents the survival rate of adult of E. formosa under short-term low-temperature stress at each developmental stage.
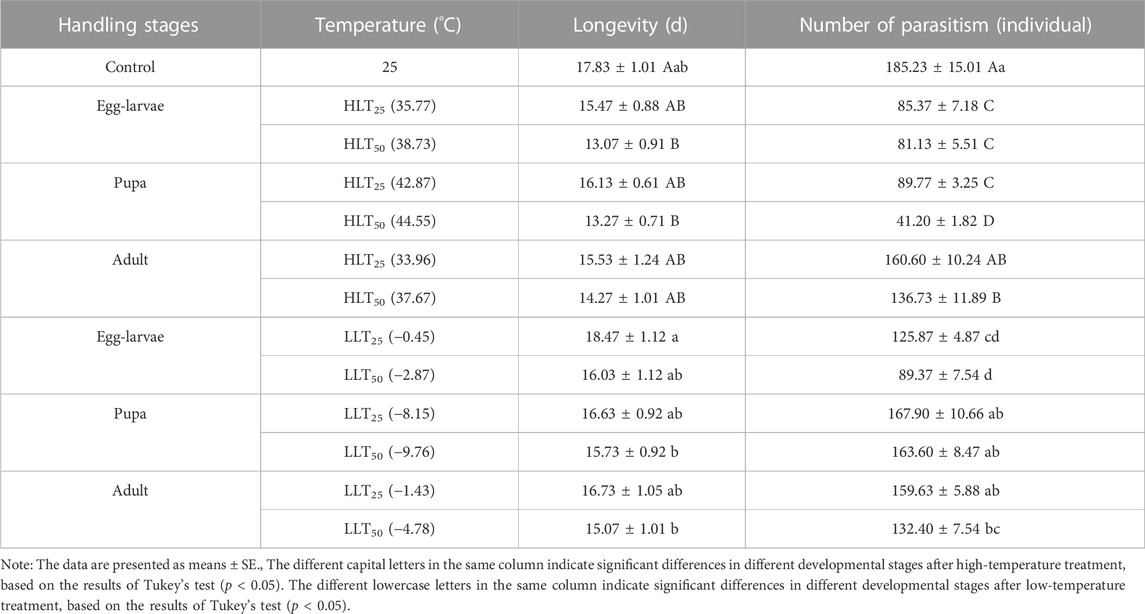
TABLE 4. Effects of short-term extreme temperature stress on the parasitic ability of Encarsia formosa (host: Bemisia tabaci).
Furthermore, the total amount of parasitism decreased for all developmental stages of E. formosa under short-term extreme temperature stress. Egg–larvae were affected the most, followed by pupae and adults. Compared to the control group, the total number of adults with parasitism decreased significantly after egg–larvae were subjected to HLT25 and HLT50 treatments (85.37 individuals and 81.13 individuals, respectively), while pupae exposed to HLT25 and HLT50 treatments yielded 89.77 individuals and 41.20 individuals, respectively. Table 4 shows that the number of adults with parasitism was significantly lower in the HLT50 treatment group compared to the control group (136.73 individuals) (F6,203 = 31.88, p = 0.0001). Additionally, the number of adults with parasitism was lower in the LLT25 treatment group (125.87 individuals) and the LLT50 treatment group (89.37 individuals) compared to the control group. The number of adults with parasitism was also lower in the LLT50 treatment group (132.40 individuals) compared to the control group. These results indicate that the short-term exposure to extreme temperatures had a negative effect on parasitism. However, there was no significant difference in the total amount of parasitism after other treatments compared to the control group (F6,203 = 12.64, p = 0.0001) (Table 4).
The parasitism peaks were more concentrated and prominent in the short-term temperature stress groups of HLT25, HLT50, LLT25, and LLT50. The peaks were followed by a rapid decline in all groups. The parasitic peak of the control group was 14.83 individuals on the 4th day after emergence, followed by a relatively gentle downward trend. Exposure of pupae and adults to short-term extreme temperature stress resulted in earlier parasitism peaks. The parasitic peaks of pupae under HLT25, HLT50, LLT25, and LLT50 treatments, which were 12.27 individuals, 6.37 individuals, 16.23 individuals, and 14.27 individuals, respectively, occurred on the 3rd, 1st, 2nd, and 3rd day after emergence. Similarly, the parasitic peaks of adults after HLT25, HLT50, LLT25, and LLT50 treatments, which were 17.97 individuals, 15.03 individuals, 17.17 individuals, and 15.37 individuals, respectively, occurred on the 1st, 3rd, 2nd, and 2nd day after emergence. Finally, the parasitic peaks of egg–larvae after HLT25, HLT50, LLT25, and LLT50 treatments, which were 12.22 individuals, 10.27 individuals, 10.57 individuals, and 12.33 individuals, respectively, were delayed until the 10th, 7th, 5th, and 8th day after emergence, respectively (Figure 2).
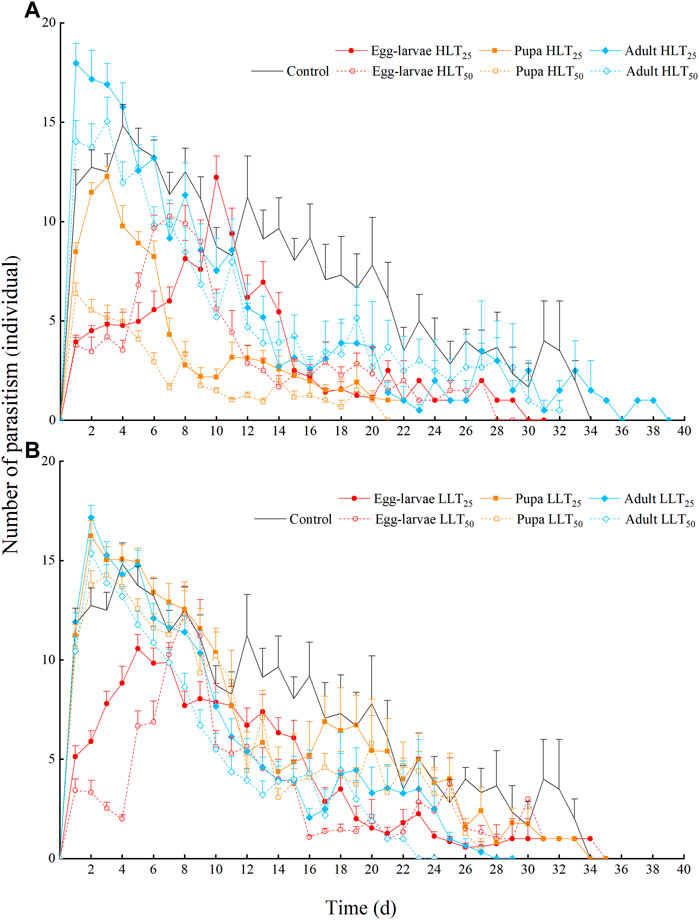
FIGURE 2. The daily number of adults with parasitism for Encarsia formosa (host: Bemisia tabaci) under short-term extreme temperature stress at each developmental stage. Note: The data are presented as means + SE. Fig. (A) presents the daily number of adults with parasitism for Encarsia formosa under short-term high-temperature stress at each developmental stage. Fig. (B) presents the daily number of adults with parasitism for Encarsia formosa under short-term low-temperature stress at each developmental stage.
3.4 Period of development and adult longevity of the F1 generation of Encarsia formosa under short-term extreme temperature stress
The development time of the F1 generation of E. formosa was prolonged after egg-larvae of E. formosa were subjected to short-term extreme high-temperature stress treatment. The development time of the F1 generation of E. formosa was shortened after the pupae were subjected to a short-term extreme high temperature stress treatment. In addition, the development time of the F1 generation of E. formosa at the same developmental stage under extremely high-temperature stress shortened with the increase of stress temperature. After egg–larvae were subjected to the short-term treatments with HLT25 and HLT50, the egg–pupa development time of the F1 generation was longer than that of the control, with values of 7.90 days and 7.80 days, respectively. The pupa–adult development time was the shortest (5.78 days) for the F1 generation after the pupa was subjected to the short-term treatment with HLT25. After the egg–larvae were subjected to the short-term treatment with LLT25 and the pupa was subjected to the short-term treatment with LLT50, the developmental duration of the F1 generation between egg and adult was 15.49 days and 11.98 days, respectively. These values were significantly different from the value of 13.69 days observed for the control (developmental time of egg–adult of the F1 generation: F6,63 = 13.53, p = 0.0001) (Table 5).
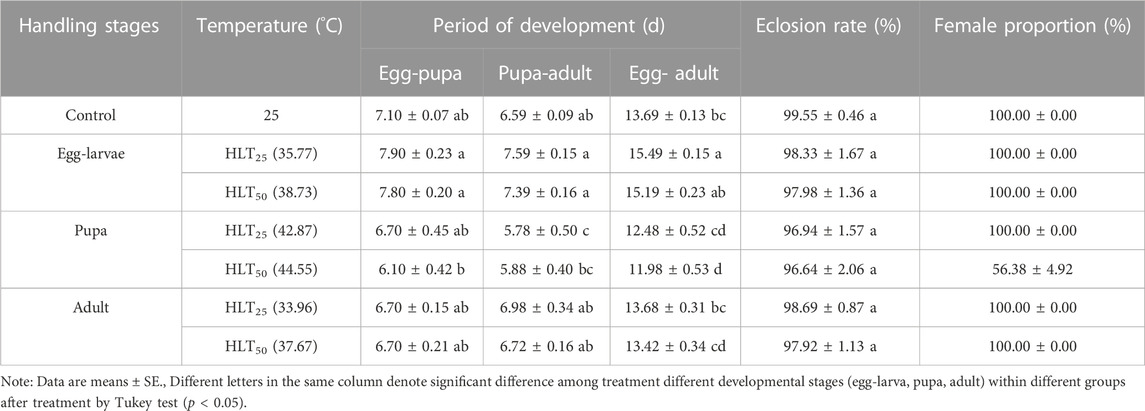
TABLE 5. The period of development of the F1 generation of Encarsia formosa (host: Bemisia tabaci) under short-term high-temperature stress at each developmental stage.
The developmental time of the F1 generation was prolonged after egg–larvae and adults of E. formosa were briefly exposed to extreme low-temperature stress. At the same developmental stage, the F1 developmental duration of E. formosa under extremely low temperature stress was shortened with the decrease in stress temperature. The egg–pupa development time of the F1 generation was shorter than that of the control after the pupa was exposed to the short-term treatments of LLT25 and LLT50, with the values of 6.90 days and 6.70 days observed for the former generation, respectively. The development time of pupa–adult and egg–adult of F1 generation was 7.74 days and 15.74 days, respectively, and these values were significantly higher than that of the control after the egg–larvae were subjected to the short-term treatment of LLT25 (development time of pupa-adult of F1 generation: F6,63 = 4.29, p = 0.0011; development time egg-adult of F1 generation: F6,63 = 8.95, p = 0.0001) (Table 6).
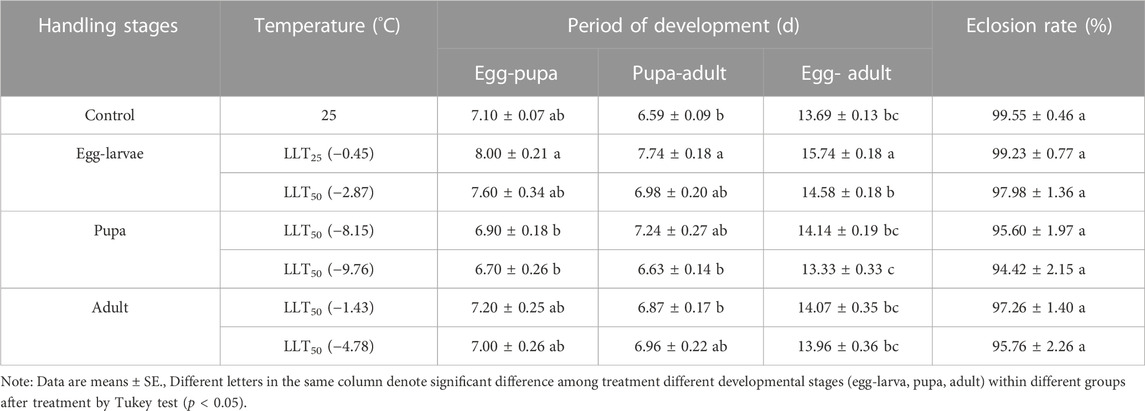
TABLE 6. The period of development of the F1 generation of Encarsia formosa (host: Bemisia tabaci) under short-term low-temperature stress at each developmental stage.
The eclosion rates of the F1 generation were lower at different developmental stages of E. formosa under short-term extreme temperature stress compared to the control. However, there was no significant difference among the treatments (short-term extreme high-temperature stress: F6,63 = 0.57, p = 0.7565; short-term extreme low-temperature stress: F6,63 = 1.44, p = 0.2125). The emergence rate of the F1 generation after pupae were subjected to short-term extreme temperature stress was the lowest and did not exceed 97%. In the F1 generation of E. formosa, male adults appeared after pupae were exposed to the short-term treatment of HLT50, while the proportion of females was just 56.38%. In contrast, male individuals did not appear in the other short-term temperature treatments (Table 5; 6).
After short-term extreme temperature stress at different developmental stages of E. formosa, the death of F1 generation adults of E. formosa occurred mainly on the 12th–20th day, and the survival rate showed a significant downward trend (Figure 3). The adult longevity of the F1 generation was 13.07 days and 13.27 days after egg–larvae were subjected to the short-term treatments of HLT25 and HLT50. The female adult longevity of the F1 generation was 12.87 days and 11.13 days, respectively, after the pupae were subjected to the short-term treatments of HLT25 and HLT50. The male adult longevity of the F1 generation was 10.77 days after the pupae were subjected to the short-term treatment of HLT50. Additionally, the adult longevity of the F1 generation was 13.87 days after the adults were subjected to the short-term treatment of HLT50, and this value was significantly shorter than the corresponding value for the control (F7,232 = 6.92, p = 0.0001). The adult longevity of the F1 generation was not significantly different from that of the control after exposure of egg–larvae to the LLT25 and LLT50 treatments and also after exposure of adults to the LLT25 treatment. Conversely, the adult longevity of the F1 generation was significantly lower than that of the control after exposure of pupae to the LLT25 and LLT50 treatments, with values of 13.53 days and 10.57 days, respectively. Additionally, the adult longevity of the F1 generation was 11.43 days after the adults were subjected to the short-term treatment of LLT50, and this value was also significantly lower than that of the control (F6,203 = 6.68, p = 0.0001) (Figure 4).
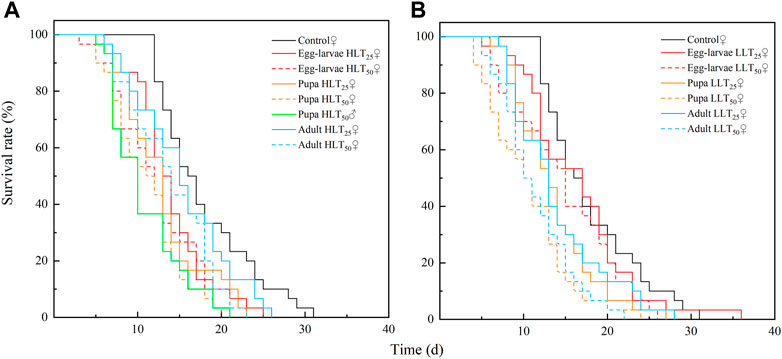
FIGURE 3. Survival rate of the F1 adult of Encarsia formosa (host: Bemisia tabaci) under short-term extreme temperature stress at each developmental stage. Note: Fig. (A) presents the adult survival rate of the F1 adult of Encarsia formosa under short-term high-temperature stress at each developmental stage. Fig. (B) presents the adult survival rate of the F1 adult of E. formosa under short-term low-temperature stress at each developmental stage.
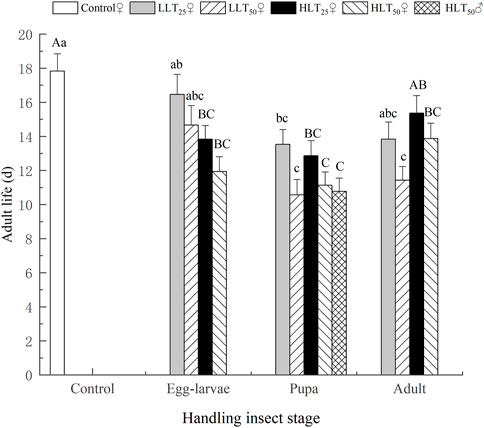
FIGURE 4. Adult longevity of the F1 generation of Encarsia formosa (host: Bemisia tabaci) under short-term extreme temperature stress at each developmental stage. Note: The different capital letters above the bars denote significant differences within the same developmental stages in the high-temperature groups after treatment, based on the results of Tukey’s test (p < 0.05). The different lowercase letters above the bars denote significant differences within the same developmental stages in the low-temperature groups after treatment, based on the results of Tukey’s test (p < 0.05).
4 Discussion
Insects have a specific temperature range suitable for their growth, and temperatures beyond this range can inhibit their normal life activities and even result in death (Bowler and Terblanche, 2008). The tolerance of insects to temperature varies at different developmental stages or instar stages (Kingsolver et al., 2011). The Bogert effect hypothesis suggests that non-mobile stages (eggs and pupae) have greater heat resistance than mobile stages (adults and larvae), as the latter can escape the harmful environment and prevent damage from high temperatures (Huey et al., 2003). Studies have shown that insect pupae contain cold-resistant substances, such as glycerol, sorbitol, and trehalose, enabling greater cold tolerance than other stages (Feng et al., 2018). The present study found that short-term exposure to extreme temperatures significantly affected the corrected mortality of E. formosa at different developmental stages. The sequence of HLT25 and HLT50 under short-term high-temperature stress was pupa > egg–larva > adult, while the sequence of LLT25 and LLT50 under short-term low-temperature stress was pupa < adult < egg–larva. These results indicate that the egg–larvae and adults of E. formosa are less tolerant to short-term extreme temperature stress. Previous studies have also reported that different developmental stages of insects exhibit different tolerances to extreme temperatures. For example, Zhang et al. (2015) found that the pupa of Plutella xylostella (Linnaeus) (Lepidoptera: Plutellidae) had a higher LT50 value than the egg, young larvae, and adults. Yang et al. (2021) reported that the pupa of B. tabaci had greater resistance to high temperatures compared to the egg, larva, and adult. Furthermore, pupae of Spodoptera frugiperda (Smith) (Lepidoptera: Noctuidae) were found to be the most tolerant to low temperatures, with a higher survival rate than other stages at −10°C and −5°C for 2 h (Zhang et al., 2021). Similarly, in this study, pupae of E. formosa were found to have the strongest tolerance to extreme temperature stress, possibly due to their low mobility and ability to resist adverse effects through innate tolerance. Conversely, the egg–larva stage exhibited the weakest tolerance to low-temperature stress, likely due to the frostbite of plant leaves that resulted in reduced survival of B. tabaci, which, in turn, could not provide adequate nutrients to the larvae of E. formosa.
Temperature plays a critical role in insect growth, development, and reproduction (Sánchez-Guillén et al., 2016). As ambient temperature rises, insects can maintain their reproductive ability of the population under high-temperature stress by shortening their developmental duration up to a certain threshold. However, beyond this threshold, the period of insect development increases (Du et al., 2007). Conversely, low temperatures slow down the metabolic rate and activity of physiological substances in insects, inhibiting their growth and development, ultimately affecting the population’s development (Onalethata et al., 2021). In this study, short-term extreme high-temperature stress caused the egg–pupa and egg–adult development durations of E. formosa to shorten, while short-term extreme low-temperature stress prolonged the pupa–adult and egg–adult developmental durations. A higher stress temperature resulted in longer developmental duration. Previous studies have shown that the developmental period of various insect species is affected by temperature stress. For instance, the developmental duration of eggs, larvae, and pupae of Leiometopon simyrides Staudinger (Lepidoptera: Noctuidae) decreased with increasing temperature within the range of 18°C–30°C, but exposure to 33°C slightly increased the developmental duration of eggs, 3rd-instar, and 5th-instar larvae (Gou et al., 2022). In S. frugiperda, Zhang H M et al. (2020) found that exposing eggs and pupae to short-term high-temperature stress affected the growth and development of larvae and pupae, respectively. Huang et al. (2020) reported that the period of development of all stages of Zeugodacus tau (Walker) (Diptera: Tephritidae) and Zeugodacus cucuribitae (Coquillett) (Diptera: Tephritidae) increased as the applied low-temperature decreased. Furthermore, Yu et al. (2022) found that at low temperatures, both the egg and larval stages of Bactrocera dorsalis (Hendel) (Diptera: Tephritidae) developed significantly longer than the control group, with the egg stage prolonging by more than twice at 0°C. Similarly, the egg and larval stages of Galeruca daurica Joannis (Coleoptera: Chrysomelidae) developed for longer periods at low temperatures (Li et al., 2015). These results are consistent with the findings of this study, which suggest that extreme temperatures significantly affect the developmental period of E. formosa at each stage.
When ambient temperature exceeds the optimal range for insect development, energy is devoted towards enhancing tolerance to extreme temperatures, thereby improving survival rate and reducing adult longevity and reproduction (Jervis et al., 2005; Zhao et al., 2017). Wolbachia in parasitic wasps regulates host reproduction by inducing cytoplasmic incompatibility, parthenogenesis, male feminization, and male killing. Inactivation of Wolbachia occurs due to high-temperature stress or antibiotic treatment, which leads to male offspring (Stouthamer and Mak, 2020; Power et al., 2022). The present study showed that short-term exposure to extreme temperature significantly decreased adult longevity and total parasitism after the pupae and adults of E. formosa were subjected to high- or low-temperature stress. Male individuals appeared in the F1 generation after the pupae were subjected to the short-term treatment of HLT50. Aroosa et al. (2022) found that the survival rate and fecundity of green peach aphid [Myzus persicae (Sulzer) (Hemiptera: Aphididae)] were significantly lower than the control after treatment at 36°C for 10 h. Sarmad et al. (2020) reported that the mating probability, fecundity, and adult longevity of Dysdercus koenigii Fabricius (Heteroptera: Pyrrhocoridae) decreased with decreasing stress temperature after exposure to short-term extreme low-temperature stress. Wolbachia density in Nasonia vitripennis (Walker) (Hymenoptera: Pteromalidae) decreased significantly after treatment with critical temperatures, which affected the insect’s reproductive mode and offspring sex ratio (Yang et al., 2020). Zhou et al. (2009) reported that high-temperature shock affects Wolbachia-induced E. formosa reproduction by increasing the proportion of male offspring. Overall, short-term extreme temperature stress could affect parasitism ability and offspring sex ratio in E. formosa, thereby reducing the control effect of E. formosa against pests.
To avoid damage to E. formosa caused by extreme temperatures, weather changes should be observed when releasing E. formosa for field control, and extreme temperature weather should be avoided. During summer, greenhouse facilities should have proper ventilation to reduce the influence of high temperatures on E. formosa growth and offspring sex ratio. During extreme temperature weather, the release amount of E. formosa should be increased to achieve better control over B. tabaci.
Data availability statement
The original contributions presented in the study are included in the article/supplementary material, further inquiries can be directed to the corresponding author.
Author contributions
G-HC, X-MZ, and M-JL designed the study; M-JL, BZ, S-WZ, J-HL, ML, J-LZ, and S-WY performed the experiments; M-JL, BZ, and X-MZ analyzed the data; M-JL, BZ, and X-MZ wrote the manuscript. All authors contributed to the article and approved the submitted version.
Funding
This research was supported by Basic Research in Yunnan Province (202201AT070269), the National Natural Science Foundation of China (31760541), Reserve Talents of Young and Middle-aged Academic and Technological Leaders in Yunnan Province (202105AC160071), the Young Top Talents of “High-level Talents Training Support Program in Yunnan Province” (YNWRQNBJ2020291), and the National Fund for Studying Abroad [LJX (2021) No. 15].
Acknowledgments
We thank Mr. Xingxing Li, Zhengxiong Jiang (Yunnan Agricultural University) for helping us to collect the E. formosa populations in the field. We thank Ms. Yuan Lu, Ye Tian and Mr. Guitao Li (Yunnan Agricultural University) for helping to feed the experimental population. We thank prof. Jian Hu (formerly in Biotechnology and Germplasm Resources Institute, Yunnan Academy of Agricultural Sciences) for helping to provide the B. tabaci MED populations. We thank Elsevier Language Editing translation company for editing the English of the manuscript.
Conflict of interest
M-JL was employed by the Yunnan Yuntianhua Co., Ltd.
The remaining authors declare that the research was conducted in the absence of any commercial or financial relationships that could be construed as a potential conflict of interest.
Publisher’s note
All claims expressed in this article are solely those of the authors and do not necessarily represent those of their affiliated organizations, or those of the publisher, the editors and the reviewers. Any product that may be evaluated in this article, or claim that may be made by its manufacturer, is not guaranteed or endorsed by the publisher.
References
Aroosa, K., Rehan, I., Shahbaz, A., Ansa, T., Muhammad, B. T., Yasir, N., et al. (2022). Effect of short-term heat stress on life table parameters of green peach aphid [Myzus persicae (Sulzer) (Hemiptera: Aphididae)]. J. King Saud. Univ. Sci. 34, 102342. doi:10.1016/j.jksus.2022.102342
Basit, M. (2019). Staus of insecticide resistance in Bemisia tabaci: Resistance, cross-resistance, stability of resistance, genetics and fitness costs. Phytoparasitica 47, 207–225. doi:10.1007/s12600–019–00722–5
Bennett, G. M., and Moran, N. A. (2015). Heritable symbiosis: The advantages and perils of an evolutionary rabbit hole. P. Natl. Acad. Sci. U. S. A. 112, 10169–10176. doi:10.1073/pnas.1421388112
Bowler, K., and Terblanche, J. S. (2008). Insect thermal tolerance: What is the role of ontogeny, ageing and senescence? Biol. Rev. 83, 339–355. doi:10.1111/j.1469–185x.2008.00046.x
Chown, S. (2006). Physiological diversity in insects: Ecological and evolutionary contexts. Adv. Insect Physiol. 33, 50–152. doi:10.1016/S0065–2806(06)33002–0
Dai, P., Ruan, C. C., Zang, L. S., Wan, F. H., and Liu, L. Z. (2014). Effects of rearing host species on the host-feeding capacity and parasitism of the whitefly parasitoid Encarsia formosa. J. Insect Sci. 14, 1–10. doi:10.1093/jis/14.1.118
Daniel, G. T., Alex, C. A., Wesley, D., Andrés, L. N., Rosa, A. S. G., and Fabricio, V. (2020). Insect responses to heat: Physiological mechanisms, evolution and ecological implications in a warming world. Biol. Rev. Camb. Philos. Soc. 95, 802–821. doi:10.1111/brv.12588
Deeksha, C., Ghongade, D. S., and Sood, A. K. (2023). Biological characteristics and parasitization potential of Encarsia formosa Gahan (Hymenoptera: Aphelinidae) on the whitefly, Trialeurodes vaporariorum Westwood (Hemiptera: Aleyrodidae), a pest of greenhouse crops in north-Western Indian Himalayas. Egypt. J. Biol. Pest Control 33, 3. doi:10.1186/s41938–023–00646–7
Du, Y., Ma, C. S., Zhao, Q. H., Ma, G., and Yang, H. P. (2007). Effects of heat stress on physiological and biochemical mechanisms of insects: A literature review. Acta Ecol. Sin. 27, 1565–1572. doi:10.3321/j.issn:1000–0933.2007.04.037
Feng, Y. Q., Zhang, L., Li, W. B., Yang, X., and Zong, S. X. (2018). Cold hardiness of overwintering larvae of Sphenoptera sp. (Coleoptera: Buprestidae) in Western China. J. Econ. Entomol. 111, 247–251. doi:10.1093/jee/tox304
Gou, W. S., Ma, W. X., Liu, N. Y., Hu, G. X., and Sun, Y. D. (2022). Effects of temperature on the growth, development, adult longevity and reproduction of Leiometopon simyrides (Lepidoptera:Noctuidae). Chin. J. Appl. Entomol. 59, 1412–1420. doi:10.7679/j.issn.2095–1353.2022.142
He, Y. Y., Liu, Y. C., Wang, K., Zhang, Y. J., Wu, Q. J., and Wang, S. L. (2019). Development and fitness of the parasitoid, Encarsia formosa (hymenoptera: Aphelinidae), on the B and Q of the sweetpotato whitefly (Hemiptera: Aleyrodidae). J. Econ. Entomol. 112, 2597–2603. doi:10.1093/jee/toz200
Huang, Y. Y., Gu, X. P., Peng, X. Q., Tao, M., Peng, L., Chen, G. H., et al. (2020). Effect of short-term low temperature on the growth, development, and reproduction of Bactrocera tau (Diptera: Tephritidae) and Bactrocera cucurbitae. J. Econ. Entomol. 113, 2141–2149. doi:10.1093/jee/toaa140
Huey, R. B., Hertz, P. E., and Sinervo, B. (2003). Behavioral drive versus behavioral inertia in evolution: A null model approach. Am. Nat. 161, 357–366. doi:10.1086/346135
Isaac, T., Elias, N. N., Apollin, F. K., Armand, D. F., Samuel, N. N., Francis, N. A., et al. (2023). Parasitism of the whitefly Bemisia tabaci by aphelinid parasitoids on cassava across five agro-ecological zones of Cameroon. Crop Prot. 168, 106241. doi:10.1016/j.cropro.2023.106241
Izumi, O., and Makoto, O. (2006). Effect of low temperature and short day length exposure on the development of Aphidius gifuensis Ashmead (Hymenoptera: Braconidae). Appl. Entomol. Zool. 41, 555–559. doi:10.1303/aez.2006.555
Jervis, M. A., Boggs, C. L., and Ferns, P. N. (2005). Egg maturation strategy and its associated trade-offs: A synthesis focusing on Lepidoptera. Ecol. Entomol. 30, 359–375. doi:10.1111/j.0307–6946.2005.00712.x
Jiang, J. X., Yang, J. H., Ji, X. Y., Zhang, H., and Wan, N. F. (2018). Experimental temperature elevation promotes the cooperative ability of two natural enemies in the control of insect herbivores. Biol. Control 117, 52–62. doi:10.1016/j.biocontrol.2017.09.001
Kanakala, S., and Ghanim, M. (2019). Global genetic diversity and geographical distribution of Bemisia tabaci and its bacterial endosymbionts. PloS One 14, e0213946. doi:10.1371/journal.pone.0213946
Kingsolver, J. G., Woods, A., Buckley, L. B., Potter, K. A., Maclean, H. J., and Higgins, J. K. (2011). Complex life cycles and the responses of insects to climate change. Integr. Comp. Biol. 51, 719–732. doi:10.1093/icb/icr015
Klockmann, M., Günter, F., and Fischer, K. (2017a). Heat resistance throughout ontogeny: Body size constrins thermal tolerance. Glob. Change Biol. 23, 686–696. doi:10.1111/gcb.13407
Klockmann, M., Krenschmidt, F., and Fischer, K. (2017b). Carried over: Heat stress in the egg stage reduces subsequent performance in a buttery. Plos One 12, e0180968. doi:10.1371/journal.pone.0180968
Lee, Y. S. (2020). Push-pull strategy for control of sweet-potato whitefly, Bemisia tabaci (Hemiptera Aleyrodidae) in a tomato greenhouse. Korean Soc. Appl. Entomol. 58, 209–218. doi:10.5656/KSAE.2019.08.0.012
Lenteren, J. C. V., Bolckmans, K., Köhl, J., Ravensberg, W. J., and Urbaneja, A. (2018). Biological control using invertebrates and microorganisms: Plenty of new opportunities. BioControl 63, 39–59. doi:10.1007/s10526–017–9801–4
Li, H., Zhou, X. R., Pang, B. P., Zhang, Z. R., Chang, J., and Shan, Y. M. (2015). Effects of low temperature stress on the supercooling capacity and development of Galeruca daurica Joannis larvae (Coleoptera: Chrysomelidae). Chin. J. Appl. Entomol. 52, 434–439. doi:10.7679/j.issn.2095?1353.2015.048
Li, M. J., Yang, S. W., Chen, G. H., Dou, W. J., Shang, H. P., and Zhang, X. M. (2022). Density and seasonal dynamics of Bemisia tabaci and its predators in different agricultural landscapes in South China. Front. Plant Sci. 13, 928634. doi:10.3389/FPLS.2022.928634
Li, Q. F. (2017). Preventative method of Trialeurodes vaporariorum by Encarsia formosa and its release technique. Hortic. Seed 3, 21–23. doi:10.16530/j.cnki.cn21–1574/s.2017.03.009
Liu, S, M., Zhou, J. C., He, Y., Xu, J. W., and Dong, H. (2021). Effects of short-term extreme heat on adult mortality and offspring fitness of Trichogramma dendrolimi Matsumura (Hymenoptera: Trichogrammatidae). J. Environ. Entomol. 43, 199–205. doi:10.3969/j.issn.1674–0858.2021.01.20
Liu, T. X. (2017). Life history of Eretmocerus melanoscutus (hymenoptera: Aphelinidae) parasitizing nymphs of Bemisia tabaci biotype B (Homoptera: Aleyrodidae). Biol. Control 42, 77–85. doi:10.1016/j.biocontrol.2007.03.008
Meehl, G. A., and Tebaldi, C. (2004). More intense, more frequent, and longer lasting heat waves in the 21st century. Science 305, 994–997. doi:10.1126/science.1098704
Onalethata, K., Reyard, M., Hannalene, D. P., and Casper, N. (2021). Developmental stage variation in Spodoptera frugiperda (Lepidoptera: Noctuidae) low temperature tolerance: Implications for overwintering. Austral Entomol. 60, 400–410. doi:10.1111/aen.12536
OuYang, F., and Ge, F. (2014). Methodology of measuring and analyzing insect cold hardiness. Chin. J. Appl. Entomol. 51, 1646–1652. doi:10.7679/j.issn.2095–1353.2014.194
Pan, L. L., Du, H., Ye, X. T., and Wang, X. W. (2021). Whitefly adaptation to and manipulation of plant resistance. Sci. China Life Sci. 64, 648–651. doi:10.1007/S11427–020–1890–9
Power, N. R., Rugman-Jones, P. F., Stouthamer, R., Ganjisaffar, F., and Perring, T. M. (2022). High temperature mortality of Wolbachia impacts the sex ratio of the parasitoid Ooencyrtus mirus (Hymenoptera: Encyrtidae). PeerJ 10, e13912. doi:10.7717/peerj.13912
Pumhan, N., Tian, M., Xu, L. L., Jiang, J., Liu, T. X., and Zhang, S. Z. (2020). Effects of heat stress and exposure duration on survival characteristics of different developmental stages of Propylaea japonica, a dominant aphidophagous ladybeetle in China. Crop Prot. 130, 105054. doi:10.1016/j.cropro.2019.105054
Roda, A., Castillo, J., Allen, C., Urbaneja, A., Pérez-Hedo, M., Weihman, S., et al. (2020). Biological control potential and drawbacks of three zoophytophagous mirid predators against Bemisia tabaci in the United States. Insects 11, 670. doi:10.3390/insects11100670
Sánchez-Guillén, R. A., Córdoba-Aguilar, A., Hansson, B., Ott, G., and Wellenreuther, M. (2016). Evolutionary consequences of climate-induced range shifts in insects. Biol. Rev. 91, 1050–1064. doi:10.1111/brv.12204
Sarmad, M., Ishfaq, A., Arif, H., and Zaka, S. M. (2020). Effect of short-term cold temperature stress on development, survival and reproduction of Dysdercus koenigii (Hemiptera: Pyrrhocoridae). Cryobiology 92, 47–52. doi:10.1016/j.cryobiol.2019.09.015
Stouthamer, R., and Mak, F. (2020). Influence of antibiotics on the offspring production of the Wolbachia - infected parthenogenetic parasitoid Encarsia formosa. J. Invertebr. Pathol. 80, 41–45. doi:10.1016/S0022–2011(02)00034–4
Wang, J., Wang, M. Q., Liu, C. X., Chen, H. Y., and Zhang, L. S. (2019). Host selection of Encarsia formosa Gahan to whiteflies and developmental performances of the parasitoid offspring. Chin. J. Biol. Control 35, 159–166. doi:10.16409/j.cnki.2095–039x.2019.02.012
Wang, T., Keller, M. A., and Hogendoorn, K. (2018). The effects of temperature on the development, fecundity and mortality of Eretmocerus warrae: Is Eretmocerus warrae better adapted to high temperatures than Encarsia formosa? Pest Manag. Sci. 75, 702–707. doi:10.1002/ps.5169
Wang, Y. M., Wu, J. X., and Wan, F. H. (2010). Response of insects to extreme high and low temperature stresses. J. Environ. Entomol. 32, 250–255. doi:10.3969/j.issn.1674–0858.2010.02.018
Yang, S. W., Tian, Y., Lu, Y., Zhang, B., Chen, G. H., and Zhang, X. M. (2023). Sublethal effect of spirotetramat on parasitic ability of Encarsia formosa Gahan. Plant Prot. 49 (2), 298–303+337. doi:10.16688/j.zwbh.2022021
Yang, W. W., Chu, X., Li, J., and Wang, N. X. (2020). Two-sex life table of Nasonia vitripennis (Hymenoptera: Pteromalidae) and the effects of Wolbachia on its reproduction and parasitism of Musca domestica (Diptera: Muscidae). J. Asia-Pac. Entomol. 24, 436–442. doi:10.1016/J.ASPEN.2020.12.010
Yang, Y. W., Liu, C., Ren, P., Chang, Q., and Li, Y, M. (2021). Response of Bemisia tabaci to high and low temperature stress and ecological control measures. Acta agricu. Boreali-occidentalis Sin. 30, 782–788. doi:10.7606/j.issn.1004–1389.2021.05.019
Yin, Y. Y., Zhai, Y. F., Lin, Q. C., Yu, Y., Zhen, L., and Chen, H. (2018). Influence of temperature on the parasitism and host-feeding capacity and of Encarsia formosa. J. Environ. Entomol. 40, 1370–1374. doi:10.3969/j.issn.1674–0858.2018.06.21
Yu, C., Zhao, R., Zhou, W., Pan, Y., Tian, H., Yin, Z., et al. (2022). Fruit fly in a challenging environment: Impact of short-term temperature stress on the survival, development, reproduction, and trehalose metabolism of Bactrocera dorsalis (Diptera: Tephritidae). Insects 13, 753. doi:10.3390/insects13080753
Zhang, H. M., Wang, Y., Yin, Y. Q., Li, X. Y., Chen, F. S., and Chen, A. D. (2020). Effects of short-term high temperature in egg and pupal stages on the growth and development of fall armyworm Spodoptera frugiperda. J. Plant Prot. 47, 831–838. doi:10.13802/j.cnki.zwbhxb.2020.2020820
Zhang, H. M., Wang, Y., Zhao, X. Q., Yin, Y. Q., Li, X. Y., Liu, Y., et al. (2022). Effects of low temperature storage on the growth and development of pupae and adults of Diadegma semiclausum. Chin. J. Biol. Control 38, 595–601. doi:10.16409/j.cnki.2095–039x.2022.05.003
Zhang, T. Q., Zhang, L., Cheng, Y. X., and Jiang, X. F. (2021). Study on the cold resistance of the fall armyworm, Spodoptera frugiperda. Plant Prot. 47, 176–181. doi:10.16688/j.zwbh.2020265
Zhang, W., Chang, X. Q., Hoffmann, A., Zhang, S., and Ma, C. S. (2015). Impact of hot events at different developmental stages of a moth: The closer to adult stage, the less reproductive output. Sci. Rep. 5, 1–9. doi:10.1038/srep10436
Zhang, X. M., Lövei, G. L., Ferrante, M., Yang, N. W., and Wan, F. H. (2020). The potential of trap and barrier cropping to decrease densities of the whitefly Bemisia tabaci MED on cotton in China. Pest Manag. Sci. 76, 366–374. doi:10.1002/ps.5524
Zhao, F., Hoffmann, A. A., Xing, K., and Ma, C. S. (2017). Life stages of an aphid living under similar thermal conditions differ in thermal performance. J. Insect Physiol. 99, 1–7. doi:10.1016/j.jinsphys.2017.03.003
Zhou, S. X., Li, Y., and Zhang, F. (2009). Influences of high temperature shock on the reproduction and development of the Wolbachia-induced parthenogenetic parasitoid wasp, Encarsia formosa (Gahan). Acta Ecol. Sin. 29, 4732–4737. doi:10.3321/j.issn:1000–0933.2009.09.017
Keywords: Bemisia tabaci, Encarsia formosa, extreme temperature, development, reproductive capacity
Citation: Li M-J, Zhang B, Chen G-H, Zhou S-W, Liu J-H, Lu M, Zhang J-L, Yang S-W and Zhang X-M (2023) Effects of short-term extreme temperature treatment on the development and reproductive capacity of Encarsia formosa. Front. Physiol. 14:1187743. doi: 10.3389/fphys.2023.1187743
Received: 21 March 2023; Accepted: 18 May 2023;
Published: 30 May 2023.
Edited by:
Waqas Wakil, University of Agriculture, PakistanReviewed by:
Parul Chaudhary, Graphic Era Hill University, IndiaDori Edson Nava, Embrapa Clima Temperado, Brazil
Nikoleta Eleftheriadou, Agricultural University of Athens, Greece
Copyright © 2023 Li, Zhang, Chen, Zhou, Liu, Lu, Zhang, Yang and Zhang. This is an open-access article distributed under the terms of the Creative Commons Attribution License (CC BY). The use, distribution or reproduction in other forums is permitted, provided the original author(s) and the copyright owner(s) are credited and that the original publication in this journal is cited, in accordance with accepted academic practice. No use, distribution or reproduction is permitted which does not comply with these terms.
*Correspondence: Xiao-Ming Zhang, enhtYWxleEAxMjYuY29t
†These authors have contributed equally to this work and share first authorship