- Department of Physiology, Anatomy and Genetics, The University of Oxford, Oxford, United Kingdom
cAMP is a universal second messenger that relies on precise spatio-temporal regulation to control varied, and often opposing, cellular functions. This is achieved via selective activation of effectors embedded in multiprotein complexes, or signalosomes, that reside at distinct subcellular locations. cAMP is also one of many pathways known to operate within the primary cilium. Dysfunction of ciliary signaling leads to a class of diseases known as ciliopathies. In Autosomal Dominant Polycystic Kidney Disease (ADPKD), a ciliopathy characterized by the formation of fluid-filled kidney cysts, upregulation of cAMP signaling is known to drive cystogenesis. For decades it has been debated whether the primary cilium is an independent cAMP sub-compartment, or whether it shares a diffusible pool of cAMP with the cell body. Recent studies now suggest it is a specific pool of cAMP generated in the cilium that propels cyst formation in ADPKD, supporting the notion that this antenna-like organelle is a compartment within which cAMP signaling occurs independently from cAMP signaling in the bulk cytosol. Here we present examples of cAMP function in the cilium which suggest this mysterious organelle is home to more than one cAMP signalosome. We review evidence that ciliary membrane localization of G-Protein Coupled Receptors (GPCRs) determines their downstream function and discuss how optogenetic tools have contributed to establish that cAMP generated in the primary cilium can drive cystogenesis.
Introduction
Compartmentalization of the cAMP pathway
The primary cilium has recently protruded from obscurity as an intriguing example of compartmentalized cAMP signaling. cAMP is a second messenger that translates signals from hormones, neurotransmitters, and other extracellular stimuli into a variety of intracellular responses, ranging from cell proliferation to apoptosis, from cell differentiation to regulation of metabolic functions. (Yamamoto et al., 1988; Weissinger et al., 1997; Stork and Schmitt, 2002). Such disparate outcomes are achieved through cAMP-dependent modulation of a limited number of effectors that, in turn, can impact a myriad of different targets, including channels, receptors, transporters and pumps, the cytoskeleton, the transcriptional machinery, metabolic enzymes and many others. Fidelity of signal transduction from a specific extracellular stimulus to the appropriate cellular function is achieved through tight spatio-temporal control of the cAMP signal. (Zaccolo et al., 2021). cAMP is synthesized from ATP by adenylyl cyclases (ACs). Activation of a G-protein coupled receptor (GPCR) by an extracellular signal (Levitzki, 1988; Mons et al., 1998; Selbie and Hill, 1998) leads to its association with a G protein, a trimeric αβγ complex, where the α subunit can either trigger (Gαs) or supress (Gαi) AC activity, resulting in increased or reduced cAMP synthesis, respectively (Rodbell et al., 1971). Mammalian cells express nine different membrane-integral AC isoforms (AC1-9) and one soluble isoform, AC10, that is not activated by Gαs but by bicarbonate (Dessauer et al., 2017; Wiggins et al., 2018). Once generated, cAMP binds to a few effector proteins: protein kinase A (PKA) (Walsh et al., 1968; Taylor et al., 2008), the exchange protein directly activated by cAMP (EPAC) (de Rooij et al., 1998), cyclic nucleotide-gated ion channels (Fesenko et al., 1985), and Popeye domain containing proteins (POPDC) (Brand, 2005). PKA, the most extensively studied cAMP effector, when inactive is a heterotetramer resulting from the association of a regulatory (R) dimer and two catalytic (C) subunits. (Walsh et al., 1968; Corbin and Keely, 1977; Taylor et al., 1993). Combinations of 4R subunits (RIα, RIβ, RIIα, and RIIβ) and 3C subunits (Cα, Cβ, and Cϒ) can make up the inactive PKA holoenzyme R2C2. (Diskar et al., 2010). When cAMP binds to the R subunits, a conformational change occurs, releasing the inhibitory effect of R over the 2C subunits, allowing the C subunits to phosphorylate protein targets (Kim et al., 2007; Taylor et al., 2013). Although for a long time the consensus has been that, upon cAMP binding to R subunits the C subunit dissociates from the holoenzyme to then go on to phosphorylate target proteins, the concept of a freely diffusing C subunit is at odds with the model of compartmentalisation. More recently, alternative hypotheses have been put forwards to explain how the effects of active PKA may remain local (Chao et al., 2019). In one model, target phosphorylation does not require C dissociation from R subunit but binding of cAMP is sufficient to induce a conformational change that exposes the catalytic pocket in C which can then phosphorylate targets within a range of about 15–25 nm (Smith et al., 2017). An alternative hypothesis is that cells express a significant excess of R subunits, that are therefore not coupled with C. On activation of PKA, C is released but can quickly be recaptured by free R subunits that are present nearby. (Walker-Gray et al., 2017).
cAMP is hydrolysed by phosphodiesterases (PDEs). PDEs are a super-family of enzymes that includes 11 families (PDE1-11). Of these, PDE4, PDE7, and PDE8 selectively hydrolyse cAMP. PDE1, PDE2, PDE3, PDE10, and PDE11 hydrolyse both cAMP and cGMP, whereas PDE5, PDE6, and PDE9 are cGMP selective. Several families of PDEs include multiple genes and each gene can generate differently spliced transcripts or be transcribed from different initiation sites, resulting in a large number of PDE isoforms. These isoforms display different affinities for cAMP and enzyme kinetics, their activity can be regulated by different mechanisms (e.g., phosphorylation, Ca2+ or cGMP binding) and individual isoforms localise to specific subcellular sites, providing a means to generate subcellular cAMP gradients, or confined cAMP pools, via differential cAMP degradation at different locations. (Marchmont and Houslay, 1980; Conti et al., 1984; Jurevicius and Fischmeister, 1996; Mongillo et al., 2004; Bender and Beavo, 2006; Lugnier, 2006; Di Benedetto et al., 2008). In addition to the PDEs, the cAMP signal mediated by PKA, can also be quenched by phosphatases (PPs), which counteract PKA phosphorylation of target proteins (Ingebritsen and Cohen, 1983; Francis et al., 2011). A further class of proteins that contributes to compartmentalise the cAMP signal are the A-kinase Anchoring Proteins (AKAPs) which dock PKA to specific cellular locations where proteins that are phosphorylated by PKA are also localised. As a result, when the AKAP-tethered PKA is activated by cAMP, it preferentially phosphorylates the nearby target. In addition to PKA and its targets, AKAPs can also bind other signaling molecules, thus assembling signaling platforms, or signalosomes, where the cAMP signal can be locally generated (e.g., through AKAP association with GPCR or ACs), delivered (via tethered of PKA or EPAC) and regulated (through association of AKAPs with PDEs, PPs, other kinases) (Colledge and Scott, 1999; Wong and Scott, 2004).
The primary cilium
Due to the compartmentalised nature of cAMP signaling, it is no surprise that often organelles are the sites where cAMP signalosomes are found. (Langeberg and Scott, 2015; Monterisi and Zaccolo, 2017; Torres-Quesada et al., 2017). The primary cilium, an organelle that had long been ignored since it was first discovered in 1898 by Zimmerman, (Zimmermann, 1898), has more recently garnered much attention due to the fact that mutations in ciliary proteins lead to a family of diseases known as “ciliopathies”, which are characterised by developmental defects, sensory malfunction, obesity, reproductive problems, and cysts of the liver and kidneys (Hildebrandt et al., 2011). The primary cilium is a thin projection that extends from the surface of most mammalian cells, consisting of a microtubule-based axoneme and a surrounding membrane. The cilium is anchored to the cell by a basal body, which is derived from the centrosome. This antenna-like organelle plays a vital role in mechanosensory functions (Bangs and Anderson, 2017; Kiesel et al., 2020; Ho and Stearns, 2021), such as fluid-flow sensing, and is important for photoreception (Bujakowska et al., 2017), and olfaction (Doroquez et al., 2014). The cilium is also a hub for cell signaling, hosting a variety of pathways including Sonic Hedgehog, Wnt, Notch, Hippo, PDGF, MTOR, TGF-beta, Ca2+ and cAMP signaling (Wheway et al., 2018). In the first identified ciliopathy, Autosomal Dominant Polycystic Kidney Disease (ADPKD), excessive cAMP signaling was shown to drive cystogenesis. ADPKD is caused by mutations in either Polycystin 1 (PC1) or Polycystin 2 (PC2), two ciliary proteins which regulate intracellular and ciliary Ca2+ signaling. cAMP and Ca2+ signaling were demonstrated to work hand-in hand to elicit intracellular and ciliary responses, but there has been much debate on whether the primary cilium harbors its own pool of cAMP, independent of cytosolic cAMP, or whether this second messenger freely diffuses between the organelle and the cell body. Recent research supports the notion that it is ciliary cAMP which drives cystogenesis in ADPKD and that ciliary cAMP signaling controls Hedgehog transcription. (Moore et al., 2016; Hansen et al., 2022). A functionally independent pool of ciliary cAMP begs the question of whether specific cAMP signalosomes exist within the cilium, especially since the primary cilium and the cAMP pathway are involved in a variety of intracellular processes. Below we discuss the evidence in support of multiple cAMP subdomains being present within the cilium and centrosomes, we explore the role of cAMP compartmentalisation in the context of ADPKD and we discuss the technology that has been used, and could be further developed, to detect compartmentalisation of the cAMP signal within the organelle.
cAMP signalosomes in the primary cilium
To understand how the primary cilium could function as a compartmentalized cAMP microenvironment, it is important to consider the structure of the primary cilium. The organelle is highly conserved through eukaryotic evolution and is found from Chlamydomonas and Tetrahymena to worms, flies, mice and humans. (Hilgendorf et al., 2016). The axoneme of the cilium extends from the basal body, which forms from the mother centriole of most cell types during cell cycle arrest. The basal body is constituted by a group of specialized proteins which function as a platform for cilia growth. (Satir and Christensen, 2007). A transition zone (TZ) separates the ciliary sub-domain from the cytosol, strictly gating the proteins of the ciliary compartment (Reiter et al., 2012). Mutations in proteins of the transition zone lead to ciliopathies, such as Meckel-Gruber Syndrome (MKS), Joubert Syndrome (JBTS), Bardet-Biedl Syndrome (BBS), and Nephronophthisis (NPHP) (Reiter et al., 2012). The ciliary membrane differs from the plasma membrane and is constituted of a unique bilayer where the amount and localization of distinct phosphoinositide lipid species is defined by opposing actions of lipid kinases and phosphatases (Conduit and Vanhaesebroeck, 2020). Aside from its intricate structure, the ciliary membrane also hosts a plethora of receptors, including GPCRs (Hilgendorf et al., 2016) and signaling pathways that operate in concert with cAMP signaling. Furthermore, since the surface to volume ratio of the cilium is 13-fold higher than that of the cell body, (Arena and Hofer, 2021), if Gαs-coupled GPCRs have a similar density on ciliary and cell membranes, then the higher surface to volume ratio of the primary cilium should make the organelle a cAMP hotspot. A ciliary pool of cAMP has been shown to regulate the best characterized signaling pathway within the cilium, the Sonic Hedgehog (SHh) pathway (Bangs and Anderson, 2017).
cAMP and regulation of hedgehog activity
Hedgehog signaling works as a mitogen, driving important functions such as growth and morphogenesis in a variety of vertebrate tissues, particularly the nervous system, limbs, and skeleton (Chiang et al., 1996). In the absence of the SHh ligand, the SHh receptor patched 1 (Ptch1) represses the activator smoothened (Smo), inhibiting the downstream pathway. (Figure 1). Binding of one of three ligands, Sonic, Indian, or Desert Hedgehog to Ptch1, releases Smo from Ptch1, allowing Smo to mediate splicing of activating forms of glioma-associated oncogene (Gli) transcription factors. (Ho and Stearns, 2021) (Figure 1). Primary cilia play three different roles in Hedgehog signaling. Firstly, as the location where the Ptch1 receptor is concentrated, the cilium is the site of initiation of Hedgehog signaling. Secondly, Ptch1 repression of Smo occurs in the cilium, and when signaling is activated, Smo accumulates in the ciliary membrane (Corbit et al., 2005). Thirdly, the primary cilium is necessary for the processing of Gli2 and Gli3, as these transcription factors travel via Intra Flagellar Transport (IFT) to the top of the cilia, where they are cleaved into activator or repressor forms, translating the SHh signal into changes in cellular function (Huangfu et al., 2003; Haycraft et al., 2005; Ho and Stearns, 2021). The hedgehog pathway is known to be regulated by cAMP signaling through a negative feedback loop, orchestrated at the base of the cilium, whereby PKA phosphorylation of Gli2 restrains its activation (Tuson et al., 2011). In response, SHh reduces ciliary cAMP and PKA activity through regulation of phosphatidylinositol (3,4,5)-triphosphate (PIP3) and consequent Ca2+ signaling, which inhibits AC5 and AC6 and cAMP synthesis (Moore et al., 2016). Such a balanced mechanism between cAMP and Hedgehog would require tight spatio-temporal control of both pathways, and would therefore suggest a ciliary cAMP signalosome is present in the cilium to direct this process.
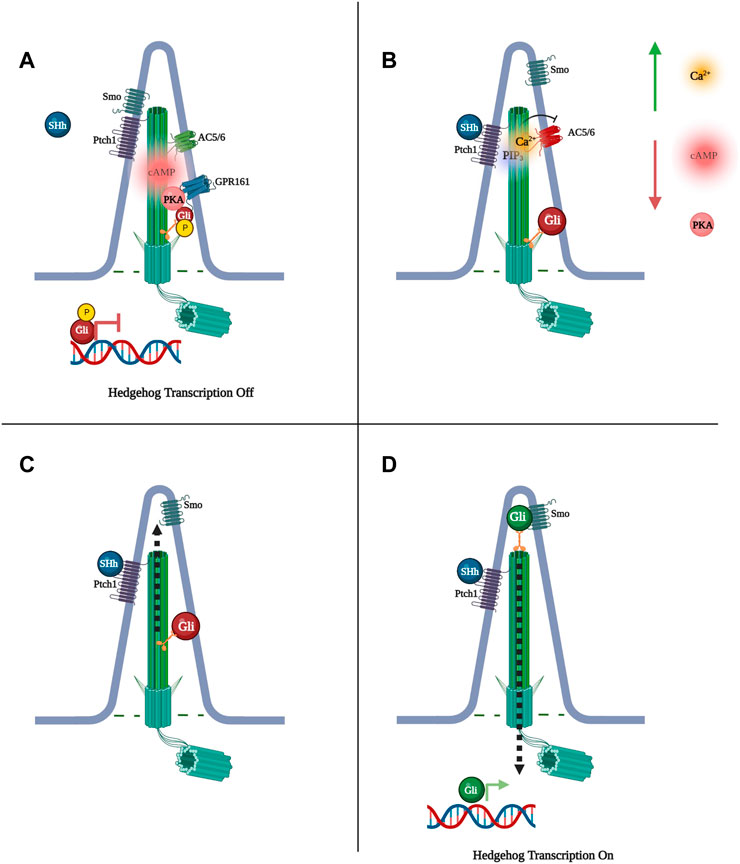
FIGURE 1. Sonic Hedgehog (SHh) signaling is regulated by Protein Kinase A (PKA) phosphorylation of glioma-associated oncogene (Gli) transcription factors. (A) When PKA phosphorylates Gli, Gli cannot be processed by Smo into its active state and SHh-dependent transcription does not occur. (B) Binding of Shh to Pitch1 activates phosphatidylinositol (3,4,5)-triphosphate (PIP3), increasing ciliary Ca2+ levels and preventing cAMP synthesis via Ca2+-inhibited adenylyl cyclase 5 and 6 (AC5/6), subsequently decreasing PKA phosphorylation of Gli. (C) Gli moves to the tip of the primary cilium. (D) Smoothened (Smo) is freed to activate Gli, Gli exits the cilium to initiate transcription.
Studies have identified the orphan receptor of the rhodopsin GPCR family, Gpr161, to localize to the cilium, where its transport is regulated by the tubby family protein Tulp3 and IFT-A. (Mukhopadhyay et al., 2013). Constitutive activity of Gpr161 increases cAMP in the cilium, altering Gli3 to its repressor form, resulting in suppression of SHh signaling. PKA phosphorylation of Gli at the base of the cilium leads to Gli repression, suggesting that Gpr161 works through cAMP and subsequent PKA activation to silence SHh signaling (Huangfu et al., 2003; Haycraft et al., 2005; Ho and Stearns, 2021). Furthermore, SHh signaling results in Gpr161 exit from the cilium, suppressing receptor signaling, while loss of Gpr161 leads to SHh hyperactivity, supporting a feedback loop between Gpr161 and SHh. (Mukhopadhyay et al., 2013). Research in zebrafish also identified Gpr161 as a high-affinity AKAP for PKA RI whereby RI binds the hydrophobic protein-protein interaction interface in the cytoplasmic terminal tail of Gpr161. This particular study showed that the RI-Gpr161 complex localizes to the plasma membrane but, on overexpression, RI and Gpr161 were shown to also interact at the primary cilium. A L465 mutation in Gpr161, which disrupts Gpr161 interaction with PKA, led to significantly reduced ciliary localization of RIα-GFP, suggesting Gpr161 functions at the cilium as an AKAP for PKA. (Bachmann et al., 2016; Pal et al., 2016). Interestingly, in primary hippocampal neurons Gpr161 has been suggested to couple with the calcium activated AC3 (Mukhopadhyay et al., 2013), potentially constituting a signalosome that is distinct from the Ptch1/PIP3/AC5/6, and undergoes opposing regulation by calcium. The endogenous ligand for Gpr161 remains unknown.
Another orphan receptor that has been identified in the primary cilium, Gpr175, was shown to interact with ciliary Gαi to decrease cAMP levels and PKA activity, modulating the formation of Gli repressor. However, Gpr175 depletion only led to a small reduction in SHh signalling, suggesting that Gpr175 plays a regulatory, rather than an essential and direct role in the SHh pathway (Singh et al., 2015). Based on these studies, at least one compartmentalised cAMP signal appears to exist in the cilium where it regulates Hedgehog transcription. Whether Gpr161 and Gpr175 are part of the same or independent signalosome is currently unknown.
cAMP signaling and cilium length
The length of the primary cilium has been investigated in relation to ciliopathies such as ADPKD, however it is uncertain whether increased cilia length aggravates disease progression or whether it functions as a compensatory mechanism. The general view is that altered ciliary length in either direction is detrimental to cellular homeostasis (Scarinci et al., 2022). To complicate things further, cAMP appears to contribute both to ciliary growth and retraction, suggesting that more than one cAMP signalosome exists in the cilium to regulate the organelle’s length.
A number of studies support a role of cAMP in primary cilium elongation. For example, Prostaglandin E2 (PGE2) and its Gαs-coupled receptor, EP4, were shown to promote cilia formation as well as elongation (Figure 2). PGE2 is exported from the cell to the extracellular space to bind the EP receptor on the cilium membrane. In Zebrafish the leakytail (lkt) mutation, which affects the ATP-binding cassette transporter protein (ABCC4) responsible for the export of PGE2, shows ciliogenesis deficits (Jin and Zhong, 2022), suggesting the EP4 receptor interacts with specific ciliary ACs to generate cAMP and thus regulate organelle growth.
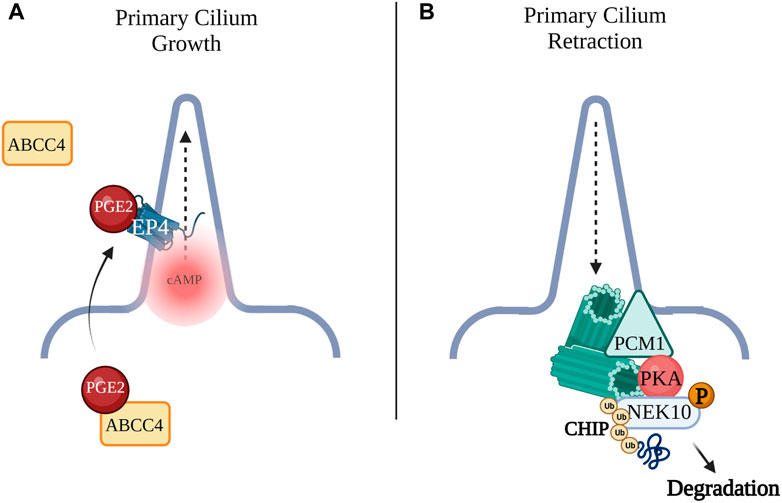
FIGURE 2. (A) Prostaglandin E2 (PGE2) is exported by the ATP-binding cassette transporter protein (ABCC4) across the plasma membrane to the extracellular space where PGE2 can bind the Gαs-coupled receptor, EP4 to promote cilia formation as well as elongation. (B) Pericentriolar material 1 (PCM1) acts as an AKAP for PKA. GPCR activation of PKA leads to phosphorylation of NEK10, a kinase required for ciliary biogenesis. Phosphorylation of NIMA-related Kinase 10 (NEK10) by PKA leads to ubiquitination and proteolysis of the kinase by E3 ligase CHIP leading to cilia retraction.
Another study demonstrated that cAMP and PKA regulate both cilia length and function in vascular endothelial cells. In these cells, activation of PKA significantly increased cilia length through a pathway involving cofilin and protein phosphatase-1 (PP-1)-mediated restructuring of actin stress fibers into cortical filamentous actin. The change in cilia length upon cAMP upregulation also led to enhanced flow sensing activity (Abdul-Majeed et al., 2012).
Consistent with a role of cAMP in promoting cilia length, higher levels of the second messenger are found in cystic tissue from ADPKD patients where the primary cilia of renal cells are abnormally elongated, (Shao et al., 2020), although in the renal ciliopathy it is unclear whether elongated cilia are a result of increased levels of cAMP, or whether altered signaling of a longer cilium leads to enhanced cAMP signaling. Narrowing in on specific ciliary cAMP signalosomes affecting cilium length could help explain why these structural ciliary patterns are observed, and targeting such signalosomes could potentially be a therapeutic strategy for ciliopathies such as ADPKD.
The cAMP pathway not only promotes cilium elongation, but it also appears to drive cilia retraction, thus regulating two opposing cellular functions. The primary cilium and the centrosome are interdependent and are regulated by a complex network of PKA phosphorylation targets, suggesting that distinct cAMP signalosomes may function to control cilia retraction. Primary cilium assembly is induced when cells leave the cell cycle to enter G0 and ciliary vesicles begin to dock at distal sites of the basal body (Porpora et al., 2018). Axonemal microtubules grow and when the nascent cilium fuses with the plasma membrane, it matures (Sánchez and Dynlacht, 2016). The pericentriolar matrix, which acts as a scaffold for ciliogenesis, is constituted by multiple proteins, including pericentriolar material 1 (PCM1). PCM1 depletion has been shown to lead to loss of primary cilia and PCM1 interaction with CEP290 and Bardet-Biedl Syndrome (BBS) proteins are required for ciliogenesis (Kim et al., 2008). CEP290 and PCM1, together, recruit to the cilium Rab8, a small GTPase which functions with the BBS proteins to promote ciliogenesis (Kim et al., 2008). Of note, PCM1 has also been identified as an AKAP that anchors PKA at the centrosome. In addition, GPCR activation of PKA leads to phosphorylation of NIMA-related Kinase 10 (NEK10), a kinase required for ciliary biogenesis in both mammals and lower vertebrates. Phosphorylation of NEK10 by PKA leads to ubiquitination and proteolysis of the kinase by E3 ligase CHIP Figure 2. This suggests that a cAMP signalosome, with PCM1 possibly operating as an AKAP, exists at the centrosomes where it coordinates cilia resorption. (Porpora et al., 2018). Loss of cilia due to aberrant PKA/NEK10/CHIP function was demonstrated in several ciliopathies and cancers such as glioblastoma, astrocytoma, and high-grade prostate cancer (Valente et al., 2014; Porpora et al., 2018).
The PKA/NEK10/CHIP structure is not the only cAMP complex reported at the centrosome. Senatore et al. (2021) used several models, including Medaka fish, to demonstrate that PKA phosphorylation of the Orofacial Digital Type 1 (OFD1) protein coordinates OFD1 proteolysis at the centrosome through the E3 ubiquitin ligase praja2 and the ubiquituin-proteasome system (UPS). In line with these findings, non-phosphorylatable OFD1 mutants were shown to markedly change cilia morphology and dynamics. The impaired ciliogenesis observed in the Medaka fish upon disruption of this signaling axis was found to lead to developmental defects. This data is consistent with the phenotype observed in the OFD1 syndrome, an X-linked congenital ciliopathy which manifests with facial, oral cavity and digital malformations and, in the majority of cases, with polycystic kidney disease. (Senatore et al., 2021). In another study, AC3 stimulation, believed to originate from the primary cilium, led to activation of PKA at the centrosomes of embryonic, postnatal and adult migrating neurons, hinting at a further cAMP signalosome linking the two interdependent organelles. In these neurons, stimulation of centrosomal PKA was demonstrated to regulate centrosome organization, nucleokinesis as well as cell migration (Stoufflet et al., 2020). Whether AC3 forms a complex with PCM1, functioning as an AKAP at the centrosome, and whether AC3 activation leads to downstream phosphorylation of OFD1 or NEK10, is not known. Data however support cAMP compartmentalization at the centrosomes, where the second messenger not only regulates ciliogenesis and cilium retraction, but modulates the cell cycle (Terrin et al., 2012). Excessive cell proliferation is not only a key aspect of ADPKD, but also a prominent driver of cancer and targeting the cAMP signalosome regulating cell cycle progression could help tackle an extensive number of pathological conditions.
GPCR signalling in the cilium
In addition to Gpr161 and Gpr175 discussed above, the primary cilium hosts multiple other GPCRs. Several studies show that the same GPCR can localise to the cilium or to the plasma membrane but achieves distinct outcomes depending on its localisation. For example, the primary cilia of cholangiocytes are mechano-, chemo- and osmosensory organelles that also control proliferation of these epithelial cells. (Masyuk et al., 2006; Masyuk et al. 2008; Masyuk et al. 2013). Cholangiocytes line bile ducts, where they transport bile acid and secrete bicarbonate (Tabibian et al., 2013). Bile acid signalling is transmitted through TGR5, a GPCR which localises to the plasma membrane, the subapical compartment and the cilium (Keitel and Häussinger, 2011; Keitel and Häussinger, 2012; Masyuk et al., 2015). The presence or absence of cilia in cultured cholangiocytes determines the impact of TGR5 agonists. (Masyuk, Masyuk, and LaRusso 2015) In non-ciliated cholangiocytes, TGR5 show marked colocalization with Gαs and its activation leads to elevation in cAMP, ERK pathway inhibition and increased cell proliferation. On the other hand, in ciliated cholangiocytes TGR5 couples to Gαi, and agonist stimulation leads to decreased cAMP, activation of ERK, and reduced cell proliferation (Masyuk et al., 2015). These findings suggest that TGR5 is functionally paired to Gαs at the plasma membrane, but interacts with Gαi when in the cilium.
Other GPCRs may reside in the same primary cilium to control the same process via different signalling mechanisms. For example, Melanocortin-4 Receptor (MC4R) is a GPCR involved in food consumption and mutations in this receptor are the most common cause of monogenic obesity (Vaisse et al., 1998; Vaisse et al., 2000; Lubrano-Berthelier et al., 2006). MC4R was recently reported to localise to the primary cilium and a genetic approach showed that ciliary localisation of MCR4 in neurons is required for proper MC4R Gαs signalling (Walker-Gray et al., 2017). Genetic knock out (KO) of intraflagellar transport protein 88 (lft88) ablated primary cilia in mice and proved cilia are necessary for pharmacological activation of MC4R and subsequent anorexigenic effect. Cilium removal in MC4R-expressing cells from the mouse hypothalamus phenocopied germline loss of MC4R, which is characterised by obesity. Since MCR4 activation leads to AC stimulation, the study also tested whether inhibiting ACs in the cilia of MC4R-expressing neurons had consequences on food intake. Indeed, inhibition of ciliary ACs was achieved by expressing the constitutively active Gαi-coupled receptor, GPR88, exclusively in the cilium. Silencing of AC’s in the organelle caused hyperphagia and obesity. The authors propose that defects in ciliary localisation of MC4R lead to loss of receptor sensitivity, inherited obesity syndromes and accounts for the high rates of obesity found in other ciliopathies (Patel, 1999; Wang et al., 2021). Furthermore, genetically modified mice which cannot transport NPY2R, another GPCR controlling food intake, in neuronal cilia, but normally express the receptor at the plasma membrane, develop an obese phenotype and are insensitive to the anorexigenic ligand PYY3-36, indicating that NPY2R ciliary localisation is vital for ligand-dependant signalling to control food intake (Loktev and Jackson, 2013). Just like MC4R, NPY2R is found in cilia of hypothalamus neurons. Although there is no clear evidence that the two receptors reside in the same cilium, both receptors require ciliary localisation to mediate the same function within the same brain region. Interestingly, NPY2R is coupled to G0/Gi, and thus its activation reduce cAMP, while MC4R is Gαs coupled and its activation increases ciliary cAMP (Figure 3). The contrasting signalling modality of these receptors hints at two separate ciliary signalosomes with different signaling components, potentially localizing within one cilium, working to balance the same process.
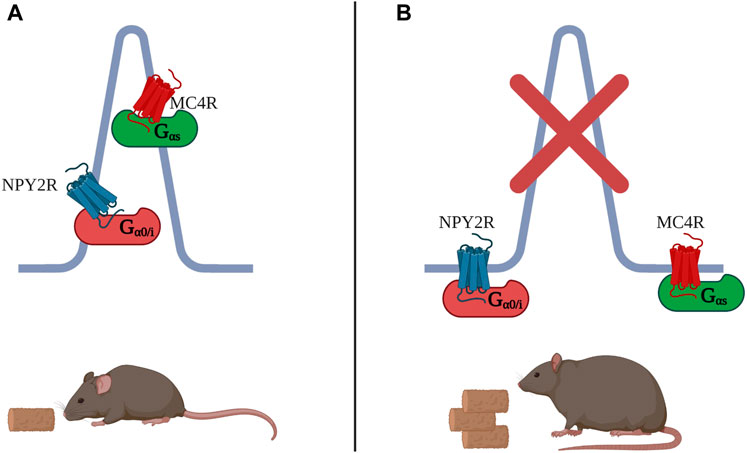
FIGURE 3. (A) MC4R is a Gαs-coupled receptor which localizes to the primary cilium of hypothalamus neurons to control food intake. Also in cilia of hypothalamus neurons, NPY2R regulates food intake, but in contrast to MC4R, it does so through decrease in cAMP, as NPY2R is Gαi-coupled. (B) Both MC4R and NPY2R, when excluded from the cilium, fail to repress food intake. Many ciliopathies as well as complete loss of primary cilia lead to obesity.
Kisspeptin is another GPCR localised to the cilium in gonadotropin-releasing hormone (GnRH) secreting neurons. Kisspeptin signalling is involved in the onset of puberty and mature reproductive function (Koemeter-Cox et al., 2014). In adult animals, multi-ciliated GnRH neurons have several KISS1R-positive primary cilia, and the percentage of these multi-ciliated neurons increases with postnatal and sexual development. Disruption of these cilia leads to a marked decrease in kisspeptin-mediated GnRH action (Koemeter-Cox et al., 2014). Recently, the β-adrenergic receptor (β2AR) has also been shown to localise to neuronal cilia in mouse hippocampus (Yao et al., 2016) where its activation contributes to hippocampal synaptic plasticity (Hagena et al., 2016). Of note, β2AR colocalises in neuronal cilia with the non-selective cation channel polycystic kidney disease 2-like 1 (Pkd2l1). Mice lacking Pkd2l1 have disrupted β2AR ciliary localisation, decreased cAMP in the brain, and higher rates of pentylenetetrazol-induced seizures (Yao et al., 2016). The hypothesis is that the Gαs coupled β2AR forms a complex at the cilium with Pkd2l1 to increase cAMP signalling and inhibit neuronal excitability (Yao et al., 2016).
TGR5, MC4R, NPY2R, β2AR and Kisspeptin are examples of how the same GPCR at the cilium can result in one outcome while a different response is triggered when the same receptor operates from the plasma membrane. How the primary cilium can achieve such feats is still a mystery. One hypothesis would look to the cilium as a highly compartmentalised organelle. Perhaps the complexes that interact with the receptor, such as AKAPs and G-proteins, are distinct at the two locations. Further, the downstream effectors of GPCRs and cAMP at the cilium could be different to effectors localised in the cell body. GPCR in the cilium could also be in closer proximity to downstream functional components and a temporal aspect could be at play whereby cilium elements could be engaged more rapidly than those in the cytosol. Finally, enhanced isolation of the cAMP signal, due to the primary cilium structure, might likewise contribute to the distinct function of these GPCRs. Whatever explanation turns out to be correct, it is likely that compartmentalisation of cAMP at the cilium plays a role.
Ciliary cAMP signalosomes and ADPKD
ADPKD has been linked with multiprotein complexes that could operate as cAMP signalosomes. The vasopressin type 2 receptor (V2R) localises to the primary cilium of the epithelial-like pig kidney cell line (LLC-PK) where it couples with Ca2+ inhibited AC5/AC6 (Figure 4). (Masyuk et al., 2006; Raychowdhury et al., 2009; Chien et al., 2010) Stimulation of V2R with Arginine-Vasopressin (AVP), in the presence of ATP, activates cation-selective channels in the ciliary membrane, to similar levels as addition of the non-specific adenylyl cyclase activator, forskolin. PKA inhibition after V2R stimulation reduced cation channel activity, suggesting V2R signals through ciliary cAMP synthesis for downstream effects such as PKA phosphorylation of aquaporin 2 (AQP2) and the transcription factor cAMP-response element binding protein (CREB) (Raychowdhury et al., 2009). Vasopressin levels are elevated in animal models as well as in patients affected by ADPKD (Meijer et al., 2011; Sherpa et al., 2019). Dysregulation of the V2R-generated cAMP pool in ADPKD is believed to influence fluid secretion and cell proliferation, which promotes cystogenesis (Cassola et al., 1993; Meijer et al., 2011; Reif et al., 2011; Rinschen et al., 2014). Consistently, Tolvaptan, a highly selective V2R antagonist approved for the treatment of ADPKD, has been shown to significantly reduce kidney volume and renal function decline in phase three clinical trials (Cassola et al., 1993).
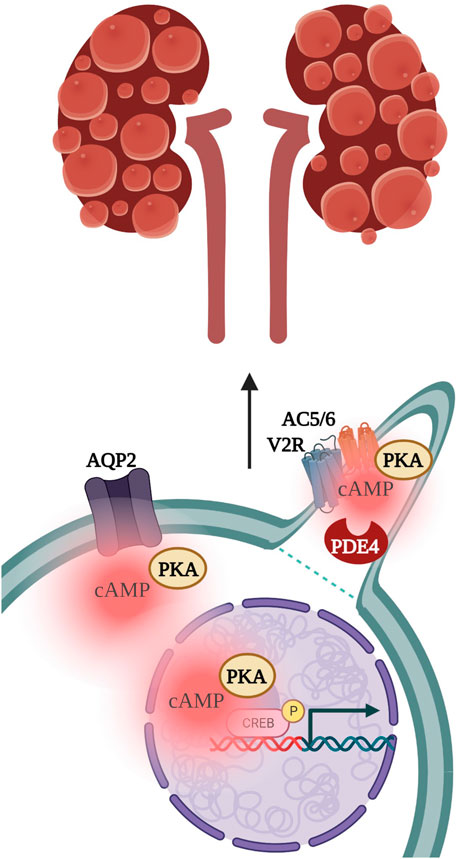
FIGURE 4. Vasopressin stimulation of the vasopressin type 2 receptor (V2R) localised to the primary cilium activates PKA-dependent phosphorylation of Aquaporin 2 (AQP2) channels and of the transcription factor cAMP-response element binding protein (CREB), to subsequently drive kidney cystogenesis. PKA, protein kinase A; AC5/6, adenylyl cyclase 5 and 6; PDE4, phosphodiesterase 4.
Another set of proteins has been shown to form a complex with AC5 and AC6 in the primary cilium. Choi et al. found AC5/AC6 to interact with PDE4C, AKAP150, PKA, as well as Polycystin 1 and Polycystin-2 (Choi et al., 2011). As aforementioned, in ADPKD, a decrease in ciliary Ca2+, due to loss of PC1 or PC2 function activates AC5/6, increasing intracellular cAMP and driving cystogenesis (Choi et al., 2011; Rees et al., 2014; Spirli et al., 2017; Wang et al., 2018). The link between V2R and the PDE4C/AKAP150/PKA complex has not yet been made, but it is possible V2R couples with AC5/6 at AKAP150 to generate a pool of cAMP which functions in a feedback loop with polycystin-regulated Ca2+ activity, and which is controlled by PDE4C function. A way to confirm whether such a hypothesis is correct would be to measure cAMP using a Fluorescence resonance energy transfer (FRET) sensor localising to the AKAP150 complex in the primary cilium. In the final section we will discuss the evolution of imaging techniques that have been used to study cAMP signalling in the primary cilium.
Studying cAMP signalling in the primary cilium with optogenetic tools
FRET imaging of cAMP reporters
Ever since “ciliopathies” such as ADPKD were recognised as a severe class of diseases caused by dysfunction of the primary cilium, understanding ciliary signalling, including cAMP signalling, emerged as a priority. However, measuring cAMP signalling within the cilium, or even imaging the organelle, with its inherent reactional movements and transient nature, is challenging. FRET-based reporters for cAMP have been developed by several groups to narrow in on GPCR signalling in the cilium and, while some findings support the notion that the cilium is an independent cAMP subdomain, others serve to reject this hypothesis. Jiang et al. (2019) developed targeted sensors to measure cAMP in the primary cilium by fusing the EPAC-based sensors EPAC-H187 or EPAC-H188 to Arl13B, a protein exclusively localised to the cilium, (Duldulao et al., 2009), generating Arl13B-H187 and Arl13B-H188. No difference was found in ciliary versus cytosolic cAMP with either Arl13B-H187 or Arl13B-H188 in Inner Medullary Collecting Duct (IMCD3) cells. The authors also investigated which GPCRs are active in the cilium and found that, when overexpressed in IMCD3, V2R is primarily localised at the plasma membrane but vasopressin stimulation led to a sustained cAMP response of equal magnitude in the primary cilium and in the cytosol (Jiang et al., 2019). This finding suggests that cAMP synthesized in response to V2R stimulation at the plasma membrane leads to diffusion of cAMP through the transition zone into the cilium.
The cAMP response to the serotonin receptor, 5-hydroxytryptamine (5-HT6R), was also measured using several different FRET sensors with somewhat contrasting results. In one study, 5HT6 was found to localise to the cilium of primary neuronal cells. Over-expression of the serotonin receptor in the cilium of primary neurons elongated the organelle and increased dendritic branching, with inhibition of the GPCR causing the reverse phenotype. Rescue of 5HT6 in 5HT6R-KO mice was enough to increase cilia length and dendritic expansion, but primarily in neurons where 5HT6 localised exclusively to the cilium rather than also to the plasma membrane (Lesiak et al., 2018). The same study investigated a possible link between enhanced cAMP signalling upon 5-HT6R stimulation and increased cilia length but failed to see changes in cAMP signalling upon receptor stimulation (Lesiak et al., 2018). (Jiang et al. (2019) also set out to measure cAMP signalling upon 5HT6 activation. In their experiments, recombinant mCherry- 5-HT6R showed exclusive localisation to the cilium in some cells, while in others it was found both in the cilium and at the plasma membrane. The latter cells responded to serotonin stimulation with increased cAMP levels both in the cilium and in the cytosol, but cells with ciliary localisation of mCherry- 5-HT6R showed no cAMP response to serotonin. The same result was observed when the FRET sensor was directly fused to 5-HT6R (5-HT6 -EpacH187) (Jiang et al., 2019). These results are in line with previous studies from Lesiak et al. (2018) showing that GPCR activity was only observed after treating cells with ciliary localisation of 5HT6R with Smoothened agonist (SAG) but not without prior treatment, hinting that without SHh signalling there is no Gαs activity of 5HT6R at the cilium (Chaumont-Dubel et al., 2020). In a very elegant follow-up study by Jiang et al. (2019), IMCD3 overexpressing 5-HT6R and treated with digitonin, which results in permeabilization of the plasma membrane but not of the ciliary membrane, showed no cAMP response in the cell body or the cilium on application of 5-HT, indicating lack of 5-HT6R function in the cilium. However, stimulation of the same cells with the non-specific cAMP activator, forskolin, led to a small but reproducible cAMP response in the cilium but not the cytosol, indicating that the primary cilium is capable of generating its own cAMP when GPCR function is bypassed. In other cell types, e.g., 3T3 and mouse embryonic fibroblasts (MEFs), stimulation of endogenous 5-HT6R did produce a detectable increase in cAMP in the cilium, which was greatly enhanced when cells were pre-treated with SAG.
Overall, the findings of Jiang et al. delineate GPCR function in the cilium, but fail to observe a robust difference between the cAMP response at the cilium and the cell body or between the basal cAMP levels in the two compartments. In contrast, Moore et al. generated a PKA activity sensor targeted to the cilium via fusion of 5-HT6R to AKAR4, a FRET-based PKA activity reporter (Depry and Zhang, 2011), and in MEFs showed that PKA activity within the cilium is markedly higher than in the cytosol (Moore et al., 2016). In line with this finding, basal levels of cAMP were determined using the cAMP Difference Detector in situ (cADDis) reporters (Tewson et al., 2016) and mCherry-cADDis in the cell body reported 800 nM basal cAMP whereas 5-HT6 -mCherry-cADDis in the cilium reported 4 μM basal cAMP. Furthermore, after siRNA knock down (KD) of AC5/AC6 in IMCD3, 5-HT6 -mCherry-cADDis detected decreased levels of basal ciliary cAMP, confirming AC5/AC6 are responsible for cAMP production in the cilium. Knock down of Gαs in MEFs was confirmed with β2AR stimulation completely inhibiting cAMP signaling in the cilium. However, Gαs KD did not affect basal ciliary cAMP levels, mirroring Jiang’s findings that ACs function independently to GPCR signalling in the cilium for basal cAMP generation. Moore et al. (2016), also found higher levels of PIP3 in the cilium and hypothesized PIP3 might control AC5/6 function. Live-cell imaging using the FRET PIP3 sensor InPAkt targeted to the cilium via fusion to 5HT6 indeed demonstrated higher local levels of PIP3 compared to the whole cell. Since AC5/6 are inhibited by Ca2+, and SAG raises Ca2+ levels in the cilium, (Delling et al., 2013), the cilium-targeted Ca2+ sensor 5HT6-mCherry-GECO1.0 was used to measure Ca2+ after SHh stimulation. Ciliary Ca2+ was augmented after SHh activation but cAMP levels were reduced, supporting the hypothesis of Ca2+-dependent inhibition of AC5/6 in the cilium. In summary, Moore et al. (2016), were able to show ciliary and cytosolic differences in cAMP signalling, whereby in the cilium, cAMP was upregulated not through GPCR but via PIP3 signalling, and, in contrast to Lesiak et al. and Jiang et al., downregulated through SHh. Moore’s findings do, however, suggest a ciliary cAMP signalosome whereby AC5/6 are regulated by Ca2+ rather than Gαs. Such a paradigm is consistent with Choi et al. (2011) findings, where Polycystin regulation of Ca2+ signalling modulates AC function at AKAP150 to regulate downstream ciliary cAMP.
cAMP can diffuse freely through the TZ, so it is no surprise that its level ultimately equilibrates in the cilium and cytosol. However, the cAMP pathway does rely on the tight spatio-temporal compartmentalisation of its signal, and far less-isolated cAMP subdomains than the primary cilium have been defined (Surdo et al., 2017; Anton et al., 2022; Subramaniam et al., 2023). For example, at the plasma membrane of cardiac myocytes adrenergic signalling activates PKA to phosphorylate several downstream substrates such as Phospholamban (PLB), Troponin I (TnI), and β2AR, resulting in increased strength of contraction. At the same membrane, Prostaglandin E1 (PGE1) stimulation leads to cytosolic elevation of cAMP with no effect on downstream PKA phosphorylation of these targets or increase in ventricular force generation (Hayes et al., 1980; Di Benedetto et al., 2008). Due to the high surface to volume ratio, one would expect to see in the cilium a higher concentration of cAMP than cytosolic, and Moore et al. have indeed reported increased PKA activity at the cilium (Moore et al., 2016; Arena and Hofer, 2021). The inability to detect differences in cAMP levels between the two compartments may depend on the properties of the cAMP reporters used. cAMP in cells diffuses at a speed in the range of 136–780 µm2/s (Agarwal et al., 2016) and the average cilium length is 3–8 µm. Even assuming the slowest cAMP diffusion rate, the highest acquisition rates achievable with FRET imaging while limiting bleaching of the fluorescent signal (typically one image every 3–5 s) may still be too slow to detect swift diffusion of cAMP through the primary cilium. An alternative explanation is that Arl13B may not be the best anchoring protein for detection of compartmentalised ciliary cAMP, as Arl13B localisation in the cilium might be too far away from relevant ciliary cAMP signalosomes, which may be as small as few tens of nanometres (Surdo et al., 2017; Anton et al., 2022).
Photo-activatable adenylyl cyclases (bPACs)
As mentioned above, targeting of FRET reporters to the cilium via fusion to GPCR has proven challenging. Alternative ciliary-targeting strategies, such as use of ciliary AKAPs, may overcome current limitations, although the identity of these proteins remains largely elusive. Other tools have also been developed, such as photo-activatable ACs (bPACs) as well as Designer Receptors Exclusively Activated by Designer Drugs (DREADDs) and a recent series of elegant experiments used these reagents in combination with FRET imaging to manipulate cAMP production in a more targeted manner.
A ground-breaking study carried out by Truong et al. (2021) used ciliary-bPACs (Stierl et al., 2011) to exclusively generate cAMP within the primary cilium, and showed that this cAMP goes on to strictly affect ciliary, but not cytosolic PKA. Ciliary PKA then exerts different effects on SHh signaling than PKA activated by cytosolic cAMP. Cilium-bPACs were expressed in developing zebrafish embryos and activated using pulsed light to specifically synthesise cAMP at the cilium and not in the cell body. Activation of ciliary bPACs led to somite geometry and neural tube anomalies in the embryos, reminiscent of aberrant SHh signalling. When bPACs localising to the cytosol (cyto-bPAC) were activated, an increase in cAMP was still observed, but embryos did not show developmental defects. Gli:mCherry, which induces mCherry expression upon SHh signal activation, was used alongside cilia-bPAC or cyto-bPAC to confirm that the developmental defects observed upon ciliary cAMP stimulation were indeed SHh related. Only ciliary-bPAC, when exposed to light, attenuated the SHh response. SHh gene expression was also altered via cilia-derived cAMP, but not via cytosolic surges in cAMP (Truong et al., 2021).
Truong et al. (2021) also asked whether basal cAMP levels differed between the two cellular compartments. The cAMP indicator, Pink Flamindo, becomes more fluorescent upon binding to cAMP. (Harada et al., 2017). To test whether cAMP diffuses through the TZ, ciliary Pink Flamindo was used to measure cAMP changes in the cilium upon cyto-bPAC activation. Cyto-bPAC activation enhanced cAMP levels in the cilium, however, surprisingly, not to the same extent as when ciliary-bPACs were triggered. These findings suggest that cAMP can diffuse from the cytosol to the cilium, but cytosolic cAMP does not match the levels of local cAMP production in the organelle. A possible explanation for such observations is that when cytosolic cAMP is stimulated, the second messenger can diffuse from the cell body to the cilioplasm. However, when both ciliary and cytosolic receptors are activated, or if only ciliary GPCRs are stimulated, then the primary cilium generates a significantly concentrated pool of cAMP, with excess second messenger spilling into the cytosol.
DREADDs are designer Gαs -coupled receptors which are strictly activated by cognate designer drugs such as clozapine-N-oxide (CNO). Truong et al., used DREADDs to test their hypothesis that ciliary GPCRs regulate SHh signalling and not plasma membrane receptors. Arl13B was used to localise DREADDs to the cilium and the complex tagged with GFP. Activation of cilia-DREADD with CNO, in the presence of SAG, inhibited Gli1 expression, but no attenuation of Gli1 was observed when cytosolic-DREADD was stimulated in the same fashion in NIH/3T3. These results suggest ciliary activation of GPCRs is responsible for regulation of SHh signalling, although they contradict findings by Moore et al. (2016) showing that Gαs signalling does not control the SHh pathway.
Truong et al. (2021) also asked whether the ciliary cAMP signal was transmitted through ciliary or cytosolic PKA. Dominant-negative PKA (dnPKA), with no catalytic function, was targeted to either the basal body, the cilium or the cytosol. Cells expressing Gli:mCherry and one of the three dnPKAs demonstrated that only ciliary-dnPKA increased levels of SHh signalling and only ciliary-dnPKA altered levels of the transcription factor Engrailed (EN), which regulates somite patterning (Barresi et al., 2001). These studies support the presence of a fully independent ciliary cAMP signalosome that includes GPCRs, ACs, and PKA and that regulates SHh transcription.
Ciliary cAMP drives cystogenesis
The optogenetic approaches described above are powerful tools and promise to provide novel insight that can be exploited for the treatment of ciliopathies. In a recent study, optogenetics was used in combination with RNA sequencing to better characterise the role of ciliary-cAMP signaling in ADPKD. With the aid of ciliary and cytosolic bPACs Hansen et al., 2022 corroborate the previous finding that the primary cilium is a cAMP signaling domain distinct from the cytosol. To address whether ciliary cAMP drives cystogenesis, the group generated a mouse IMCD3 cell line stably expressing ciliary or cytosolic bPAC. When these cells were stimulated with either the universal AC activator, forskolin, or PGE2 in a 3D in vitro culture system to emulate the in vivo cyst environment, only photo-activation of ciliary-bPAC, and not photo-activation of cytosolic-bPAC led to cyst formation (Figure 5). (Elberg et al., 2012) The group went on to show that ciliary cAMP signalling engages the mTOR pathway to increase cell proliferation, which in turn drives cystogenesis. If a cilium-confined pool of cAMP is the trigger for cyst formation, then selective manipulation of this local pool of second messenger, perhaps via activation of PDEs, may provide a viable strategy to control cyst growth with potentially significantly limited side effects. Isoforms of the PDE4C family had previously been shown to localise to primary cilia and to associate with the PC1/PC2 complex as well as AC5/6, (Choi et al., 2011), and PDE4 inhibition had been shown to be sufficient to induce cystogenesis in MDCK cells as well as primary cells derived from ADPKD patients (Omar et al., 2019). Consistently, inhibition of PDE4 in cells expressing ciliary or cytosolic bPAC IMCD3 induced cyst growth in cilia-bPAC cells in the dark, and exacerbated cyst formation when cells were exposed to light (Hansen et al., 2022). Treatment with a small-molecule activator of PDE4 long-forms indeed abrogated cystogenesis. RNA sequencing of cilia-bPAC versus cytosolic-bPAC stimulated cells revealed that signal transduction, GPCR signalling, metabolism and gene expression pathways were all upregulated selectively in the ciliary-bPAC stimulated cells and CREB was found to be a prominent transcription factor upregulated upon cilia-bPAC stimulation, indicating that the ciliary cAMP signal maintains specificity of downstream target activation (Hansen et al., 2022). Together, these findings suggest ADPKD is driven specifically by excess ciliary cAMP signalling. Additionally, Hansen et al. highlight the role of PDE4 as the main hydrolyser of cAMP in the organelle. The prominent role of PDE4 in the hydrolysis of cAMP in the cilium suggest that pharmacological activation of PDE4 long-form isoforms could be a therapeutic strategy for ADPKD Other PDEs may be involved in the regulation of cAMP at locations that contribute to the pathogenesis of ADPKD. An alternative and more selective approach to pharmacological manipulation of PDEs activity would be to interfere with the interaction of selected relevant PDE isoforms with proteins that anchor the PDE within cAMP signalosomes, including those within the cilium, to achieve a change in cAMP levels only at that specific location and a more targeted functional effect.
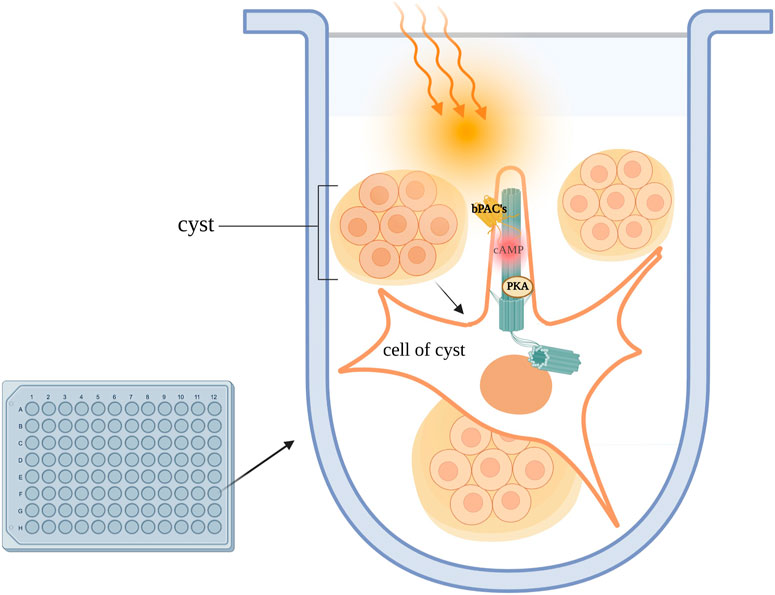
FIGURE 5. Kidney cells are plated in a 3D culture Matrigel/collagen mix and exposed to light, activating ciliary-bPACs. Enhanced ciliary cAMP synthesis due to AC stimulation leads to activation of ciliary PKA. Ciliary PKA phosphorylates downstream targets which drive cystogenesis in in-vitro models of PKD.
Conclusion
FRET imaging using cilia-targeted reporters alongside the application of optogenetic tools provides strong support to the notion that the cilium can handle cAMP independently of the cytosol. Furthermore, many examples have highlighted how ciliary localization of GPCRs leads to a distinct cAMP response compared to stimulation of the same GPCRs when it is localized to the cell body plasma membrane. These findings indicate that the cilium is a vital center for signaling control, and suggest it may be possible to manipulate selectively cAMP within the cilium to treat ciliopathies such as ADPKD with subcellular precision. However, many questions about the regulation and role of ciliary cAMP remain unanswered. For example, how many cAMP signalosomes exist in the primary cilium and how is their activity coordinated? Why do GPCRs localized to the cilium behave differently when localized to other parts of the plasma membrane? What PDEs control ciliary cAMP from diffusing into the cell body, and could PDE isoforms exclusively localized to the cilium be directly targeted for precision medicine? Could ciliary cAMP signaling be altered without disturbing cytosolic cAMP? With the help of current tools and development of future technology, the full characterization of ciliary cAMP signaling will be possible, with the potential of revealing new insights into the many functions of this mysterious organelle.
Author contributions
The review was written by EP and edited by MZ.
Funding
MZ is supported by the British Heart Foundation (RG/17/6/32944 and RE/18/3/34214) and EP is supported by Biotechnology and Biological Sciences Research Council (BBSRC) UKRI.
Conflict of interest
The authors declare that the research was conducted in the absence of any commercial or financial relationships that could be construed as a potential conflict of interest.
Publisher’s note
All claims expressed in this article are solely those of the authors and do not necessarily represent those of their affiliated organizations, or those of the publisher, the editors and the reviewers. Any product that may be evaluated in this article, or claim that may be made by its manufacturer, is not guaranteed or endorsed by the publisher.
References
Abdul-Majeed, S., Moloney, B. C., and Nauli, S. M. (2012). 'Mechanisms regulating cilia growth and cilia function in endothelial cells. Cell Mol. Life Sci. 69, 165–173. doi:10.1007/s00018-011-0744-0
Agarwal, S. R., Clancy, C. E., and Harvey, R. D. (2016). 'Mechanisms restricting diffusion of intracellular cAMP. Sci. Rep. 6, 19577. doi:10.1038/srep19577
Anton, S. E., Kayser, C., Maiellaro, I., Nemec, K., Möller, J., Koschinski, A., et al. (2022). 'Receptor-associated independent cAMP nanodomains mediate spatiotemporal specificity of GPCR signaling. Cell 185, 1130–1142.e11. doi:10.1016/j.cell.2022.02.011
Arena, D. T., and Hofer, A. M. (2021). 'Cyclic AMP does double duty to support parallel signaling in primary cilium and cytosol. Cell Calcium 97, 102436. doi:10.1016/j.ceca.2021.102436
Bachmann, V. A., Mayrhofer, J. E., Ilouz, R., Tschaikner, P., Raffeiner, P., Röck, R., et al. (2016). 'Gpr161 anchoring of PKA consolidates GPCR and cAMP signaling. Proc. Natl. Acad. Sci. U. S. A. 113, 7786–7791. doi:10.1073/pnas.1608061113
Bangs, F., and Anderson, K. V. (2017). 'Primary cilia and mammalian hedgehog signaling. Cold Spring Harb. Perspect. Biol. 9, a028175. doi:10.1101/cshperspect.a028175
Barresi, M. J., D'Angelo, J. A., Hernández, L. P., and Devoto, S. H. (2001). 'Distinct mechanisms regulate slow-muscle development. Curr. Biol. 11, 1432–1438. doi:10.1016/s0960-9822(01)00428-6
Bender, A. T., and Beavo, J. A. (2006). 'Cyclic nucleotide phosphodiesterases: Molecular regulation to clinical use. Pharmacol. Rev. 58, 488–520. doi:10.1124/pr.58.3.5
Brand, T. (2005). The Popeye domain-containing gene family. Cell Biochem. Biophys. 43, 95–103. doi:10.1385/CBB:43:1:095
Bujakowska, K. M., Liu, Q., and Pierce, E. A. (2017). 'Photoreceptor cilia and retinal ciliopathies. Cold Spring Harb. Perspect. Biol. 9, a028274. doi:10.1101/cshperspect.a028274
Cassola, A. C., Giebisch, G., and Wang, W. (1993). 'Vasopressin increases density of apical low-conductance K+ channels in rat CCD. Am. J. physiology 264, F502–F509. doi:10.1152/ajprenal.1993.264.3.F502
Chao, Y. C., Surdo, N. C., Pantano, S., and Zaccolo, M. (2019). 'Imaging cAMP nanodomains in the heart. Biochem. Soc. Trans. 47, 1383–1392. doi:10.1042/BST20190245
Chaumont-Dubel, S., Dupuy, V., Bockaert, J., Bécamel, C., and Marin, P. (2020). The 5-HT(6) receptor interactome: New insight in receptor signaling and its impact on brain physiology and pathologies. Neuropharmacology 172, 107839. doi:10.1016/j.neuropharm.2019.107839
Chiang, C., Litingtung, Y., Lee, E., Young, K. E., Corden, J. L., Westphal, H., et al. (1996). 'Cyclopia and defective axial patterning in mice lacking Sonic hedgehog gene function. Nature 383, 407–413. doi:10.1038/383407a0
Chien, C. L., Wu, Y. S., Lai, H. L., Chen, Y. H., Jiang, S. T., Shih, C. M., et al. (2010). Impaired water reabsorption in mice deficient in the type VI adenylyl cyclase (AC6). FEBS Lett. 584, 2883–2890. doi:10.1016/j.febslet.2010.05.004
Choi, Y. H., Suzuki, A., Hajarnis, S., Ma, Z., Chapin, H. C., Caplan, M. J., et al. (2011). 'Polycystin-2 and phosphodiesterase 4C are components of a ciliary A-kinase anchoring protein complex that is disrupted in cystic kidney diseases. Proc. Natl. Acad. Sci. U. S. A. 108, 10679–10684. doi:10.1073/pnas.1016214108
Colledge, M., and Scott, J. D. (1999). 'AKAPs: From structure to function. Trends Cell Biol. 9, 216–221. doi:10.1016/s0962-8924(99)01558-5
Conduit, S. E., and Vanhaesebroeck., B. (2020). 'Phosphoinositide lipids in primary cilia biology. Biochem. J. 477, 3541–3565. doi:10.1042/BCJ20200277
Conti, M., Kasson, B. G., and Hsueh, A. J. (1984). 'Hormonal regulation of 3',5'-adenosine monophosphate phosphodiesterases in cultured rat granulosa cells. Endocrinology 114, 2361–2368. doi:10.1210/endo-114-6-2361
Corbin, J. D., and Keely, S. L. (1977). 'Characterization and regulation of heart adenosine 3':5'-monophosphate-dependent protein kinase isozymes. J. Biol. Chem. 252, 910–918. doi:10.1016/s0021-9258(19)75184-9
Corbit, K. C., Aanstad, P., Veena, S., Norman, A. R., Stainier, D. Y. R., and Reiter, J. F. (2005). Vertebrate Smoothened functions at the primary cilium. Nature 437, 1018–1021. doi:10.1038/nature04117
de Rooij, J., Zwartkruis, F. J., Verheijen, M. H., Cool, R. H., Nijman, S. M., Wittinghofer, A., et al. (1998). 'Epac is a Rap1 guanine-nucleotide-exchange factor directly activated by cyclic AMP. Nature 396, 474–477. doi:10.1038/24884
Delling, M., DeCaen, P. G., Doerner, J. F., Febvay, S., and Clapham, D. E. (2013). 'Primary cilia are specialized calcium signalling organelles. Nature 504, 311–314. doi:10.1038/nature12833
Depry, C., and Zhang, J. (2011). 'Using FRET-based reporters to visualize subcellular dynamics of protein kinase A activity. Methods Mol. Biol. 756, 285–294. doi:10.1007/978-1-61779-160-4_16
Dessauer, C. W., Watts, V. J., Ostrom, R. S., Conti, M., Dove, S., and Seifert, R. (2017). 'International union of basic and clinical pharmacology. CI. Structures and small molecule modulators of mammalian adenylyl cyclases. Pharmacol. Rev. 69, 93–139. doi:10.1124/pr.116.013078
Di Benedetto, G., Zoccarato, A., Lissandron, V., Terrin, A., Li, X., Houslay, M. D., et al. (2008). 'Protein kinase A type I and type II define distinct intracellular signaling compartments. Circ. Res. 103, 836–844. doi:10.1161/CIRCRESAHA.108.174813
Diskar, M., Zenn, H-M., Kaupisch, A., Kaufholz, M., Brockmeyer, S., Sohmen, D., et al. (2010). 'Regulation of cAMP-dependent protein kinases: The human protein kinase X (PrKX) reveals the role of the catalytic subunit alphaH-alphaI loop. J. Biol. Chem. 285, 35910–35918. doi:10.1074/jbc.M110.155150
Doroquez, D. B., Berciu, C., Anderson, J. R., Sengupta, P., and Nicastro, D. (2014). 'A high-resolution morphological and ultrastructural map of anterior sensory cilia and glia in Caenorhabditis elegans. Elife 3, e01948. doi:10.7554/eLife.01948
Duldulao, N. A., Lee, S., and Sun., Z. (2009). Cilia localization is essential for in vivo functions of the Joubert syndrome protein Arl13b/Scorpion. Development 136, 4033–4042. (Cambridge, England). doi:10.1242/dev.036350
Elberg, D., Turman, M. A., Pullen, N., and Elberg, G. (2012). Prostaglandin E2 stimulates cystogenesis through EP4 receptor in IMCD-3 cells. Prostagl. Other Lipid Mediat 98, 11–16. doi:10.1016/j.prostaglandins.2012.03.005
Fesenko, E. E., Kolesnikov, S. S., and Lyubarsky, A. L. (1985). 'Induction by cyclic GMP of cationic conductance in plasma membrane of retinal rod outer segment. Nature 313, 310–313. doi:10.1038/313310a0
Francis, S. H., Blount, M. A., and Corbin, J. D. (2011). 'Mammalian cyclic nucleotide phosphodiesterases: Molecular mechanisms and physiological functions. Physiol. Rev. 91, 651–690. doi:10.1152/physrev.00030.2010
Hagena, H., Hansen, N., and Manahan-Vaughan, D. (2016). 'β-Adrenergic control of hippocampal function: Subserving the choreography of synaptic information storage and memory. Cereb. Cortex 26, 1349–1364. doi:10.1093/cercor/bhv330
Hansen, J. N., Kaiser, F., Leyendecker, P., Stüven, B., Krause, J. H., Derakhshandeh, F., et al. (2022). 'A cAMP signalosome in primary cilia drives gene expression and kidney cyst formation. EMBO Rep. 23, e54315. doi:10.15252/embr.202154315
Harada, K., Ito, M., Wang, X., Tanaka, M., Wongso, D., Konno, A., et al. (2017). 'Red fluorescent protein-based cAMP indicator applicable to optogenetics and in vivo imaging. Sci. Rep. 7, 7351. doi:10.1038/s41598-017-07820-6
Haycraft, C. J., Banizs, B., Aydin-Son, Y., Zhang, Q., Michaud, E. J., and Yoder, B. K. (2005). 'Gli2 and Gli3 localize to cilia and require the intraflagellar transport protein polaris for processing and function. PLoS Genet. 1, e53. doi:10.1371/journal.pgen.0010053
Hayes, J. S., Brunton, L. L., and Mayer, S. E. (1980). Selective activation of particulate cAMP-dependent protein kinase by isoproterenol and prostaglandin E1. J. Biol. Chem. 255, 5113–5119. doi:10.1016/s0021-9258(19)70757-1
Hildebrandt, F., Benzing, T., and Katsanis, N. (2011). Ciliopathies. N. Engl. J. Med. 364, 1533–1543. doi:10.1056/NEJMra1010172
Hilgendorf, K. I., Johnson, C. T., and Jackson, P. K. (2016). 'The primary cilium as a cellular receiver: Organizing ciliary GPCR signaling. Curr. Opin. Cell Biol. 39, 84–92. doi:10.1016/j.ceb.2016.02.008
Ho, E. K., and Stearns, T. (2021). Hedgehog signaling and the primary cilium: Implications for spatial and temporal constraints on signaling. Development 148, dev195552. (Cambridge, England). doi:10.1242/dev.195552
Huangfu, D., Liu, A., Rakeman, A. S., Murcia, N. S., Niswander, L., and Anderson, K. V. (2003). 'Hedgehog signalling in the mouse requires intraflagellar transport proteins. Nature 426, 83–87. doi:10.1038/nature02061
Ingebritsen, T. S., and Cohen, P. (1983). 'Protein phosphatases: Properties and role in cellular regulation. Science 221, 331–338. doi:10.1126/science.6306765
Jiang, J. Y., Falcone, J. L., Curci, S., and Hofer, A. M. (2019). 'Direct visualization of cAMP signaling in primary cilia reveals up-regulation of ciliary GPCR activity following Hedgehog activation. Proc. Natl. Acad. Sci. U. S. A. 116, 12066–12071. doi:10.1073/pnas.1819730116
Jin, D., and Zhong, T. P. (2022). 'Prostaglandin signaling in ciliogenesis and development. J. Cell Physiol. 237, 2632–2643. doi:10.1002/jcp.30659
Jurevicius, J., and Fischmeister, R. (1996). cAMP compartmentation is responsible for a local activation of cardiac Ca2+ channels by beta-adrenergic agonists. Proc. Natl. Acad. Sci. 93, 295–299. doi:10.1073/pnas.93.1.295
Keitel, V., and Häussinger, D. (2011). 'TGR5 in the biliary tree. Dig. Dis. 29, 45–47. doi:10.1159/000324127
Keitel, V., and Häussinger, D. (2012). Perspective: TGR5 (Gpbar-1) in liver physiology and disease. Clin. Res. Hepatol. Gastroenterol. 36, 412–419. doi:10.1016/j.clinre.2012.03.008
Kiesel, P., Alvarez Viar, G., Tsoy, N., Maraspini, R., Gorilak, P., Varga, V., et al. (2020). 'The molecular structure of mammalian primary cilia revealed by cryo-electron tomography. Nat. Struct. Mol. Biol. 27, 1115–1124. doi:10.1038/s41594-020-0507-4
Kim, C., Cecilia, Y., Cheng, S., Saldanha, A., and Taylor, S. S. (2007). 'PKA-I holoenzyme structure reveals a mechanism for cAMP-dependent activation. Cell 130, 1032–1043. doi:10.1016/j.cell.2007.07.018
Kim, J., Krishnaswami, S. R., and Gleeson, J. G. (2008). 'CEP290 interacts with the centriolar satellite component PCM-1 and is required for Rab8 localization to the primary cilium. Hum. Mol. Genet. 17, 3796–3805. doi:10.1093/hmg/ddn277
Koemeter-Cox, A. I., Sherwood, T. W., Green, J. A., Steiner, R. A., Berbari, N. F., Mykytyn, K., et al. (2014). Primary cilia enhance kisspeptin receptor signaling on gonadotropin-releasing hormone neurons. Proc. Natl. Acad. Sci. U. S. A. 111, 10335–10340. doi:10.1073/pnas.1403286111
Langeberg, L. K., and Scott, J. D. (2015). 'Signalling scaffolds and local organization of cellular behaviour. Nat. Rev. Mol. Cell Biol. 16, 232–244. doi:10.1038/nrm3966
Lesiak, A. J., Brodsky, M., Cohenca, N., Croicu, A. G., and Neumaier, J. F. (2018). Restoration of physiological expression of 5-HT(6) receptor into the primary cilia of null mutant neurons lengthens both primary cilia and dendrites. Mol. Pharmacol. 94, 731–742. doi:10.1124/mol.117.111583
Levitzki, A. (1988). From epinephrine to cyclic AMP. Science 241, 800–806. doi:10.1126/science.2841758
Loktev, A. V., and Jackson, P. K. (2013). 'Neuropeptide Y family receptors traffic via the Bardet-Biedl syndrome pathway to signal in neuronal primary cilia. Cell Rep. 5, 1316–1329. doi:10.1016/j.celrep.2013.11.011
Lubrano-Berthelier, C., Dubern, B., Lacorte, J. M., Picard, F., Shapiro, A., Zhang, S., et al. (2006). 'Melanocortin 4 receptor mutations in a large cohort of severely obese adults: Prevalence, functional classification, genotype-phenotype relationship, and lack of association with binge eating. J. Clin. Endocrinol. Metab. 91, 1811–1818. doi:10.1210/jc.2005-1411
Lugnier, C. (2006). 'Cyclic nucleotide phosphodiesterase (PDE) superfamily: A new target for the development of specific therapeutic agents. Pharmacol. Ther. 109, 366–398. doi:10.1016/j.pharmthera.2005.07.003
Marchmont, R. J., and Houslay, M. D. (1980). 'Insulin trigger, cyclic AMP-dependent activation and phosphorylation of a plasma membrane cyclic AMP phosphodiesterase. Nature 286, 904–906. doi:10.1038/286904a0
Masyuk, A. I., Gradilone, S. A., Banales, J. M., Huang, B. Q., Masyuk, T. V., Larusso, N. F., et al. (2008). 'Cholangiocyte primary cilia are chemosensory organelles that detect biliary nucleotides via P2Y12 purinergic receptors. Am. J. physiology. Gastrointest. liver physiology 295, G725–G734. doi:10.1152/ajpgi.90265.2008
Masyuk, A. I., Huang, B. Q., Radtke, B. N., Gajdos, G. B., Splinter, P. L., Masyuk, T. V., et al. (2013). Ciliary subcellular localization of TGR5 determines the cholangiocyte functional response to bile acid signaling. Am. J. physiology. Gastrointest. liver physiology 304, G1013–G1024. doi:10.1152/ajpgi.00383.2012
Masyuk, A. I., Masyuk, T. V., Splinter, P. L., Huang, B. Q., Stroope, A. J., and LaRusso, N. F. (2006). 'Cholangiocyte cilia detect changes in luminal fluid flow and transmit them into intracellular Ca2+ and cAMP signaling. Gastroenterology 131, 911–920. doi:10.1053/j.gastro.2006.07.003
Masyuk, T. V., Masyuk, A. I., and LaRusso, N. F. (2015). 'TGR5 in the cholangiociliopathies. Dig. Dis. 33, 420–425. doi:10.1159/000371696
Meijer, E., Boertien, W. E., Zietse, R., and Gansevoort, R. T. (2011). 'Potential deleterious effects of vasopressin in chronic kidney disease and particularly autosomal dominant polycystic kidney disease. Kidney Blood Press Res. 34, 235–244. doi:10.1159/000326902
Mongillo, M., McSorley, T., Evellin, S., Sood, A., Lissandron, V., Terrin, A., et al. (2004). 'Fluorescence resonance energy transfer-based analysis of cAMP dynamics in live neonatal rat cardiac myocytes reveals distinct functions of compartmentalized phosphodiesterases. Circ. Res. 95, 67–75. doi:10.1161/01.RES.0000134629.84732.11
Mons, N., Decorte, L., Jaffard, R., and Cooper, D. M. (1998). 'Ca2+-sensitive adenylyl cyclases, key integrators of cellular signalling. Life Sci. 62, 1647–1652. doi:10.1016/s0024-3205(98)00122-2
Monterisi, S., and Zaccolo, M. (2017). 'Components of the mitochondrial cAMP signalosome. Biochem. Soc. Trans. 45, 269–274. doi:10.1042/BST20160394
Moore, B. S., Stepanchick, A. N., Tewson, P. H., Hartle, C. M., Zhang, J., Quinn, A. M., et al. (2016). Cilia have high cAMP levels that are inhibited by Sonic Hedgehog-regulated calcium dynamics. Proc. Natl. Acad. Sci. U. S. A. 113, 13069–13074. doi:10.1073/pnas.1602393113
Mukhopadhyay, S., Wen, X., Ratti, N., Loktev, A., Rangell, L., Scales, S. J., et al. (2013). The ciliary G-protein-coupled receptor Gpr161 negatively regulates the Sonic hedgehog pathway via cAMP signaling. Cell 152, 210–223. doi:10.1016/j.cell.2012.12.026
Omar, F., Findlay, J. E., Carfray, G., Allcock, R. W., Jiang, Z., Moore, C., et al. (2019). 'Small-molecule allosteric activators of PDE4 long form cyclic AMP phosphodiesterases. Proc. Natl. Acad. Sci. U. S. A. 116, 13320–13329. doi:10.1073/pnas.1822113116
Pal, K., Hwang, S. H., Somatilaka, B., Badgandi, H., Jackson, P. K., DeFea, K., et al. (2016). Smoothened determines β-arrestin-mediated removal of the G protein-coupled receptor Gpr161 from the primary cilium. J. Cell Biol. 212, 861–875. doi:10.1083/jcb.201506132
Patel, Y. C. (1999). Somatostatin and its receptor family. Front. Neuroendocrinol. 20, 157–198. doi:10.1006/frne.1999.0183
Porpora, M., Sauchella, S., Rinaldi, L., Delle Donne, R., Sepe, M., Torres-Quesada, O., et al. (2018). 'Counterregulation of cAMP-directed kinase activities controls ciliogenesis. Nat. Commun. 9, 1224. doi:10.1038/s41467-018-03643-9
Raychowdhury, M. K., Ramos, A. J., Zhang, P., McLaughin, M., Dai, X. Q., Chen, X. Z., et al. (2009). 'Vasopressin receptor-mediated functional signaling pathway in primary cilia of renal epithelial cells. Am. J. Physiol. Ren. Physiol. 296, F87–F97. doi:10.1152/ajprenal.90509.2008
Rees, S., Kittikulsuth, W., Roos, K., Strait, K. A., Van Hoek, A., and Kohan, D. E. (2014). Adenylyl cyclase 6 deficiency ameliorates polycystic kidney disease. J. Am. Soc. Nephrol. 25, 232–237. doi:10.1681/ASN.2013010077
Reif, G. A., Yamaguchi, T., Nivens, E., Fujiki, H., Pinto, C. S., and Wallace, D. P. (2011). Tolvaptan inhibits ERK-dependent cell proliferation, Cl⁻ secretion, and in vitro cyst growth of human ADPKD cells stimulated by vasopressin. Am. J. Physiol. Ren. Physiol. 301, F1005–F1013. doi:10.1152/ajprenal.00243.2011
Reiter, J. F., Blacque, O. E., and Leroux, M. R. (2012). The base of the cilium: Roles for transition fibres and the transition zone in ciliary formation, maintenance and compartmentalization. EMBO Rep. 13, 608–618. doi:10.1038/embor.2012.73
Rinschen, M. M., Schermer, B., and Thomas, B. (2014). 'Vasopressin-2 receptor signaling and autosomal dominant polycystic kidney disease: From bench to bedside and back again. J. Am. Soc. Nephrol. JASN 25, 1140–1147. doi:10.1681/ASN.2013101037
Rodbell, M., Birnbaumer, L., Pohl, S. L., and Krans, H. M. (1971). The glucagon-sensitive adenyl cyclase system in plasma membranes of rat liver. J. Biol. Chem. 246, 1877–1882. doi:10.1016/s0021-9258(18)62390-7
Sánchez, I., and Dynlacht, B. D. (2016). Cilium assembly and disassembly. Nat. Cell Biol. 18, 711–717. doi:10.1038/ncb3370
Satir, P., and Christensen, S. T. (2007). Overview of structure and function of mammalian cilia. Annu. Rev. Physiol. 69, 377–400. doi:10.1146/annurev.physiol.69.040705.141236
Scarinci, N., Perez, P. L., Cantiello, H. F., and Cantero, M. D. R. (2022). Polycystin-2 (TRPP2) regulates primary cilium length in LLC-PK1 renal epithelial cells. Front. Physiol. 13, 995473. doi:10.3389/fphys.2022.995473
Selbie, L. A., and Hill, S. J. (1998). 'G protein-coupled-receptor cross-talk: The fine-tuning of multiple receptor-signalling pathways. Trends Pharmacol. Sci. 19, 87–93. doi:10.1016/s0165-6147(97)01166-8
Senatore, E., Chiuso, F., Rinaldi, L., Intartaglia, D., Delle Donne, R., Pedone, E., et al. (2021). 'The TBC1D31/praja2 complex controls primary ciliogenesis through PKA-directed OFD1 ubiquitylation. Embo J. 40, e106503. doi:10.15252/embj.2020106503
Shao, L., El-Jouni, W., Kong, F., Ramesh, J., Kumar, R. S., Shen, X., et al. (2020). Genetic reduction of cilium length by targeting intraflagellar transport 88 protein impedes kidney and liver cyst formation in mouse models of autosomal polycystic kidney disease. Kidney Int. 98, 1225–1241. doi:10.1016/j.kint.2020.05.049
Sherpa, R. T., Mohieldin, A. M., Pala, R., Wachten, D., Ostrom, R. S., and Nauli, S. M. (2019). 'Sensory primary cilium is a responsive cAMP microdomain in renal epithelia. Sci. Rep. 9, 6523. doi:10.1038/s41598-019-43002-2
Singh, J., Wen, X., and Scales, S. J. (2015). The orphan G protein-coupled receptor Gpr175 (Tpra40) enhances hedgehog signaling by modulating cAMP levels. J. Biol. Chem. 290, 29663–29675. doi:10.1074/jbc.M115.665810
Smith, F. D., Esseltine, J. L., Nygren, P. J., Veesler, D., Byrne, D. P., Vonderach, M., et al. (2017). Local protein kinase A action proceeds through intact holoenzymes. Science 356, 1288–1293. doi:10.1126/science.aaj1669
Spirli, C., Mariotti, V., Villani, A., Fabris, L., Fiorotto, R., and Strazzabosco, M. (2017). 'Adenylyl cyclase 5 links changes in calcium homeostasis to cAMP-dependent cyst growth in polycystic liver disease. J. Hepatol. 66, 571–580. doi:10.1016/j.jhep.2016.10.032
Stierl, M., Stumpf, P., Udwari, D., Gueta, R., Hagedorn, R., Losi, A., et al. (2011). Light modulation of cellular cAMP by a small bacterial photoactivated adenylyl cyclase, bPAC, of the soil bacterium Beggiatoa. J. Biol. Chem. 286, 1181–1188. doi:10.1074/jbc.M110.185496
Stork, P. J., and Schmitt, J. M. (2002). Crosstalk between cAMP and MAP kinase signaling in the regulation of cell proliferation. Trends Cell Biol. 12, 258–266. doi:10.1016/s0962-8924(02)02294-8
Stoufflet, J., Chaulet, M., Doulazmi, M., Fouquet, C., Dubacq, C., Métin, C., et al. (2020). 'Primary cilium-dependent cAMP/PKA signaling at the centrosome regulates neuronal migration. Sci. Adv. 6, eaba3992. doi:10.1126/sciadv.aba3992
Subramaniam, G., Schleicher, K., Kovanich, D., Anna, Z., Folkmanaite, M., Chao, Y-C., et al. (2023). Integrated proteomics unveils nuclear PDE3A2 as a regulator of cardiac myocyte hypertrophy. Circulation Res. 132, 828–848. doi:10.1161/CIRCRESAHA.122.321448
Surdo, N. C., Berrera, M., Koschinski, A., Brescia, M., Machado, M. R., Carr, C., et al. (2017). FRET biosensor uncovers cAMP nano-domains at β-adrenergic targets that dictate precise tuning of cardiac contractility. Nat. Commun. 8, 15031. doi:10.1038/ncomms15031
Tabibian, J. H., Masyuk, A. I., Masyuk, T. V., O'Hara, S. P., and LaRusso, N. F. (2013). 'Physiology of cholangiocytes. Compr. Physiol. 3, 541–565. doi:10.1002/cphy.c120019
Taylor, S. S., Kim, C., Cheng, C. Y., Brown, S. H., Wu, J., and Kannan, N. (2008). 'Signaling through cAMP and cAMP-dependent protein kinase: Diverse strategies for drug design. Biochim. Biophys. Acta 1784, 16–26. doi:10.1016/j.bbapap.2007.10.002
Taylor, S. S., Radzio-Andzelm, E., Knighton, D. R., Ten Eyck, L. F., Sowadski, J. M., Herberg, F. W., et al. (1993). Crystal structures of the catalytic subunit of cAMP-dependent protein kinase reveal general features of the protein kinase family. Receptor 3, 165–172.
Taylor, S. S., Zhang, P., Steichen, J. M., Keshwani, M. M., and Kornev, A. P. (2013). 'PKA: Lessons learned after twenty years. Biochim. Biophys. Acta 1834, 1271–1278. doi:10.1016/j.bbapap.2013.03.007
Terrin, A., Monterisi, S., Stangherlin, A., Zoccarato, A., Koschinski, A., Surdo, N. C., et al. (2012). 'PKA and PDE4D3 anchoring to AKAP9 provides distinct regulation of cAMP signals at the centrosome. J. Cell Biol. 198, 607–621. doi:10.1083/jcb.201201059
Tewson, P. H., Martinka, S., Shaner, N. C., Hughes, T. E., and Quinn, A. M. (2016). 'New DAG and cAMP sensors optimized for live-cell assays in automated laboratories. J. Biomol. Screen 21, 298–305. doi:10.1177/1087057115618608
Torres-Quesada, O., Mayrhofer, J. E., and Stefan, E. (2017). The many faces of compartmentalized PKA signalosomes. Cell Signal 37, 1–11. doi:10.1016/j.cellsig.2017.05.012
Truong, M. E., Bilekova, S., Choksi, S. P., Li, W., Bugaj, L. J., Xu, K., et al. (2021). Vertebrate cells differentially interpret ciliary and extraciliary cAMP. Cell 184, 2911–2926.e18. doi:10.1016/j.cell.2021.04.002
Tuson, M., Mu, H., and Anderson, K. V. (2011). Protein kinase A acts at the basal body of the primary cilium to prevent Gli2 activation and ventralization of the mouse neural tube. Development 138, 4921–4930. (Cambridge, England). doi:10.1242/dev.070805
Vaisse, C., Clement, K., Durand, E., Hercberg, S., Guy-Grand, B., and Froguel, P. (2000). Melanocortin-4 receptor mutations are a frequent and heterogeneous cause of morbid obesity. J. Clin. investigation 106, 253–262. doi:10.1172/JCI9238
Vaisse, C., Clement, K., Guy-Grand, B., and Froguel, P. (1998). 'A frameshift mutation in human MC4R is associated with a dominant form of obesity. Nat. Genet. 20, 113–114. doi:10.1038/2407
Valente, E. M., Rosti, R. O., Gibbs, E., and Gleeson, J. G. (2014). 'Primary cilia in neurodevelopmental disorders. Nat. Rev. Neurol. 10, 27–36. doi:10.1038/nrneurol.2013.247
Walker-Gray, R., Stengel, F., and Gold, M. G. (2017). Mechanisms for restraining cAMP-dependent protein kinase revealed by subunit quantitation and cross-linking approaches. Proc. Natl. Acad. Sci. U. S. A. 114, 10414–10419. doi:10.1073/pnas.1701782114
Walsh, D. A., Perkins, J. P., and Krebs, E. G. (1968). An adenosine 3',5'-monophosphate-dependant protein kinase from rabbit skeletal muscle. J. Biol. Chem. 243, 3763–3765. doi:10.1016/s0021-9258(19)34204-8
Wang, Q., Cobo-Stark, P., Patel, V., Somlo, S., Han, P. L., and Igarashi, P. (2018). Adenylyl cyclase 5 deficiency reduces renal cyclic AMP and cyst growth in an orthologous mouse model of polycystic kidney disease. Kidney Int. 93, 403–415. doi:10.1016/j.kint.2017.08.005
Wang, Y., Bernard, A., Comblain, F., Yue, X., Paillart, C., Zhang, S., et al. (2021). 'Melanocortin 4 receptor signals at the neuronal primary cilium to control food intake and body weight. J. Clin. Invest. 131, e142064. doi:10.1172/JCI142064
Weissinger, E. M., Eissner, G., Grammer, C., Fackler, S., Haefner, B., Yoon, L. S., et al. (1997). Inhibition of the Raf-1 kinase by cyclic AMP agonists causes apoptosis of v-abl-transformed cells. Mol. Cell Biol. 17, 3229–3241. doi:10.1128/mcb.17.6.3229
Wheway, G., Nazlamova, L., and Hancock, J. T. (2018). Signaling through the primary cilium. Front. Cell Dev. Biol. 6, 8. doi:10.3389/fcell.2018.00008
Wiggins, S. V., Steegborn, C., Levin, L. R., and Buck, J. (2018). 'Pharmacological modulation of the CO(2)/HCO(3)(-)/pH-calcium-and ATP-sensing soluble adenylyl cyclase. Pharmacol. Ther. 190, 173–186. doi:10.1016/j.pharmthera.2018.05.008
Wong, W., and Scott, J. D. (2004). AKAP signalling complexes: Focal points in space and time. Nat. Rev. Mol. Cell Biol. 5, 959–970. doi:10.1038/nrm1527
Yamamoto, K. K., Gonzalez, G. A., Biggs, W. H., and Montminy, M. R. (1988). 'Phosphorylation-induced binding and transcriptional efficacy of nuclear factor CREB. Nature 334, 494–498. doi:10.1038/334494a0
Yao, G., Luo, C., Harvey, M., Wu, M., Schreiber, T. H., Du, Y., et al. (2016). Disruption of polycystin-L causes hippocampal and thalamocortical hyperexcitability. Hum. Mol. Genet. 25, 448–458. doi:10.1093/hmg/ddv484
Zaccolo, M., Zerio, A., and Lobo, M. J. (2021). Subcellular organization of the cAMP signaling pathway. Pharmacol. Rev. 73, 278–309. doi:10.1124/pharmrev.120.000086
Keywords: primary cilium, cAMP signaling, GPCR (G protein coupled receptor), autosomal dominanat polycystic kidney disease (ADPKD), FRET microscopy
Citation: Paolocci E and Zaccolo M (2023) Compartmentalised cAMP signalling in the primary cilium. Front. Physiol. 14:1187134. doi: 10.3389/fphys.2023.1187134
Received: 15 March 2023; Accepted: 25 April 2023;
Published: 09 May 2023.
Edited by:
Yury Ladilov, Charité University Medicine Berlin, GermanyReviewed by:
Lonny Ray Levin, Cornell University, United StatesLasse Kristoffer Bak, Rigshospitalet, Denmark
Copyright © 2023 Paolocci and Zaccolo. This is an open-access article distributed under the terms of the Creative Commons Attribution License (CC BY). The use, distribution or reproduction in other forums is permitted, provided the original author(s) and the copyright owner(s) are credited and that the original publication in this journal is cited, in accordance with accepted academic practice. No use, distribution or reproduction is permitted which does not comply with these terms.
*Correspondence: Ester Paolocci, ZXN0ZXIucGFvbG9jY2lAd29sZnNvbi5veC5hYy51aw==; Manuela Zaccolo, bWFudWVsYS56YWNjb2xvQGRwYWcub3guYWMudWs=