- Département de Pédiatrie, Research Center of the Québec Heart and Lung Institute, Université Laval, Quebec City, QC, Canada
CO2 is a fundamental component of living matter. This chemical signal requires close monitoring to ensure proper match between metabolic production and elimination by lung ventilation. Besides ventilatory adjustments, CO2 can also trigger innate behavioral and physiological responses associated with fear and escape but the changes in brain CO2/pH required to induce ventilatory adjustments are generally lower than those evoking fear and escape. However, for patients suffering from panic disorder (PD), the thresholds for CO2-evoked hyperventilation, fear and escape are reduced and the magnitude of those reactions are excessive. To explain these clinical observations, Klein proposed the false suffocation alarm hypothesis which states that many spontaneous panics occur when the brain’s suffocation monitor erroneously signals a lack of useful air, thereby maladaptively triggering an evolved suffocation alarm system. After 30 years of basic and clinical research, it is now well established that anomalies in respiratory control (including the CO2 sensing system) are key to PD. Here, we explore how a stress-related affective disorder such as PD can disrupt respiratory control. We discuss rodent models of PD as the concepts emerging from this research has influenced our comprehension of the CO2 chemosensitivity network, especially structure that are not located in the medulla, and how factors such as stress and biological sex modulate its functionality. Thus, elucidating why hormonal fluctuations can lead to excessive responsiveness to CO2 offers a unique opportunity to gain insights into the neuroendocrine mechanisms regulating this key aspect of respiratory control and the pathophysiology of respiratory manifestations of PD.
1 Introduction and overview
As a chemist, Antoine Lavoisier was the first to acknowledge CO2 as a “fundamental component of living matter”. While his significant contributions to modern physiology did not save him from the guillotine, CO2 is now acknowledged as a chemical signal that requires close monitoring to ensure proper match between metabolic production and elimination by lung ventilation. Although accurate, this approach neglects the fact that CO2 accumulation is a sign of an impoverished air quality such that in many species (including rodents and humans), CO2 can trigger innate behavioral and physiological responses associated with fear and escape. These responses are highly adaptive because leaving the room (rather than hyperventilating) is perhaps the simplest (and most efficient) way to deal with a hypercarbic environment! Clearly, the changes in brain CO2/pH required to induce ventilatory adjustments are far lower than those evoking fear and escape (Guyenet and Bayliss, 2022), but in a subpopulation of patients suffering from anxiety disorders, the thresholds for CO2-evoked hyperventilation, fear and escape are reduced and the magnitude of those reactions are excessive. This trait can then initiate a vicious circle: if the increase in breathing is disproportionate, the perception of respiratory efforts along with the excessive CO2 loss (hypocapnia) can trigger a variety of physical sensations ranging from headaches to chest pain/discomfort (Gardner, 1996). Panic disorder (PD) patients misinterpret these physiological signals as life-threatening and experience strong emotional reactions that can lead to full-blown panic attacks encompassing fear of dying, shortness of breath, and a choking sensation (Gardner, 1996; Gorman et al., 2000; Nardi et al., 2009). To explain these clinical observations, Donald Klein proposed “the false suffocation alarm hypothesis” which states that “many spontaneous panics occur when the brain’s suffocation monitor erroneously signals a lack of useful air, thereby maladaptively triggering an evolved suffocation alarm system” (Klein, 1993). This model has been refined (Feinstein et al., 2022; Kinkead et al., 2022), but has generally stood the test of time. After 30 years of basic and clinical research, it is now well established that anomalies in respiratory control (including the CO2 sensing system) are key to PD. Moreover, the intense fear and anxiety experienced by PD patients highlight the functional and anatomical overlaps that exists between the neural circuits that control breathing and those that regulate emotions, fear and escape responses (Schenberg, 2016; Venkatraman et al., 2017). Each of these systems has been studied extensively in isolation, but in recent years, the study of their functional intersection has offered a novel and broader view of respiratory neurobiology. Recent work from Feldman and collaborators has deciphered the pathways by which inspiratory rhythm originating from the pre-Bötzinger complex affects emotions (Ashhad et al., 2022). Here, we will look at this relationship from a different angle; namely, we will explore how a stress-related affective disorder such as PD can influence respiratory control. We focus on rodent models of PD as the concepts emerging from this research has influenced our comprehension of the CO2 chemosensitivity network and how factors such as stress and biological sex modulate its functionality.
2 Panic disorder: Definitions and sex-based differences in respiratory manifestations of neural control dysfunction
Panic disorder is an anxiety disorder characterized by recurrent panic attacks that are acute, unexpected, and that occur without a clear trigger (World-Health-Organization, 1992; American-Psychiatric-Association, 1994). A panic attack is defined as an episode of overwhelming physical distress and cognitive anxiety during which the patient rapidly develops intense symptoms such as air hunger, sweating, heart palpitations, shortness of breath, hyperventilation, and fear of dying (Hoppe et al., 2012). As such, PD is perhaps one of the most overwhelming experiences that a person can endure (Moreira et al., 2013). While the DSM-V definition of PD spans across 13 different symptoms, the respiratory PD subtype has been identified as the most common, the most pervasive, and the most disabling form of PD (Roberson-Nay and Kendler, 2011). This demonstrates the prominence of the respiratory distress symptoms in PD (Wilhelm et al., 2001; Roberson-Nay et al., 2010; Hoppe et al., 2012; Rappaport et al., 2017) and, depending on theoretical standpoints, hyperventilation can thus be viewed as a cause, a correlate, or a consequence of panic attacks (Nardi et al., 2009).
During World War I, it was observed that CO2 rebreathing while wearing a gas mask can bring some soldiers to remove the gas mask and/or induce a panic attack (Ritchie, 1992); it was proposed at the time that soldiers prone to the “irritable heart” are excessively sensitive to this stimulus (Drury, 1918). Today, CO2 inhalation is commonly used as a diagnostic tool for PD (Battaglia and Perna, 1995; Gorman et al., 2001; Hoppe et al., 2012). An increased CO2 response is acknowledged as a distinctive biomarker of this population and remains a central readout of PD that is easily and non-inferentially modeled in the laboratory. Furthermore, PD patients show an abnormally elevated respiratory variability owing to excessive sighing and an increased rate of apnea both during sleep and wakefulness (Stein et al., 1995; Bystritsky et al., 2000; Abelson et al., 2001; Nardi et al., 2009; Garbarino et al., 2020; Feinstein et al., 2022). The sum of these physiological symptoms indicate that both the regulation and perception of breathing are dysfunctional in PD patients (Gorman et al., 2000; Sinha et al., 2000; Katzman et al., 2002; van Duinen et al., 2007; Abelson et al., 2008; Nardi et al., 2009; Abelson et al., 2010; Grassi et al., 2013).
In North America and Europe, PD affects ∼5% of the general population (Hoppe et al., 2012; Meng and D'Arcy, 2012; Bandelow and Michaelis, 2015) and its sexual dimorphism is striking: the prevalence rate of women who have PD or with excessive physiological and behavioral responses to CO2 inhalation is 2–3 times that of men (Wilhelm and Roth, 2001; Pigott, 2003; Donner and Lowry, 2013). The incidence of PD rises at puberty (Reardon et al., 2009) and in young adolescent girls, pubertal stage predicts panic attack occurrence (Hayward et al., 1992). Furthermore, the panicogenic effects of CO2 inhalation are highest during the pre-menstrual phase (Nillni et al., 2017), thus indicating that, by comparison with healthy subjects, women suffering from PD are more sensitive to the sudden drop in ovarian hormones taking place during the last days of the luteal phase (Reardon et al., 2009; Nillni et al., 2011; Nillni et al., 2017). Cyclic fluctuation in ovarian hormones is a normal physiological process, but in a subpopulation of women, they contribute to the onset of PD and its recurrent exacerbations (Reed and Wittchen, 1998; Gorman et al., 2001; Lovick, 2014). Thus, elucidating why hormonal fluctuations can lead to excessive responsiveness to CO2 offers a unique opportunity to gain insights into the neuroendocrine mechanisms regulating this key aspect of respiratory control and the pathophysiology of respiratory manifestations of PD.
3 Early life stress and PD-related respiratory disturbances in rodents and humans
In mammals (including humans), exposure to adversities during early life alters brain development and is a significant risk for disease (Graham et al., 1999; Buitelaar et al., 2003; Fumagalli et al., 2007; Shonkoff et al., 2009; Charil et al., 2010). Conditions such as maternal depression, unstable parental environment, and special medical care at birth that interfere with mother-infant interactions are stressful to the infant. The sum of current data from clinical and basic research indicates that these forms of early life stress predispose to behavioural and cognitive disorders as well as excessive CO2 sensitivity and PD that emerge at adolescence, especially in females (Battaglia et al., 1995; Gunnar, 2003; Battaglia et al., 2009; Shonkoff et al., 2009; D'Amato et al., 2011). Thus, the deleterious impacts of early life stress remain latent and are revealed by the rise in ovarian function that takes place at puberty; subsequent fluctuations of ovarian hormones exacerbate PD-related respiratory symptoms in a cyclic fashion. To gain insight into the basic neuroendocrine mechanisms of PD, repeated cross fostering (RCF) and neonatal maternal separation (NMS) have been use in mice and rats (respectively) as clinically relevant models of early life stress and using the ventilatory response to CO2 as a main physiological outcome (Battaglia et al., 2014).
The hypercapnic ventilatory response (HcVR) changes significantly during development (Putnam et al., 2005; Tenorio-Lopes et al., 2020) and progressive increase in the expression TASK 1 and TASK 2 channels in the hypothalamus likely contribute to this process (Wang et al., 2021); however, the molecular signal initiating their expression remains unknown. In sexually mature mammals (including humans), sex-based differences in the intensity of the CO2 response are well documented but are highly heterogeneous (Gargaglioni et al., 2019). In mice, RCF augments the HcVR of pups (P16-20) and adults mainly by augmenting the tidal volume response; however, this effect is similar in both sexes (D'Amato et al., 2011; Luchetti et al., 2015; Cittaro et al., 2016; Battaglia et al., 2018). In adult C57BL6 mice, NMS elicits a modest increase of the HcVR only in females (Elliot-Portal et al., 2021). In rats, the ventilatory response to 5% CO2 of pre-pubertal rat pups (P14 - P15) is very weak with no evidence of sex- or NMS-related effects (Tenorio-Lopes et al., 2020). At adulthood, the HcVR of control (non-stressed) male and female rats is similar, but the effects of NMS on the CO2 response differ strikingly between sexes in ways that are very similar to clinical observations of PD. Specifically, the minute ventilation response to CO2 inhalation (5% CO2; 10 min) of NMS females is 60%–80% larger than controls and NMS-related increase of the CO2 response is i) sex-specific (limited to females), ii) peaks during proestrus, and iii) is not observed prior to puberty (Genest et al., 2007; Kinkead et al., 2009) (Figure 1). These differences in the developmental and sex-specific effects of early life stress on the HcVR of mice and rats likely reflect inter-species differences in stress responses of rodents (Beery and Kaufer, 2015). Regardless, both models have advanced our comprehension of the pathophysiology of PD. What follows is a summary of the main mechanisms that contribute to stress-related increase in CO2 response.
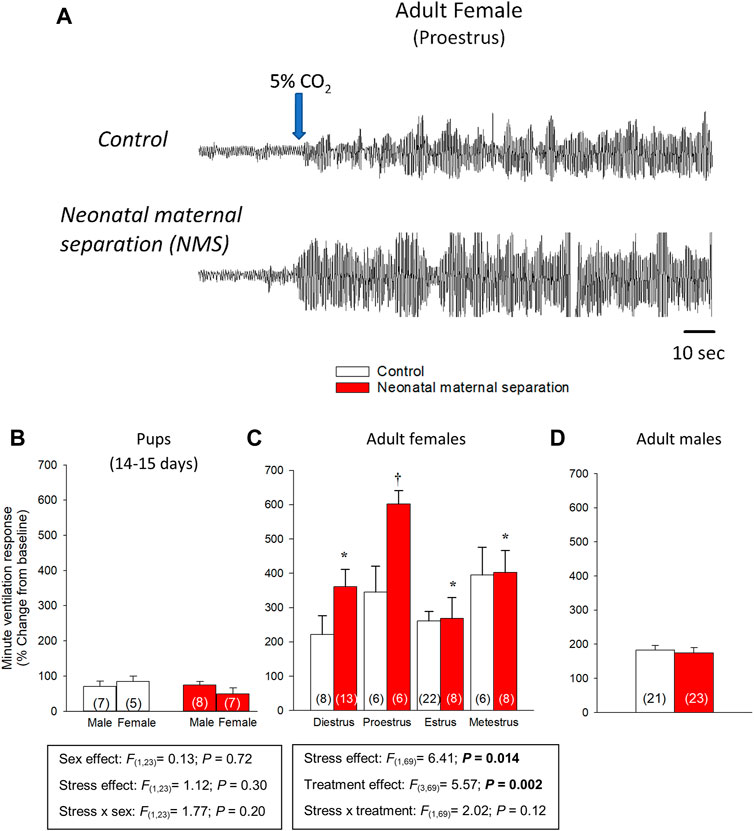
FIGURE 1. Influence of neonatal stress and reproductive status on the magnitude of ventilatory response to CO2 in rats. (A) Original plethysmography recordings comparing ventilatory activity at rest and upon exposure to hypercapnia (5% CO2 in air). Recordings were obtained from adult rats during the proestrus phase; females were either raised under standard conditions (top trace) or subjected to neonatal maternal separation (bottom trace; 3 h/day, postnatal days 3–12). Blue arrow indicates the onset of the exposition to 5% CO2 for 10 min. Comparison of the minute ventilation responses to hypercapnia between control rats (white bars) and rats subjected to neonatal maternal separation (NMS; red bars). Data expressed as percent change from baseline (room air) in (B) pups, adult (C) females and (D) males. Data from males are from (Genest et al., 2007; Tenorio-Lopes et al., 2017); they are reported for comparison and were not included in the statistical analyses. Data are reported as means ± SEM * indicates a value significantly different from corresponding proestrus value at p < 0.05; † indicates a value significantly different from corresponding control value at p < 0.05. Adapted with permission from (Tenorio-Lopes et al., 2020).
4 Early life stress alters non-medullary structures with CO2 sensing properties
4.1 The amygdala
Within the medial temporal lobes, the amygdalar complex is responsible for perception and processing of stimuli; it also initiates and terminates emotional reactions (Marek et al., 2013). Briefly, this complex is composed of three main structures: the medial amygdala (MeA), the central amygdala (CeA) and the basolateral amygdala (BLA) (Figure 2). The basolateral part of the amygdala (BLA) is of great interest to this mini-review because it has inherent CO2 sensing properties; much like “classical” CO2-sensing neurons of the medulla, BLA neurons can detect CO2 (Ziemann et al., 2009). While direct comparisons are not possible, we can estimate that the CO2/H+ sensitivity of BLA neurons is ∼ 10 times less than that of the retrotrapezoid nucleus (RTN), the main CO2 sensing structure in respiratory control (Guyenet et al., 2019). Nonetheless, CO2-induced stimulation of the BLA elicits emotional and physiological responses associated with fear and panic-related states (Ziemann et al., 2009). The BLA interacts with the medial amygdala (MeA) that regulates innate emotional behaviors; it relays olfactory information to hypothalamic nuclei involved in reproduction and defense behaviors. Interestingly, sex-based differences in anatomy, laterality, function, and sensitivity to steroid hormones of the MeA are well documented in humans and rodents (Rodrigues et al., 2009; Cahill, 2010; Goldstein et al., 2010; Edelmann and Auger, 2011; Buss et al., 2012). Both regions project to the central amygdala (CeA) (Keshavarzi et al., 2014), which is the amygdala’s output pathway because it initiates autonomic and respiratory responses (Veening et al., 1984). The CeA projects directly onto rhythmogenic neurons of the pre-Bötzinger complex, the nucleus of the solitary tract (NTS), and the RTN (Petrov et al., 1995; Rosin et al., 2006; Ulrich-Lai and Herman, 2009; Yang et al., 2020). Furthermore, stimulation of the CeA excites the inspiratory cycle (Harper et al., 1984). In our studies using c-fos mRNA as a marker of neuronal activation, the CeA has emerged as an important candidate in the initiation of an excessive ventilatory response to CO2 in NMS, especially in female rats (Kinkead et al., 2014).
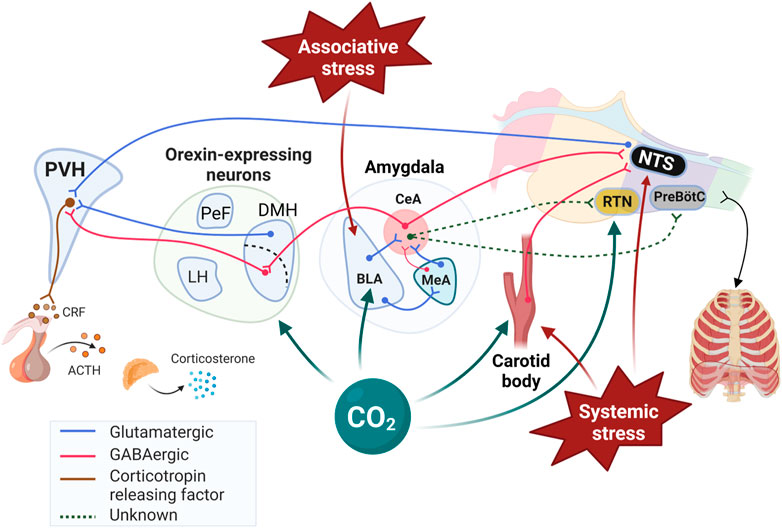
FIGURE 2. Schematic representation of stress-related neuronal inputs and CO2 chemosenstivity in cardiorespiratory control. While retrotrapezoid nucleus (RTN) of the medulla is well established as a highly sensitive to CO2/H+ responsible for fine respiratory adjustments, the Figure 1) highlights other “non-medullary” sites that respond to CO2/H+, 2) identifies the pathways by which they initiate significant cardiorespiratory and behavioral responses, and 3) illustrates how these structures are part of the neural network initiating and regulating the response to stress. Note that the interactions between the PVH and medullary structures regulating breathing are not shown for simplicity. The review by (Zhang et al., 2021) provided valuable informations on network and the neurotransmitters contributing to those interactions. PVH: paraventricular nucleus of the hypothalamus; BLA: Basolateral amygdala; CeA: Central nucleus of the amygdala; MeA: Medial nucleus of the amygdala; ORX; Orexin producing neurons; DMH: Dorsomedial hypothalamic nucleus; PeF: Perifornical area; LH: Lateral hypothalamus; NTS: Nucleus of the solitary tract; CRF: Corticotropin releasing factor; ACTH: Adrenocorticotropic hormone; PreBötC: Pre-Bötzinger complex. Created with BioRender.com.
Owing to its role in the regulation of emotional reactions, the amygdala contributes to the pathogenesis of anxiety (Feinstein et al., 2022); however, observations made on patients with Urbach–Wiethe disease, a rare genetic disorder leading to focal bilateral amygdala lesions, have raised questions concerning its contribution to PD. Briefly, Urbach–Wiethe patients show no fear and avoidance behavior to external threats such as snakes, tarantulas, and a range of traumatic life events, but exhibit a significantly higher rate of CO2-evoked fear and panic than a sample of demographically-matched healthy participants (Feinstein et al., 2013; Feinstein et al., 2022). In mice, electrolytic lesions of the amygdala inhibited fear-like behavior (freezing); however, the ventilatory response was not tested (Taugher et al., 2020). In pediatric subjects, electrical stimulation of the medial subregion of the basal nuclei, cortical and medial nuclei induces apnea (Rhone et al., 2020); similar procedures in the lateral and basolateral amygdala of adults also results in respiratory inhibition (Dlouhy et al., 2015). The fact that these patients do not notice respiratory arrest or report dyspnea indicate that their ability to perceive the rise in CO2 is blunted; the inverse relationship between CO2 activation of the MeA and the HcVR of male rats is in line with this observation (Tenorio-Lopes et al., 2017). Although compelling, this interpretation requires caution because amygdalar lesions in humans and mice were heterogeneous and clinical studies generally involve a limited number of participants. Nonetheless, the sum of these observations i) highlights an important neural distinction between “external” threats conveyed via visual and auditory pathways, versus threats conveyed through “internal” sensory channels (e.g., chemoreceptive); ii) suggests that rather than inducing panic, the amygdala inhibits it, especially when it is evoked by an internal threat such as elevated levels of CO2, and iii) this inhibition likely originates from a subpopulation of CeA neurons considering that as discussed previously, the CeA is generally acknowledged for its stimulatory influence on breathing.
4.2 Orexin neurons
Orexins A and B (ORX; also known as hypocretins) are regulatory peptides produced by neurons located in the dorso-medial, perifornical, and lateral hypothalamus (DMH, PeF, and LH, respectively). Orexin neurons have extensive projections throughout the central nervous system; however, the organisation of this system is dichotomous: LH neurons stimulate motivated behaviors such as appetite for food and other rewards such as abused drugs (Harris and Aston-Jones, 2006), whereas neurons of the PeF and DMH act in parallel to influence arousal, sleep/wake states, and cardiorespiratory function (Johnson et al., 2010; Nattie and Li, 2012; Ciriello et al., 2013; Barnett and Li, 2020). Interestingly, the DMH/PeF region was initially termed the “panic area” because its activation induced a “panic-like state” in experimental animals (DiMicco et al., 2002; Díaz-Casares et al., 2009). Today, a “hyperactive” ORX system is a leading hypothesis in the pathophysiology of PD (Johnson et al., 2010; Abreu et al., 2020). This is based on the fact that ORX levels in the cerebrospinal fluid of PD patients is elevated by comparison with healthy subjects (Johnson et al., 2010) (Figure 3) and in adult rats, previous exposure to early life stress (maternal deprivation/separation) augments ORXA levels in hypothalamus extracts (Feng et al., 2007; Tenorio-Lopes et al., 2020). Furthermore, PD patients show abnormal levels of expression the HCRTR1 gene which encodes for ORX1 receptors (Johnson et al., 2010; Gottschalk et al., 2019).
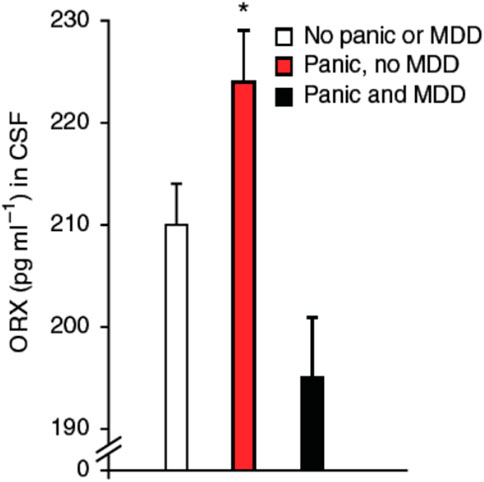
FIGURE 3. Orexin concentrations in cerebrospinal fluid (CSF) obtained by lumbar puncture in subjects with panic anxiety with or without major depressive disorder (MDD). Subjects who presented with acute suicidal behavior were systematically assessed for psychiatric symptoms utilizing the comprehensive psychopathological rating scale (CPRS), where item 3 (inner tension) assesses panic anxiety. A threshold cut off at 1.5 on this item was used to define a subject as having significant panic symptoms. All subjects with substance abuse and traces of anti-depressive, neuroleptic or anxiolytic medication in the blood were excluded from the analysis. Subjects with panic anxiety without MDD (n = 12); subjects with both panic and co-morbid MDD (n = 13); and subjects without panic, without MDD (n = 28). Data are reported as means ± SD; * indicates significant differences from other groups, using Kruskall Wallis ANOVA (p = 0.004); and two-tailed Mann-Whitney U-test (subjects with panic and MDD, p = 0.002; subjects without panic, p = 0.01). Reproduce with permission from Johnson et al. (2010).
Orexin acts on two receptors (ORX1 and ORX2) and their expression in the pontomedullary areas of the autonomic and respiratory network overlap partially (Marcus et al., 2001). ORXA can bind to both receptors whereas ORXB binds primarily to ORX2 (Carrive and Kuwaki, 2017). The fact that the basal respiratory activity of ORX-knock out mice is similar to that of wild-type indicates that ORX neurons have limited impacts on breathing at rest (Nakamura et al., 2007; Berteotti et al., 2020) and while there is evidence indicating that deletion of ORX neurons increases apneic events during sleep (Nakamura et al., 2007), this effect is not always observed (Berteotti et al., 2020). However, activation of ORX neurons potentiates chemoreflexes and there is growing evidence indicating that ORX neurons have CO2-sensing properties (Gestreau et al., 2008; Li and Nattie, 2014; Carrive and Kuwaki, 2017). In mice, exposure to CO2 (10% CO2; 3 h) augments the number of c-FOS immunolabeling in ORXA expressing neurons of the PeF and DMH (but not LH) (Sunanaga et al., 2009). Results from electrophysiological experiments provide more direct support as they show that acidification of the extracellular milieu increases intrinsic excitability and firing rate of ORX cells, whereas alkalinization depresses it. Furthermore, this effect involves acid-induced closure of K+ channels in the orexin cell membrane (Williams et al., 2007). These responses resemble those of known chemosensory neurons; however, the authors did not specify the specific location of the populations of ORX neurons that were recorded. Regulation of ORX neurons is greatly influenced by gonadal hormones and data show that the intensity of expression of ORX1 receptors in the hypothalamus parallels ovarian hormone levels. In rats, their expression peaks during the proestrus phase (Silveyra et al., 2009). As a result, Grafe and Bhatnagar proposed that the ORX system is fundamental to sex-based differences in stress-related neurological disorders such as PD (Grafe and Bhatnagar, 2018). Estradiol (E2) is of great interest in this context because E2 levels rise during proestrus and its inhibitory actions on ORX neurons reduce their responsiveness to stress (Shors et al., 2001). To evaluate the role of E2 in regulating ORX neurons we first used immunohistochemistry and data convincingly show that under resting conditions, OVX augments the ratio of c-FOS/ORXA immunolabeled cells in control females, especially in the DMH. Conversely, OVX had no significant effect in NMS females because the number of labeled cells was already elevated in intact females (Tenorio-Lopes and Kinkead, 2021). We then used whole cell recording to evaluate how changes in E2 across the estrus cycle affects synaptic inputs converging onto ORX neurons and our results showed that NMS reduces E2-mediated inhibition of ORX neurons (Figure 4). During proestrus, the excitatory post-synaptic current (EPSC) frequency of control females was the lowest whereas in NMS females the frequencies were the highest observed in this group. These observations provide a plausible explanation for the higher c-FOS/ORXA immunolabeling, greater ORXA levels measured in hypothalamic extract, and the high efficacy of systemic administration of the selective ORX1 receptor antagonist SB-334867 (15 mg/kg; IP) at reducing the HcVR of NMS female rats. Of note, this drug-induced attenuation of the HcVR was most important during the proestrus phase; in controls, this treatment had no significant effect on the HcVR, regardless of the estrus phase (Tenorio-Lopes and Kinkead, 2021). The sum of these data indicates that stress-induced disruption of E2 signalling is an important mechanism in a rat model of PD and that ORX neurons is an important site of action (Figures 2, 5).
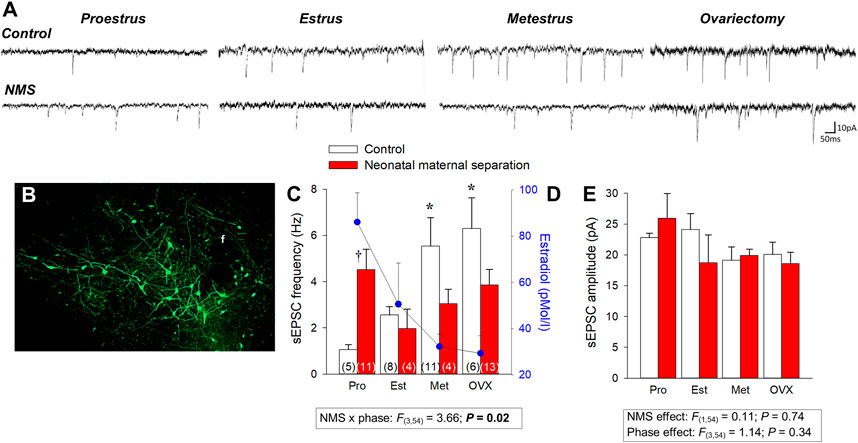
FIGURE 4. Neonatal maternal separation stress (NMS) reduces the spontaneous excitatory postsynaptic currents (sEPSC) recorded in GFP-labeled orexin neurons in response to changes in 17β-estradiol (E2) level in intact and ovarectomized rats. (A) Comparison of sEPSC recordings from orexin neurons between cells during different phase of the estrus cycle and 2 weeks following ovariectomy (OVX); tissue slices originated from females raised under control conditions (top traces) or subjected to NMS (bottom traces; 3 h/day, postnatal days 3–12). (B) Photomicrograph illustrating GFP-labeled orexin neurons; the fornix (f) is shown as a landmark. (C) Population data of EPSC frequencies recorded during 3 distinct phases of the estrus cycle and following OVX. (D) Baseline E2 values are reported for comparison; values from NMS and controls were pooled since they are not statistically different. (E) Reports sEPSC amplitudes. Data are reported as means ± SEM; *p < 0.05 compare to corresponding proestrus value; † p < 0.05 compare to corresponding control. Adapted from Tenorio-Lopes et al (2020).
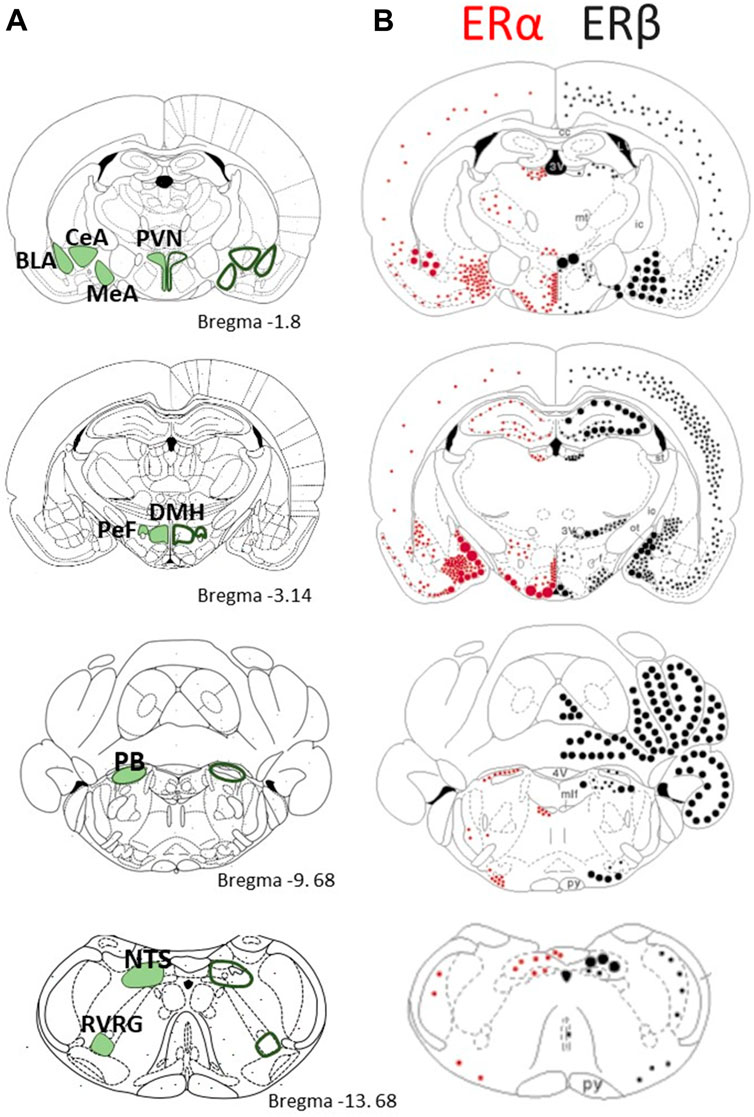
FIGURE 5. Comparison of the distribution of estrogen receptors α and β in brain regions that are responsive to CO2/H+ sensing and/or contribute to the stress response (A) Schmatics on the left present a series of coronal section modified from the rat brain atlas of Paxinos and Watson (1998) with emphasis on key structure with CO2 sensing properties or with established roles in respiratory control; the stereotaxic reference (distance from bregma) is indicated. (B) Schematics on the right present the distribution of ERα (red dots) and ERβ (black dots) mRNA in the rat brain. Small dots represent 1–5 labeled cells; medium dots 6–10 labeled cells; large dots represent approximately 50 labeled cells. Adapted with permission from Shughrue et al., 1997. PVH: Paraventricular nucleus of the hypothalamus; BLA: Basolateral amygdala; CeA: Central nucleus of the amygdala; MeA: Medial nucleus of the amygdala; DMH: Dorsomedial hypothalamic nucleus; PeF: Perifornical area; PB: Parabrachial nucleus; NTS: Nucleus of the solitary tract; RVRG: Rostral ventral respiratory group.
5 Early life stress and its impacts on other mechanisms contributing to CO2 sensing
5.1 Acid-sensing ion channels (ASICs)
The ventilatory response to CO2 is determined by multiple chemosensory structures with specialised capacity for detecting changes in CO2/H+ in their vicinity that project to respiratory neurons to initiate a robust increase in breathing. Acid-sensing ion channels (ASICs) are widely expressed in the brain, including the ventrolateral medulla, where they play a pivotal role in driving CO2/H+ chemosensing and triggering emotional and physiological responses (Song et al., 2016). Regardless of their biological sex, PD patients show variation of the ACCN2 gene, the human ortholog of the Asic1a (Smoller et al., 2014). Consistent with human data, mRNA transcript analysis of the brainstems of male and female mice show heightened ASIC expression in RCF exposed animals (Cittaro et al., 2016); although ASICs are comprised of multiple subunits the authors presumably refer to ASIC1A but this was not specified.
The fact that inactivation of ASIC channels with amiloride attenuates the HcVR of RCF animals but not controls strongly suggests that overexpression of these ion channels is an important mechanism in the abnormal respiratory phenotype associated with PD (Battaglia et al., 2018). However, the fact that amiloride has non-specific effects on a number of other receptors and transporters needs to be considered.
5.2 The carotid bodies
Strategically located at the bifurcation of the carotid arteries, the carotid bodies are main sensors of O2 levels in the arterial blood; however, they also respond to changes in arterial CO2/H+ (Iturriaga et al., 2021). They project to the medulla where they provide powerful chemosensory signals to the respiratory network. Because carotid body stimulation by potassium cyanide injection stimulates fear and escape responses (Schimitel et al., 2012), we determined whether these chemosensors contribute to the excessive HcVR of NMS females. To do so, we compared the responsiveness of the carotid bodies to changes in O2 and CO2 using an ex vivo preparation and the results convincingly showed that NMS does not affect peripheral CO2 sensing in either sex (Soliz et al., 2016). We therefore concluded that anomalies in the CO2 chemoreflex takes place within the brain.
We first evaluated the contribution of the central nervous system (CNS) to this phenotype using anesthetised rats (Dumont and Kinkead, 2010; Dumont et al., 2011; Dumont and Kinkead, 2011), but this approach eliminated the NMS-induced increase of the ventilatory response to CO2 reported in awake females (Dumont et al., 2011). This led us to propose that NMS disrupts anesthesia-sensitive structures responsible for the cognitive and/or emotional perception of the CO2 stimulus (Dumont et al., 2011). This inference was first based on the notion that CO2 is both a systemic and associative stressor. In other words, CO2 is capable of stimulating both physiological (i.e., respiratory) reflexes via conventional pathways and strong emotional and associative reactions, such as fear and escape responses that, in turn, further stimulate breathing (Schenberg, 2016). These observations and the current background knowledge brought our attention to the amygdala.
5.3 Microglia
Microglia are the immune cells of the brain that are mainly known for scavenging the CNS for infectious agents, damaged or unnecessary neurons and synapses. However, there is growing evidence indicating that uncoupling neuron–microglia interactions alters neuroplasticity and contributes to anxiety- or depressive-like behaviors (Koo and Wohleb, 2021). Microglia express cell death–associated gene-8 (TDAG8), an acid-sensing G-protein coupled receptor which is necessary for full expression of CO2-evoked fear (Vollmer et al., 2016). Specifically, freezing and blood pressure responses to CO2 inhalation (5% CO2; 10 min) of TDAG8 deficient mice are lower than those reported in wildtype animals; however, the HcVR does not differ between genotypes (Vollmer et al., 2016). Subsequent experiments demonstrated that upon CO2 exposure, microglia release the proinflammatory cytokine IL-1β which then activates neurons. Quantification of microglial activation and electrophysiological assessment of the CO2 responses were performed in the subfornical organ, a circumventricular organ that lacks a blood brain barrier. Based on comparisons of the cell’s firing rate response of subfornical neurons, the sensitivity to CO2/H+ is ∼10 times less than that reported for the RTN (Guyenet et al., 2019). It was argued that blood-born compounds can have access to the CNS via this route such that this structure acts as an integrative site for the maintenance of homeostasis (Vollmer et al., 2016). This explanation raises the possibility that the area postrema plays a similar role in respiratory manifestations of PD. The area postrema is a medullary circumventricular organ with chemosensing properties located above the NTS; it expresses inward rectifier K+ channels (Kir) associated with CO2 chemosensitivity (Wu et al., 2004) and projects to the RTN, a key medullary structure in CO2 chemodetection (Rosin et al., 2006). This idea is certainly worth exploring.
5.4 Estrogens
Gonadal hormones are “the usual suspects” in mechanistic studies aiming to explain sex-based differences in physiological function. The contribution of 17β-estradiol (E2) is intriguing owing to its multiple and heterogeneous influences on the stress response. On the one hand, the onset of PD-related respiratory disturbances coincides with the rise in circulating E2 at puberty and reports of panic attacks in some women receiving E2-replacement therapy (Price and Heil, 1988; Hayward et al., 1992). This is consistent with the view that E2 is a potent stimulant of the hypothalamic pathways regulating the stress response; female rats in proestrus (high estradiol, high progesterone) and estrus (recent exposure to peak estradiol), have elevated basal and stress induced corticotropic hormone (ACTH) and corticosterone (Viau and Meaney, 1991; Heck and Handa, 2019). On the other hand, E2-replacement therapy may reduce panic symptoms in women and transdermal E2 treatment in menopausal women has been reported to blunt the acute stress response (Lindheim et al., 1992; Chung et al., 1995). In rats, E2-supplementation of ovariectomized females can reduce the response to chronic recurrent stress by attenuating the output of the paraventricular nucleus of the hypothalamus (PVN) (Gerrits et al., 2005). The sum of these observations underlies the view that E2 has anxiolytic properties (Österlund, 2010; Borrow and Handa, 2017). Thus, there is no clear consensus and these apparent discrepancies reflect challenges commonly encountered in stress studies in which the responses vary depending of the intensity, nature, and duration of the challenge used. Furthermore, the sex, species, age/ovarian status of the female along with environmental factors such as nutrition contribute to the variability of estrogen’s actions (Borrow and Handa, 2017; Heck and Handa, 2019). As we discuss below, the two main E2 receptors (ERα and ERβ) have opposing actions on network function, such that slight changes in their relative expression can alter E2’s net effects and thus explain the heterogeneity in its effects (Kunte et al., 2014; Borrow and Handa, 2017).
E2 was initially shown to act via “classical” ERα and ERβ that are ligand-activated transcription factors influencing gene expression; however, both receptors are also expressed outside the nucleus where they induce non-genomic actions. E2 binds equally well to ERα and ERβ, but the two receptors are not functionally interchangeable; the differences in their localisation throughout the rodent brain support this functional divergence (Figure 5; adapted from (Shughrue et al., 1997). Interestingly, the distribution of ERα and ERβ is similar between sexes (Hara et al., 2015), but the levels of expression are generally greater in females (Garcia-Segura et al., 2001). E2 also exerts rapid effects via membrane-bound G-protein estrogen receptors (GPERs); their discovery being more recent (1990s), the responses induced by GPERs are less documented (Hara et al., 2015; Barton et al., 2018). Together, these receptors allow E2 to alter the structure and function of neuronal networks via multiple mechanisms with time courses ranging from seconds to days (Evrard and Balthazart, 2004). We know for instance that E2 facilitates the transmission of electrical signals by promoting synaptic transmission via ERα. The concurrent actions of E2 on ERβ promote the formation of dendritic spines such that in the hippocampus, the spine density fluctuates with the estrus cycle and peaks on the day of proestrus (Woolley and McEwen, 1992; Tan et al., 2012), which is the phase when the largest CO2 response in NMS females were observed (Figure 1) (Tenorio-Lopes et al., 2020). E2 is strongly linked with anxiety disorders and a common view is that activation of ERβ is responsible for its anxiolytic effects whereas ERα initiate fear and anxiety-like behaviors (Walf and Frye, 2006; Frye et al., 2008; Borrow and Handa, 2017). Such generalisation requires caution, however, because each receptor type has distinct effects on glutamatergic and GABAergic signalling. The relative expression of each receptor type thus determines the balance between excitation and inhibition and E2’s net effect on a system (Woolley, 2007; Liu et al., 2008; Tan et al., 2012; Tian et al., 2013). Still, this balance is plastic and factors such as E2 levels and stress influence the relative expression of ERs. For instance, acute immobilization stress augments ERα immunolabeling in the PVN and medullary noradrenergic neurons (A2 area) of females (Estacio et al., 1996). Conversely, E2 generally reduces ERs in the hypothalamus (Simerly and Young, 1991; Garcia-Segura et al., 2001). GPERs also contribute to anxious phenotypes but their role remains unclear because opposing behavioral responses have been reported (Tian et al., 2013; Borrow and Handa, 2017).
Disruption of E2-signalling has therefore emerged as a key mechanism in anxiety disorders (Östlund et al., 2003; Albert et al., 2015) and although respiratory symptoms are an important feature of PD, our comprehension of the actions of E2 on the respiratory control system (including CO2 sensing) is still in its infancy, especially in females. Because female rats previously subjected to NMS closely replicate ontogenic and cyclic features of respiratory manifestations of PD, we took advantage of this model to further our understanding of the contribution of E2 on the ventilatory response to CO2.
Comparison of basal E2 and progesterone levels between NMS and controls across the estrus cycle does not indicate that NMS affects the gonadotropic axis at rest (Figure 6) (Dumont et al., 2011; Tenorio-Lopes et al., 2020). However, analysis of samples harvested following CO2 inhalation shows that this acute challenge stimulates E2 release during proestrus in controls but not in NMS females (Tenorio-Lopes et al., 2020) (Figure 6). We then noted that during proestrus, the intensity of the hyperventilatory response observed in NMS females was inversely proportional to E2 levels observed following CO2 exposure (Tenorio-Lopes et al., 2020). These data indicate that high E2 is a powerful inhibitor of the ventilatory response to CO2 but the E2 level achieved in NMS females is insufficient to prevent an excessive HcVR, especially during proestrus (Tenorio-Lopes et al., 2020). We then tested those conclusions by injecting E2 (3, 10, or 25 µg) in ovariectomized (OVX) females once per day every 4 days to restore E2 level within physiological range and mimic cyclic fluctuations. The last injection was performed ∼2 h prior to ventilatory measurements. Consistent with previous observations in rats, OVX reduced the HcVR (Marques et al., 2015), but the drop was greater in NMS females such that their HcVR (post-OVX) was comparable to that of controls with intact gonads (Tenorio-Lopes et al., 2020). Results from supplementation experiments clearly show that in NMS females, E2’s actions are biphasic with an increasing stimulatory effect until plasma levels reached the range observed during proestrus (∼150 pMol/l); higher doses no longer stimulated the HcVR. In contrast, E2’s influence on the HcVR of controls was limited. In a preliminary and unpublished experiment, we determined whether ERβ contributes to this process by testing the effect of acute IP injection of E2 (40 μg/kg) and the selective ERβ agonist diarylpropionitrile (DPN, 0.1 mg/kg) 1 h prior to CO2 inhalation test. Preliminary observations suggest that, at these doses, this treatment is more effective than E2 at reducing the HcVR, especially in NMS females (Figure 7). These results require further validation and raise questions about the stimulatory actions of a selective ERα agonist on the HcVR. Notwithstanding, E2 can be an important modulator of the neural pathways regulating the CO2 response; it would be interesting to determine whether those actions take place within “classical” medullary circuits or involve more rostral structures. As we discuss below, recent data revealed orexin producing neurons of the hypothalamus as key players in the process.
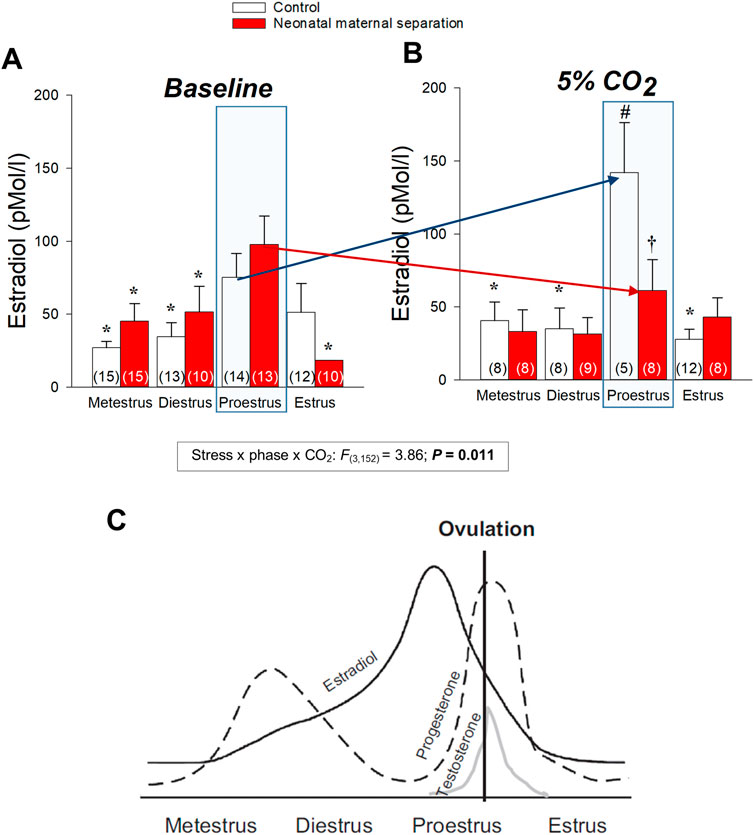
FIGURE 6. Neonatal stress disrupts the plasma 17β-estradiol (E2) response to CO2 inhalation of female rats. Plasma E2 levels measured across the different phases of the estrus cycle (A) in room air and (B) 30 min following exposition to 5% CO2 for 10 min. The numbers in brackets indicate the number of replicates in each group. (C) Schematic representation of the changes in estradiol, progesterone, and testosterone across the estrus cycle in rat. Repreoduc with persmission from (Pfaus et al., 2015). Data are reported as means ± SEM; * indicates a value different from corresponding proestrus value at p < 0.05; † indicates a value different from corresponsing control value at p < 0.05; # indicates a value signifincatly different from corresponsing baseline value at p < 0.05 compare to baseline value. Reproduced with permission from (Tenorio-Lopes et al., 2020).
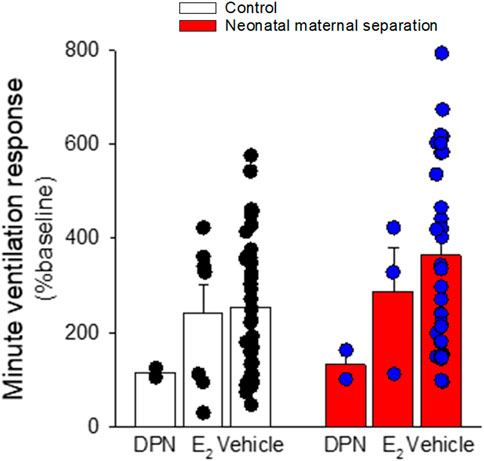
FIGURE 7. 17-β estradiol supplementation attenuates the ventilatory response to the CO2 inhalation (5% CO2; 10 min) in female rats. Rats with intact ovaries either received vehicle, 17-β estradiol (40 μg/kg), or the ER-β agonist diarylpropionitrile (DPN; 0.1 mg/kg) 1 h before the onset of the experiment. Data are reported as means ± SEM; all phases of the cycle are combined. The low number of replicates in DPN treated rats did not allow proper statistical analysis.
6 Conclusion and future directions
CO2 monitoring is essential to respiratory homeostasis and health; consequently, deciphering the cellular and molecular underpinnings of CO2 sensing and the neural networks driving reflexive responses has been a long-standing quest for physiologists. The presence of CO2 sensing neurons on the ventral surface of the medulla has been suspected since the 1960s and today, the RTN is firmly established as a primary structure in feedback regulation of breathing (Guyenet and Bayliss, 2022). The use of modern, “state of the art” approaches has led to important discoveries regarding the role and function of the RTN and we now know that this structure responds to very small changes in CO2/H+ to induce precise respiratory adjustments without producing any conscious aversive sensation (dyspnea), stress, or arousal (Guyenet and Bayliss, 2022). This mini-review and other contributions to this special issue demonstrate that other (non-medullary) brain regions are important contributors to central CO2 chemosenstivity (Nattie and Li, 2011). Figure 2 illustrates how these various structures interact to influence breathing and how E2 related signalling influences network function. While the evidence indicating that these structures can reflexively induce arousal and behavioral responses is compelling, further experiments are necessary to determine their specific contribution to respiratory control since the threshold for their activation seems greater than the RTN. Moreover, it is imperative to determine whether their response to CO2 is the result of a direct action of CO2/H+ or “network driven” changes. Although the contribution of these structures to homeostasis maybe limited under “standard” (healthy) conditions, their contribution to various respiratory disorders is convincing. For instance, CO2-induced arousal contributes to sleep fragmentation during sleep apnea (Kaur et al., 2017; Kaur and Saper, 2019; Kaur et al., 2020) and impairment of this arousal response may be important in the pathophysiology of sudden unexpected death in epilepsy, sudden infant death syndrome, and sleep apnea (Buchanan and Richerson, 2010; Smith et al., 2018; Buchanan, 2019). Here, our discussion focused on PD and the mechanistic studies performed in this context brings further support to this notion as they show that the excessive HcVR observed in stressed female rats reflect abnormal CO2 sensing taking place in structures near the hypothalamus.
Author contributions
All authors listed have made a substantial, direct, and intellectual contribution to the work and approved it for publication.
Funding
DA-M was supported by a postdoctoral scholarship from the Fonds de Recherche du Québec en Santé and Réseau de Recherche en Santé Respiratoie du Québec. MG is supported by a graduate scholarship from the Fonds de Recherche du Québec en Santé. RK’s research program has been supported by the Canadian Institutes of Health Research.
Conflict of interest
The authors declare that the research was conducted in the absence of any commercial or financial relationships that could be construed as a potential conflict of interest.
Publisher’s note
All claims expressed in this article are solely those of the authors and do not necessarily represent those of their affiliated organizations, or those of the publisher, the editors and the reviewers. Any product that may be evaluated in this article, or claim that may be made by its manufacturer, is not guaranteed or endorsed by the publisher.
References
Abelson, J. L., Khan, S., and Giardino, N. (2010). HPA axis, respiration and the airways in stress-A review in search of intersections. Biol. Psychol. 84 (1), 57–65. doi:10.1016/j.biopsycho.2010.01.021
Abelson, J. L., Khan, S., Lyubkin, M., and Giardino, N. (2008). Respiratory irregularity and stress hormones in panic disorder: Exploring potential linkages. Depress. Anxiety 25 (10), 885–887. doi:10.1002/da.20317
Abelson, J. L., Weg, J. G., Nesse, R. M., and Curtis, G. C. (2001). Persistent respiratory irregularity in patients with panic disorder. Biol. Psychiatry 49 (7), 588–595. doi:10.1016/s0006-3223(00)01078-7
Abreu, A. R., Molosh, A. I., Johnson, P. L., and Shekhar, A. (2020). Role of medial hypothalamic orexin system in panic, phobia and hypertension. Brain Res. 1731, 145942. doi:10.1016/j.brainres.2018.09.010
Albert, K., Pruessner, J., and Newhouse, P. (2015). Estradiol levels modulate brain activity and negative responses to psychosocial stress across the menstrual cycle. Psychoneuroendocrinology 59, 14–24. doi:10.1016/j.psyneuen.2015.04.022
American-Psychiatric-Association (1994). Diagnostic and statistical manual of mental disorders: DSM-IV. Washington: American-Psychiatric-Association.
Ashhad, S., Kam, K., Del Negro, C. A., and Feldman, J. L. (2022). Breathing rhythm and pattern and their influence on emotion. Annu. Rev. Neurosci. 45 (1), 223–247. doi:10.1146/annurev-neuro-090121-014424
Bandelow, B., and Michaelis, S. (2015). Epidemiology of anxiety disorders in the 21st century. Dialogues Clin. Neurosci. 17 (3), 327–335. doi:10.31887/DCNS.2015.17.3/bbandelow
Barnett, S., and Li, A. (2020). Orexin in respiratory and autonomic regulation, health and diseases. Compr. Physiol. 10, 345–363. doi:10.1002/cphy.c190013
Barton, M., Filardo, E. J., Lolait, S. J., Thomas, P., Maggiolini, M., and Prossnitz, E. R. (2018). Twenty years of the G protein-coupled estrogen receptor GPER: Historical and personal perspectives. J. Steroid Biochem. Mol. Biol. 176, 4–15. doi:10.1016/j.jsbmb.2017.03.021
Battaglia, M., Bertella, S., Politi, E., Bernardeschi, L., Perna, G., Gabriele, A., et al. (1995). Age at onset of panic disorder: Influence of familial liability to the disease and of childhood separation anxiety disorder. Am. J. Psychiatry 152 (9), 1362–1364. doi:10.1176/ajp.152.9.1362
Battaglia, M., Ogliari, A., D’Amato, F., and Kinkead, R. (2014). Early-life risk factors for panic and separation anxiety disorder: Insights and outstanding questions arising from human and animal studies of CO2 sensitivity. Neurosci. Biobehav. Rev. 46 (3), 455–464. doi:10.1016/j.neubiorev.2014.04.005
Battaglia, M., and Perna, G. (1995). The 35% CO2 challenge in panic disorder: Optimization by receiver operating characteristic (ROC) analysis. J. Psychiatr. Res. 29 (2), 111–119. doi:10.1016/0022-3956(94)00045-s
Battaglia, M., Pesenti-Gritti, P., Medland, S. E., Ogliari, A., Tambs, K., and Spatola, C. M. (2009). A genetically informed study of the association between childhood separation anxiety, sensitivity to co2, panic disorder, and the effect of childhood parental loss. Archives General Psychiatry 66 (1), 64–71. doi:10.1001/archgenpsychiatry.2008.513
Battaglia, M., Rossignol, O., Bachand, K., D’Amato, F. R., and Koninck, Y. D. (2018). Amiloride modulation of carbon dioxide hypersensitivity and thermal nociceptive hypersensitivity induced by interference with early maternal environment. J. Psychopharmacol. 0 (0), 101–108. doi:10.1177/0269881118784872
Beery, A. K., and Kaufer, D. (2015). Stress, social behavior, and resilience: Insights from rodents. Neurobiol. Stress 1, 116–127. doi:10.1016/j.ynstr.2014.10.004
Berteotti, C., Lo Martire, V., Alvente, S., Bastianini, S., Matteoli, G., Silvani, A., et al. (2020). Effect of ambient temperature on sleep breathing phenotype in mice: The role of orexins. J. Exp. Biol. 223 (13), jeb219485. doi:10.1242/jeb.219485
Borrow, A. P., and Handa, R. J. (2017). Estrogen receptors modulation of anxiety-like behavior. Vitam. Horm. 103, 27–52. doi:10.1016/bs.vh.2016.08.004
Buchanan, G. F. (2019). Impaired CO2-induced arousal in SIDS and SUDEP. Trends Neurosci. 42 (4), 242–250. doi:10.1016/j.tins.2019.02.002
Buchanan, G. F., and Richerson, G. B. (2010). Central serotonin neurons are required for arousal to CO2. Proc. Natl. Acad. Sci. 107 (37), 16354–16359. doi:10.1073/pnas.1004587107
Buitelaar, J. K., Huizink, A. C., Mulder, E. J., de Medina, P. G. R., and Visser, G. H. A. (2003). Prenatal stress and cognitive development and temperament in infants. Neurobiol. Aging 24, S53–S60. doi:10.1016/s0197-4580(03)00050-2
Buss, C., Davis, E. P., Shahbaba, B., Pruessner, J. C., Head, K., and Sandman, C. A. (2012). Maternal cortisol over the course of pregnancy and subsequent child amygdala and hippocampus volumes and affective problems. Proc. Natl. Acad. Sci. 109 (20), E1312–E1319. doi:10.1073/pnas.1201295109
Bystritsky, A., Craske, M., Maidenberg, E., Vapnik, T., and Shapiro, D. (2000). Autonomic reactivity of panic patients during a CO2 inhalation procedure. Depress. Anxiety 11 (1), 15–26. doi:10.1002/(sici)1520-6394(2000)11:1<15:aid-da3>3.0.co;2-w
Cahill, L. (2010). “Chapter 3 - sex influences on brain and emotional memory: The burden of proof has shifted,” in Progress in brain research. Editor S. Ivanka (Netherlands: Elsevier).
Carrive, P., and Kuwaki, T. (2017). “Orexin and central modulation of cardiovascular and respiratory function,” in Current topics in behavioral neurosciences. Editors A. J. Lawrence, and L. de Lecea (Netherlands: Elsevier).
Charil, A., Laplante, D. P., Vaillancourt, C., and King, S. (2010). Prenatal stress and brain development. Brain Res. Rev. 65 (1), 56–79. doi:10.1016/j.brainresrev.2010.06.002
Chung, C. K., Remington, N. D., and Suh, B. Y. (1995). Estrogen replacement therapy may reduce panic symptoms. J. Clin. Psychiatry 56 (11), 533.
Ciriello, J., Caverson, M. M., McMurray, J. C., and Bruckschwaiger, E. B. (2013). Co-localization of hypocretin-1 and leucine-enkephalin in hypothalamic neurons projecting to the nucleus of the solitary tract and their effect on arterial pressure. Neuroscience 250, 599–613. doi:10.1016/j.neuroscience.2013.07.054
Cittaro, D., Lampis, V., Luchetti, A., Coccurello, R., Guffanti, A., Felsani, A., et al. (2016). Histone modifications in a mouse model of early adversities and panic disorder: Role for Asic1 and neurodevelopmental genes. Sci. Rep. 6 (1), 25131. doi:10.1038/srep25131
D'Amato, F. R., Zanettini, C., Lampis, V., Coccurello, R., Pascucci, T., Ventura, R., et al. (2011). Unstable maternal environment, separation anxiety, and heightened CO2 sensitivity induced by gene-by-environment interplay. PLoS ONE 6 (4), e18637. doi:10.1371/journal.pone.0018637
Díaz-Casares, A., López-González, M. V., Peinado-Aragonés, C. A., Lara, J. P., González-Barón, S., and Dawid-Milner, M. S. (2009). Role of the parabrachial complex in the cardiorespiratory response evoked from hypothalamic defense area stimulation in the anesthetized rat. Brain Res. 1279, 58–70. doi:10.1016/j.brainres.2009.02.085
DiMicco, J. A., Samuels, B. C., Zaretskaia, M. V., and Zaretsky, D. V. (2002). The dorsomedial hypothalamus and the response to stress: Part renaissance, part revolution. Pharmacol. Biochem. Behav. 71 (3), 469–480. doi:10.1016/s0091-3057(01)00689-x
Dlouhy, B. J., Gehlbach, B. K., Kreple, C. J., Kawasaki, H., Oya, H., Buzza, C., et al. (2015). Breathing inhibited when seizures spread to the amygdala and upon amygdala stimulation. J. Neurosci. 35 (28), 10281–10289. doi:10.1523/jneurosci.0888-15.2015
Donner, N., and Lowry, C. (2013). Sex differences in anxiety and emotional behavior. Pflügers Archiv - Eur. J. Physiology 465 (5), 601–626. doi:10.1007/s00424-013-1271-7
Drury, A. N. (1918). The percentage of carbon dioxide in the alveolar air, and the tolerance to accumulating carbon dioxide in case of so-called "irritable heart. Heart 7, 165–173.
Dumont, F. S., Biancardi, V., and Kinkead, R. (2011). Hypercapnic ventilatory response of anesthetized female rats subjected to neonatal maternal separation: Insight into the origins of panic attacks? Respir. Physiology Neurobiol. 175 (2), 288–295. doi:10.1016/j.resp.2010.12.004
Dumont, F. S., and Kinkead, R. (2011). Neonatal stress and abnormal hypercapnic ventilatory response of adult male rats: The role of central chemodetection and pulmonary stretch receptors. Respir. Physiology Neurobiol. 179 (2-3), 158–166. doi:10.1016/j.resp.2011.07.012
Dumont, F. S., and Kinkead, R. (2010). Neonatal stress and attenuation of the hypercapnic ventilatory response in adult male rats: The role of carotid chemoreceptors and baroreceptors. Am. J. Physiol. Regul. Integr. Comp. Physiol. 299 (5), R1279–R1289. doi:10.1152/ajpregu.00446.2010
Edelmann, M. N., and Auger, A. P. (2011). Epigenetic impact of simulated maternal grooming on estrogen receptor alpha within the developing amygdala. Brain, Behav. Immun. 25 (7), 1299–1304. doi:10.1016/j.bbi.2011.02.009
Elliot-Portal, E., Arias-Reyes, C., Laouafa, S., Tam, R., Kinkead, R., and Soliz, J. (2021). Cerebral erythropoietin prevents sex-dependent disruption of respiratory control induced by early life stress. Front. Physiology 12 (2280), 701344. doi:10.3389/fphys.2021.701344
Estacio, M. A. C., Yamada, S., Tsukamura, H., Hirunagi, K., and Maeda, K.-i. (1996). Effect of fasting and immobilization stress on estrogen receptor immunoreactivity in the brain in ovariectomized female rats. Brain Res. 717 (1), 55–61. doi:10.1016/0006-8993(96)00022-4
Evrard, H. C., and Balthazart, J. (2004). Rapid regulation of pain by estrogens synthesized in spinal dorsal horn neurons. J. Neurosci. 24 (33), 7225–7229. doi:10.1523/JNEUROSCI.1638-04.2004
Feinstein, J. S., Buzza, C., Hurlemann, R., Follmer, R. L., Dahdaleh, N. S., Coryell, W. H., et al. (2013). Fear and panic in humans with bilateral amygdala damage. Nat. Neurosci. 16 (3), 270–272. doi:10.1038/nn.3323
Feinstein, J. S., Gould, D., and Khalsa, S. S. (2022). Amygdala-driven apnea and the chemoreceptive origin of anxiety. Biol. Psychol. 170, 108305. doi:10.1016/j.biopsycho.2022.108305
Feng, P., Vurbic, D., Wu, Z., and Strohl, K. P. (2007). Brain orexins and wake regulation in rats exposed to maternal deprivation. Brain Res. 1154 (0), 163–172. doi:10.1016/j.brainres.2007.03.077
Frye, C. A., Koonce, C. J., Edinger, K. L., Osborne, D. M., and Walf, A. A. (2008). Androgens with activity at estrogen receptor beta have anxiolytic and cognitive-enhancing effects in male rats and mice. Hormones Behav. 54 (5), 726–734. doi:10.1016/j.yhbeh.2008.07.013
Fumagalli, F., Molteni, R., Racagni, G., and Riva, M. A. (2007). Stress during development: Impact on neuroplasticity and relevance to psychopathology. Prog. Neurobiol. 81 (4), 197–217. doi:10.1016/j.pneurobio.2007.01.002
Garbarino, S., Bardwell, W. A., Guglielmi, O., Chiorri, C., Bonanni, E., and Magnavita, N. (2020). Association of anxiety and depression in obstructive sleep apnea patients: A systematic review and meta-analysis. Behav. Sleep. Med. 18 (1), 35–57. doi:10.1080/15402002.2018.1545649
Garcia-Segura, L. M., Azcoitia, I., and DonCarlos, L. L. (2001). Neuroprotection by estradiol. Prog. Neurobiol. 63 (1), 29–60. doi:10.1016/S0301-0082(00)00025-3
Gardner, W. N. (1996). The pathophysiology of hyperventilation disorders. Chest 109 (2), 516–534. doi:10.1378/chest.109.2.516
Gargaglioni, L. H., Marques, D. A., and Patrone, L. G. A. (2019). Sex differences in breathing. Comp. Biochem. Physiology Part A Mol. Integr. Physiology 238, 110543. doi:10.1016/j.cbpa.2019.110543
Genest, S. E., Gulemetova, R., Laforest, S., Drolet, G., and Kinkead, R. (2007). Neonatal maternal separation induces sex-specific augmentation of the hypercapnic ventilatory response in awake rat. J. Appl. Physiol. 102, 1416–1421. doi:10.1152/japplphysiol.00454.2006
Gerrits, M., Grootkarijn, A., Bekkering, B. F., Bruinsma, M., Boer, J. A. D., and Horst, G. J. T. (2005). Cyclic estradiol replacement attenuates stress-induced c-Fos expression in the PVN of ovariectomized rats. Brain Res. Bull. 67 (1–2), 147–155. doi:10.1016/j.brainresbull.2005.06.021
Gestreau, C., Bevengut, M., and Dutschmann, M. (2008). The dual role of the orexin/hypocretin system in modulating wakefulness and respiratory drive. Curr. Opin. Pulm. Med. 14 (6), 512–518. doi:10.1097/MCP.0b013e32831311d3
Goldstein, J. M., Jerram, M., Abbs, B., Whitfield-Gabrieli, S., and Makris, N. (2010). Sex differences in stress response circuitry activation dependent on female hormonal cycle. J. Neurosci. 30 (2), 431–438. doi:10.1523/jneurosci.3021-09.2010
Gorman, J. M., Kent, J., Martinez, J., Browne, S., Coplan, J., and Papp, L. A. (2001). Physiological changes during carbon dioxide inhalation in patients with panic disorder, major depression, and premenstrual dysphoric disorder: Evidence for a central fear mechanism. Arch. Gen. Psychiatry 58 (2), 125–131. doi:10.1001/archpsyc.58.2.125
Gorman, J. M., Kent, J. M., Sullivan, G. M., and Coplan, J. D. (2000). Neuroanatomical hypothesis of panic disorder, revised. Am. J. Psychiatry 157 (4), 493–505. doi:10.1176/appi.ajp.157.4.493
Gottschalk, M. G., Richter, J., Ziegler, C., Schiele, M. A., Mann, J., Geiger, M. J., et al. (2019). Orexin in the anxiety spectrum: Association of a HCRTR1 polymorphism with panic disorder/agoraphobia, CBT treatment response and fear-related intermediate phenotypes. Transl. Psychiatry 9 (1), 75. doi:10.1038/s41398-019-0415-8
Grafe, L. A., and Bhatnagar, S. (2018). The contribution of orexins to sex differences in the stress response. Brain Res. 1731, 145893. doi:10.1016/j.brainres.2018.07.026
Graham, Y. P., Heim, C., Goodman, S. H., Miller, A. H., and Nemeroff, C. B. (1999). The effects of neonatal stress on brain development: Implications for psychopathology. Dev. Psychopathol. 11 (3), 545–565. doi:10.1017/s0954579499002205
Grassi, M., Caldirola, D., Vanni, G., Guerriero, G., Piccinni, M., Valchera, A., et al. (2013). Baseline respiratory parameters in panic disorder: A meta-analysis. J. Affect. Disord. 146 (2), 158–173. doi:10.1016/j.jad.2012.08.034
Gunnar, M. R. (2003). Integrating neuroscience and psychological approaches in the study of early experiences. Ann. N. Y. Acad. Sci. 1008, 238–247. doi:10.1196/annals.1301.024
Guyenet, P. G., and Bayliss, D. A. (2015). Neural control of breathing and CO2 homeostasis. Neuron 87 (5), 946–961. doi:10.1016/j.neuron.2015.08.001
Guyenet, P. G., and Bayliss, D. A. (2022). Central respiratory chemoreception. Handb. Clin. Neurol. 188, 37–72. doi:10.1016/b978-0-323-91534-2.00007-2
Guyenet, P. G., Stornetta, R. L., Souza, G. M. P. R., Abbott, S. B. G., Shi, Y., and Bayliss, D. A. (2019). The retrotrapezoid nucleus: Central chemoreceptor and regulator of breathing automaticity. Trends Neurosci. 42 (11), 807–824. doi:10.1016/j.tins.2019.09.002
Hara, Y., Waters, E. M., McEwen, B. S., and Morrison, J. H. (2015). Estrogen effects on cognitive and synaptic health over the lifecourse. Physiol. Rev. 95 (3), 785–807. doi:10.1152/physrev.00036.2014
Harper, R. M., Frysinger, R. C., Trelease, R. B., and Marks, J. D. (1984). State-dependent alteration of respiratory cycle timing by stimulation of the central nucleus of the amygdala. Brain Res. 306 (1–2), 1–8. doi:10.1016/0006-8993(84)90350-0
Harris, G. C., and Aston-Jones, G. (2006). Arousal and reward: A dichotomy in orexin function. Trends Neurosci. 29 (10), 571–577. doi:10.1016/j.tins.2006.08.002
Hayward, C., Killen, J. D., Hammer, L. D., Litt, I. F., Wilson, D. M., Simmonds, B., et al. (1992). Pubertal stage and panic attack history in sixth- and seventh-grade girls. Am. J. Psychiatry 149 (9), 1239–1243. doi:10.1176/ajp.149.9.1239
Heck, A. L., and Handa, R. J. (2019). Sex differences in the hypothalamic–pituitary–adrenal axis’ response to stress: An important role for gonadal hormones. Neuropsychopharmacology 44 (1), 45–58. doi:10.1038/s41386-018-0167-9
Hoppe, L. J., Ipser, J., Gorman, J. M., and Stein, D. J. (2012). “Panic disorder,” in Handbook of clinical neurology. Editors F. B. J. MichaelAminoff, and F. S. Dick (Netherlands: Elsevier).
Iturriaga, R., Alcayaga, J., Chapleau, M. W., and Somers, V. K. (2021). Carotid body chemoreceptors: Physiology, pathology, and implications for health and disease. Physiol. Rev. 101 (3), 1177–1235. doi:10.1152/physrev.00039.2019
Johnson, P. L., Truitt, W., Fitz, S. D., Minick, P. E., Dietrich, A., Sanghani, S., et al. (2010). A key role for orexin in panic anxiety. Nat. Med. 16 (1), 111–115. doi:10.1038/nm.2075
Katzman, M. A., Struzik, L., Vijay, N., Coonerty-Femiano, A., Mahamed, S., and Duffin, J. (2002). Central and peripheral chemoreflexes in panic disorder. Psychiatry Res. 113 (1-2), 181–192. doi:10.1016/s0165-1781(02)00238-x
Kaur, S., De Luca, R., Khanday, M. A., Bandaru, S. S., Thomas, R. C., Broadhurst, R. Y., et al. (2020). Role of serotonergic dorsal raphe neurons in hypercapnia-induced arousals. Nat. Commun. 11 (1), 2769. doi:10.1038/s41467-020-16518-9
Kaur, S., and Saper, C. B. (2019). Neural circuitry underlying waking up to hypercapnia. Front. Neurosci. 13 (401), 401. doi:10.3389/fnins.2019.00401
Kaur, S., Wang, J. L., Ferrari, L., Thankachan, S., Kroeger, D., Venner, A., et al. (2017). A genetically defined circuit for arousal from sleep during hypercapnia. Neuron 96 (5), 1153–1167. doi:10.1016/j.neuron.2017.10.009
Keshavarzi, S., Sullivan, R. K. P., Ianno, D. J., and Sah, P. (2014). Functional properties and projections of neurons in the medial amygdala. J. Neurosci. 34 (26), 8699–8715. doi:10.1523/jneurosci.1176-14.2014
Kinkead, R., Gagnon, M., Carrier, M.-C., Fournier, S., and Ambrozio-Marques, D. (2022). Amygdala-driven apnea: A breath of fresh air in respiratory neurobiology. Biol. Psychol. 170, 108307. doi:10.1016/j.biopsycho.2022.108307
Kinkead, R., Montandon, G., Bairam, A., Lajeunesse, Y., and Horner, R. L. (2009). Neonatal maternal separation disrupts regulation of sleep and breathing in adult male rats. Sleep 32 (12), 1611–1620. doi:10.1093/sleep/32.12.1611
Kinkead, R., Tenorio, L., Drolet, G., Bretzner, F., and Gargaglioni, L. (2014). Respiratory manifestations of panic disorder in animals and humans: A unique opportunity to understand how supramedullary structures regulate breathing. Respir. Physiol. Neurobiol. 204 (0), 3–13. doi:10.1016/j.resp.2014.06.013
Klein, D. F. (1993). False suffocation alarms, spontaneous panics, and related conditions. An integrative hypothesis. Archives General Psychiatry 50 (4), 306–317. doi:10.1001/archpsyc.1993.01820160076009
Koo, J. W., and Wohleb, E. S. (2021). How stress shapes neuroimmune function: Implications for the neurobiology of psychiatric disorders. Biol. Psychiatry 90 (2), 74–84. doi:10.1016/j.biopsych.2020.11.007
Kunte, H., Harms, L., Plag, J., Hellweg, R., and Kronenberg, G. (2014). Acute onset of panic attacks after transdermal estrogen replacement. General Hosp. Psychiatry 36 (5), e7. doi:10.1016/j.genhosppsych.2014.05.009
Li, A., and Nattie, E. E. (2014). Orexin, cardio-respiratory function and hypertension. Front. Neurosci. 8, 22. doi:10.3389/fnins.2014.00022
Lindheim, S. R., Legro, R. S., Bernstein, L., Stanczyk, F. Z., Vijod, M. A., Presser, S. C., et al. (1992). Behavioral stress responses in premenopausal and postmenopausal women and the effects of estrogen. Am. J. Obstetrics Gynecol. 167 (6), 1831–1836. doi:10.1016/0002-9378(92)91783-7
Liu, F., Day, M., Muñiz, L. C., Bitran, D., Arias, R., Revilla-Sanchez, R., et al. (2008). Activation of estrogen receptor-β regulates hippocampal synaptic plasticity and improves memory. Nat. Neurosci. 11 (3), 334–343. doi:10.1038/nn2057
Lovick, T. A. (2014). Sex determinants of experimental panic attacks. Neurosci. Biobehav Rev. 46P3, 465–471. doi:10.1016/j.neubiorev.2014.03.006
Luchetti, A., Oddi, D., Lampis, V., Centofante, E., Felsani, A., Battaglia, M., et al. (2015). Early handling and repeated cross-fostering have opposite effect on mouse emotionality. Front. Behav. Neurosci. 9 (93), 93. doi:10.3389/fnbeh.2015.00093
Marcus, J. N., Aschkenasi, C. J., Lee, C. E., Chemelli, R. M., Saper, C. B., Yanagisawa, M., et al. (2001). Differential expression of orexin receptors 1 and 2 in the rat brain. J. Comp. Neurology 435 (1), 6–25. doi:10.1002/cne.1190
Marek, R., Strobel, C., Bredy, T. W., and Sah, P. (2013). The amygdala and medial prefrontal cortex: Partners in the fear circuit. J. Physiology 591 (10), 2381–2391. doi:10.1113/jphysiol.2012.248575
Marques, D. A., Carvalho, D. d., Silva, G. S. F. d., Szawka, R. E., Anselmo-Franci, J. A., Bícego, K. C., et al. (2015). Ventilatory, metabolic, and thermal responses to hypercapnia in female rats: Effects of estrous cycle, ovariectomy, and hormonal replacement. J. Appl. Physiology 119 (1), 61–68. doi:10.1152/japplphysiol.00254.2015
Meng, X., and D'Arcy, C. (2012). Common and unique risk factors and comorbidity for 12-month mood and anxiety disorders among Canadians. Can. J. Psychiatry 57 (8), 479–487. doi:10.1177/070674371205700806
Moreira, F., Gobira, P., Viana, T., Vicente, M., Zangrossi, H., and Graeff, F. (2013). Modeling panic disorder in rodents. Cell Tissue Res. 354, 119–125. doi:10.1007/s00441-013-1610-1
Nakamura, A., Zhang, W., Yanagisawa, M., Fukuda, Y., and Kuwaki, T. (2007). Vigilance state-dependent attenuation of hypercapnic chemoreflex and exaggerated sleep apnea in orexin knockout mice. J. Appl. Physiology 102 (1), 241–248. doi:10.1152/japplphysiol.00679.2006
Nardi, A. E., Freire, R. C., and Zin, W. A. (2009). Panic disorder and control of breathing. Respir. Physiology Neurobiol. 167, 133–143. doi:10.1016/j.resp.2008.07.011
Nattie, E., and Li, A. (2011). “Central chemoreceptors: Locations and functions,” in Comprehensive physiology (New Jersey: John Wiley & Sons, Inc).
Nattie, E., and Li, A. (2012). “Chapter 4 - respiration and autonomic regulation and orexin,” in Progress in brain research. Editor S. Anantha (Netherlands: Elsevier).
Nillni, Y. I., Pineles, S. L., Rohan, K. J., Zvolensky, M. J., and Rasmusson, A. M. (2017). The influence of the menstrual cycle on reactivity to a CO2 challenge among women with and without premenstrual symptoms. Cogn. Behav. Ther. 46 (3), 239–249. doi:10.1080/16506073.2016.1236286
Nillni, Y. I., Toufexis, D. J., and Rohan, K. J. (2011). Anxiety sensitivity, the menstrual cycle, and panic disorder: A putative neuroendocrine and psychological interaction. Clin. Psychol. Rev. 31 (7), 1183–1191. doi:10.1016/j.cpr.2011.07.006
Österlund, M. K. (2010). Underlying mechanisms mediating the antidepressant effects of estrogens. Biochimica Biophysica Acta (BBA) - General Subj. 1800 (10), 1136–1144. doi:10.1016/j.bbagen.2009.11.001
Östlund, H., Keller, E., and Hurd, Y. L. (2003). Estrogen receptor gene expression in relation to neuropsychiatric disorders. Ann. N. Y. Acad. Sci. 1007 (1), 54–63. doi:10.1196/annals.1286.006
Petrov, T., Krukoff, T. L., and Jhamandas, J. H. (1995). Convergent influence of the central nucleus of the amygdala and the paraventricular hypothalamic nucleus upon brainstem autonomic neurons as revealed by c-fos expression and anatomical tracing. J. Neurosci. Res. 42 (6), 835–845. doi:10.1002/jnr.490420612
Pfaus, J. G., Jones, S. L., Flanagan-Cato, L. M., and Blaustein, J. D. (2015). “Chapter 50 - female sexual behavior,” in Knobil and neill's physiology of reproduction. Editors T. M. Plant, and A. J. Zeleznik Fourth Edition (San Diego: Academic Press).
Pigott, T. A. (2003). Anxiety disorders in women. Psychiatric Clin. N. Am. 26 (3), 621–672. vi-vii. doi:10.1016/s0193-953x(03)00040-6
Price, W. A., and Heil, D. (1988). Estrogen-induced panic attacks. Psychosomatics 29 (4), 433–435. doi:10.1016/s0033-3182(88)72347-6
Putnam, R. W., Conrad, S. C., Gdovin, M. J., Erlichman, J. S., and Leiter, J. C. (2005). Neonatal maturation of the hypercapnic ventilatory response and central neural CO2 chemosensitivity. Respir. Physiol. Neurobiol. 149, 165–179. doi:10.1016/j.resp.2005.03.004
Rappaport, L. M., Sheerin, C., Carney, D. M., Towbin, K. E., Leibenluft, E., Pine, D. S., et al. (2017). Clinical correlates of carbon dioxide hypersensitivity in children. J. Am. Acad. Child Adolesc. Psychiatry 56 (12), 1089–1096. doi:10.1016/j.jaac.2017.09.423
Reardon, L. E., Leen-Feldner, E. W., and Hayward, C. (2009). A critical review of the empirical literature on the relation between anxiety and puberty. Clin. Psychol. Rev. 29 (1), 1–23. doi:10.1016/j.cpr.2008.09.005
Reed, V., and Wittchen, H. U. (1998). DSM-IV panic attacks and panic disorder in a community sample of adolescents and young adults: How specific are panic attacks? J. Psychiatr. Res. 32 (6), 335–345. doi:10.1016/s0022-3956(98)00014-4
Rhone, A. E., Kovach, C. K., Harmata, G. I. S., Sullivan, A. W., Tranel, D., Ciliberto, M. A., et al. (2020). A human amygdala site that inhibits respiration and elicits apnea in pediatric epilepsy. JCI Insight 5 (6), e134852. doi:10.1172/jci.insight.134852
Ritchie, E. C. (1992). Treatment of gas mask phobia. Mil. Med. 157 (2), 104–106. doi:10.1093/milmed/157.2.104
Roberson-Nay, R., and Kendler, K. S. (2011). Panic disorder and its subtypes: A comprehensive analysis of panic symptom heterogeneity using epidemiological and treatment seeking samples. Psychol. Med. 41, 2411–2421. doi:10.1017/S0033291711000547
Roberson-Nay, R., Klein, D. F., Klein, R. G., Mannuzza, S., Moulton, J. L., Guardino, M., et al. (2010). Carbon dioxide hypersensitivity in separation-anxious offspring of parents with panic disorder. Biol. psychiatry 67 (12), 1171–1177. doi:10.1016/j.biopsych.2009.12.014
Rodrigues, S. M., LeDoux, J. E., and Sapolsky, R. M. (2009). The influence of stress hormones on fear circuitry. Annu. Rev. Neurosci. 32 (1), 289–313. doi:10.1146/annurev.neuro.051508.135620
Rosin, D. L., Chang, D. A., and Guyenet, P. G. (2006). Afferent and efferent connections of the rat retrotrapezoid nucleus. J. Comp. Neurology 499 (1), 64–89. doi:10.1002/cne.21105
Schenberg, L. (2016). “A neural systems approach to the study of respiratory-type panic disorder,” in Panic disorder. Editors A. Nardi, and R. RCR (Switzerland: Springer international Publishing).
Schimitel, F. G., de Almeida, G. M., Pitol, D. N., Armini, R. S., Tufik, S., and Schenberg, L. C. (2012). Evidence of a suffocation alarm system within the periaqueductal gray matter of the rat. Neuroscience 200, 59–73. doi:10.1016/j.neuroscience.2011.10.032
Shonkoff, J. P., Boyce, W. T., and McEwen, B. S. (2009). Neuroscience, molecular biology, and the childhood roots of health disparities: Building a new framework for health promotion and disease prevention. JAMA 301 (21), 2252–2259. doi:10.1001/jama.2009.754
Shors, T. J., Chua, C., and Falduto, J. (2001). Sex differences and opposite effects of stress on dendritic spine density in the male versus female Hippocampus. J. Neurosci. 21 (16), 6292–6297. doi:10.1523/jneurosci.21-16-06292.2001
Shughrue, P. J., Lane, M. V., and Merchenthaler, I. (1997). Comparative distribution of estrogen receptor-alpha and -beta mRNA in the rat central nervous system. J. Comp. Neurol. 388 (4), 507–525. doi:10.1002/(sici)1096-9861(19971201)388:4<507:aid-cne1>3.0.co;2-6
Silveyra, P., Cataldi, N. I., Lux-Lantos, V., and Libertun, C. (2009). Gonadal steroids modulated hypocretin/orexin type-1 receptor expression in a brain region, sex and daytime specific manner. Regul. Pept. 158 (1–3), 121–126. doi:10.1016/j.regpep.2009.08.002
Simerly, R. B., and Young, B. J. (1991). Regulation of estrogen receptor messenger ribonucleic acid in rat hypothalamus by sex steroid hormones. Mol. Endocrinol. 5 (3), 424–432. doi:10.1210/mend-5-3-424
Sinha, S., Papp, L. A., and Gorman, J. M. (2000). How study of respiratory physiology aided our understanding of abnormal brain function in panic disorder. J. Affect Disord. 61 (3), 191–200. doi:10.1016/s0165-0327(00)00337-2
Smith, H. R., Leibold, N. K., Rappoport, D. A., Ginapp, C. M., Purnell, B. S., Bode, N. M., et al. (2018). Dorsal raphe serotonin neurons mediate CO2-induced arousal from sleep. J. Neurosci. 38 (8), 1915–1925. doi:10.1523/jneurosci.2182-17.2018
Smoller, J. W., Gallagher, P. J., Duncan, L. E., McGrath, L. M., Haddad, S. A., Holmes, A. J., et al. (2014). The human ortholog of acid-sensing ion channel gene ASIC1a is associated with panic disorder and amygdala structure and function. Biol. Psychiatry 76 (11), 902–910. doi:10.1016/j.biopsych.2013.12.018
Soliz, J., Tam, R., and Kinkead, R. (2016). Neonatal maternal separation augments carotid body response to hypoxia in adult males but not female rats. Front. Physiology 7 (432), 432. doi:10.3389/fphys.2016.00432
Song, N., Guan, R., Jiang, Q., Hassanzadeh, C. J., Chu, Y., Zhao, X., et al. (2016). Acid-sensing ion channels are expressed in the ventrolateral medulla and contribute to central chemoreception. Sci. Rep. 6 (1), 38777. doi:10.1038/srep38777
Stein, M. B., Millar, T. W., Larsen, D. K., and Kryger, M. H. (1995). Irregular breathing during sleep in patients with panic disorder. Am. J. Psychiatry 152 (8), 1168–1173. doi:10.1176/ajp.152.8.1168
Sunanaga, J., Deng, B.-S., Zhang, W., Kanmura, Y., and Kuwaki, T. (2009). CO2 activates orexin-containing neurons in mice. Respir. Physiology Neurobiol. 166 (3), 184–186. doi:10.1016/j.resp.2009.03.006
Tan, X.-j., Dai, Y.-b., Wu, W.-f., Kim, H.-J., Barros, R. P. A., Richardson, T. I., et al. (2012). Reduction of dendritic spines and elevation of GABAergic signaling in the brains of mice treated with an estrogen receptor β ligand. Proc. Natl. Acad. Sci. 109 (5), 1708–1712. doi:10.1073/pnas.1121162109
Taugher, R. J., Dlouhy, B. J., Kreple, C. J., Ghobbeh, A., Conlon, M. M., Wang, Y., et al. (2020). The amygdala differentially regulates defensive behaviors evoked by CO2. Behav. Brain Res. 377, 112236. doi:10.1016/j.bbr.2019.112236
Tenorio-Lopes, L., Fournier, S., Henry, M. S., Bretzner, F., and Kinkead, R. (2020). Disruption of estradiol regulation of orexin neurons: A novel mechanism in excessive ventilatory response to CO2 inhalation in a female rat model of panic disorder. Transl. Psychiatry 10 (1), 394. doi:10.1038/s41398-020-01076-x
Tenorio-Lopes, L., Henry, M. S., Marques, D., Tremblay, M. È., Drolet, G., Bretzner, F., et al. (2017). Neonatal maternal separation opposes the facilitatory effect of castration on the respiratory response to hypercapnia of the adult male rat: Evidence for the involvement of the medial amygdala. J. Neuroendocrinol. 29 (12), e12550. doi:10.1111/jne.12550
Tenorio-Lopes, L., and Kinkead, R. (2021). Sex-specific effects of stress on respiratory control: Plasticity, adaptation, and dysfunction. Compr. Physiol. 11, 2097–2134. doi:10.1002/cphy.c200022
Tian, Z., Wang, Y., Zhang, N., Guo, Y.-y., Feng, B., Liu, S.-b., et al. (2013). Estrogen receptor GPR30 exerts anxiolytic effects by maintaining the balance between GABAergic and glutamatergic transmission in the basolateral amygdala of ovariectomized mice after stress. Psychoneuroendocrinology 38 (10), 2218–2233. doi:10.1016/j.psyneuen.2013.04.011
Ulrich-Lai, Y. M., and Herman, J. P. (2009). Neural regulation of endocrine and autonomic stress responses. Nat. Rev. Neurosci. 10 (6), 397–409. doi:10.1038/nrn2647
van Duinen, M. A., Schruers, K. R. J., Maes, M., and Griez, E. J. L. (2007). CO2 challenge induced HPA axis activation in panic. Int. J. Neuropsychopharmacol. 10 (06), 797–804. doi:10.1017/S1461145706007358
Veening, J. G., Swanson, L. W., and Sawchenko, P. E. (1984). The organization of projections from the central nucleus of the amygdala to brainstem sites involved in central autonomic regulation: A combined retrograde transport-immunohistochemical study. Brain Res. 303 (2), 337–357. doi:10.1016/0006-8993(84)91220-4
Venkatraman, A., Edlow, B. L., and Immordino-Yang, M. H. (2017). The brainstem in emotion: A review. Front. Neuroanat. 11 (15), 15. doi:10.3389/fnana.2017.00015
Viau, V., and Meaney, M. J. (1991). Variations in the hypothalamic-pituitary-adrenal response to stress during the estrous cycle in the rat. Endocrinology 129 (5), 2503–2511. doi:10.1210/endo-129-5-2503
Vollmer, L. L., Ghosal, S., McGuire, J. L., Ahlbrand, R. L., Li, K.-Y., Santin, J. M., et al. (2016). Microglial acid sensing regulates carbon dioxide-evoked fear. Biol. Psychiatry 80 (7), 541–551. doi:10.1016/j.biopsych.2016.04.022
Walf, A. A., and Frye, C. A. (2006). A review and update of mechanisms of estrogen in the hippocampus and amygdala for anxiety and depression behavior. Neuropsychopharmacology 31 (6), 1097–1111. doi:10.1038/sj.npp.1301067
Wang, X., Guan, R., Zhao, X., Chen, J., Zhu, D., Shen, L., et al. (2021). TASK1 and TASK3 in orexin neuron of lateral hypothalamus contribute to respiratory chemoreflex by projecting to nucleus tractus solitarius. FASEB J. 35 (5), e21532. doi:10.1096/fj.202002189R
Wilhelm, F. H., Gevirtz, R., and Roth, W. T. (2001). Respiratory dysregulation in anxiety, functional cardiac, and pain disorders. Assessment, phenomenology, and treatment. Behav. Modif. 25 (4), 513–545. doi:10.1177/0145445501254003
Wilhelm, F. H., and Roth, W. T. (2001). The somatic symptom paradox in DSM-IV anxiety disorders: Suggestions for a clinical focus in psychophysiology. Biol. Psychol. 57 (1–3), 105–140. doi:10.1016/S0301-0511(01)00091-6
Williams, R. H., Jensen, L. T., Verkhratsky, A., Fugger, L., and Burdakov, D. (2007). Control of hypothalamic orexin neurons by acid and CO2. PNAS 104 (25), 10685–10690. doi:10.1073/pnas.0702676104
Woolley, C., and McEwen, B. (1992). Estradiol mediates fluctuation in hippocampal synapse density during the estrous cycle in the adult rat. J. Neurosci. 12 (7), 2549–2554. doi:10.1523/jneurosci.12-07-02549.1992
Woolley, C. S. (2007). Acute effects of estrogen on neuronal physiology. Annu. Rev. Pharmacol. Toxicol. 47 (1), 657–680. doi:10.1146/annurev.pharmtox.47.120505.105219
World-Health-Organization (1992). The ICD-10 classification of mental and behavioural disorders: Clinical descriptions and diagnostic guidelines. Genève: World-Health-Organization.
Wu, J., Xu, H., Shen, W., and Jiang, C. (2004). Expression and coexpression of CO2-sensitive Kir channels in brainstem neurons of rats. J. Membr. Biol. 197 (3), 179–191. doi:10.1007/s00232-004-0652-4
Yang, C. F., Kim, E. J., Callaway, E. M., and Feldman, J. L. (2020). Monosynaptic projections to excitatory and inhibitory preBötzinger complex neurons. Front. Neuroanat. 14 (58), 58. doi:10.3389/fnana.2020.00058
Zhang, W.-H., Zhang, J.-Y., Holmes, A., and Pan, B.-X. (2021). Amygdala circuit substrates for stress adaptation and adversity. Biol. Psychiatry 89 (9), 847–856. doi:10.1016/j.biopsych.2020.12.026
Keywords: hyperventilation, sex-based differences, control of breathing, orexin, estradiol (17ß-estradiol), maternal separation anxiety
Citation: Kinkead R, Ambrozio-Marques D, Fournier S, Gagnon M and Guay LM (2023) Estrogens, age, and, neonatal stress: panic disorders and novel views on the contribution of non-medullary structures to respiratory control and CO2 responses. Front. Physiol. 14:1183933. doi: 10.3389/fphys.2023.1183933
Received: 10 March 2023; Accepted: 21 April 2023;
Published: 17 May 2023.
Edited by:
George Bradley Richerson, The University of Iowa, United StatesReviewed by:
David D. Kline, University of Missouri, United StatesStefano Bastianini, University of Bologna, Italy
John Wemmie, The University of Iowa, United States
Copyright © 2023 Kinkead, Ambrozio-Marques, Fournier, Gagnon and Guay. This is an open-access article distributed under the terms of the Creative Commons Attribution License (CC BY). The use, distribution or reproduction in other forums is permitted, provided the original author(s) and the copyright owner(s) are credited and that the original publication in this journal is cited, in accordance with accepted academic practice. No use, distribution or reproduction is permitted which does not comply with these terms.
*Correspondence: Richard Kinkead, Richard.Kinkead@fmed.ulaval.ca