- 1Department of Breast Surgery, West China Hospital, Sichuan University, Chengdu, China
- 2Health Management Center, West China Hospital of Sichuan University, Chengdu, Sichuan, China
- 3Department of Rehabilitation Medicine, Key Laboratory of Rehabilitation Medicine, West China Hospital, Sichuan University, Chengdu, Sichuan, China
- 4Institute of Rehabilitation Medicine, West China Hospital, Sichuan University, Chengdu, Sichuan, China
Metabolic defects increase the risk of skeletal muscle diseases, and muscle impairment might worsen metabolic disruption, leading to a vicious cycle. Both brown adipose tissue (BAT) and skeletal muscle play important roles in non-shivering thermogenesis to regulate energy homeostasis. BAT regulates body temperature, systemic metabolism, and seretion of batokines that have positive or negative impacts on skeletal muscle. Conversely, muscle can secrete myokines that regulate BAT function. This review explained the crosstalk between BAT and skeletal muscle, and then discussed the batokines and highlighted their impact on skeletal muscle under physiological conditions. BAT is now considered a potential therapeutic target for obesity and diabetes treatment. Moreover, manipulation of BAT may be an attractive approach for the treatment of muscle weakness by correcting metabolic deficits. Therefore, exploring BAT as a potential treatment for sarcopenia could be a promising avenue for future research.
1 Introduction
Adipose tissue is a vital organ that plays a crutial role in energy metabolism, insulin sensitivity, and energy balance of the body. It consists of two main types: white fat tissue (WAT) and brown fat tissue (BAT). Additionally, skeletal muscle is an endocrine organ that can influence other tissues in the body. While numerous studies have explored the functions of BAT and skeletal muscle in maintaining energy homeostasis, the exact mechanism of the direct crosstalk between BAT and muscle dysfunction remains unclear (Bal et al., 2017).
The interscapular depot may be a prominent site of BAT in rodents, playing a crucial role in systemic energyhomeostasis through thermogenesis (Betz and Enerback, 2018). Brown and beige adipocytes are some of the major sites to catabolize stored energy to generate heat by non-shivering thermogenesis (NST) through uncoupling protein-1 (UCP-1) (Ikeda and Yamada, 2020; Roesler and Kazak, 2020). By taking up excess glucose and lipids to generate heat, activation of BAT can prevent obesity and metabolic abnormalities (Liu et al., 2013a; Yuan et al., 2016; O’Mara et al., 2020; Orava et al., 2011). Furthermore, BAT is a potential endocrine organ, capable of regulating metabolism in distal organs, through secreting of factors in an autocrine/paracrine manner. For instance, BAT can secret insulin, adiponectin, and leptin, which are beneficial for improve muscle mass and preventing muscle weakness.
In this review, we analyze the metabolic mechanism underlying the crosstalk between BAT and skeletal muscle. BAT also functions as a complex and highly dynamic endocrine organ, by releasing signalling molecules, including “batokines,” and bioactive lipids known as “lipokines,” that may positively or negatively sffect signaling in skeletal muscle (Villarroya et al., 2013; Villarroya et al., 2019). Consequenntly, targeting BAT could be a promising approach for treating metabolic disease like obesity and sarcopenia, opening uo a new avenue for further exploration.
2 The metabolic role of brown adipose tissue and skeletal muscle
BAT and skeletal muscle are both crutial for thermogenesis. While BAT was first identified as a thermogenic organ in the 1960s (Pan and Chen, 2021), it was commonly believed that BAT only existed in newborns in humans, with low levels present in adults and no apparent physiological significance. However, recent advances in positron emission computed tomography and/or X-ray tomography (18F-FDG-PET/CT) scanning, combined with molecular identification of localized tissue sampling, have shown that functional BAT does exist in adult humans, although it does decline with age. These findings have challenged the traditional view of BAT in human physiology (Cypess et al., 2009; Virtanen et al., 2009). Moreover, adipocytes in BAT contain multilocular smaller lipid roplets to storage energy, which are also rich mitochondria contains UCP-1. UCP-1 can dissipate the proton gradient of mitochondria and generate heat to maintain temperature of the core body (Pani et al., 2022).
Recent studies have shown that myocytes and brown adipocytes are both derived from mesenchymal stem cells and share similar precursor transcriptomes (Bal et al., 2016; Bal et al., 2017; Scheele and Wolfrum, 2020; Pan and Chen, 2021; Sahu et al., 2023), and both BAT and skeletal muscle use energy substrates including fat and glucose, as fuel to generate heat (Pani et al., 2022). From a metabolic perspective, the thermogenesis of BAT and skeletal muscle is in a dynamic balance. The shivering thermogenesis of skeletal muscle (e.g., skeletal muscular contraction) is responsible for heat generation in humans (Betz and Enerback, 2018), thus it is related to locomotion (Pani et al., 2022). In addition, both BAT and skeletal muscle are important sites of NST in mammals and rodents (Bal et al., 2016; Bal et al., 2017). It is assumed that the presence of active BAT might minimize the importance of muscle based on NST. Skeletal muscle was proved to be important during cold adaptation through research using birds, which lacked BAT (Bal et al., 2016). Furthermore, it is reported that physical exercise may promote fat browning via myokines, leading to thermogenesis of BAT (Rodriguez et al., 2020).
In specific, mitochondrial uncoupling is a process during which substrate oxidation can be uncoupled from ATP production, resulting in heat loss directly. This process is considered a promising target for improving energy expenditure of the whole body (Conley et al., 2007). This is primarily because of the high oxidative capacity of muscle (van den Berg et al., 2011). The mitochondrial protein UCP1 provides the primary molecular driving force for BAT thermogenic power (Virtanen et al., 2009), as a marker of brown adipocytes (Betz and Enerback, 2018). In rodents, thermogenic activity of brown adipocytes is based on the activation of β3 adrenergic receptors (β3ARs) by norepinephrine produced by axons projecting from the hypothalamus. Produced heat spreads quickly throughout the body from the well-vascularized BAT. It is worth noting that controversy does exist, with regard to whether β3AR receptors are the main effectors of BAT stimulation in humans. Riis-Vestergaard et al. (2020) demonstrated β1AR to be the prime receptor responsible for adrenergic regulation of human BAT activity. Heat production is a consequence of fatty acid oxidation that is uncoupled from ATP production by UCP1 on the inner mitochondrial membrane. Energy is lost directly in the form of heat without ATP generation. Thus, fat is consumed by uncoupling fatty acid oxidation from ATP production, generating heat (Cannon and Nedergaard, 2004). Muscle fibers express UCP2 and UCP3, which are similar to UCP1. It is speculated that these proteins can also contribute to NST as uncoupling proteins, but high-level expression of UCP2 and UCP3 cannot compensate for the loss of UCP1 in brown adipocytes in Ucp1−/− mice (Golozoubova et al., 2001; Betz and Enerback, 2018). Fasting can increase levels of UCP2 and UCP3 in skeletal muscle of humans and mice, while acute cold exposure might decrease the UCP3 expression in human muscle (Millet et al., 1997; Schrauwen et al., 2002). It seems that UCP2 and UCP3 can facilitate lipid metabolism and metabolic adaptation instead of uncoupling activity. Interestingly, skeletal muscle can also promote cold-induced thermogenesis through mechanisms unrelated to uncoupling proteins and independent of shivering through calcium cycling by SERCA (Bal et al., 2012). These findings suggest that both NST in skeletal muscle and BAT play an essential role in thermogenesis and energy expenditure, with each complementing the other.
A study proposed that overexpression of UCP1 in mice might increase skeletal muscle mitochondrial uncoupling, energy expenditure of the whole body, and prevent glucose intolerance and high-fat diet induced obesity (Choi et al., 2007). Similarly, overexpression of UCP3 can prevent insulin resistance and high-fat diet induced obesity, which is likely due to non-regulated and non-physiological mitochondrial uncoupling (MacLellan et al., 2005; Choi et al., 2007). It has been have shown that BAT status and increased BAT activity in humans may improve metabolic diseases related to obesity, especially visceral adiposity (Wibmer et al., 2021). Moreover, exposing healthy adults to cold to stimulate BAT activity has been found to improve glucose metabolism and insulin sensitivity (Orava et al., 2011). These findings suggest that BAT has great potential as a therapeutic target for obesity and diabetes treatment. In conclusion, both BAT and skeletal muscle play important roles in energy homeostasis, and utilizing their thermogenesis mechanism can be a promising strategy for treating metabolic disease.
3 How does brown fat thermogenesis affect muscle?
3.1 BAT, metabolism, and skeletal muscle
The role of muscle in energy regulation and glucose metabolism is significant. In muscle dysfunction diseases, glucose uptake is impaired, leading to metabolic dysfunction and a vicious cycle of aggravation (Eknoyan, 1999; Tournissac et al., 2021). It is possible that there is a crosstalk between BAT and skeletal muscle (Figure 1; Table 1). Additionally, stimulation of BAT activity may be beneficial for treating muscle weakness and improving glucose metabolism, which will be discussed in Section 4.
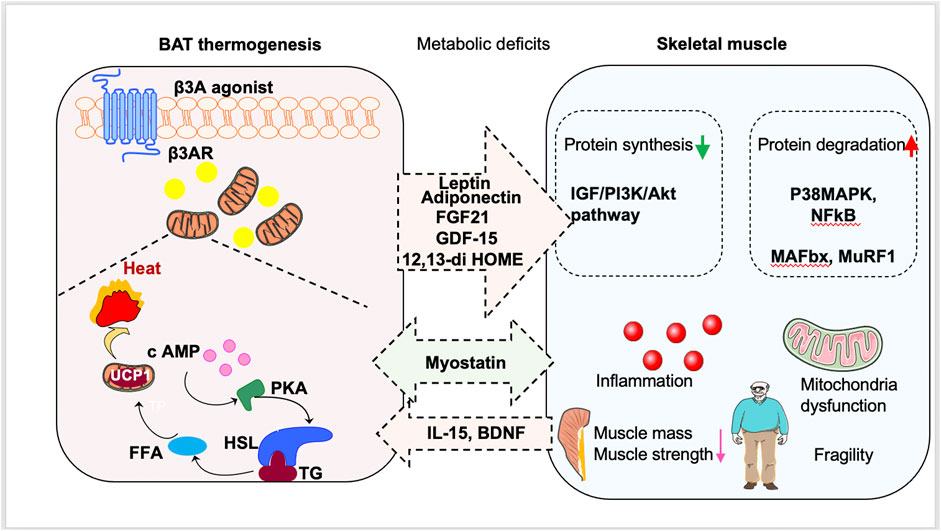
FIGURE 1. Promotion of brown fat tissue (BAT) thermogenesis as a new way to treat sarcopenia. Stimulation of BAT activity might be beneficial for sarcopenia patients by antagonizing metabolic deficits. BAT can also secrete batokines to regulate the occurrence and development of sarcopenia. Moreover, muscle-derived myokines induced by exercise can stimulate browning of white adipose tissue and delay the muscle dysfunction.
3.2 Hormones regulate BAT thermogenesis and their roles in muscle function
3.2.1 Positive regulators of skeletal muscle function
In clinical studies, insulin has been used to treat muscle dysfunction and improve glucose uptake (Jiménez-Osorio et al., 2016). It binds to the insulin receptor (IR) and phosphorylated insulin receptor substrate (IRS), activating the downstream phosphatidylinositol 3-kinase (PI3K)/protein kinase B (Akt) signaling pathway, which promotes glucose uptake in skeletal muscle cells (Jiménez-Osorio et al., 2016). Bouchi et al. (2017) found that patients who received insulin treatment showed higher skeletal muscle index and muscle mass in their lower extremities. A longitudinal study showed that insulin therapy preserved muscle mass but not muscle function as assessed by hand grip strength, suggesting insulin antagonism in patients with T2DM (Ferrari et al., 2020). Thus, insulin treatment may attenuate the progression of sarcopenia in patients with T2DM (Bouchi et al., 2017). BAT is known to regulate insulin signaling. In a mouse model of high-fat diet (HFD)-induced obesity, transplanted BAT significantly attenuated adipose tissue inflammation, reversed body weight and insulin resistance, and improved overall glucose tolerance. In contrast, extirpation of the inter-scapular BAT aggravated obesity, adipose tissue inflammation, and insulin resistance (Shankar et al., 2019). Moreover, insulin may regulate BAT activity. Deficiency of the insulin receptor in BAT results in a decreased thermogenic capacity for BAT and impaired glucose tolerance (Guerra et al., 2001). In diabetic mice, insulin treatment increased UCP1 expression in BAT. Maintenance of normal UCP1 requires both insulin and the sympathetic nervous system, indicating that insulin regulation of BAT thermogenic function involves sympathetic activation (Géloën and Trayhurn, 1990).
In summary, there is a link between insulin signaling and BAT activity. As such, insulin treatment may improve skeletal muscle index and potentially alleviate sarcopenia through the effects of BAT activity. However, this hypothesis requires further investigation to confirm its validity. It is important to note that while insulin has been shown to increase muscle mass in patients with T2DM, it can also increases weight gain, which must be taken into consideration (Sinha et al., 1996; Sallé et al., 2004).
Batokines are factors secreted from metabolically active BAT, and they might provide support for BAT oxidation and coordinate BAT activity with systemic metabolism (Villarroya et al., 2017; Scheele and Wolfrum, 2020).
Adiponectin is an adipokine mainly secreted from white adipose tissue (Choi et al., 2020). But BAT can also secret adiponectin, with a high degree of specificity and a multiple biological functions (Song et al., 2020). Adiponectin has garnered attention for its beneficial effects on muscles. For instance, C2C12 myoblast cells treated with globular adiponectin or a mimetic of globular adiponectin (GTDF) had significantly higher differentiation and fusion indices than vehicle-treated cells (Singh et al., 2017) via downregulation of key genes related to muscle wasting (atrogin-1 and MuRF1) (Singh et al., 2017), and GTDF could also protect rats against muscle wasting due to catabolic stimuli (China et al., 2017). Moreover, adiponectin can increase glucose uptake in muscle cells through mediation of GLUT4 (Yoon et al., 2006). Adiponectin can enhance the oxidation of fatty acids and glucose uptake by stimulating AMPK, contributing to the regulation of glucose and lipid metabolism and regulating the energy homeostasis of organisms (Kadowaki and Yamauchi, 2005; Yoon et al., 2006; Sente et al., 2016; Song et al., 2020). Thus, the therapeutic target for impaired muscle function might be adiponectin. It was shown that myoblast survival and apoptosis are inhibited by adiponectin-driven autophagy, which promotes muscle differentiation (Gamberi et al., 2016). Similarly, the evidence suggested that the levels of autophagy-related genes (e.g., LC3 and beclin-I) were decreased in skeletal muscle of adiponectin knockout mice, along with the decreased myopathic phenotype (Gamberi et al., 2016). In addition, it has been shown that the activation of adiponectin signaling may be protective against muscle wasting by combining with T-cadherin to promote muscle regeneration (Komici et al., 2021). Adiponectin’s insulin-sensitizing effect on muscleincreases the use of fatty acid and glucose resources while simultaneously promoting myogenesis. Furthermore, adiponectin protects against muscle protein degradation by upregulating the IRS-1 signaling pathway (Priego et al., 2021).
Leptin, an adipokine mainly produced by white adipose tissue and BAT, as well as the periosteum and placenta (Obradovic et al., 2021). Skeletal muscle expresses leptin receptors, which can be upregulated due to disuse atrophy (Hamrick et al., 2010). Leptin can have beneficial influences on muscle. For instance, in leptin-deficient ob/ob mice, intraperitoneal administration of leptin corrected decreased skeletal muscle atrophy and mass (Sáinz et al., 2009). Leptin administration can also increase myonectin and myogenin transcript levels, stimulate myocyte proliferation, and reduce the mRNA levels of cytokines associatedwith muscle wasting in ob/ob mice, including MuRF1 and MAFbx (Rodríguez et al., 2015). Moreover, it has been reported that leptin can increase muscle mass by reducing the expression of atrophy-associated factors, including MuRF1, myostatin, and MAFbx, in muscles, indicating that leptin is significantly related to sarcopenia severity and risk (Li et al., 2019). It has also been reported that leptin may play an important role in activating molecular pathways associated with muscle repair and regeneration. Moreover, leptin can also change the expression profile significantly in muscle-derived stem cells (Hamrick et al., 2010). Muscle regeneration may be mediated by leptin indirectly through inhibiting miR-489, and muscle satellite cells can be maintained in a quiescent state. Therefore, leptin is beneficial for improving muscle regeneration and repair (Priego et al., 2021). Leptin is also reported to have a thermogenic effect. Leptin stimulates glucose uptake by BAT, raises BAT temperature (Haque et al., 1999), and positively regulates UCP1 expression in rodent BAT (Sarmiento et al., 1997).
BAT is also a source for lipokines, which are a class of lipids that act as signaling molecules and influences systemic metabolism (Cao et al., 2008; Liu et al., 2013b; Lynes et al., 2017; Stanford et al., 2018). 12,13-dihydroxy-9Z-octadecenoic acid (12,13-diHOME) is an oxylipin, which is released from BAT following cold or exercise, has been reported to increase fatty acid uptake and oxidation in skeletal muscle (Stanford et al., 2018; Macêdo et al., 2022). Stanford et al. (2018) found that an acute bout of exercise increases plasma 12,13-diHOME levels in both humans and mice. Moreover, exercise induces the release of 12,13-diHOME from BAT, acting as an endocrine signal that stimulates fatty acid release for working skeletal muscle, and a possible role in metabolic regulation during exercise (Lynes et al., 2017). Both thermogenesis of muscle and BAT generate heat after exercise. Moreover, treatment of diet-induced obese mice with 12,13-diHOME protects against cold challenge and high-fat diet-induced obesity (Lynes et al., 2017; Shen et al., 2021). These findings suggest that 12,13-diHOME, which is induced by BAT, is involved in metabolic changes triggered by exercise.
3.2.2 Negative regulators of skeletal muscle function
FGF21 is a cold-induced endocrine hormone of BAT (Lee et al., 2014) that is involved in several metabolic conditions, such as weight loss, decreased body fat, and browning of white adipose tissue, which lead to increased energy expenditure (Fisher et al., 2012). Serum FGF21 levels are significantly increased in individuals with T2DM and obesity, which can be deleterious for sarcopenic individuals with physical frailty (Zhang et al., 2008; Chen et al., 2011; Xiao et al., 2012). These contradictory observations suggest that FGF21 may be compensatory factor during disease. The role of FGF21 in muscle is similar to serum. For example, the effect of FGF21 on glucose uptake by myotubes is similar to the effect of insulin (Rosales-Soto et al., 2020), with upregulated FGF21 expression in skeletal muscle protecting against diet-induced obesity and insulin resistance (Kim et al., 2013; Pereira et al., 2017). Others found that greater FGF21 is associated with reduced handgrip strength (Conte et al., 2019), indicating that increased FGF21 acts as an adaptive regulator that counteracts muscle stress imposed by mitochondrial dysfunction. In addition, it is found that FGF21 is also an Akt-regulated myokine which can be secreted by skeletal muscle (Raschke and Eckel, 2013). As a stimulator of Akt1 signalling, resistance training exercise might improve metabolic disorders related to obesity via FGF21 production, promoting WAT browing because of endocrine effects. Thus, FGF21 is potential to be bidirectional cross-talk between BAT and skeletal muscle (Rodriguez et al., 2020).
In addition to FGF21, thermogenic BAT is also an important physiological source for GDF15 (Flicker et al., 2019). GDF15 has shown potential as a blood biomarker for human mitochondrial disorders (Fujita et al., 2020). A variety of mouse muscle models have demonstrated increased GDF15 expression in muscle, as well as increased circulating GDF15 levels during mitochondrial stress (Khan et al., 2017; Morrow et al., 2017; Ost et al., 2020; Poulsen et al., 2020). Overexpression of GDF15 in muscle reduces local muscle mass, indicating that GDF15 can lead to muscle atrophy (Conte et al., 2019; Johann et al., 2021), which is more closely related to muscle function and strength than muscle mass (Kim et al., 2020). Tang demonstrated mTORC1 activation increased the expression of GDF15 by phosphorylation of STAT3, inducing oxidative stress and catabolic changes. Blocking mTORC1 in aging mice downregulates the expression of GDF15 and STAT3’s phosphorylation in skeletal muscle, decreasing oxidative stress and muscle fiber damage and loss. These results suggest that increased GDF15 signaling may contribute to age-related muscle atrophy induced by chronically increased mTORC1 activity (Tang et al., 2019).
3.3 Exerkines and their roles in BAT function
3.3.1 Negative regulators of BAT
Myostatin is a protein secreted by skeletal and cardiac muscles (McPherron et al., 1997) that inhibits skeletal muscle growth (McPherron et al., 1997). Myostatin can induce oxidative stress and produce ROS in skeletal muscle cells, leading to muscle wasting during sarcopenia (Sriram et al., 2011). In addition, myostatin is negatively associated with the thermogenic capacity of BAT and the browning of WAT (Braga et al., 2013; Shan et al., 2013). Kong noted that BAT controls skeletal muscle function by secreting myostatin (Kong et al., 2018). A loss of interferon regulatory factor 4 (IRF4) in BAT, which was previously recognized as a regulator of adipogenesis by the same group (Eguchi et al., 2008), resulted in increased myogenic gene expression in BAT and myostatin secretion, contributing to reduced mitochondrial function and impaired exercise capacity (Kong et al., 2018). Mice overexpressing IRF4 in BAT showed reduced serum myostatin and improved running ability compared to wild-type mice (Kong et al., 2018). Furthermore, thermo-neutral temperature increases myostatin levels in murine BAT, leading to reduced exercise capacity (Kong et al., 2018). These findings suggest that myostatin plays a dual role as a negative regulator of BAT and a regulator of skeletal muscle function as a batokine produced by BAT.
3.3.2 Positive regulators of BAT
IL-15 has been shown to play a critical role in the regulation of BAT metabolism and thermogenesis (Almendro et al., 2008). It has been found to increase BAT activity and induce browning of white adipose tissue (WAT) by activating the expression of brown fat-specific genes such as UCP1, PGC-1α, and PRDM16. IL-15 also promotes the proliferation and differentiation of brown adipocyte precursor cells, resulting in more mature brown adipocytes in BAT (Duan et al., 2017). These effects of IL-15 on BAT have been shown to enhance energy expenditure and improve metabolic health, making it a potential therapeutic target for obesity and related metabolic disorders.
Cold-induced BDNF has been found to play a significant role in the regulation of BAT. Specifically, in BAT, BDNF increases Ucp1 mRNA level and UCP1 protein expression (Wang et al., 2007), and enhances thermogenesis (An et al., 2015). Studies have shown that cold exposure induces the expression of BDNF in BAT, which in turn activates the sympathetic nervous system and stimulates BAT thermogenesis. This effect is mediated by the TrkB receptor, which is expressed in BAT and binds to BDNF to promote thermogenic activity. Furthermore, BDNF has been found to enhance the browning of white adipose tissue, which makes it more metabolically active and similar to BAT. Therefore, cold-induced BDNF has potential therapeutic benefits for treating metabolic disorders by promoting BAT activity and increasing energy expenditure (An et al., 2015; Zhu et al., 2019).
In summary, these results imply that batokines produced by BAT, including adiponectin and leptin, play a positive role in skeletal muscle function. On the other hand, FGF21 and GDF15 can lead to muscle atrophy directly or indirectly through metabolism process in BAT. Moreover, myostatin, IL-15 and BNDF can also be secreted by skeletal muscle to positively or negatively regulate BAT.
4 Targeting BAT may be a treatment method for muscle weakness
4.1 Pharmacological tools that stimulate thermogenesis
β3-ARs are present in adipose tissue and β3-AR agonists are linked to increased BAT activity and the formation of brown adipocytes in subcutaneous WAT (Finlin et al., 2018). Although no clinical trials have tested β3-AR agonists in sarcopenia, studies have shown that they can improve metabolic disorders such as T2DM and obesity in both animals and humans. Only a few studies have reported the effects of β3-AR agonists on muscle. For example, Kern used the 3-AR agonist, mirabegron (50 mg/day, for 12 weeks), to treat obesity in insulin-resistant humans and found an increase in type I fibers within skeletal muscle and numerous positive effects on skeletal muscle gene expression (Finlin et al., 2020). In line with clinical results, amibegron, a β3-AR agonist induces fibro-adipogenic progenitor–BAT differentiation, improving muscle quality and shoulder function in mice with rotator cuff tears (Wang et al., 2020; Wang et al., 2021). While it is possible that β3-AR agonists directly improve muscle function, it is unclear whether they are useful in treating sarcopenia. Future clinical trials should investigate the potential benefits of such drugs At various stages of sarcopenia.
Several alternative treatments have been proposed to boost BAT activity, including GLP-1R and DPP4i (Beiroa et al., 2014; Takeda et al., 2018). Ogawa found that older diabetic patients treated with DPP4 inhibitors had a reduced risk for affixed skeletal muscle loss (Bouchi et al., 2018). Additionally, treatment with GLP-1 RA (Liraglutide, 3.0 mg per day for 24 weeks) is well tolerated, aids in fat mass loss, reduces android fat mass, and raises skeletal muscle index in T2DM patients (Perna et al., 2016).
Further, transient receptor potential (TRP) channels, such as transient receptor potential melastatin 8 (TRPM8), a calcium-gated channel, can be activated by heat and cold and indirectly stimulate BAT thermogenesis. In the murine peripheral nervous system, the TRPM8 channel severs as the primary molecular transducer for cold sensation (Bautista et al., 2007). Menthol administration activates TRPM8, resulting in elevated UCP-1 levels, enhanced temperature in humans and mice, and protection against obesity. Through TRPM8-mediated PGC1 upregulation in skeletal muscles, dietary menthol improves exercise endurance and energy metabolism, thereby enhancing muscle function (Li et al., 2018).
As a whole, these results suggest that indirect stimulation of BAT thermogenesis may be an effective strategy for muscle function enhancement. Future preclinical and clinical trials are necessary to investigate both direct and indirect methods of stimulating BAT thermogenesis in sarcopenic animals and humans.
4.2 Other pathways to regulate muscle atrophy through BAT
The adenosine A2B receptor is the most highly expressed Gs-coupled G protein-coupled receptor (GPCR) to both skeletal muscle and BAT (Gnad et al., 2020). A study showed that the extracellular nucleoside adenosine playings a vital role in BAT function, and that the A2B receptor could activate energy expenditure and induce the release of the “batokine” FGF21 (Gnad et al., 2014). Additionally, A2B receptor activation has been shown to reduce diet-induced obesity. Moreover, the A2B stimulation counteracted the effects of aging on both BAT and skeletal muscle (Gnad et al., 2020).
5 Conclusion
Increasing BAT activity may restore thermoregulation, improve metabolic parameters, and possibly antagonize key facets of muscle dysfunction. On the other hand, BAT is an endocrine organ secreteing batokines, including adiponectin, leptin, FGF21, and GDF-15, to regulate the skeletal muscle. Myostatin is the most likely link between sarcopenia and BAT. Therefore, BAT may protect against metabolic deficits that are risk factors for skeletal muscle disease by regulation of homeostatic hormones directly and indirectly. Future research should focus on the role of BAT in muscle diseases such as sarcopenia with targeted promotion of BAT thermogenesis—a possible new means.
Author contributions
TW and YC concepted the study, YC and QH drafted the manuscript.
Funding
Supported by the National Natural Science Foundation (82102656); China Postdoctoral Science Foundation (2022T150452, 2021M692299); PostDoctor Research Project, West China Hospital, Sichuan University (2021HXBH021, 2021HXBH052). Natural Science Foundation of Sichuan Province (2022NSFSC1392, 2022NSFSC1236, 2023NSFSC1580).
Acknowledgments
We thank International Science Editing (http://www.internationalscienceediting.com) for editing this manuscript.
Conflict of interest
The authors declare that the research was conducted in the absence of any commercial or financial relationships that could be construed as a potential conflict of interest.
Publisher’s note
All claims expressed in this article are solely those of the authors and do not necessarily represent those of their affiliated organizations, or those of the publisher, the editors and the reviewers. Any product that may be evaluated in this article, or claim that may be made by its manufacturer, is not guaranteed or endorsed by the publisher.
Abbreviations
β3AR, β3 adrenergic receptors; AdipoRs, adiponectin receptors; AMPK, adenosine monophosphate-activated protein kinase; BAT, Brown adipose tissue; DAGs, diacylglycerols; DPP4i, dipeptidyl peptidase IV inhibitor; GLP-1RA, glucose-like peptide-1 receptor agonist; HFD, high-fat diet; IMAT, intramuscular adipose tissue; IMCLs, intramyocellular lipids; IR, Insulin receptor; IRS, insulin receptor substrate; LD, lipid droplet; MAPK, mitogen-activated protein kinase; NRF1, nuclear respiratory factor 1; PPARα, PPAR alpha; ROS, reactive oxygen species; T2DM, type 2 diabetes mellitus; TGs, triglycerides; TRP, transient receptor potential; TRPM8, transient receptor potential melastatin 8; UCP1, uncoupling protein 1.
References
Almendro, V., Fuster, G., Busquets, S., Ametller, E., Figueras, M., Argilés, J. M., et al. (2008). Effects of IL-15 on rat Brown adipose tissue: Uncoupling proteins and PPARs. Obes. (Silver Spring, Md.) 16 (2), 285–289. doi:10.1038/oby.2007.47
An, J. J., Liao, G. Y., Kinney, C. E., Sahibzada, N., and Xu, B. (2015). Discrete BDNF neurons in the paraventricular hypothalamus control feeding and energy expenditure. Cell. Metab. 22 (1), 175–188. doi:10.1016/j.cmet.2015.05.008
Bal, N. C., Maurya, S. K., Sopariwala, D. H., Sahoo, S. K., Gupta, S. C., Shaikh, S. A., et al. (2012). Sarcolipin is a newly identified regulator of muscle-based thermogenesis in mammals. Nat. Med. 18 (10), 1575–1579. doi:10.1038/nm.2897
Bal, N. C., Maurya, S. K., Singh, S., Wehrens, X. H. T., and Periasamy, M. (2016). Increased reliance on muscle-based thermogenesis upon acute minimization of Brown adipose tissue function. J. Biol. Chem. 291 (33), 17247–17257. doi:10.1074/jbc.M116.728188
Bal, N. C., Singh, S., Reis, F. C. G., Maurya, S. K., Pani, S., Rowland, L. A., et al. (2017). Both Brown adipose tissue and skeletal muscle thermogenesis processes are activated during mild to severe cold adaptation in mice. J. Biol. Chem. 292 (40), 16616–16625. doi:10.1074/jbc.M117.790451
Bautista, D. M., Siemens, J., Glazer, J. M., Tsuruda, P. R., Basbaum, A. I., Stucky, C. L., et al. (2007). The menthol receptor TRPM8 is the principal detector of environmental cold. Nature 448 (7150), 204–208. doi:10.1038/nature05910
Beiroa, D., Imbernon, M., Gallego, R., Senra, A., Herranz, D., Villarroya, F., et al. (2014). GLP-1 agonism stimulates Brown adipose tissue thermogenesis and browning through hypothalamic AMPK. Diabetes 63 (10), 3346–3358. doi:10.2337/db14-0302
Betz, M. J., and Enerback, S. (2018). Targeting thermogenesis in Brown fat and muscle to treat obesity and metabolic disease. Nat. Rev. Endocrinol. 14 (2), 77–87. doi:10.1038/nrendo.2017.132
Bouchi, R., Fukuda, T., Takeuchi, T., Nakano, Y., Murakami, M., Minami, I., et al. (2017). Insulin treatment attenuates decline of muscle mass in Japanese patients with type 2 diabetes. Calcif. Tissue Int. 101 (1), 1–8. doi:10.1007/s00223-017-0251-x
Bouchi, R., Fukuda, T., Takeuchi, T., Nakano, Y., Murakami, M., Minami, I., et al. (2018). Dipeptidyl peptidase 4 inhibitors attenuates the decline of skeletal muscle mass in patients with type 2 diabetes. Diabetes Metab. Res. Rev. 34 (2), e2957. doi:10.1002/dmrr.2957
Braga, M., Pervin, S., Norris, K., Bhasin, S., and Singh, R. (2013). Inhibition of in vitro and in vivo Brown fat differentiation program by myostatin. Obesity 21, 1180–1188. doi:10.1002/oby.20117
Cannon, B., and Nedergaard, J. (2004). Brown adipose tissue: Function and physiological significance. Physiol. Rev. 84 (1), 277–359. doi:10.1152/physrev.00015.2003
Cao, H., Gerhold, K., Mayers, J. R., Wiest, M. M., Watkins, S. M., and Hotamisligil, G. S. (2008). Identification of a lipokine, a lipid hormone linking adipose tissue to systemic metabolism. Cell. 134 (6), 933–944. doi:10.1016/j.cell.2008.07.048
Chen, C., Cheung, B. M. Y., Tso, A. W. K., Wang, Y., Law, L. S. C., Ong, K. L., et al. (2011). High plasma level of fibroblast growth factor 21 is an independent predictor of type 2 diabetes: A 5.4-year population-based prospective study in Chinese subjects. Diabetes Care 34 (9), 2113–2115. doi:10.2337/dc11-0294
China, S. P., Pal, S., Chattopadhyay, S., Porwal, K., Kushwaha, S., Bhattacharyya, S., et al. (2017). Globular adiponectin reverses osteo-sarcopenia and altered body composition in ovariectomized rats. Bone 105, 75–86. doi:10.1016/j.bone.2017.08.005
Choi, C. S., Fillmore, J. J., Kim, J. K., Liu, Z. X., Kim, S., Collier, E. F., et al. (2007). Overexpression of uncoupling protein 3 in skeletal muscle protects against fat-induced insulin resistance. J. Clin. Investigation 117 (7), 1995–2003. doi:10.1172/JCI13579
Choi, H. M., Doss, H. M., and Kim, K. S. (2020). Multifaceted physiological roles of adiponectin in inflammation and diseases. Int. J. Mol. Sci. 21 (4), 1219. doi:10.3390/ijms21041219
Conley, K. E., Jubrias, S. A., Amara, C. E., and Marcinek, D. J. (2007). Mitochondrial dysfunction: Impact on exercise performance and cellular aging. Exerc. Sport Sci. Rev. 35 (2), 43–49. doi:10.1249/JES.0b013e31803e88e9
Conte, M., Ostan, R., Fabbri, C., Santoro, A., Guidarelli, G., Vitale, G., et al. (2019). Human aging and longevity are characterized by high levels of mitokines. J. Gerontol. A Biol. Sci. Med. Sci. 74 (5), 600–607. doi:10.1093/gerona/gly153
Cypess, A. M., Lehman, S., Williams, G., Tal, I., Rodman, D., Goldfine, A. B., et al. (2009). Identification and importance of Brown adipose tissue in adult humans. N. Engl. J. Med. 360 (15), 1509–1517. doi:10.1056/NEJMoa0810780
Duan, Y., Li, F., Wang, W., Guo, Q., Wen, C., Li, Y., et al. (2017). Interleukin-15 in obesity and metabolic dysfunction: Current understanding and future perspectives. Obes. Rev. 18 (10), 1147–1158. doi:10.1111/obr.12567
Eguchi, J., Yan, Q. W., Schones, D. E., Kamal, M., Hsu, C. H., Zhang, M. Q., et al. (2008). Interferon regulatory factors are transcriptional regulators of adipogenesis. Cell. Metab. 7 (1), 86–94. doi:10.1016/j.cmet.2007.11.002
Eknoyan, G. (1999). Santorio Sanctorius (1561-1636) - founding father of metabolic balance studies. Am. J. Nephrol. 19 (2), 226–233. doi:10.1159/000013455
Ferrari, U., Then, C., Rottenkolber, M., Selte, C., Seissler, J., Conzade, R., et al. (2020). Longitudinal association of type 2 diabetes and insulin therapy with muscle parameters in the KORA-Age study. Acta Diabetol. 57 (9), 1057–1063. doi:10.1007/s00592-020-01523-7
Finlin, B. S., Memetimin, H., Confides, A. L., Kasza, I., Zhu, B., Vekaria, H. J., et al. (2018). Human adipose beiging in response to cold and mirabegron. JCI Insight 3 (15), e121510. doi:10.1172/jci.insight.121510
Finlin, B. S., Memetimin, H., Zhu, B., Confides, A. L., Vekaria, H. J., El Khouli, R. H., et al. (2020). The β3-adrenergic receptor agonist mirabegron improves glucose homeostasis in obese humans. J. Clin. Invest. 130 (5), 2319–2331. doi:10.1172/JCI134892
Fisher, F. M., Kleiner, S., Douris, N., Fox, E. C., Mepani, R. J., Verdeguer, F., et al. (2012). FGF21 regulates PGC-1α and browning of white adipose tissues in adaptive thermogenesis. Genes. Dev. 26 (3), 271–281. doi:10.1101/gad.177857.111
Flicker, D., Sancak, Y., Mick, E., Goldberger, O., and Mootha, V. K. (2019). Exploring the in vivo role of the mitochondrial calcium uniporter in Brown fat bioenergetics. Cell. Rep. 27 (5), 1364–1375. doi:10.1016/j.celrep.2019.04.013
Fujita, Y., Ito, M., and Ohsawa, I. (2020). Mitochondrial stress and GDF15 in the pathophysiology of sepsis. Archives Biochem. Biophysics 696, 108668. doi:10.1016/j.abb.2020.108668
Gamberi, T., Modesti, A., Magherini, F., D'Souza, D. M., Hawke, T., and Fiaschi, T. (2016). Activation of autophagy by globular adiponectin is required for muscle differentiation. Biochim. Biophys. Acta 1863 (4), 694–702. doi:10.1016/j.bbamcr.2016.01.016
Géloën, A., and Trayhurn, P. (1990). Regulation of the level of uncoupling protein in Brown adipose tissue by insulin requires the mediation of the sympathetic nervous system. FEBS Lett. 267 (2), 265–267. doi:10.1016/0014-5793(90)80941-b
Gnad, T., Scheibler, S., von Kügelgen, I., Scheele, C., Kilić, A., Glöde, A., et al. (2014). Adenosine activates Brown adipose tissue and recruits beige adipocytes via A2A receptors. Nature 516 (7531), 395–399. doi:10.1038/nature13816
Gnad, T., Navarro, G., Lahesmaa, M., Reverte-Salisa, L., Copperi, F., Cordomi, A., et al. (2020). Adenosine/A2B receptor signaling ameliorates the effects of aging and counteracts obesity. Cell. Metab. 32 (1), 56–70. doi:10.1016/j.cmet.2020.06.006
Golozoubova, V., Hohtola, E., Matthias, A., Jacobsson, A., Cannon, B., and Nedergaard, J. (2001). Only UCP1 can mediate adaptive nonshivering thermogenesis in the cold. Faseb J. 15 (11), 2048–2050. doi:10.1096/fj.00-0536fje
Guerra, C., Navarro, P., Valverde, A. M., Arribas, M., Brüning, J., Kozak, L. P., et al. (2001). Brown adipose tissue-specific insulin receptor knockout shows diabetic phenotype without insulin resistance. J. Clin. Invest. 108 (8), 1205–1213. doi:10.1172/JCI13103
Hamrick, M. W., Herberg, S., Arounleut, P., He, H. Z., Shiver, A., Qi, R. Q., et al. (2010). The adipokine leptin increases skeletal muscle mass and significantly alters skeletal muscle miRNA expression profile in aged mice. Biochem. Biophys. Res. Commun. 400 (3), 379–383. doi:10.1016/j.bbrc.2010.08.079
Haque, M. S., Minokoshi, Y., Hamai, M., Iwai, M., Horiuchi, M., and Shimazu, T. (1999). Role of the sympathetic nervous system and insulin in enhancing glucose uptake in peripheral tissues after intrahypothalamic injection of leptin in rats. Diabetes 48 (9), 1706–1712. doi:10.2337/diabetes.48.9.1706
Ikeda, K., and Yamada, T. (2020). UCP1 dependent and independent thermogenesis in Brown and beige adipocytes. Front. Endocrinol. 11, 498. doi:10.3389/fendo.2020.00498
Jiménez-Osorio, A. S., Monroy, A., and Alavez, S. (2016). Curcumin and insulin resistance-Molecular targets and clinical evidences. Biofactors 42 (6), 561–580. doi:10.1002/biof.1302
Johann, K., Kleinert, M., and Klaus, S. (2021). The role of GDF15 as a myomitokine. Cells 10 (11), 2990. doi:10.3390/cells10112990
Kadowaki, T., and Yamauchi, T. (2005). Adiponectin and adiponectin receptors. Endocr. Rev. 26 (3), 439–451. doi:10.1210/er.2005-0005
Khan, N. A., Nikkanen, J., Yatsuga, S., Jackson, C., Wang, L., Pradhan, S., et al. (2017). mTORC1 regulates mitochondrial integrated stress response and mitochondrial myopathy progression. Cell. Metab. 26 (2), 419–428. doi:10.1016/j.cmet.2017.07.007
Kim, K. H., Jeong, Y. T., Oh, H., Kim, S. H., Cho, J. M., Kim, Y. N., et al. (2013). Autophagy deficiency leads to protection from obesity and insulin resistance by inducing Fgf21 as a mitokine. Nat. Med. 19 (1), 83–92. doi:10.1038/nm.3014
Kim, H., Kim, K. M., Kang, M. J., and Lim, S. (2020). Growth differentiation factor-15 as a biomarker for sarcopenia in aging humans and mice. Exp. Gerontol. 142, 111115. doi:10.1016/j.exger.2020.111115
Komici, K., Dello Iacono, A., De Luca, A., Perrotta, F., Bencivenga, L., Rengo, G., et al. (2021). Adiponectin and sarcopenia: A systematic review with meta-analysis. Front. Endocrinol. (Lausanne) 12, 576619. doi:10.3389/fendo.2021.576619
Kong, X., Yao, T., Zhou, P., Kazak, L., Tenen, D., Lyubetskaya, A., et al. (2018). Brown adipose tissue controls skeletal muscle function via the secretion of myostatin. Cell. Metab. 28 (4), 631–643. doi:10.1016/j.cmet.2018.07.004
Lee, P., Linderman, J. D., Smith, S., Brychta, R. J., Wang, J., Idelson, C., et al. (2014). Irisin and FGF21 are cold-induced endocrine activators of Brown fat function in humans. Cell. Metab. 19 (2), 302–309. doi:10.1016/j.cmet.2013.12.017
Li, C., Li, J., Xiong, X., Liu, Y., Lv, Y., Qin, S., et al. (2018). TRPM8 activation improves energy expenditure in skeletal muscle and exercise endurance in mice. Gene 641, 111–116. doi:10.1016/j.gene.2017.10.045
Li, C. W., Yu, K., Shyh-Chang, N., Li, G. X., Jiang, L. J., Yu, S. L., et al. (2019). Circulating factors associated with sarcopenia during ageing and after intensive lifestyle intervention. J. Cachexia Sarcopenia Muscle 10 (3), 586–600. doi:10.1002/jcsm.12417
Liu, X., Zheng, Z., Zhu, X., Meng, M., Li, L., Shen, Y., et al. (2013). Brown adipose tissue transplantation improves whole-body energy metabolism. Cell. Res. 23 (6), 851–854. doi:10.1038/cr.2013.64
Liu, S., Brown, J. D., Stanya, K. J., Homan, E., Leidl, M., Inouye, K., et al. (2013). A diurnal serum lipid integrates hepatic lipogenesis and peripheral fatty acid use. Nature 502 (7472), 550–554. doi:10.1038/nature12710
Lynes, M. D., Leiria, L. O., Lundh, M., Bartelt, A., Shamsi, F., Huang, T. L., et al. (2017). The cold-induced lipokine 12,13-diHOME promotes fatty acid transport into Brown adipose tissue. Nat. Med. 23 (5), 631–637. doi:10.1038/nm.4297
Macêdo, A. P. A., Muñoz, V. R., Cintra, D. E., and Pauli, J. R. (2022). 12,13-diHOME as a new therapeutic target for metabolic diseases. Life Sci. 290, 120229. doi:10.1016/j.lfs.2021.120229
MacLellan, J. D., Gerrits, M. F., Gowing, A., Smith, P. J. S., Wheeler, M. B., and Harper, M. E. (2005). Physiological increases in uncoupling protein 3 augment fatty acid oxidation and decrease reactive oxygen species production without uncoupling respiration in muscle cells. Diabetes 54 (8), 2343–2350. doi:10.2337/diabetes.54.8.2343
McPherron, A. C., Lawler, A. M., and Lee, S. J. (1997). Regulation of skeletal muscle mass in mice by a new TGF-beta superfamily member. Nature 387 (6628), 83–90. doi:10.1038/387083a0
Millet, L., Vidal, H., Andreelli, F., Larrouy, D., Riou, J. P., Ricquier, D., et al. (1997). Increased uncoupling protein-2 and -3 mRNA expression during fasting in obese and lean humans. J. Clin. Investigation 100 (11), 2665–2670. doi:10.1172/JCI119811
Morrow, R. M., Picard, M., Derbeneva, O., Leipzig, J., McManus, M. J., Gouspillou, G., et al. (2017). Mitochondrial energy deficiency leads to hyperproliferation of skeletal muscle mitochondria and enhanced insulin sensitivity. Proc. Natl. Acad. Sci. U. S. A. 114 (10), 2705–2710. doi:10.1073/pnas.1700997114
Obradovic, M., Sudar-Milovanovic, E., Soskic, S., Essack, M., Arya, S., Stewart, A. J., et al. (2021). Leptin and obesity: Role and clinical implication. Front. Endocrinol. 12, 585887. doi:10.3389/fendo.2021.585887
O'Mara, A. E., Johnson, J. W., Linderman, J. D., Brychta, R. J., McGehee, S., Fletcher, L. A., et al. (2020). Chronic mirabegron treatment increases human Brown fat, HDL cholesterol, and insulin sensitivity. J. Clin. Invest. 130 (5), 2209–2219. doi:10.1172/JCI131126
Orava, J., Nuutila, P., Lidell, M. E., Oikonen, V., Noponen, T., Viljanen, T., et al. (2011). Different metabolic responses of human Brown adipose tissue to activation by cold and insulin. Cell. Metab. 14 (2), 272–279. doi:10.1016/j.cmet.2011.06.012
Ost, M., Igual Gil, C., Coleman, V., Keipert, S., Efstathiou, S., Vidic, V., et al. (2020). Muscle-derived GDF15 drives diurnal anorexia and systemic metabolic remodeling during mitochondrial stress. EMBO Rep. 21 (3), e48804. doi:10.15252/embr.201948804
Pan, R., and Chen, Y. (2021). Management of oxidative stress: Crosstalk between Brown/beige adipose tissues and skeletal muscles. Front. Physiol. 12, 712372. doi:10.3389/fphys.2021.712372
Pani, S., Dey, S., Pati, B., Senapati, U., and Bal, N. C. (2022). Brown to white fat transition overlap with skeletal muscle during development of larger mammals: Is it a coincidence? J. Endocr. Soc. 6 (12), bvac151. doi:10.1210/jendso/bvac151
Pereira, R. O., Tadinada, S. M., Zasadny, F. M., Oliveira, K. J., Pires, K. M. P., Olvera, A., et al. (2017). OPA1 deficiency promotes secretion of FGF21 from muscle that prevents obesity and insulin resistance. Embo J. 36 (14), 2126–2145. doi:10.15252/embj.201696179
Perna, S., Guido, D., Bologna, C., Solerte, S. B., Guerriero, F., Isu, A., et al. (2016). Liraglutide and obesity in elderly: Efficacy in fat loss and safety in order to prevent sarcopenia. A perspective case series study. Aging Clin. Exp. Res. 28 (6), 1251–1257. doi:10.1007/s40520-015-0525-y
Poulsen, N. S., Madsen, K. L., Hornsyld, T. M., Eisum, A. S. V., Fornander, F., Buch, A. E., et al. (2020). Growth and differentiation factor 15 as a biomarker for mitochondrial myopathy. Mitochondrion 50, 35–41. doi:10.1016/j.mito.2019.10.005
Priego, T., Martín, A. I., González-Hedström, D., Granado, M., and López-Calderón, A. (2021). Role of hormones in sarcopenia. Vitam. Horm. 115, 535–570. doi:10.1016/bs.vh.2020.12.021
Raschke, S., and Eckel, J. (2013). Adipo-myokines: Two sides of the same coin--mediators of inflammation and mediators of exercise. Mediat. Inflamm. 2013, 320724. doi:10.1155/2013/320724
Riis-Vestergaard, M. J., Richelsen, B., Bruun, J. M., Li, W., Hansen, J. B., and Pedersen, S. B. (2020). Beta-1 and not beta-3 adrenergic receptors may Be the primary regulator of human Brown adipocyte metabolism. J. Clin. Endocrinol. Metab. 105 (4), dgz298. doi:10.1210/clinem/dgz298
Rodríguez, A., Becerril, S., Méndez-Giménez, L., Ramírez, B., Sáinz, N., Catalán, V., et al. (2015). Leptin administration activates irisin-induced myogenesis via nitric oxide-dependent mechanisms, but reduces its effect on subcutaneous fat browning in mice. Int. J. Obes. (Lond) 39 (3), 397–407. doi:10.1038/ijo.2014.166
Rodriguez, A., Catalán, V., Ramírez, B., Unamuno, X., Portincasa, P., Gómez-Ambrosi, J., et al. (2020). Impact of adipokines and myokines on fat browning. J. Physiol. Biochem. 76 (2), 227–240. doi:10.1007/s13105-020-00736-2
Roesler, A., and Kazak, L. (2020). UCP1-independent thermogenesis. Biochem. J. 477 (3), 709–725. doi:10.1042/BCJ20190463
Rosales-Soto, G., Diaz-Vegas, A., Casas, M., Contreras-Ferrat, A., and Jaimovich, E. (2020). Fibroblast growth factor-21 potentiates glucose transport in skeletal muscle fibers. J. Mol. Endocrinol. 65, 85–95. doi:10.1530/JME-19-0210
Sahu, B., Tikoo, O., Pati, B., Senapati, U., and Bal, N. C. (2023). Role of distinct fat depots in metabolic regulation and pathological implications. Rev. Physiol. Biochem. Pharmacol. 186, 135–176. doi:10.1007/112_2022_73
Sáinz, N., Rodríguez, A., Catalán, V., Becerril, S., Ramírez, B., Gómez-Ambrosi, J., et al. (2009). Leptin administration favors muscle mass accretion by decreasing FoxO3a and increasing PGC-1alpha in ob/ob mice. PLoS One 4 (9), e6808. doi:10.1371/journal.pone.0006808
Sallé, A., Guilloteau, G., Ryan, M., Bouhanick, B., and Ritz, P. (2004). Effect of insulin treatment on the body composition of Type 2 diabetic patients. Diabet. Med. 21 (12), 1298–1303. doi:10.1111/j.1464-5491.2004.01335.x
Sarmiento, U., Benson, B., Kaufman, S., Ross, L., Qi, M., Scully, S., et al. (1997). Morphologic and molecular changes induced by recombinant human leptin in the white and Brown adipose tissues of C57BL/6 mice. Lab. Invest. 77 (3), 243–256.
Scheele, C., and Wolfrum, C. (2020). Brown adipose crosstalk in tissue plasticity and human metabolism. Endocr. Rev. 41 (1), 53–65. doi:10.1210/endrev/bnz007
Schrauwen, P., Westerterp-Plantenga, M. S., Kornips, E., Schaart, G., and van Marken Lichtenbelt, W. D. (2002). The effect of mild cold exposure on UCP3 mRNA expression and UCP3 protein content in humans. Int. J. Obes. Relat. Metabolic Disord. J. Int. Assoc. Study Obes. 26 (4), 450–457. doi:10.1038/sj.ijo.0801943
Sente, T., Van Berendoncks, A. M., Hoymans, V. Y., and Vrints, C. J. (2016). Adiponectin resistance in skeletal muscle: Pathophysiological implications in chronic heart failure. J. Cachexia Sarcopenia Muscle 7 (3), 261–274. doi:10.1002/jcsm.12086
Shan, T., Liang, X., Bi, P., and Kuang, S. (2013). Myostatin knockout drives browning of white adipose tissue through activating the AMPK-PGC1α-Fndc5 pathway in muscle. FASEB J. 27, 1981–1989. doi:10.1096/fj.12-225755
Shankar, K., Kumar, D., Gupta, S., Varshney, S., Rajan, S., Srivastava, A., et al. (2019). Role of Brown adipose tissue in modulating adipose tissue inflammation and insulin resistance in high-fat diet fed mice. Eur. J. Pharmacol. 854, 354–364. doi:10.1016/j.ejphar.2019.02.044
Shen, C. L., Ramamoorthy, S., Kaur, G., Dufour, J. M., Wang, R., Mo, H., et al. (2021). Dietary annatto-extracted tocotrienol reduces inflammation and oxidative stress, and improves macronutrient metabolism in obese mice: A metabolic profiling study. Nutrients 13 (4), 1267. doi:10.3390/nu13041267
Singh, A. K., Shree, S., Chattopadhyay, S., Kumar, S., Gurjar, A., Kushwaha, S., et al. (2017). Small molecule adiponectin receptor agonist GTDF protects against skeletal muscle atrophy. Mol. Cell. Endocrinol. 439, 273–285. doi:10.1016/j.mce.2016.09.013
Sinha, A., Formica, C., Tsalamandris, C., Panagiotopoulos, S., Hendrich, E., DeLuise, M., et al. (1996). Effects of insulin on body composition in patients with insulin-dependent and non-insulin-dependent diabetes. Diabet. Med. 13 (1), 40–46. doi:10.1002/(SICI)1096-9136(199601)13:1<40::AID-DIA991>3.0.CO;2-U
Song, A., Dai, W., Jang, M. J., Medrano, L., Li, Z., Zhao, H., et al. (2020). Low- and high-thermogenic Brown adipocyte subpopulations coexist in murine adipose tissue. J. Clin. Invest. 130 (1), 247–257. doi:10.1172/JCI129167
Sriram, S., Subramanian, S., Sathiakumar, D., Venkatesh, R., Salerno, M. S., McFarlane, C. D., et al. (2011). Modulation of reactive oxygen species in skeletal muscle by myostatin is mediated through NF-κB. Aging Cell. 10 (6), 931–948. doi:10.1111/j.1474-9726.2011.00734.x
Stanford, K. I., Lynes, M. D., Takahashi, H., Baer, L. A., Arts, P. J., May, F. J., et al. (2018). 12,13-diHOME: An exercise-induced lipokine that increases skeletal muscle fatty acid uptake. Cell. Metab. 27 (5), 1111–1120. doi:10.1016/j.cmet.2018.03.020
Takeda, K., Sawazaki, H., Takahashi, H., Yeh, Y. S., Jheng, H. F., Nomura, W., et al. (2018). The dipeptidyl peptidase-4 (DPP-4) inhibitor teneligliptin enhances Brown adipose tissue function, thereby preventing obesity in mice. FEBS Open Bio 8 (11), 1782–1793. doi:10.1002/2211-5463.12498
Tang, H., Inoki, K., Brooks, S. V., Okazawa, H., Lee, M., Wang, J., et al. (2019). mTORC1 underlies age-related muscle fiber damage and loss by inducing oxidative stress and catabolism. Aging Cell. 18 (3), e12943. doi:10.1111/acel.12943
Tournissac, M., Leclerc, M., Valentin-Escalera, J., Vandal, M., Bosoi, C. R., Planel, E., et al. (2021). Metabolic determinants of alzheimer's disease: A focus on thermoregulation. Ageing Res. Rev. 72, 101462. doi:10.1016/j.arr.2021.101462
van den Berg, S. A., van Marken Lichtenbelt, W., Willems van Dijk, K., and Schrauwen, P. (2011). Skeletal muscle mitochondrial uncoupling, adaptive thermogenesis and energy expenditure. Curr. Opin. Clin. Nutr. Metab. Care 14 (3), 243–249. doi:10.1097/MCO.0b013e3283455d7a
Villarroya, J., Cereijo, R., and Villarroya, F. (2013). An endocrine role for Brown adipose tissue? Am. J. Physiol. Endocrinol. Metab. 305 (5), E567–E572. doi:10.1152/ajpendo.00250.2013
Villarroya, F., Cereijo, R., Villarroya, J., and Giralt, M. (2017). Brown adipose tissue as a secretory organ. Nat. Rev. Endocrinol. 13 (1), 26–35. doi:10.1038/nrendo.2016.136
Villarroya, J., Cereijo, R., Gavaldà-Navarro, A., Peyrou, M., Giralt, M., and Villarroya, F. (2019). New insights into the secretory functions of Brown adipose tissue. J. Endocrinol. 243 (2), R19–r27. doi:10.1530/JOE-19-0295
Virtanen, K. A., Lidell, M. E., Orava, J., Heglind, M., Westergren, R., Niemi, T., et al. (2009). Functional Brown adipose tissue in healthy adults. N. Engl. J. Med. 360 (15), 1518–1525. doi:10.1056/NEJMoa0808949
Wang, C., Bomberg, E., Billington, C., Levine, A., and Kotz, C. M. (2007). Brain-derived neurotrophic factor in the hypothalamic paraventricular nucleus increases energy expenditure by elevating metabolic rate. Am. J. Physiol. Regul. Integr. Comp. Physiol. 293 (3), R992–R1002. doi:10.1152/ajpregu.00516.2006
Wang, Z., Liu, X., Jiang, K., Kim, H., Kajimura, S., and Feeley, B. T. (2020). Intramuscular Brown fat activation decreases muscle atrophy and fatty infiltration and improves gait after delayed rotator cuff repair in mice. Am. J. Sports Med. 48 (7), 1590–1600. doi:10.1177/0363546520910421
Wang, Z., Liu, X., Liu, M., Jiang, K., Kajimura, S., Kim, H., et al. (2021). β(3)-Adrenergic receptor agonist treats rotator cuff fatty infiltration by activating beige fat in mice. J. Shoulder Elb. Surg. 30 (2), 373–386. doi:10.1016/j.jse.2020.06.006
Wibmer, A. G., Becher, T., Eljalby, M., Crane, A., Andrieu, P. C., Jiang, C. S., et al. (2021). Brown adipose tissue is associated with healthier body fat distribution and metabolic benefits independent of regional adiposity. Cell. Rep. Med. 2 (7), 100332. doi:10.1016/j.xcrm.2021.100332
Xiao, Y., Xu, A., Law, L. S. C., Chen, C., Li, H., Li, X., et al. (2012). Distinct changes in serum fibroblast growth factor 21 levels in different subtypes of diabetes. J. Clin. Endocrinol. Metab. 97 (1), E54–E58. doi:10.1210/jc.2011-1930
Yoon, M. J., Lee, G. Y., Chung, J. J., Ahn, Y. H., Hong, S. H., and Kim, J. B. (2006). Adiponectin increases fatty acid oxidation in skeletal muscle cells by sequential activation of AMP-activated protein kinase, p38 mitogen-activated protein kinase, and peroxisome proliferator-activated receptor alpha. Diabetes 55 (9), 2562–2570. doi:10.2337/db05-1322
Yuan, X., Hu, T., Zhao, H., Huang, Y., Ye, R., Lin, J., et al. (2016). Brown adipose tissue transplantation ameliorates polycystic ovary syndrome. Proc. Natl. Acad. Sci. U. S. A. 113 (10), 2708–2713. doi:10.1073/pnas.1523236113
Zhang, X., Yeung, D. C. Y., Karpisek, M., Stejskal, D., Zhou, Z. G., Liu, F., et al. (2008). Serum FGF21 levels are increased in obesity and are independently associated with the metabolic syndrome in humans. Diabetes 57 (5), 1246–1253. doi:10.2337/db07-1476
Keywords: metabolic defects, thermogenesis, brown adipose tissue, insulin resistance, skeletal muscle
Citation: Chen Y, Hu Q, Wang C and Wang T (2023) The crosstalk between BAT thermogenesis and skeletal muscle dysfunction. Front. Physiol. 14:1132830. doi: 10.3389/fphys.2023.1132830
Received: 14 January 2023; Accepted: 11 April 2023;
Published: 21 April 2023.
Edited by:
Valentina Di Felice, University of Palermo, ItalyReviewed by:
Naresh Chandra Bal, KIIT University, IndiaP. Trayhurn, University of Liverpool, United Kingdom
Copyright © 2023 Chen, Hu, Wang and Wang. This is an open-access article distributed under the terms of the Creative Commons Attribution License (CC BY). The use, distribution or reproduction in other forums is permitted, provided the original author(s) and the copyright owner(s) are credited and that the original publication in this journal is cited, in accordance with accepted academic practice. No use, distribution or reproduction is permitted which does not comply with these terms.
*Correspondence: Changyi Wang, Y2hhbmd5aTExQHNjdS5lZHUuY24=; Tiantian Wang, dGlhbnRpYW53YW5nQHNjdS5lZHUuY24=