- 1Maternal and Children’s Health Research Institute, Shunde Women and Children’s Hospital, Guangdong Medical University, Foshan, China
- 2Institute of Respiratory, Shunde Women and Children’s Hospital, Guangdong Medical University, Foshan, China
Ferroptosis represents a novel non-apoptotic form of regulated cell death that is driven by iron-dependent lipid peroxidation and plays vital roles in various diseases including cardiovascular diseases, neurodegenerative disorders and cancers. Plenty of iron metabolism-related proteins, regulators of lipid peroxidation, and oxidative stress-related molecules are engaged in ferroptosis and can regulate this complex biological process. Sirtuins have broad functional significance and are targets of many drugs in the clinic. Recently, a growing number of studies have revealed that sirtuins can participate in the occurrence of ferroptosis by affecting many aspects such as redox balance, iron metabolism, and lipid metabolism. This article reviewed the studies on the roles of sirtuins in ferroptosis and the related molecular mechanisms, highlighting valuable targets for the prevention and treatment of ferroptosis-associated diseases.
1 Introduction
1.1 Ferroptosis
Ferroptosis is characterized by fatal lipid reactive oxygen species (ROS) and massive iron-dependent cell death. Before ferroptosis was defined, cell death is classified into apoptosis, autophagy-related cell death, and necrosis according to distinct morphological features (Kroemer et al., 2005). However, Dolma and Yagodac et al. found that cell death induced by RAS-selective lethal (RSL) compounds could not be classified as the traditional form of cell death (Dolma et al., 2003; Yagoda et al., 2007). In 2012, Dixon proposed the concept of ferroptosis based on distinct morphological, biochemical, and genetic features of RSL-induced cell death (Dixon et al., 2012). After that, studies related to ferroptosis is growing exponentially.
Ferroptosis mainly involves three mechanisms. The first one is an increased intracellular free iron content: extracellular Fe3+ is transported into intracellular endosomes through transferrin and reduced to Fe2+, which is released into the cytoplasmic iron pool via the divalent metal-ion transporter 1 (DMT1), while the excess iron is stored in ferritin (Frazer and Anderson, 2014; Xie et al., 2016). Under some circumstances, ferritin releases Fe2+ via “ferritinophagy” which initiates a Fenton reaction with the NADPH oxidase NOX, or with H2O2 produced by the mitochondrial electron transport chain, generating excessive ROS and free radicals (Jiang et al., 2021). The second one is the impaired glutathione peroxidase 4 (GPX4) activity: phospholipid hydroperoxides (PLOOHs) are executioners of ferroptosis. GPX4 is the major enzyme that catalyzes the reduction of PLOOHs in mammals (Yang et al., 2014). Generally, GPX4 requires two electrons from GSH for the reduction of phospholipid and cholesteryl hydroperoxides to their corresponding alcohols, thereby reducing PLOOHs; the blockade of the antioxidant system, cystine-glutamate antiporter (system Xc−), can lead to insufficient glutathione (GSH) synthesis. The system Xc− consists of two subunits (SLC7A11 and SLC3A2) that transport cystines into cells and glutamate out of the cells simultaneously. Cystine is a dimer of cysteine (Murphy et al., 1989). Cysteine is an important component in the synthesis of GSH and its deficiency will cause insufficient cellular GSH synthesis, which will affect the function of GPX4, ultimately leading to ferroptosis (Stockwell et al., 2017; Hassannia et al., 2019). The third important one is lipid peroxidation: polyunsaturated fatty acids (PUFA) are important initiators of lipid peroxidation (Jiang et al., 2021). Phospholipids containing polyunsaturated acyl tails (PL-PUFAs), activated by enzymes such as long-chain fatty acyl-CoA synthetase 4 (ACSL4) and lysolecithin acyltransferase, mainly promote lipid peroxidation (Gill and Valivety, 1997). In the final stage of ferroptosis, lipid peroxidation directly or indirectly induces the formation of pores in the cell membrane, which triggers cell death (Kagan et al., 2017). Ferroptosis has its unique characteristics. Morphologically, ferroptosis is characterized mainly by dysfunction of mitochondria as excess iron drives the peroxidation of plasma-membrane lipids and affects the fluidity and integrity of the plasma membrane, which results in the rupture of the outer mitochondrial membrane, shrinkage, cristae reduction, and even disappearance, and ultimately disrupts mitochondrial function (Jiang et al., 2021). Metabolically, in addition to increased intracellular iron, ferrous ions, and ROS, ferroptosis is often accompanied by reduced GSH metabolism and other changes (Zhang et al., 2022a). More specific mechanisms of ferroptosis have been reported in several studies (Dixon et al., 2012; Hadian and Stockwell, 2020; Jiang et al., 2021; Qi et al., 2022; Stockwell, 2022). Figure 1 shows the main mechanism of ferroptosis.
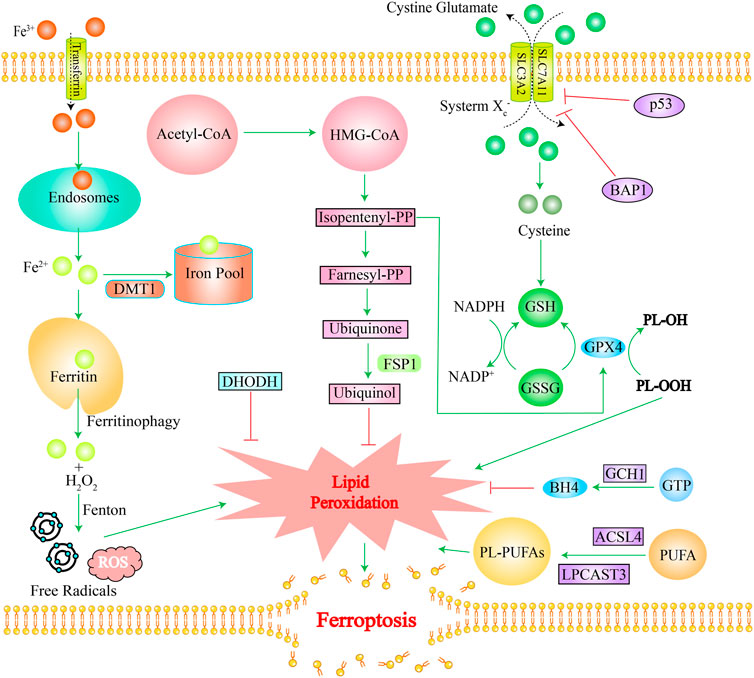
FIGURE 1. The main mechanism of ferroptosis. Green lines stand for promotion effects and red lines stand for inhibition effects. Iron overload, ROS accumulation, and lipid peroxidation induce ferroptosis. The GPX4 pathway, FS1-Ubiquinol pathway, GCH1-BH4 pathway, and DHODH pathway inhibit ferroptosis. Ferritin releases Fe2+ via “ferritinophagy” which initiates a Fenton reaction with H2O2 produced, generating excessive ROS and free radicals. DHODH detoxifies lipid peroxides and inhibits ferroptosis. FSP1 converts Ubiquinone into Ubiquinol, thereby inhibiting lipid peroxidation. GPX4 requires GSH as a cofactor to promote inhibition of lipid peroxidation. The system Xc—exchanges cystine and glutamic acid, which are converted to cysteine for glutathione synthesis. BAPI and p53 genes can inhibit cystine uptake by inhibiting the expression of SLC7A11. GCH1 catalyzes GTP to produce BH4, reshaping lipids to inhibit ferroptosis. ACSL4 and LPCAT3 are necessary for production of PL-PUFAs, which promote ferroptosis. Abbreviation: DMT1, divalent metal transporter 1; Acetyl-CoA, Acetyl-coenzyme A; FSP1, ferroptosis suppressor protein 1; BAP1, BRCA1 associated protein 1; GSH, glutathione; GSSG, Oxidized glutathione; BH4, Tetrahydrobiopterin; GCH1, GTP cyclohydrolase-1; DHODH, dihydrolactate dehydrogenase; GTP, guanosine triphosphate cyclohydrolase-1; ACSL4, long-chain fatty acyl-CoA; LPCAST3, lysolecithin acyltransferase 3; HMG-CoA, 3-Hydroxy-3-Methyl-Glutaryl-CoA; PP, diphosphate.
Ferroptosis plays a significant role in tumors, neurodegenerative diseases, cardiovascular and cerebrovascular diseases, metabolic diseases, and respiratory diseases (Galaris et al., 2006; Dixon et al., 2012; Heemels, 2016; El Hout et al., 2018; Ma et al., 2019; Yoshida et al., 2019; Wang et al., 2021a; Cheng et al., 2021; Chen et al., 2022a). For instance, in neoplastic diseases, high ROS levels and high iron contents in cancer cells render them more susceptible to ferroptosis than normal cells due to their high metabolism (Dixon et al., 2012; Sosa et al., 2013; Spangler et al., 2016). In neurodegenerative diseases, regional redistribution of iron in brain tissue leads to ferroptosis in portions of the brain tissue (Yan and Zhang, 2019). Ferroptosis induced by intracellular lipid peroxidation and iron accumulation in mitochondria may be an important cause of cardiovascular and cerebrovascular disease since blood circulation is essential for iron transportation (Wortmann et al., 2013; Zhang et al., 2022b). In metabolic diseases such as diabetes, cells in a hyperglycemic environment will produce excess ROS, which can easily cause lipid metabolism disorders and further lead to ferroptosis (Yung et al., 2016; Han et al., 2020). In COVID-19, the SARS-CoV-2 virus can cause cellular iron uptake, depletion of the GSH-GPX4 axis, and ROS overproduction, which all contribute to the Fenton reaction to generate excess lipid hydroperoxides and hydroxyl radicals, ultimately accelerating ferroptosis (Fratta Pasini et al., 2021). Currently, studies have indicated that iron chelators as well as anti-lipid peroxidation drugs may suppress or ameliorate these diseases (Hanson et al., 2009; Dare et al., 2015). It's worth noting that multiple endogenous antioxidant defense systems in the cells can regulate ferroptosis. Notably, the sirtuins family can regulate ferroptosis via mediating multiple target genes and is a potential target for the treatment of ferroptosis-related diseases.
1.2 Sirtuins family
In 1979, Amar Klar identified a protein (mating-type regulator, MAR1) that could silence a gene locus in yeast (Klar et al., 1979). Then, three proteins with similar functions were found by other investigators (Tissenbaum and Guarente, 2001; Rogina and Helfand, 2004; Viswanathan and Guarente, 2011), all of which were uniformly named sirtuins (Frye, 2000). Among them, MAR1 was designated as Sir2 (Grunstein, 1997). In 1999, Kaeberlein et al. unveiled that sirtuins could significantly extend yeast lifespan by 30% (Kaeberlein et al., 1999), which attracted much attention. Mammal sirtuins comprise seven isozymes (SIRT1-SIRT7). Based on molecular genetic analysis of different biological types and domain sequences, the seven sirtuins can be classified into four distinct classes (Frye, 2000; Greiss and Gartner, 2009; Donmez and Guarente, 2010; Cen et al., 2011; Teixeira et al., 2020): class I (SIRT1, 2, and 3), class II (SIRT4), class III (SIRT5), and class IV (SIRT6 and SIRT7) (Roth et al., 2013). Sirtuins are all composed of a small domain (a Zn2+ domain consisting of about 40 amino acids) and a large domain (a Rossman fold consisting of about 200 amino acids) (Chang and Guarente, 2014). Only when NAD+ and acetyl-lysine can enter the active site from either end of the cleft between these two domains, the molecular conformation of the sirtuins can be stretched and extended to activate the function of deacetylases and ADP ribosyltransferases (Bordo, 2013).
Sirtuins are differentially expressed in tissues and organs, and the tissues and organs with high expression of sirtuins members are summarized in Table 1. Collectively, sirtuins are mainly highly expressed in tissues, organs, and embryos with high metabolic rates. Additionally, sirtuins also have different cellular localizations and functions in cells. SIRT1, SIRT6, and SIRT7 are mainly located in the nucleus, SIRT3, SIRT4, and SIRT5 are mainly situated in the mitochondria, whereas, only SIRT2 is mainly presented in the cytoplasm (Vaquero, 2009; Houtkooper et al., 2012; Wang and Lin, 2021). Notably, the subcellular localization of these sirtuins also depends on the state of the cell as well as molecular interactions; for example, SIRT1 and SIRT2 can translocate between the nucleus and the cytoplasm, and SIRT3 can shuttle between mitochondria and the nucleus, where they can interact with proteins (Houtkooper et al., 2012; Bonkowski and Sinclair, 2016). The sirtuins family acts as an NAD+-dependent histone deacetylase and the function is linked to deacetylation and ADP-ribosyltransferase activity. SIRT1, SIRT2, and SIRT3 have strong histone deacetylase activity, whereas, SIRT4-SIRT7 have weak deacetylase activity (Vaquero, 2009). In addition, SIRT4 and SIRT6 possess ADP-ribosyltransferase activity (Chen et al., 2015). They can modulate multiple pathways such as glucose and fatty acid metabolism, anti-aging, apoptosis, DNA repair, neuronal production, inflammatory responses, and even the regulation of the circadian clock by regulating post-translational modifications of histones and transcription factors (Haigis and Sinclair, 2010; Chang and Guarente, 2014).
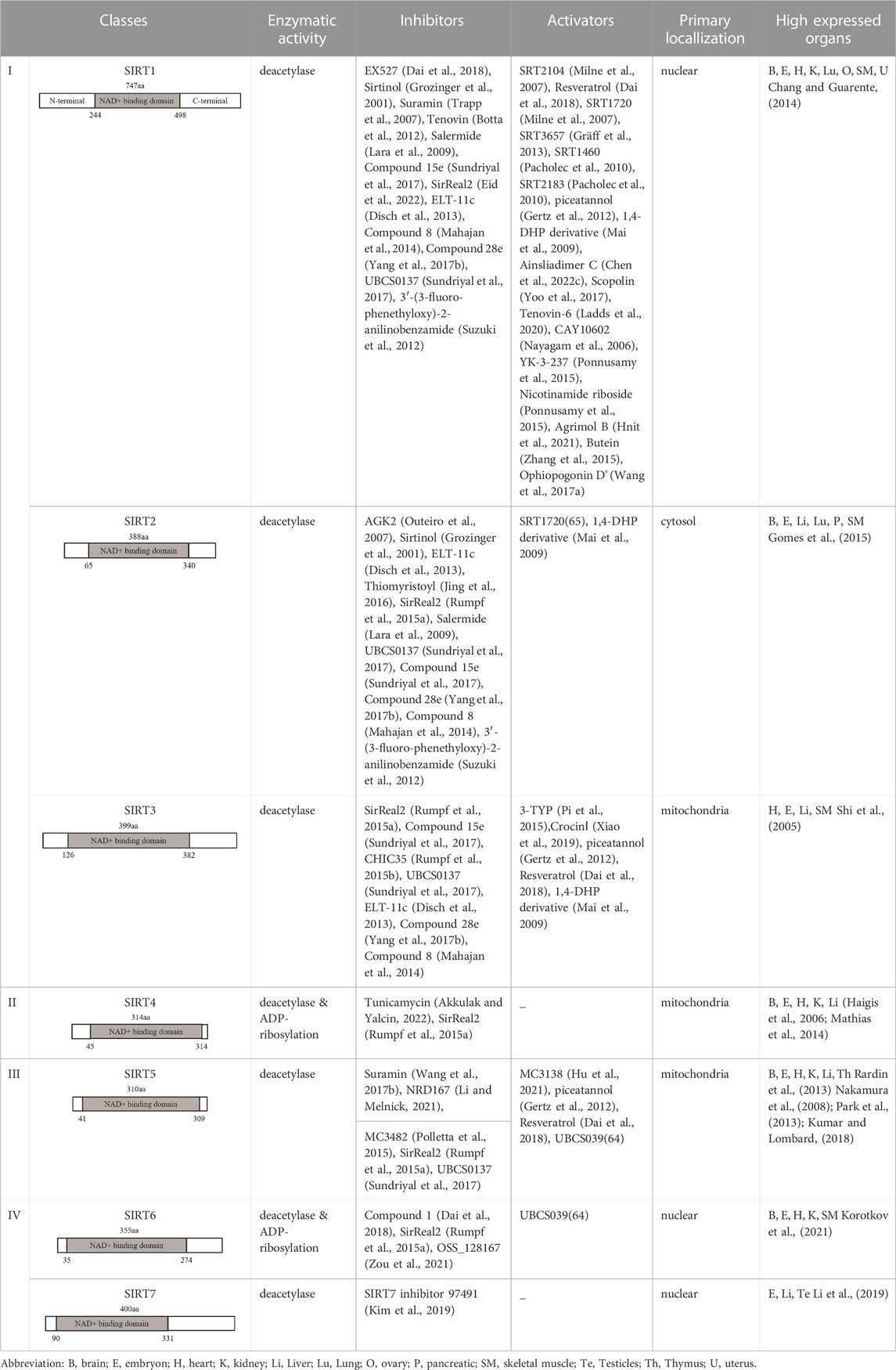
TABLE 1. Molecular structure, enzymatic activity, regulators, localization, and tissue expression patterns of sirtuins.
Sirtuins have been identified as therapeutic targets for many diseases (such as tumors, cardiovascular diseases, and nervous system diseases) (Ford et al., 2005; Ajami et al., 2017; Kane and Sinclair, 2018; Manjula et al., 2020), so the regulators of sirtuins are research hotspots in recent years. For example, Selisistat (EX 527, SEN0014196) is currently the only SIRT1-targeted drug in the stages of clinical trials for the treatment of Huntington’s disease (Rollnik, 2015). Selisistat can occupy the nicotinamide binding site and the c-pocket adjacent to nicotinamide and bind to NAD+ ribose or the coproduct 2′-O-acetyl-ADP-ribose, showing a strong inhibitory effect on SIRT1 and weak inhibitory effects on SIRT2, SIRT3, and SRT6 but no effect on SIRT5 (Napper et al., 2005; Gertz et al., 2013). Inhibitors of sirtuins effectively induce cellular and physiological effects through diverse substrate sites (Rajabi et al., 2018), while activators of sirtuins only require an allosteric binding site, and, therefore activators have stronger targeting specificity and fewer side effects (Dai et al., 2018). A synthetic sirtuin-activating compound with imidazothiazole as its core can potently activate SIRT1 (Milne et al., 2007). For example, there are currently 14 clinical trials (www.clinicaltrials.gov) for SRT2104 showing beneficial effects (Baksi et al., 2014; van der Meer et al., 2015; Sands et al., 2016). The classification, molecular structure, function, inhibitors, activators, and subcellular localization of mammalian sirtuins are listed in Table 1.
The sirtuins family not only can effectively reduce ROS, suppress inflammation, and decrease lipid peroxide and iron levels but also can protect against or alleviate ferroptosis. At present, the development and gradual maturing of activators and inhibitors of sirtuins provide a powerful tool for investigating the roles of sirtuins in ferroptosis.
2 Roles of sirtuins in ferroptosis
2.1 SIRT1
SIRT1, the earliest and most intensively studied sirtuin family member of class III deacetylases, possesses potent deacetylase activity and is widely involved in multiple biological processes including aging, apoptosis, autophagy, stress, inflammation, and energy metabolism (Guo et al., 2015; Mu et al., 2015; Li et al., 2016). SIRT1 has recently been demonstrated to regulate ferroptosis via mediating multiple target genes.
P53: SIRT1 can deacetylate p53 to suppress ferroptosis. P53 can regulate ferroptosis through pathways dependent or independent of GPX4 (Espinosa-Diez et al., 2015; Chen et al., 2021). On the one hand, p53 can induce ferroptosis by directly inhibiting the GPX4 pathway (Liu et al., 2021a). On the other hand, under ROS stress, p53 can either inhibit cystine uptake by repressing SLC7A11 expression or enhance cellular ferroptosis via indirectly strengthening the function of the lipoxygenase (ALOX) family (Jiang et al., 2015; Chu et al., 2019). Doxorubicin (Dox) has been proven to induce ferroptosis in cardiac cells by initiating the Fenton reaction, producing large amounts of ROS as well as reducing the levels of antioxidant substances (GPX4, SOD, GSH, etc.) (Zhang et al., 2022b). De et al. found that overexpression of SIRT1 repressed the acetylation of p53 and decreased the ROS level in DOX-induced cardiac cells (De Angelis et al., 2015). In light of this finding, we speculated that SIRT1 could suppress DOX-elicited ferroptosis in cardiac cells by deacetylating p53, which was demonstrated by the following evidence. Ma et al. revealed that SIRT1 rescued the suppressive effect of p53 on SLC7A11 by reducing the acetylation level of p53, thereby inhibiting ferroptosis evoked by myocardial ischemia/reperfusion (Ma et al., 2020). Furthermore, SIRT1 has been demonstrated to repress p53 acetylation at the K382 site and the expression level of ACSL4 as well as inhibit lipid peroxidation and the level of malondialdehyde (MDA), a product of lipid peroxidation, while increasing the expression of GPX4 and SLC7A11, thereby alleviating acute lung injury caused by heat stress by inhibiting ferroptosis (Chen et al., 2022b).
Nuclear factor erythroid2-related factor 2 (NRF2): SIRT1 can also exert anti-ferroptosis effects by activating NRF2, a central regulator of the antioxidant stress response (Ma, 2013; Bellezza et al., 2018). NRF2 also acts as a master regulator of iron metabolism and ferroptosis, and most of the genes related to ferroptosis identified to date are target genes of NRF2 (Dodson et al., 2019; Anandhan et al., 2020). Firstly, NRF2 can’t only upregulate ferritin heavy chain 1 (FTH1) and ferritin light chain (FTL) expression to increase the free iron storage in ferritin but also reduce the intracellular iron content and inhibit cellular free iron accumulation via inducing ferroportin (FPN), the only membrane iron exporter (Pietsch et al., 2003; Harada et al., 2011; Kerins and Ooi, 2018). Secondly, NRF2 prevents lipid peroxidation by activating the transcription of the GPX4 gene and activating the expression of the genes involved in the GSH biosynthetic pathway (catalytic and regulatory subunit of glutamate-cysteine ligase, glutathione reductase, SLC7A11), as well as the genes that can promote the reduction of oxidized glutathione (GSSG) to GSH (glucose 6-phosphate dehydrogenase, 6-phosphogluconate dehydrogenase, malic enzyme) (Lu, 2009; Shin et al., 2018; Dodson et al., 2019). These findings collectively illustrate that NRF2 can effectively inhibit ferroptosis. The suppressive effect of SIRT1-NRF2 on ferroptosis has been validated in a variety of diseases. Fisetin exerts its therapeutic effects on DOX-induced cardiomyopathy by inhibiting ferroptosis via SIRT1/NRF2 pathway activation in the rat model of DOX-induced cardiomyopathy and H9c2 cells (Li et al., 2021). Tang et al. also demonstrated that miR-138-5p could exert anti-ferroptosis effects by activating the SIRT1/NRF2 pathway, thereby alleviating diabetic retinopathy (Tang et al., 2022). Zeng et al. demonstrated that resveratrol can alleviate sepsis-induced cardiomyopathy by activating SIRT1 and upregulating the expression of NRF2 to against ferroptosis (Zeng et al., 2023). Wang et al. elucidated that Ulinastatin blocks ferroptosis in liver injury triggered by acetaminophen overdose via the SIRT1-NRF2-HO-1 pathway (Wang et al., 2021b). Also, the latest study has illustrated that mesenchymal stem cell (MSC)-derived exosomes can inhibit hippocampal ferroptosis to alleviate delayed neurocognitive recovery in aged mice via activating the SIRT1/NRF2/HO-1 pathway, while inhibition of SIRT1 activity can abolish the protective effect exerted by MSC-derived exosomes (Liu et al., 2022a). Furthermore, Dang et al. unraveled that Edaravone alleviated ferroptosis in depression and anxiety-like behaviors through the SIRT1/NRF2/HO-1/GPX-4 pathway (Dang et al., 2022). Peroxisome proliferator-activated receptor gamma coactivator 1α (PGC-1α) can regulate mitochondrial biogenesis, upregulate the expression of antioxidant enzymes, and decrease the levels of ROS (Krishnan et al., 2012). NRF2 can regulate PGC-1α to modulate ferroptosis (Miao et al., 2022). Additionally, SIRT1 can also directly activate PGC-1α. SIRT1 can interact with PGC-1α at specific lysine residues and deacetylate it in a NAD (+)-dependent manner, whereby activating the transcriptional activity of PGC-1α, promoting mitochondriogenesis, and suppressing oxidative stress (Rodgers et al., 2005; Baur et al., 2006). The anti-diabetic drug canagliflozin (Cana) potentiates the activity of SIRT1 and AMPK and their downstream target PGC-1α and promotes oxidative phosphorylation in adipocytes, thereby reducing insulin resistance (Yang et al., 2020).
Liang et al. (2023) showed that mitochondrial damage resulting from SIRT1 inactivation played an important role in ferroptosis caused by Epothilone B in schwann cells. Liu et al. found identified that pumilio 2 (PUM2) promoted ferroptosis by inhibiting SIRT1/SLC7A11, aggravated neuroinflammation and brain damage induced by ischemia-reperfusion injury (Liu et al., 2023). In addition, activation of SIRT1 and GPX4 by calorie restriction protects against the ferroptosis-caused kidney injury in the Sprague Dawley rat model of contrast-induced nephropathy (Fang et al., 2021). Ferritinophagy is a form of cell-selective autophagy mediated by nuclear receptor coactivator 4 (NCOA4), which degrades ferritin (mainly FTH1) in autophagosomes, leading to the release of free iron from ferritin-bound iron (Santana-Codina et al., 2021). Ferritinophagy has been linked to ferroptosis (Zhou et al., 2020a). Autophagy has also been proven to promote ferroptosis through the removal of ferritin (Hou et al., 2016). The SIRT1-autophagy axis can protect foam cells from ferroptosis caused by inflammatory cytokines (Su et al., 2021). Recently, some scholars also inferred that the SIRT1-autophagy axis could inhibit oxidative stress-induced ferroptosis in nucleus pulposus cells (Zhou and Ruan, 2022). Silencing miR-34a, a target repressor of SIRT1, can increase SIRT1 expression and decrease hepatic triglyceride accumulation and lipid ROS production in the presence of iron (Wang et al., 2020). Hao et al. reported that treatment of rat pheochromocytoma cells (PC12 cells) with metal cadmium led to ferroptosis by up-regulating miR-34a-5p expression and inhibiting SIRT1 expression (Hao et al., 2022).
In addition to the evidence of direct involvement in ferroptosis, the role of SIRT1 in iron overload and resistance to oxidative stress also suggests its potential mechanisms affecting ferroptosis. Transcription factors of the forkhead box class O (FOXO) family can regulate a variety of genes involved in antioxidant defense (Klotz et al., 2015). FOXO1 has been demonstrated to inhibit lipid accumulation (Kitakata et al., 2021). Iron overload can result in a reduction in the expression of SIRT1 and an increase in acetylated FOXO1 in the nucleus as well as an increased level of oxidative stress. However, FOXO1 acetylation can be reversed when SIRT1 is overexpressed, initiating antioxidant functions and protecting against liver damage from ferroptosis (Das et al., 2016). In addition, studies showed that, by the inhibition of the activity of FOXO1, insulin suppresses gluconeogenesis in the liver and decreases blood glucose, thereby alleviating diabetes (Puigserver et al., 2003; Semova et al., 2022). In diabetes, pancreatic β-cell cells undergo ferroptosis (Bao et al., 2023). We therefore speculate that insulin may regulate FOXO1 by activating SIRT1 to alleviate ferroptosis. FOXO3a can induce antioxidant responses by regulating manganese superoxide dismutase (MnSOD, SOD2) and catalase (CAT) and also regulate mitochondrial activity by inhibiting the c-myc function, thereby blocking the hypoxia-dependent increase in the ROS level (Ferber et al., 2012). SIRT1 exerts its anti-oxidative function in 293T cells under conditions of oxidative stress by interacting with FOXO3a (Brunet et al., 2004). Moreover, SRT2104, as an activator of SIRT1, decreases the synthesis of 4-hydroxynonenal (4-HNE), a marker of lipid peroxides, in the liver and muscle and decreases MnSOD levels in muscle (Mercken et al., 2014).
SIRT1 can repress ferroptosis in cells in the vast majority of cases, but several pieces of negative evidence have also been reported. For example, Zhou et al. (2020b) found that intestine-specific SIRT1 knockout reduces the iron accumulation level in ethanol-induced liver injury to protect against ethanol-induced ferroptosis in hepatocytes. Based on this study, we speculated that SIRT1 knockout could lead to a decrease in iron uptake by intestinal epithelial cells, thereby reducing iron content in the liver and ultimately alleviating ferroptosis in hepatocytes. Moreover, Lee and Sui et al. also revealed that inhibition or silencing of SIRT1 antagonized ferroptosis in head and neck cancer and human papillary carcinoma cells. In other words, SIRT1 overexpression could result in ferroptosis in head and neck cancer and human papillary carcinoma cells, thus preventing tumor progression (Sui et al., 2019; Lee et al., 2020), which might be attributed to the mechanism that SIRT1 overexpression affected cellular homeostasis. When Wang et al. (2023) studied the damage of fluorine to the liver in chicken, they found that sodium fluoride upregulated SIRT1 and triggered the production of ferroptosis. Taken together, SIRT1 can regulate the progression of ferroptosis through multiple pathways and is a valuable potential target for the treatment of ferroptosis-related diseases.
2.2 SIRT2
SIRT2 can deacetylate more than 30 substrate proteins and exert regulatory functions in metabolism, lipogenesis, cell cycle progression, oxidative stress, inflammation, etc (Lee et al., 2019). Additionally, SIRT2 can regulate ferroptosis. In a rat model of neuropathic damage, Zhang et al. found that SIRT2 upregulated the expression of FPN1 and GPX4 and downregulated that of ACSL4, leading to reduced iron contents and lipid peroxidation levels, thus alleviating ferroptosis (Zhang et al., 2022c). Gao et al. (2021) demonstrated that in a mouse model of traumatic brain injury, SIRT2 suppressed ferroptosis following traumatic brain injury by decreasing the acetylation level of p53.
Oxidative stress induces massive generation of ROS that damage proteins, lipids as well as nucleic acids, which is an important process in ferroptosis. Studies have shown that the deacetylation function of SIRT2 plays a vital role in redox balance, suggesting that SIRT2 may modulate ferroptosis. Similar to SIRT1, SIRT2 can deacetylate PGC1-α (Krishnan et al., 2012) and FOXO3a (Chen et al., 2013) to upregulate the expression of antioxidant enzymes and downregulate the levels of ROS, thereby alleviating cell injury. NADPH is an important molecule that counteracts oxidative damage by maintaining the reduced-form glutathione. SIRT2 can also deacetylate and activate 6-phosphogluconate dehydrogenase, a key enzyme of the pentose-phosphate pathway, thereby increasing cytosolic NADPH levels and reducing cellular oxidative damage (Wang et al., 2014). Cao et al. (2016) unveiled that SIRT2 inhibitors inhibited Akt phosphorylation and decreased nuclear NRF2 levels, which in turn decreased cellular glutamate cysteine ligase (GCL) and GSH levels, attenuating cellular antioxidant capacity. Yang et al. found that SIRT2 decreased the total and nuclear NRF2 activity and expression via binding to and deacetylating NRF2 on lysines 506 and 508 sites, while a decrease in nuclear NRF2 levels further decreased FPN1 expression and finally reduced the cellular iron export (Yang et al., 2017a). In iron-deficient hepatocytes, this improving effect of SIRT2 on cellular iron level contributes to cell survival. However, whether SIRT2 can elevate cellular iron levels in the presence of high iron levels requires further investigation.
2.3 SIRT3
Although all sirtuins can extend the lifespan of yeast, a linkage disequilibrium analysis in 710 subjects has suggested that only SIRT3 correlates with the human lifespan (Bellizzi et al., 2007). SIRT3 is localized in mitochondria, but under cellular stress conditions, such as various stimuli (ultraviolet irradiation and chemotherapy), SIRT3 can transfer from mitochondria into the nucleus to exert NAD⁺-dependent deacetylation function (Alqarni et al., 2021). SIRT3 also exerts crucial roles in the electron transport chain, fatty acid oxidation, amino acid metabolism, iron metabolism, redox balance, and the tricarboxylic acid (TCA) cycle (Finley and Haigis, 2012), by which it regulates the activities of specific metabolic enzymes as well as ATP synthesis, metabolism, and intracellular signaling to relieve oxidative stress (Michishita et al., 2005). Additionally, SIRT3 can regulate mitochondrial disorders, such as cancer, sleep disorders, and Alzheimer’s disease (Lee et al., 2018; Lin et al., 2020; Ouyang et al., 2022). Studies have shown that SIRT3 can regulate diseases by inhibiting ferroptosis.
In the nervous system, ferroptosis is a major contributor to oligodendrocyte (OL) death triggered by glutamate, while inhibition of SIRT3 promotes glutamate-induced ferroptosis in OLs and consequently decreases cell survival, which is critical to the pathobiology of stroke and traumatic brain injury (Novgorodov et al., 2018). The ketogenic diet (KD) is famous for its neuroprotective effects. Compared with the long-chain triglyceride-enriched KD (LKD), the medium-chain triglyceride-enriched KD (MKD) has a stronger preventive effect against the cognitive deficits elicited by sleep deprivation, which may be associated with MKD-caused higher SIRT3 protein levels and inhibition of ferroptosis (Wang et al., 2022).
The role of SIRT3 in ferroptosis seems paradoxical in tumors. Cancer cells have vigorous metabolism, and mitochondria are the cellular power plant. It has been illustrated that SIRT3 colocalizes with p53 in mitochondria and deacetylates p53 (Novgorodov et al., 2018). Hence, the anti-ferroptosis effect of SIRT3 in tumor cells potentially functions in the mitochondria. SIRT3 has been also substantiated to suppress p53-mediated ferroptosis under ROS stress stimulation in human osteosarcoma and melanoma cells (Jin et al., 2021). Conversely, in the presence of high glucose concentrations and ferroptosis-inducing compounds, SIRT3 deficiency can disrupt the AMPK-mTOR pathway and increase GPX4 expression to suppress ferroptosis in trophoblasts (Han et al., 2020). Intriguingly, SIRT3 also plays an opposing role in gallbladder cancer. Liu et al. unraveled that SIRT3 silencing could inhibit Akt-dependent death in gallbladder cancer cell lines; vice versa, overexpression of SIRT3 boosted ferroptosis in gallbladder cancer cells and repressed tumor initiation and progression (Liu et al., 2021b). P53 is well-known as a very important target of SIRT3, and p53 mutation or loss-of-function frequently occurs in tumor cells, but neither of the latter two studies assessed the function of p53, which may be one of the reasons for the aforementioned contradictory effects of SIRT3 on ferroptosis.
In the heart, the angiotensin receptor enkephalin inhibitor LCZ696 increased the expression of SIRT3 and deacetylated its target gene SOD2 by activating AKT, thereby inhibiting ferroptosis, and ultimately preventing cardiac toxicity caused by DOX (Liu et al., 2022b). In the respiratory system, cigarette smoke extract inactivated the NRF2/SIRT3 signaling pathway through ROS, thereby promoting the expression of iNOS, ultimately leading to ferroptosis in bronchial epithelial cells (Zi et al., 2023). In the reproductive system, metformin can improve polycystic ovary syndrome by upregulating the expression level of SIRT3 and activating the SIRT3/AMPK/mTOR pathway to inhibit ferroptosis (Peng et al., 2023).
Iron overload, ROS production, and lipid peroxidation are important factors in the development of ferroptosis. Iron regulatory proteins (IRPs) are implicated in the maintenance of cellular iron homeostasis and can regulate the expression of iron metabolism-associated genes by binding to the iron-responsive element (IRE) of target mRNAs (Pantopoulos, 2004). Mitochondrial SIRT3 regulates cellular iron metabolism via controlling the IRP1 activity, while SIRT3 deficiency leads to an imbalance of cellular iron homeostasis. SIRT3 overexpression decreases the expression of TfR1 (a membrane-associated glycoprotein critical for iron uptake and cell proliferation) and suppresses pancreatic cancer cell proliferation by repressing IRP1 (Jeong et al., 2015). In addition, the knockdown of SIRT3 can exacerbate iron overload and NADPH oxidase-derived ROS production while increasing the levels of acetylated p53, HO-1, and FPN (Feng et al., 2020). It has been evidenced that SIRT3 regulates iron overload-induced hepatocyte death through the Wnt/β-catenin pathway (Mandala et al., 2021). SIRT3 contributes to the maintenance of GSH in the reduced and activated state. SIRT3 converts O2− to H2O2 as well as NADP+ to the TCA cycle of NADPH and also deacetylates isocitrate dehydrogenase 2 (IDH2) and MnSOD, thereby maintaining the oxidative balance (Qiu et al., 2010; Tao et al., 2010; Shi et al., 2017; Sidorova-Darmos et al., 2018). Angiotensin-receptor blocker LCZ696 can suppress DOX-caused cardiotoxicity by activating the AKT/SIRT3/SOD2 pathway and protecting against ferroptosis (Liu et al., 2022b). Besides, SIRT3 can activate NRF2, a key regulator of ferroptosis, stimulating the transcription of downstream antioxidant genes (Gao et al., 2018). Reversely, NRF2 also regulates SIRT3 expression and reduces ROS levels in neuronal cells (Gao et al., 2018).
Altogether, SIRT3 can mediate ferroptosis through multiple pathways in either the mitochondria or the nucleus. However, SIRT3 shows different regulatory roles in ferroptosis in different cells and under different stress conditions, which requires additional in-depth investigation.
2.4 Other sirtuins family members
There is currently no definite evidence that SIRT4 can affect ferroptosis, but SIRT4 can compensate for each other with SIRT1 and SIRT3. SIRT4 knockdown in primary hepatocytes increases SIRT1 expression (Nasrin et al., 2010), whereas, SIRT4 overexpression in mice with Angiotensin II-induced cardiac hypertrophy inhibits the binding of MnSOD to mitochondrial SIRT3 and increases MnSOD acetylation levels to reduce its activity, resulting in increased ROS accumulation upon Ang II stimulation (Luo et al., 2017). There is also no evidence for a direct effect of SIRT5 on ferroptosis, but SIRT5 can also suppress the progression of oxidative stress (Liang et al., 2017). Studies have shown that SIRT5 overexpression in neuroblastoma (Liang et al., 2017) and cardiomyocytes (Liu et al., 2013) can reduce the level of oxidative stress caused by H2O2. Furthermore, SIRT5 can deacetylate SOD1, thus strengthening the antioxidant effect of SOD1 against ROS (Lin et al., 2013). SIRT5 may inhibit ferroptosis by reducing oxidative stress, which remains to be investigated yet. Undoubtedly, more studies are needed to demonstrate the correlations of SIRT4 and SIRT5 with ferroptosis.
Studies have reported that SIRT6 can also inhibit ferroptosis. Cai et al. found that SIRT6 silencing led to the inactivation of the Keap1/NRF2 pathway and downregulation of GPX4 expression, promoting ferroptosis in gastric cancer cells (Cai et al., 2021). In addition, SIRT6 can protect human mesenchymal stem cells against oxidative stress-associated damage by activating NRF2 (Pan et al., 2016). Wang’s team demonstrated that sodium hydrosulfide (NaHS) had anti-inflammatory effects and anti-ferroptosis effects by up-regulating SIRT6 in type 1 diabetic mouse model (Wang et al., 2021c). Recently, SIRT6 has been demonstrated to inhibit nuclear transcription of NF-κB and inactivate NF-κB to facilitate ferroptosis in pancreatic cancer, thereby exerting anti-tumor effects (Gong et al., 2022). Fang et al. (2022) demonstrated that SIRT6 overexpression could reverse the decreased levels of GPX4, SLC7A11, and GSH and reduce the cellular accumulation of MDA and ROS to inhibit ferroptosis, ultimately restoring bone formation and angiogenesis and relieving femoral head necrosis. Mi et al. demonstrated that melatonin inhibited ferroptosis in rat lens epithelial cells through SIRT6/p-NRF2/GPX4 and SIRT6/NCOA4/FTH1 pathways, neutralized lipid peroxidation toxicity, and delayed the formation of cataract caused by ultraviolet rays exposure (Mi et al., 2023). In addition to direct evidence, SIRT6 can also modulate ferroptosis-associated genes, which indirectly demonstrates that SIRT6 can regulate ferroptosis. SIRT6 deacetylates and destabilizes p53 (Ghosh et al., 2018), and, in turn, the transcription factor p53 increases the expression of miR-34a that represses SIRT6 (Lefort et al., 2013). The regulation of SIRT6 by miR-34a may form a positive feedback loop for the p53 function. Moreover, it has been elucidated that SIRT6 can reduce the level of oxidative stress by activating the AMPK-FOXO3a axis, thereby protecting cardiomyocytes from ischemia-reperfusion injury (Wang et al., 2016). Pan et al. (2016) found that SIRT6 also co-activated NRF2 and its downstream antioxidant factors to protect mesenchymal stem cells from oxidative stress.
In chronic renal diseases caused by hypertension, SIRT7 can alleviate renal ferroptosis and epithelial-mesenchymal transition in hypertensive states by promoting the KLF15/NRF2 signaling pathway, thereby reducing renal fibrosis, injury, and dysfunction (Li et al., 2022). SIRT7 can regulate ferroptosis-associated mitochondrial ferritin expression and p53 activity. Carles Díez-López conducted genome-wide and TaqMan® low-density array analyses and determined low SIRT7 and mitochondrial ferritin levels in patients with chronic heart failure and systemic iron deficiency; they also demonstrated that low-to-moderate levels of SIRT7 and mitochondrial ferritin were associated with an increased risk of all-cause mortality and admission to hospital with heart failure (Diez-Lopez et al., 2021). The function of SIRT7 to deacetylate p53 remains controversial. Vakhrusheva et al. found increased levels of acetylated K382 on p53 in SIRT7-knockout mice, and in vitro experiments also proved that the deacetylase activity of SIRT7 was comparable to that of SIRT1 with the p53 peptide acetylated at K382 as a substrate (Vakhrusheva et al., 2008). However, Barber et al. revealed that SIRT7 could not deacetylate p53-K382 either in vitro or in vivo (Barber et al., 2012). Likewise, Michishita et al. demonstrated that SIRT7 purified from Hi5 insect cells also failed to deacetylate p53-K382 (Michishita et al., 2005).
Collectively, the family members of sirtuins (deacetylases) are all implicated in varying degrees in the regulation of the ferroptosis process, and the regulatory roles of the sirtuins family in ferroptosis, the molecular mechanisms, and the diseases involved are summarized in Table 2 and Figure 2.
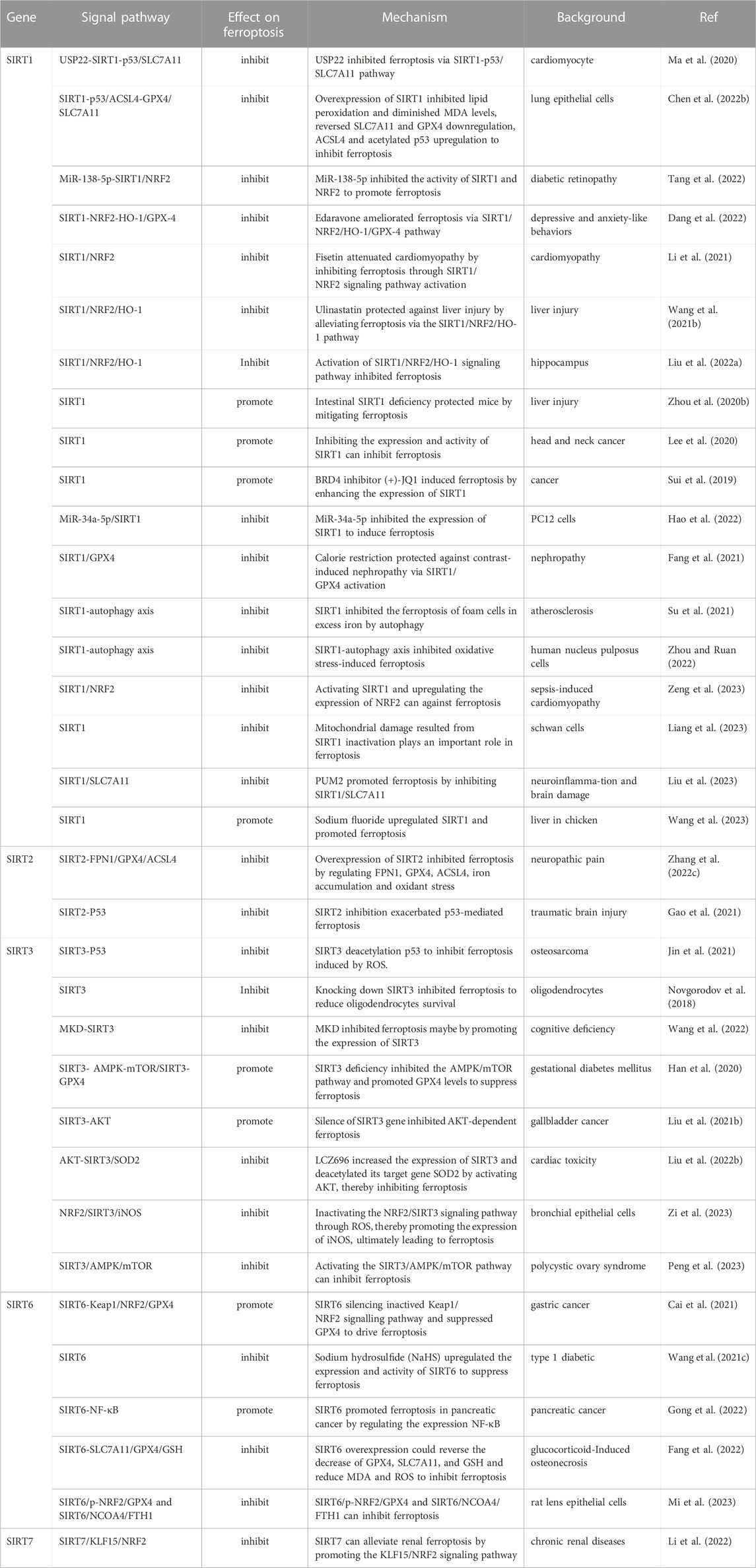
TABLE 2. The regulatory roles, molecular mechanisms, and research background of sirtuins in ferroptosis.
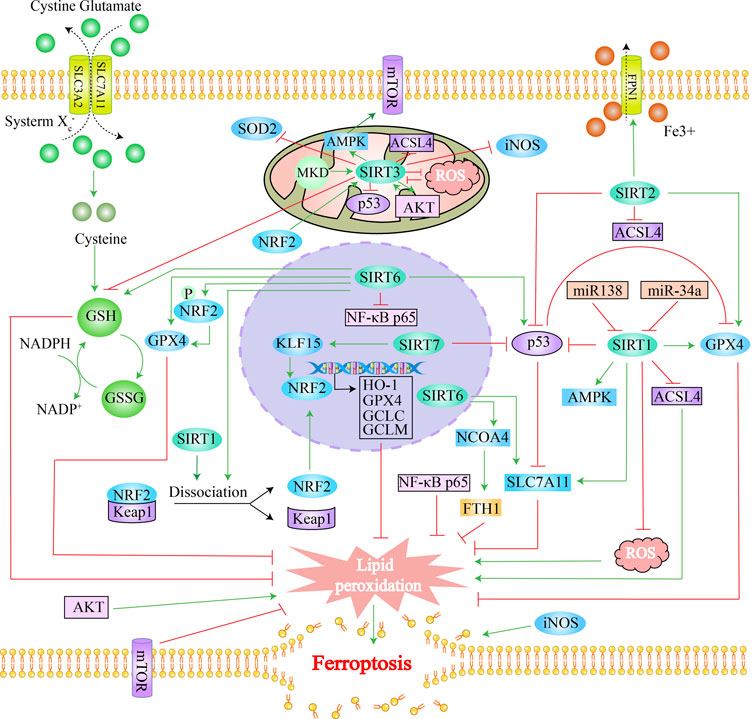
FIGURE 2. Overview of sirtuins and ferroptosis: Green lines represent promotion effects and red lines represent inhibition effects in the figure. We constructed sirtuins family pathways related to ferroptosis using Adobe illustrator software according to the gene pathways described in the text. Schematic diagram illustrating the distribution of sirtuins family members in the cell, their regulatory mechanisms, as well as functions. Sirtuins regulate ferroptosis by mediating lipid peroxides, ROS levels as well as iron content through different molecular pathways.
3 Implications of bioinformatic analysis
To more clearly and intuitively view the pathways involved in ferroptosis mediated by sirtuins, the genes interacting with 7 sirtuins family members and ferroptosis-associated genes were screened from Genecards and Ferrdb (http://www.zhounan.org/ferrdb/) databases, respectively; then a Venn diagram of sirtuins-related genes and ferroptosis-related genes was plotted in the Evenn website (http://www.ehbio.com/test/venn/#/) as depicted in Figure 3 and Supplementary Table S1. From this Venn diagram, 188 potential interaction targets were found in the intersection. Among these potential targets, MTOR, TP53, NFE2L2, HIF1A, HMOX1, G6PD and KEAP1 were mentioned in the text, while the remaining genes are to be investigated. To gain more insight into the relationship between these 188 genes and sirtuins, the overlapping genes in the above Venn diagram were imported into the STRING database and a protein-protein interaction (PPI) network was constructed. The potential key direct action targets were screened using the Cytoscape tool. A total of 115 nodes and 324 lines are shown in Figure 4. The results showed a degree 30 for all sirtuins, a degree of 104 for SIRT1, a degree of 37 for SIRT2, a degree of 44 for SIRT3, a degree of 32 for SIRT4, a degree of 35 for SIRT5, a degree of 38 for SIRT6, and a degree of 34 for SIRT7. The aforesaid findings illustrated that sirtuins were strongly linked to multiple ferroptosis-associated genes. We listed multiple ferroptosis-associated genes interacting with sirtuins in the Supplementary Table S1, and more mechanisms of sirtuins regulating ferroptosis-related genes have not been identified, which is our future research direction.
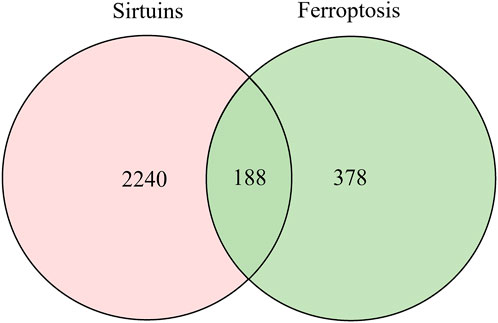
FIGURE 3. Venn diagram of downstream regulatory genes in sirtuins and ferroptosis: After screening the sirtuins family-related genes and ferroptosis-related genes from the Genecards and Ferrdb databases, respectively, a Venn diagram of the genes linked to the seven members of the sirtuins family and the genes related to ferroptosis was constructed in the Evenn webpage.
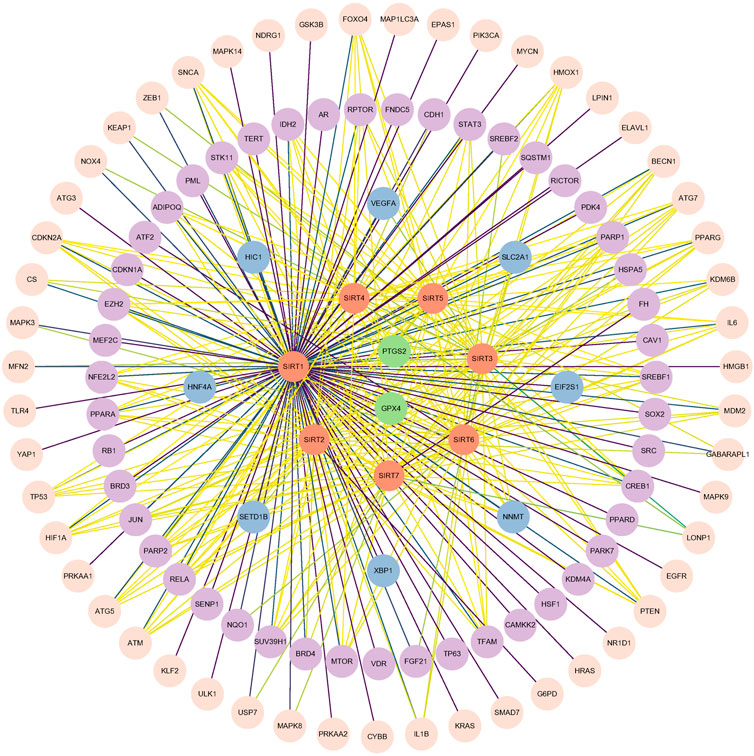
FIGURE 4. The interaction data with humans as species were derived from STRING (http://string-db.org, accessed 17 March 2023) based on the intersection in the Venn diagram (Figure 3), and this map shows the direct interaction between seven members in the sirtuins family and proteins involved in ferroptosis after data processing with Cytoscape tool. Orange circles denote sirtuins members, and other circles denote ferroptosis-associated genes. The lines (yellow-green-purple) indicate the gradual enhancement of protein-protein interaction.
4 Perspectives
Ferroptosis is a lipid peroxidative damage caused by overload free iron. Ferroptosis has been found to occur in a variety of diseases, including cardiovascular diseases, tumors, liver diseases, kidney diseases, neurological diseases, metabolic diseases, respiratory diseases (such as the global pandemic COVID-19) and immune system diseases. With the in-depth study of the mechanism of ferroptosis, targeted drugs have been developed rapidly. These drugs can mainly be divided into several categories, including targeting the Xc system (such as piperazine erastin), GPX4 (such as altretamine), iron metabolism pathway (such as dihydroartemisinin), lipid metabolism pathway (such as Zileuton) and other metabolic pathways (such as NADH, NADPH). Although there are a variety of drugs targeting ferroptosis, the treatment might be limited by potential side effects. Sirtuins-specific drugs have been developed, some of which have already been applied in clinical trials. In addition, we have predicted 188 target genes of sirtuins associated with ferroptosis through bioinformatics analysis, most of which have not been reported yet. It is worth mentioning that the in-depth study of these pathways may provide targets and a theoretical basis for the development of ferroptosis-targeted drugs.
Current researches related to ferroptosis mechanisms mainly focus on lipid metabolism, ROS, and iron metabolism. With a deeper understanding of ferroptosis and the sirtuins family, researchers are increasingly aware of the link between sirtuins and ferroptosis. Of particular importance, the homeostasis of both lipid ROS and iron metabolism levels can be regulated by sirtuins target genes. For instance, p53 is an important ferroptosis trigger, and NRF2 is a key antioxidant gene, both of which can be regulated by SIRT1 (Ma, 2013; De Angelis et al., 2015; Bellezza et al., 2018). Mitochondria, the main organelle for producing ROS, are multifaceted regulators of ferroptosis. How important the role of SIRT3, SIRT6 and SIRT7, which are located in mitochondria, plays in scavenging ROS and the related ferroptosis remains to be elucidated. In addition, current studies on the role of sirtuins in ferroptosis are limited in the context of diseases, while their functions in ontogenesis have not been reported. The exploration of these fields may help researchers better understand the mechanism of sirtuins in ferroptosis.
Author contributions
JZ wrote the manuscript. GM and YW conducted this study and critically revised the manuscript. JG and SH processed the data and pictures. YC, FL, XX, and RC corrected the article. All authors contributed to the article and approved the submitted version.
Funding
Support for this work includes funding from the National Natural Science Foundation of China (81770034 and 81670252), Guangdong Basic and Applied Basic Foundation (2022A1515140167, 2022A1515110234, 2019A1515011306 and 2020A1515010240), Doctor Startup Fund of Shunde Women and Children’s Hospital of Guangdong Medical University (2020BSQD003 and 2021BSQD001), Discipline construction project of Guangdong Medical University (2021XKJSPT001).
Conflict of interest
The authors declare that the research was conducted in the absence of any commercial or financial relationships that could be construed as a potential conflict of interest.
Publisher’s note
All claims expressed in this article are solely those of the authors and do not necessarily represent those of their affiliated organizations, or those of the publisher, the editors and the reviewers. Any product that may be evaluated in this article, or claim that may be made by its manufacturer, is not guaranteed or endorsed by the publisher.
Supplementary material
The Supplementary Material for this article can be found online at: https://www.frontiersin.org/articles/10.3389/fphys.2023.1131201/full#supplementary-material
References
Ajami, M., Pazoki-Toroudi, H., Amani, H., Nabavi, S. F., Braidy, N., Vacca, R. A., et al. (2017). Therapeutic role of sirtuins in neurodegenerative disease and their modulation by polyphenols. Neurosci. Biobehav Rev. 73, 39–47. doi:10.1016/j.neubiorev.2016.11.022
Akkulak, A., and Yalcin, G. D. (2022). The interaction of SIRT4 and Calreticulin during ER stress in glia cells. Gene 814, 146135. doi:10.1016/j.gene.2021.146135
Alqarni, M. H., Foudah, A. I., Muharram, M. M., and Labrou, N. E. (2021). The pleiotropic function of human sirtuins as modulators of metabolic pathways and viral infections. Cells 10 (2), 460. doi:10.3390/cells10020460
Anandhan, A., Dodson, M., Schmidlin, C. J., Liu, P., and Zhang, D. D. (2020). Breakdown of an ironclad defense system: The critical role of NRF2 in mediating ferroptosis. Cell Chem. Biol. 27 (4), 436–447. doi:10.1016/j.chembiol.2020.03.011
Baksi, A., Kraydashenko, O., Zalevkaya, A., Stets, R., Elliott, P., Haddad, J., et al. (2014). A phase II, randomized, placebo-controlled, double-blind, multi-dose study of SRT2104, a SIRT1 activator, in subjects with type 2 diabetes. Br. J. Clin. Pharmacol. 78 (1), 69–77. doi:10.1111/bcp.12327
Bao, L., Jin, Y., Han, J., Wang, W., Qian, L., and Wu, W. (2023). Berberine regulates GPX4 to inhibit ferroptosis of islet β cells. Planta Med. 89 (3), 254–261. doi:10.1055/a-1939-7417
Barber, M. F., Michishita-Kioi, E., Xi, Y., Tasselli, L., Kioi, M., Moqtaderi, Z., et al. (2012). SIRT7 links H3K18 deacetylation to maintenance of oncogenic transformation. Nature 487 (7405), 114–118. doi:10.1038/nature11043
Baur, J. A., Pearson, K. J., Price, N. L., Jamieson, H. A., Lerin, C., Kalra, A., et al. (2006). Resveratrol improves health and survival of mice on a high-calorie diet. Nature 444 (7117), 337–342. doi:10.1038/nature05354
Bellezza, I., Giambanco, I., Minelli, A., and Donato, R. (2018). Nrf2-Keap1 signaling in oxidative and reductive stress. Biochim. Biophys. Acta Mol. Cell Res. 1865 (5), 721–733. doi:10.1016/j.bbamcr.2018.02.010
Bellizzi, D., Dato, S., Cavalcante, P., Covello, G., Di Cianni, F., Passarino, G., et al. (2007). Characterization of a bidirectional promoter shared between two human genes related to aging: SIRT3 and PSMD13. Genomics 89 (1), 143–150. doi:10.1016/j.ygeno.2006.09.004
Bonkowski, M. S., and Sinclair, D. A. (2016). Slowing ageing by design: The rise of NAD(+) and sirtuin-activating compounds. Nat. Rev. Mol. Cell Biol. 17 (11), 679–690. doi:10.1038/nrm.2016.93
Bordo, D. (2013). Structure and evolution of human sirtuins. Curr. Drug Targets 14 (6), 662–665. doi:10.2174/1389450111314060007
Botta, G., De Santis, L. P., and Saladino, R. (2012). Current advances in the synthesis and antitumoral activity of SIRT1-2 inhibitors by modulation of p53 and pro-apoptotic proteins. Curr. Med. Chem. 19 (34), 5871–5884. doi:10.2174/092986712804143303
Brunet, A., Sweeney, L. B., Sturgill, J. F., Chua, K. F., Greer, P. L., Lin, Y., et al. (2004). Stress-dependent regulation of FOXO transcription factors by the SIRT1 deacetylase. Science 303 (5666), 2011–2015. doi:10.1126/science.1094637
Cai, S., Fu, S., Zhang, W., Yuan, X., Cheng, Y., and Fang, J. (2021). SIRT6 silencing overcomes resistance to sorafenib by promoting ferroptosis in gastric cancer. Biochem. Biophys. Res. Commun. 577, 158–164. doi:10.1016/j.bbrc.2021.08.080
Cao, W., Hong, Y., Chen, H., Wu, F., Wei, X., and Ying, W. (2016). SIRT2 mediates NADH-induced increases in Nrf2, GCL, and glutathione by modulating Akt phosphorylation in PC12 cells. FEBS Lett. 590 (14), 2241–2255. doi:10.1002/1873-3468.12236
Cen, Y., Youn, D. Y., and Sauve, A. A. (2011). Advances in characterization of human sirtuin isoforms: Chemistries, targets and therapeutic applications. Curr. Med. Chem. 18 (13), 1919–1935. doi:10.2174/092986711795590084
Chang, H. C., and Guarente, L. (2014). SIRT1 and other sirtuins in metabolism. Trends Endocrinol. Metab. 25 (3), 138–145. doi:10.1016/j.tem.2013.12.001
Chen, B., Zang, W., Wang, J., Huang, Y., He, Y., Yan, L., et al. (2015). The chemical biology of sirtuins. Chem. Soc. Rev. 44 (15), 5246–5264. doi:10.1039/c4cs00373j
Chen, C., Ren, Y. M., Zhu, J. Z., Chen, J. L., Feng, Z. L., Zhang, T., et al. (2022). Ainsliadimer C, a disesquiterpenoid isolated from Ainsliaea macrocephala, ameliorates inflammatory responses in adipose tissue via Sirtuin 1-NLRP3 inflammasome axis. Acta Pharmacol. Sin. 43 (7), 1780–1792. doi:10.1038/s41401-021-00797-z
Chen, D., Chu, B., Yang, X., Liu, Z., Jin, Y., Kon, N., et al. (2021). iPLA2β-mediated lipid detoxification controls p53-driven ferroptosis independent of GPX4. Nat. Commun. 12 (1), 3644. doi:10.1038/s41467-021-23902-6
Chen, H., Lin, X., Yi, X., Liu, X., Yu, R., Fan, W., et al. (2022). SIRT1-mediated p53 deacetylation inhibits ferroptosis and alleviates heat stress-induced lung epithelial cells injury. Int. J. Hyperth. 39 (1), 977–986. doi:10.1080/02656736.2022.2094476
Chen, J., Chan, A. W., To, K. F., Chen, W., Zhang, Z., Ren, J., et al. (2013). SIRT2 overexpression in hepatocellular carcinoma mediates epithelial to mesenchymal transition by protein kinase B/glycogen synthase kinase-3β/β-catenin signaling. Hepatology 57 (6), 2287–2298. doi:10.1002/hep.26278
Chen, J., Li, X., Ge, C., Min, J., and Wang, F. (2022). The multifaceted role of ferroptosis in liver disease. Cell Death Differ. 29 (3), 467–480. doi:10.1038/s41418-022-00941-0
Cheng, H., Feng, D., Li, X., Gao, L., Tang, S., Liu, W., et al. (2021). Iron deposition-induced ferroptosis in alveolar type II cells promotes the development of pulmonary fibrosis. Biochim. Biophys. Acta Mol. Basis Dis. 1867 (12), 166204. doi:10.1016/j.bbadis.2021.166204
Chu, B., Kon, N., Chen, D., Li, T., Liu, T., Jiang, L., et al. (2019). ALOX12 is required for p53-mediated tumour suppression through a distinct ferroptosis pathway. Nat. Cell Biol. 21 (5), 579–591. doi:10.1038/s41556-019-0305-6
Dai, H., Sinclair, D. A., Ellis, J. L., and Steegborn, C. (2018). Sirtuin activators and inhibitors: Promises, achievements, and challenges. Pharmacol. Ther. 188, 140–154. doi:10.1016/j.pharmthera.2018.03.004
Dang, R., Wang, M., Li, X., Wang, H., Liu, L., Wu, Q., et al. (2022). Edaravone ameliorates depressive and anxiety-like behaviors via Sirt1/Nrf2/HO-1/Gpx4 pathway. J. Neuroinflammation 19 (1), 41. doi:10.1186/s12974-022-02400-6
Dare, A. J., Bolton, E. A., Pettigrew, G. J., Bradley, J. A., Saeb-Parsy, K., and Murphy, M. P. (2015). Protection against renal ischemia-reperfusion injury in vivo by the mitochondria targeted antioxidant MitoQ. Redox Biol. 5, 163–168. doi:10.1016/j.redox.2015.04.008
Das, S. K., DesAulniers, J., Dyck, J. R., Kassiri, Z., and Oudit, G. Y. (2016). Resveratrol mediates therapeutic hepatic effects in acquired and genetic murine models of iron-overload. Liver Int. 36 (2), 246–257. doi:10.1111/liv.12893
De Angelis, A., Piegari, E., Cappetta, D., Russo, R., Esposito, G., Ciuffreda, L. P., et al. (2015). SIRT1 activation rescues doxorubicin-induced loss of functional competence of human cardiac progenitor cells. Int. J. Cardiol. 189, 30–44. doi:10.1016/j.ijcard.2015.03.438
Diez-Lopez, C., Tajes Orduna, M., Enjuanes Grau, C., Moliner Borja, P., Gonzalez-Costello, J., Garcia-Romero, E., et al. (2021). Blood differential gene expression in patients with chronic heart failure and systemic iron deficiency: Pathways involved in pathophysiology and impact on clinical outcomes. J. Clin. Med. 10 (21), 4937. doi:10.3390/jcm10214937
Disch, J., Evindar, G., Chiu, C., Blum, C., Dai, H., Jin, L., et al. (2013). Discovery of thieno[3,2-d]pyrimidine-6-carboxamides as potent inhibitors of SIRT1, SIRT2, and SIRT3. J. Med. Chem. 56 (9), 3666–3679. doi:10.1021/jm400204k
Dixon, S. J., Lemberg, K. M., Lamprecht, M. R., Skouta, R., Zaitsev, E. M., Gleason, C. E., et al. (2012). Ferroptosis: An iron-dependent form of nonapoptotic cell death. Cell 149 (5), 1060–1072. doi:10.1016/j.cell.2012.03.042
Dodson, M., Castro-Portuguez, R., and Zhang, D. D. (2019). NRF2 plays a critical role in mitigating lipid peroxidation and ferroptosis. Redox Biol. 23, 101107. doi:10.1016/j.redox.2019.101107
Dolma, S., Lessnick, S. L., Hahn, W. C., and Stockwell, B. R. (2003). Identification of genotype-selective antitumor agents using synthetic lethal chemical screening in engineered human tumor cells. Cancer Cell 3, 285–296. doi:10.1016/s1535-6108(03)00050-3
Donmez, G., and Guarente, L. (2010). Aging and disease: Connections to sirtuins. Aging Cell 9 (2), 285–290. doi:10.1111/j.1474-9726.2010.00548.x
Eid, M., Dzreyan, V., and Demyanenko, S. (2022). Sirtuins 1 and 2 in the acute period after photothrombotic stroke: Expression, localization and involvement in apoptosis. Front. Physiol. 13, 782684. doi:10.3389/fphys.2022.782684
El Hout, M., Dos Santos, L., Hamaï, A., and Mehrpour, M. (2018). A promising new approach to cancer therapy: Targeting iron metabolism in cancer stem cells. Semin. Cancer Biol. 53, 125–138. doi:10.1016/j.semcancer.2018.07.009
Espinosa-Diez, C., Miguel, V., Mennerich, D., Kietzmann, T., Sánchez-Pérez, P., Cadenas, S., et al. (2015). Antioxidant responses and cellular adjustments to oxidative stress. Redox Biol. 6, 183–197. doi:10.1016/j.redox.2015.07.008
Fang, D., Wang, Y., Zhang, Z., Yang, D., Gu, D., He, B., et al. (2021). Calorie restriction protects against contrast-induced nephropathy via SIRT1/GPX4 activation. Oxid. Med. Cell Longev. 2021, 2999296. doi:10.1155/2021/2999296
Fang, L., Zhang, G., Wu, Y., Li, Z., Gao, S., and Zhou, L. (2022). SIRT6 prevents glucocorticoid-induced osteonecrosis of the femoral head in rats. Oxid. Med. Cell Longev. 2022, 6360133. doi:10.1155/2022/6360133
Feng, X., Su, H., He, X., Chen, J. X., and Zeng, H. (2020). SIRT3 deficiency sensitizes angiotensin-II-induced renal fibrosis. Cells 9 (11), 2510. doi:10.3390/cells9112510
Ferber, E. C., Peck, B., Delpuech, O., Bell, G. P., East, P., and Schulze, A. (2012). FOXO3a regulates reactive oxygen metabolism by inhibiting mitochondrial gene expression. Cell Death Differ. 19 (6), 968–979. doi:10.1038/cdd.2011.179
Finley, L. W., and Haigis, M. C. (2012). Metabolic regulation by SIRT3: Implications for tumorigenesis. Trends Mol. Med. 18 (9), 516–523. doi:10.1016/j.molmed.2012.05.004
Ford, J., Jiang, M., and Milner, J. (2005). Cancer-specific functions of SIRT1 enable human epithelial cancer cell growth and survival. Cancer Res. 65 (22), 10457–10463. doi:10.1158/0008-5472.CAN-05-1923
Fratta Pasini, A. M., Stranieri, C., Girelli, D., Busti, F., and Cominacini, L. (2021). Is ferroptosis a key component of the process leading to multiorgan damage in COVID-19? Antioxidants (Basel) 10 (11), 1677. doi:10.3390/antiox10111677
Frazer, D. M., and Anderson, G. J. (2014). The regulation of iron transport. Biofactors 40 (2), 206–214. doi:10.1002/biof.1148
Frye, R. A. (2000). Phylogenetic classification of prokaryotic and eukaryotic Sir2-like proteins. Biochem. Biophys. Res. Commun. 273 (2), 793–798. doi:10.1006/bbrc.2000.3000
Galaris, D., Barbouti, A., and Korantzopoulos, P. (2006). Oxidative stress in hepatic ischemia-reperfusion injury: The role of antioxidants and iron chelating compounds. Curr. Pharm. Des. 12 (23), 2875–2890. doi:10.2174/138161206777947614
Gao, J., Li, Y., and Song, R. (2021). SIRT2 inhibition exacerbates p53-mediated ferroptosis in mice following experimental traumatic brain injury. Neuroreport 32 (12), 1001–1008. doi:10.1097/WNR.0000000000001679
Gao, J., Liu, S., Xu, F., Liu, Y., Lv, C., Deng, Y., et al. (2018). Trilobatin protects against oxidative injury in neuronal PC12 cells through regulating mitochondrial ROS homeostasis mediated by AMPK/Nrf2/Sirt3 signaling pathway. Front. Mol. Neurosci. 11, 267. doi:10.3389/fnmol.2018.00267
Gertz, M., Fischer, F., Nguyen, G. T., Lakshminarasimhan, M., Schutkowski, M., Weyand, M., et al. (2013). Ex-527 inhibits Sirtuins by exploiting their unique NAD+-dependent deacetylation mechanism. Proc. Natl. Acad. Sci. U. S. A. 110 (30), E2772–E2781. doi:10.1073/pnas.1303628110
Gertz, M., Nguyen, G. T., Fischer, F., Suenkel, B., Schlicker, C., Fränzel, B., et al. (2012). A molecular mechanism for direct sirtuin activation by resveratrol. PLoS One 7 (11), e49761. doi:10.1371/journal.pone.0049761
Ghosh, S., Wong, S. K., Jiang, Z., Liu, B., Wang, Y., Hao, Q., et al. (2018). Haploinsufficiency of Trp53 dramatically extends the lifespan of Sirt6-deficient mice. Elife 7, e32127. doi:10.7554/eLife.32127
Gill, I., and Valivety, R. (1997). Polyunsaturated fatty acids, Part 1: Occurrence, biological activities and applications. Trends Biotechnol. 15 (10), 401–409. doi:10.1016/S0167-7799(97)01076-7
Gomes, P., Fleming Outeiro, T., and Cavadas, C. (2015). Emerging role of sirtuin 2 in the regulation of mammalian metabolism. Trends Pharmacol. Sci. 36 (11), 756–768. doi:10.1016/j.tips.2015.08.001
Gong, S., Xiong, L., Luo, Z., Yin, Q., Huang, M., Zhou, Y., et al. (2022). SIRT6 promotes ferroptosis and attenuates glycolysis in pancreatic cancer through regulation of the NF-κB pathway. Exp. Ther. Med. 24 (2), 502. doi:10.3892/etm.2022.11430
Gräff, J., Kahn, M., Samiei, A., Gao, J., Ota, K. T., Rei, D., et al. (2013). A dietary regimen of caloric restriction or pharmacological activation of SIRT1 to delay the onset of neurodegeneration. J. Neurosci. 33 (21), 8951–8960. doi:10.1523/JNEUROSCI.5657-12.2013
Greiss, S., and Gartner, A. (2009). Sirtuin/Sir2 phylogeny, evolutionary considerations and structural conservation. Mol. Cells 28 (5), 407–415. doi:10.1007/s10059-009-0169-x
Grozinger, C. M., Chao, E. D., Blackwell, H. E., Moazed, D., and Schreiber, S. L. (2001). Identification of a class of small molecule inhibitors of the sirtuin family of NAD-dependent deacetylases by phenotypic screening. J. Biol. Chem. 276 (42), 38837–38843. doi:10.1074/jbc.M106779200
Grunstein, M. (1997). Molecular model for telomeric heterochromatin in yeast. Curr. Opin. Cell Biol. 9 (3), 383–387. doi:10.1016/s0955-0674(97)80011-7
Guo, Z., Liao, Z., Huang, L., Liu, D., Yin, D., and He, M. (2015). Kaempferol protects cardiomyocytes against anoxia/reoxygenation injury via mitochondrial pathway mediated by SIRT1. Eur. J. Pharmacol. 761, 245–253. doi:10.1016/j.ejphar.2015.05.056
Hadian, K., and Stockwell, B. R. (2020). SnapShot: Ferroptosis. Cell 181 (5), 1188. doi:10.1016/j.cell.2020.04.039
Haigis, M. C., Mostoslavsky, R., Haigis, K. M., Fahie, K., Christodoulou, D. C., Murphy, A. J., et al. (2006). SIRT4 inhibits glutamate dehydrogenase and opposes the effects of calorie restriction in pancreatic beta cells. Cell 126 (5), 941–954. doi:10.1016/j.cell.2006.06.057
Haigis, M. C., and Sinclair, D. A. (2010). Mammalian sirtuins: Biological insights and disease relevance. Annu. Rev. Pathol. 5, 253–295. doi:10.1146/annurev.pathol.4.110807.092250
Han, D., Jiang, L., Gu, X., Huang, S., Pang, J., Wu, Y., et al. (2020). SIRT3 deficiency is resistant to autophagy-dependent ferroptosis by inhibiting the AMPK/mTOR pathway and promoting GPX4 levels. J. Cell Physiol. 235 (11), 8839–8851. doi:10.1002/jcp.29727
Hanson, L. R., Roeytenberg, A., Martinez, P. M., Coppes, V. G., Sweet, D. C., Rao, R. J., et al. (2009). Intranasal deferoxamine provides increased brain exposure and significant protection in rat ischemic stroke. J. Pharmacol. Exp. Ther. 330 (3), 679–686. doi:10.1124/jpet.108.149807
Hao, R., Ge, J., Song, X., Li, F., Sun-Waterhouse, D., and Li, D. (2022). Cadmium induces ferroptosis and apoptosis by modulating miR-34a-5p/Sirt1axis in PC12 cells. Environ. Toxicol. 37 (1), 41–51. doi:10.1002/tox.23376
Harada, N., Kanayama, M., Maruyama, A., Yoshida, A., Tazumi, K., Hosoya, T., et al. (2011). Nrf2 regulates ferroportin 1-mediated iron efflux and counteracts lipopolysaccharide-induced ferroportin 1 mRNA suppression in macrophages. Arch. Biochem. Biophys. 508 (1), 101–109. doi:10.1016/j.abb.2011.02.001
Hassannia, B., Vandenabeele, P., and Vanden Berghe, T. (2019). Targeting ferroptosis to iron out cancer. Cancer Cell 35 (6), 830–849. doi:10.1016/j.ccell.2019.04.002
Hnit, S. S. T., Ding, R., Bi, L., Xie, C., Yao, M., De Souza, P., et al. (2021). Agrimol B present in Agrimonia pilosa Ledeb impedes cell cycle progression of cancer cells through G(0) state arrest. Biomed. Pharmacother. 141, 111795. doi:10.1016/j.biopha.2021.111795
Hou, W., Xie, Y., Song, X., Sun, X., Lotze, M. T., Zeh, H. J., et al. (2016). Autophagy promotes ferroptosis by degradation of ferritin. Autophagy 12 (8), 1425–1428. doi:10.1080/15548627.2016.1187366
Houtkooper, R. H., Pirinen, E., and Auwerx, J. (2012). Sirtuins as regulators of metabolism and healthspan. Nat. Rev. Mol. Cell Biol. 13 (4), 225–238. doi:10.1038/nrm3293
Hu, T., Shukla, S. K., Vernucci, E., He, C., Wang, D., King, R. J., et al. (2021). Metabolic rewiring by loss of Sirt5 promotes kras-induced pancreatic cancer progression. Gastroenterology 161 (5), 1584–1600. doi:10.1053/j.gastro.2021.06.045
Jeong, S. M., Lee, J., Finley, L. W., Schmidt, P. J., Fleming, M. D., and Haigis, M. C. (2015). SIRT3 regulates cellular iron metabolism and cancer growth by repressing iron regulatory protein 1. Oncogene 34 (16), 2115–2124. doi:10.1038/onc.2014.124
Jiang, L., Kon, N., Li, T., Wang, S. J., Su, T., Hibshoosh, H., et al. (2015). Ferroptosis as a p53-mediated activity during tumour suppression. Nature 520 (7545), 57–62. doi:10.1038/nature14344
Jiang, X., Stockwell, B. R., and Conrad, M. (2021). Ferroptosis: Mechanisms, biology and role in disease. Nat. Rev. Mol. Cell Biol. 22 (4), 266–282. doi:10.1038/s41580-020-00324-8
Jin, Y., Gu, W., and Chen, W. (2021). Sirt3 is critical for p53-mediated ferroptosis upon ROS-induced stress. J. Mol. Cell Biol. 13 (2), 151–154. doi:10.1093/jmcb/mjaa074
Jing, H., Hu, J., He, B., Negrón Abril, Y. L., Stupinski, J., Weiser, K., et al. (2016). A SIRT2-selective inhibitor promotes c-myc oncoprotein degradation and exhibits broad anticancer activity. Cancer Cell 29 (3), 767–768. doi:10.1016/j.ccell.2016.04.005
Kaeberlein, M., McVey, M., and Guarente, L. (1999). The SIR2/3/4 complex and SIR2 alone promote longevity in Saccharomyces cerevisiae by two different mechanisms. Genes Dev. 13 (19), 2570–2580. doi:10.1101/gad.13.19.2570
Kagan, V. E., Mao, G., Qu, F., Angeli, J. P., Doll, S., Croix, C. S., et al. (2017). Oxidized arachidonic and adrenic PEs navigate cells to ferroptosis. Nat. Chem. Biol. 13 (1), 81–90. doi:10.1038/nchembio.2238
Kane, A. E., and Sinclair, D. A. (2018). Sirtuins and NAD(+) in the development and treatment of metabolic and cardiovascular diseases. Circ. Res. 123 (7), 868–885. doi:10.1161/CIRCRESAHA.118.312498
Kerins, M. J., and Ooi, A. (2018). The roles of NRF2 in modulating cellular iron homeostasis. Antioxid. Redox Signal 29 (17), 1756–1773. doi:10.1089/ars.2017.7176
Kim, J. H., Kim, D., Cho, S. J., Jung, K. Y., Kim, J. H., Lee, J. M., et al. (2019). Identification of a novel SIRT7 inhibitor as anticancer drug candidate. Biochem. Biophys. Res. Commun. 508 (2), 451–457. doi:10.1016/j.bbrc.2018.11.120
Kitakata, H., Endo, J., Hashimoto, S., Mizuno, E., Moriyama, H., Shirakawa, K., et al. (2021). Imeglimin prevents heart failure with preserved ejection fraction by recovering the impaired unfolded protein response in mice subjected to cardiometabolic stress. Biochem. Biophys. Res. Commun. 572, 185–190. doi:10.1016/j.bbrc.2021.07.090
Klar, A. J., Fogel, S., and Macleod, K. (1979). MAR1-a regulator of the HMa and HMalpha loci in SACCHAROMYCES CEREVISIAE. Genetics 93 (1), 37–50. doi:10.1093/genetics/93.1.37
Klotz, L. O., Sánchez-Ramos, C., Prieto-Arroyo, I., Urbánek, P., Steinbrenner, H., and Monsalve, M. (2015). Redox regulation of FoxO transcription factors. Redox Biol. 6, 51–72. doi:10.1016/j.redox.2015.06.019
Korotkov, A., Seluanov, A., and Gorbunova, V. (2021). Sirtuin 6: Linking longevity with genome and epigenome stability. Trends Cell Biol. 31 (12), 994–1006. doi:10.1016/j.tcb.2021.06.009
Krishnan, J., Danzer, C., Simka, T., Ukropec, J., Walter, K. M., Kumpf, S., et al. (2012). Dietary obesity-associated Hif1α activation in adipocytes restricts fatty acid oxidation and energy expenditure via suppression of the Sirt2-NAD+ system. Genes Dev. 26 (3), 259–270. doi:10.1101/gad.180406.111
Kroemer, G., El-Deiry, W. S., Golstein, P., Peter, M. E., Vaux, D., Vandenabeele, P., et al. (2005). Classification of cell death: Recommendations of the nomenclature committee on cell death. Cell Death Differ. 16, 1463–1467.
Kumar, S., and Lombard, D. B. (2018). Functions of the sirtuin deacylase SIRT5 in normal physiology and pathobiology. Crit. Rev. Biochem. Mol. Biol. 53 (3), 311–334. doi:10.1080/10409238.2018.1458071
Ladds, M., Popova, G., Pastor-Fernández, A., Kannan, S., van Leeuwen, I. M. M., Håkansson, M., et al. (2020). Exploitation of dihydroorotate dehydrogenase (DHODH) and p53 activation as therapeutic targets: A case study in polypharmacology. J. Biol. Chem. 295 (52), 17935–17949. doi:10.1074/jbc.RA119.012056
Lara, E., Mai, A., Calvanese, V., Altucci, L., Lopez-Nieva, P., Martinez-Chantar, M. L., et al. (2009). Salermide, a Sirtuin inhibitor with a strong cancer-specific proapoptotic effect. Oncogene 28 (6), 781–791. doi:10.1038/onc.2008.436
Lee, J., Kim, Y., Liu, T., Hwang, Y. J., Hyeon, S. J., Im, H., et al. (2018). SIRT3 deregulation is linked to mitochondrial dysfunction in Alzheimer's disease. Aging Cell 17 (1), e12679. doi:10.1111/acel.12679
Lee, J., You, J. H., Kim, M. S., and Roh, J. L. (2020). Epigenetic reprogramming of epithelial-mesenchymal transition promotes ferroptosis of head and neck cancer. Redox Biol. 37, 101697. doi:10.1016/j.redox.2020.101697
Lee, S-H., Lee, J-H., Lee, H-Y., and Min, K-J. (2019). Sirtuin signaling in cellular senescence and aging. BMB Rep. 52 (1), 24–34. doi:10.5483/BMBRep.2019.52.1.290
Lefort, K., Brooks, Y., Ostano, P., Cario-André, M., Calpini, V., Guinea-Viniegra, J., et al. (2013). A miR-34a-SIRT6 axis in the squamous cell differentiation network. Embo J. 32 (16), 2248–2263. doi:10.1038/emboj.2013.156
Li, D., Liu, X., Pi, W., Zhang, Y., Yu, L., Xu, C., et al. (2021). Fisetin attenuates doxorubicin-induced cardiomyopathy in vivo and in vitro by inhibiting ferroptosis through SIRT1/nrf2 signaling pathway activation. Front. Pharmacol. 12, 808480. doi:10.3389/fphar.2021.808480
Li, L., Dong, Z., Yang, J., Li, Q., Lei, Q., Mao, J., et al. (2019). [Progress in roles and mechanisms of deacetylase SIRT7]. Sheng Wu Gong Cheng Xue Bao 35 (1), 13–26. doi:10.13345/j.cjb.180139
Li, M., and Melnick, A. M. (2021). Non-oncogene addiction to SIRT5 in acute myeloid leukemia. Blood Cancer Discov. 2 (3), 198–200. doi:10.1158/2643-3230.BCD-21-0026
Li, X., Wang, Y., Xiong, Y., Wu, J., Ding, H., Chen, X., et al. (2016). Galangin induces autophagy via deacetylation of LC3 by SIRT1 in HepG2 cells. Sci. Rep. 6, 30496. doi:10.1038/srep30496
Li, X. T., Song, J. W., Zhang, Z. Z., Zhang, M. W., Liang, L. R., Miao, R., et al. (2022). Sirtuin 7 mitigates renal ferroptosis, fibrosis and injury in hypertensive mice by facilitating the KLF15/Nrf2 signaling. Free Radic. Biol. Med. 193, 459–473. doi:10.1016/j.freeradbiomed.2022.10.320
Liang, F., Wang, X., Ow, S. H., Chen, W., and Ong, W. C. (2017). Sirtuin 5 is anti-apoptotic and anti-oxidative in cultured SH-ep neuroblastoma cells. Neurotox. Res. 31 (1), 63–76. doi:10.1007/s12640-016-9664-y
Liang, Z., Zhang, N., Wang, X., Zhang, J., Li, K., and Lei, T. (2023). Epothilone B inactivation of Sirtuin1 promotes mitochondrial reactive oxygen species to induce dysfunction and ferroptosis of Schwann cells. Eur. J. Pharm. Sci. 181, 106350. doi:10.1016/j.ejps.2022.106350
Lin, Y., Liu, X., Tan, D., and Jiang, Z. (2020). Atractylon treatment prevents sleep-disordered breathing-induced cognitive dysfunction by suppression of chronic intermittent hypoxia-induced M1 microglial activation. Biosci. Rep. 40 (6). doi:10.1042/BSR20192800
Lin, Z. F., Xu, H. B., Wang, J. Y., Lin, Q., Ruan, Z., Liu, F. B., et al. (2013). SIRT5 desuccinylates and activates SOD1 to eliminate ROS. Biochem. Biophys. Res. Commun. 441 (1), 191–195. doi:10.1016/j.bbrc.2013.10.033
Liu, B., Che, W., Zheng, C., Liu, W., Wen, J., Fu, H., et al. (2013). SIRT5: A safeguard against oxidative stress-induced apoptosis in cardiomyocytes. Cell Physiol. Biochem. 32 (4), 1050–1059. doi:10.1159/000354505
Liu, J., Huang, J., Zhang, Z., Zhang, R., Sun, Q., Zhang, Z., et al. (2022). Mesenchymal stem cell-derived exosomes ameliorate delayed neurocognitive recovery in aged mice by inhibiting Hippocampus ferroptosis via activating SIRT1/nrf2/HO-1 signaling pathway. Oxid. Med. Cell Longev. 2022, 3593294. doi:10.1155/2022/3593294
Liu, L., Li, Y., Cao, D., Qiu, S., Li, Y., Jiang, C., et al. (2021). SIRT3 inhibits gallbladder cancer by induction of AKT-dependent ferroptosis and blockade of epithelial-mesenchymal transition. Cancer Lett. 510, 93–104. doi:10.1016/j.canlet.2021.04.007
Liu, Q., Liu, Y., Li, Y., Hong, Z., Li, S., and Liu, C. (2023). PUM2 aggravates the neuroinflammation and brain damage induced by ischemia-reperfusion through the SLC7A11-dependent inhibition of ferroptosis via suppressing the SIRT1. Mol. Cell Biochem. 478 (3), 609–620. doi:10.1007/s11010-022-04534-w
Liu, S. J., Zhao, Q., Peng, C., Mao, Q., Wu, F., Zhang, F. H., et al. (2021). Design, synthesis, and biological evaluation of nitroisoxazole-containing spiro[pyrrolidin-oxindole] derivatives as novel glutathione peroxidase 4/mouse double minute 2 dual inhibitors that inhibit breast adenocarcinoma cell proliferation. Eur. J. Med. Chem. 217, 113359. doi:10.1016/j.ejmech.2021.113359
Liu, X., Li, D., Pi, W., Wang, B., Xu, S., Yu, L., et al. (2022). LCZ696 protects against doxorubicin-induced cardiotoxicity by inhibiting ferroptosis via AKT/SIRT3/SOD2 signaling pathway activation. Int. Immunopharmacol. 113, 109379. doi:10.1016/j.intimp.2022.109379
Lu, S. C. (2009). Regulation of glutathione synthesis. Mol. Asp. Med. 30 (1-2), 42–59. doi:10.1016/j.mam.2008.05.005
Luo, Y. X., Tang, X., An, X. Z., Xie, X. M., Chen, X. F., Zhao, X., et al. (2017). SIRT4 accelerates Ang II-induced pathological cardiac hypertrophy by inhibiting manganese superoxide dismutase activity. Eur. Heart J. 38 (18), 1389–1398. doi:10.1093/eurheartj/ehw138
Ma, F., Wu, J., Jiang, Z., Huang, W., Jia, Y., Sun, W., et al. (2019). P53/NRF2 mediates SIRT1's protective effect on diabetic nephropathy. Biochim. Biophys. Acta Mol. Cell Res. 1866 (8), 1272–1281. doi:10.1016/j.bbamcr.2019.04.006
Ma, Q. (2013). Role of nrf2 in oxidative stress and toxicity. Annu. Rev. Pharmacol. Toxicol. 53, 401–426. doi:10.1146/annurev-pharmtox-011112-140320
Ma, S., Sun, L., Wu, W., Wu, J., Sun, Z., and Ren, J. (2020). USP22 protects against myocardial ischemia-reperfusion injury via the SIRT1-p53/slc7a11-dependent inhibition of ferroptosis-induced cardiomyocyte death. Front. Physiol. 11, 551318. doi:10.3389/fphys.2020.551318
Mahajan, S. S., Scian, M., Sripathy, S., Posakony, J., Lao, U., Loe, T. K., et al. (2014). Development of pyrazolone and isoxazol-5-one cambinol analogues as sirtuin inhibitors. J. Med. Chem. 57 (8), 3283–3294. doi:10.1021/jm4018064
Mai, A., Valente, S., Meade, S., Carafa, V., Tardugno, M., Nebbioso, A., et al. (2009). Study of 1,4-dihydropyridine structural scaffold: Discovery of novel sirtuin activators and inhibitors. J. Med. Chem. 52 (17), 5496–5504. doi:10.1021/jm9008289
Mandala, A., Chen, W. J., Armstrong, A., Malhotra, M. R., Chavalmane, S., McCommis, K. S., et al. (2021). PPARα agonist fenofibrate attenuates iron-induced liver injury in mice by modulating the Sirt3 and β-catenin signaling. Am. J. Physiol. Gastrointest. Liver Physiol. 321 (4), G262–G269. doi:10.1152/ajpgi.00129.2021
Manjula, R., Anuja, K., and Alcain, F. J. (2020). SIRT1 and SIRT2 activity control in neurodegenerative diseases. Front. Pharmacol. 11, 585821. doi:10.3389/fphar.2020.585821
Mathias, R. A., Greco, T. M., Oberstein, A., Budayeva, H. G., Chakrabarti, R., Rowland, E. A., et al. (2014). Sirtuin 4 is a lipoamidase regulating pyruvate dehydrogenase complex activity. Cell 159 (7), 1615–1625. doi:10.1016/j.cell.2014.11.046
Mercken, E. M., Mitchell, S. J., Martin-Montalvo, A., Minor, R. K., Almeida, M., Gomes, A. P., et al. (2014). SRT2104 extends survival of male mice on a standard diet and preserves bone and muscle mass. Aging Cell 13 (5), 787–796. doi:10.1111/acel.12220
Mi, Y., Wei, C., Sun, L., Liu, H., Zhang, J., Luo, J., et al. (2023). Melatonin inhibits ferroptosis and delays age-related cataract by regulating SIRT6/p-Nrf2/GPX4 and SIRT6/NCOA4/FTH1 pathways. Biomed. Pharmacother. 157, 114048. doi:10.1016/j.biopha.2022.114048
Miao, W., Chen, M., Chen, M., Cui, C., Zhu, Y., Luo, X., et al. (2022). Nr2f2 overexpression aggravates ferroptosis and mitochondrial dysfunction by regulating the PGC-1α signaling in diabetes-induced heart failure mice. Mediat. Inflamm. 2022, 8373389. doi:10.1155/2022/8373389
Michishita, E., Park, J. Y., Burneskis, J. M., Barrett, J. C., and Horikawa, I. (2005). Evolutionarily conserved and nonconserved cellular localizations and functions of human SIRT proteins. Mol. Biol. Cell 16 (10), 4623–4635. doi:10.1091/mbc.e05-01-0033
Milne, J. C., Lambert, P. D., Schenk, S., Carney, D. P., Smith, J. J., Gagne, D. J., et al. (2007). Small molecule activators of SIRT1 as therapeutics for the treatment of type 2 diabetes. Nature 450 (7170), 712–716. doi:10.1038/nature06261
Mu, W. L., Wang, Y. J., Xu, P., Hao, D. L., Liu, X. Z., Wang, T. T., et al. (2015). Sox2 deacetylation by Sirt1 is involved in mouse somatic reprogramming. Stem Cells 33 (7), 2135–2147. doi:10.1002/stem.2012
Murphy, T. H., Miyamoto, M., Sastre, A., Schnaar, R. L., and Coyle, J. T. (1989). Glutamate toxicity in a neuronal cell line involves inhibition of cystine transport leading to oxidative stress. Neuron 2 (6), 1547–1558. doi:10.1016/0896-6273(89)90043-3
Nakamura, Y., Ogura, M., Tanaka, D., and Inagaki, N. (2008). Localization of mouse mitochondrial SIRT proteins: Shift of SIRT3 to nucleus by co-expression with SIRT5. Biochem. Biophys. Res. Commun. 366 (1), 174–179. doi:10.1016/j.bbrc.2007.11.122
Napper, A. D., Hixon, J., McDonagh, T., Keavey, K., Pons, J. F., Barker, J., et al. (2005). Discovery of indoles as potent and selective inhibitors of the deacetylase SIRT1. J. Med. Chem. 48 (25), 8045–8054. doi:10.1021/jm050522v
Nasrin, N., Wu, X., Fortier, E., Feng, Y., Bare, O. C., Chen, S., et al. (2010). SIRT4 regulates fatty acid oxidation and mitochondrial gene expression in liver and muscle cells. J. Biol. Chem. 285 (42), 31995–32002. doi:10.1074/jbc.M110.124164
Nayagam, V. M., Wang, X., Tan, Y. C., Poulsen, A., Goh, K. C., Ng, T., et al. (2006). SIRT1 modulating compounds from high-throughput screening as anti-inflammatory and insulin-sensitizing agents. J. Biomol. Screen 11 (8), 959–967. doi:10.1177/1087057106294710
Novgorodov, S. A., Voltin, J. R., Gooz, M. A., Li, L., Lemasters, J. J., and Gudz, T. I. (2018). Acid sphingomyelinase promotes mitochondrial dysfunction due to glutamate-induced regulated necrosis. J. Lipid Res. 59 (2), 312–329. doi:10.1194/jlr.M080374
Outeiro, T. F., Kontopoulos, E., Altmann, S. M., Kufareva, I., Strathearn, K. E., Amore, A. M., et al. (2007). Sirtuin 2 inhibitors rescue alpha-synuclein-mediated toxicity in models of Parkinson's disease. Science 317 (5837), 516–519. doi:10.1126/science.1143780
Ouyang, S., Zhang, Q., Lou, L., Zhu, K., Li, Z., Liu, P., et al. (2022). The double-edged sword of SIRT3 in cancer and its therapeutic applications. Front. Pharmacol. 13, 871560. doi:10.3389/fphar.2022.871560
Pacholec, M., Bleasdale, J. E., Chrunyk, B., Cunningham, D., Flynn, D., Garofalo, R. S., et al. (2010). SRT1720, SRT2183, SRT1460, and resveratrol are not direct activators of SIRT1. J. Biol. Chem. 285 (11), 8340–8351. doi:10.1074/jbc.M109.088682
Pan, H., Guan, D., Liu, X., Li, J., Wang, L., Wu, J., et al. (2016). SIRT6 safeguards human mesenchymal stem cells from oxidative stress by coactivating NRF2. Cell Res. 26 (2), 190–205. doi:10.1038/cr.2016.4
Pantopoulos, K. (2004). Iron metabolism and the IRE/IRP regulatory system: An update. Ann. N. Y. Acad. Sci. 1012, 1–13. doi:10.1196/annals.1306.001
Park, J., Chen, Y., Tishkoff, D. X., Peng, C., Tan, M., Dai, L., et al. (2013). SIRT5-mediated lysine desuccinylation impacts diverse metabolic pathways. Mol. Cell 50 (6), 919–930. doi:10.1016/j.molcel.2013.06.001
Peng, Q., Chen, X., Liang, X., Ouyang, J., Wang, Q., Ren, S., et al. (2023). Metformin improves polycystic ovary syndrome in mice by inhibiting ovarian ferroptosis. Front. Endocrinol. (Lausanne) 14, 1070264. doi:10.3389/fendo.2023.1070264
Pi, H., Xu, S., Reiter, R. J., Guo, P., Zhang, L., Li, Y., et al. (2015). SIRT3-SOD2-mROS-dependent autophagy in cadmium-induced hepatotoxicity and salvage by melatonin. Autophagy 11 (7), 1037–1051. doi:10.1080/15548627.2015.1052208
Pietsch, E. C., Chan, J. Y., Torti, F. M., and Torti, S. V. (2003). Nrf2 mediates the induction of ferritin H in response to xenobiotics and cancer chemopreventive dithiolethiones. J. Biol. Chem. 278 (4), 2361–2369. doi:10.1074/jbc.M210664200
Polletta, L., Vernucci, E., Carnevale, I., Arcangeli, T., Rotili, D., Palmerio, S., et al. (2015). SIRT5 regulation of ammonia-induced autophagy and mitophagy. Autophagy 11 (2), 253–270. doi:10.1080/15548627.2015.1009778
Ponnusamy, M., Zhuang, M. A., Zhou, X., Tolbert, E., Bayliss, G., Zhao, T. C., et al. (2015). Activation of sirtuin-1 promotes renal fibroblast activation and aggravates renal fibrogenesis. J. Pharmacol. Exp. Ther. 354 (2), 142–151. doi:10.1124/jpet.115.224386
Puigserver, P., Rhee, J., Donovan, J., Walkey, C. J., Yoon, J. C., Oriente, F., et al. (2003). Insulin-regulated hepatic gluconeogenesis through FOXO1-PGC-1alpha interaction. Nature 423 (6939), 550–555. doi:10.1038/nature01667
Qi, Y. L., Wang, H. R., Chen, L. L., Duan, Y. T., Yang, S. Y., and Zhu, H. L. (2022). Recent advances in small-molecule fluorescent probes for studying ferroptosis. Chem. Soc. Rev. 51 (18), 7752–7778. doi:10.1039/d1cs01167g
Qiu, X., Brown, K., Hirschey, M. D., Verdin, E., and Chen, D. (2010). Calorie restriction reduces oxidative stress by SIRT3-mediated SOD2 activation. Cell Metab. 12 (6), 662–667. doi:10.1016/j.cmet.2010.11.015
Rajabi, N., Galleano, I., Madsen, A. S., and Olsen, C. A. (2018). Targeting sirtuins: Substrate specificity and inhibitor design. Prog. Mol. Biol. Transl. Sci. 154, 25–69. doi:10.1016/bs.pmbts.2017.11.003
Rardin, M. J., He, W., Nishida, Y., Newman, J. C., Carrico, C., Danielson, S. R., et al. (2013). SIRT5 regulates the mitochondrial lysine succinylome and metabolic networks. Cell Metab. 18 (6), 920–933. doi:10.1016/j.cmet.2013.11.013
Rodgers, J. T., Lerin, C., Haas, W., Gygi, S. P., Spiegelman, B. M., and Puigserver, P. (2005). Nutrient control of glucose homeostasis through a complex of PGC-1alpha and SIRT1. Nature 434 (7029), 113–118. doi:10.1038/nature03354
Rogina, B., and Helfand, S. L. (2004). Sir2 mediates longevity in the fly through a pathway related to calorie restriction. Proc. Natl. Acad. Sci. U. S. A. 101 (45), 15998–16003. doi:10.1073/pnas.0404184101
Rollnik, J. D. (2015). [Huntington's disease]. Nervenarzt 86 (6), 725–735. doi:10.1007/s00115-015-4306-9
Roth, M., Wang, Z., and Chen, W. Y. (2013). Sirtuins in hematological aging and malignancy. Crit. Rev. Oncog. 18 (6), 531–547. doi:10.1615/critrevoncog.2013010187
Rumpf, T., Gerhardt, S., Einsle, O., and Jung, M. (2015). Seeding for sirtuins: Microseed matrix seeding to obtain crystals of human Sirt3 and Sirt2 suitable for soaking. Acta Crystallogr. F. Struct. Biol. Commun. 71, 1498–1510. doi:10.1107/S2053230X15019986
Rumpf, T., Schiedel, M., Karaman, B., Roessler, C., North, B. J., Lehotzky, A., et al. (2015). Selective Sirt2 inhibition by ligand-induced rearrangement of the active site. Nat. Commun. 6, 6263. doi:10.1038/ncomms7263
Sands, B. E., Joshi, S., Haddad, J., Freudenberg, J. M., Oommen, D. E., Hoffmann, E., et al. (2016). Assessing colonic exposure, safety, and clinical activity of SRT2104, a novel oral SIRT1 activator, in patients with mild to moderate ulcerative colitis. Inflamm. Bowel Dis. 22 (3), 607–614. doi:10.1097/MIB.0000000000000597
Santana-Codina, N., Gikandi, A., and Mancias, J. D. (2021). The role of NCOA4-mediated ferritinophagy in ferroptosis. Adv. Exp. Med. Biol. 1301, 41–57. doi:10.1007/978-3-030-62026-4_4
Semova, I., Levenson, A. E., Krawczyk, J., Bullock, K., Gearing, M. E., Ling, A. V., et al. (2022). Insulin prevents hypercholesterolemia by suppressing 12α-hydroxylated bile acids. Circulation 145 (13), 969–982. doi:10.1161/CIRCULATIONAHA.120.045373
Shi, H., Deng, H. X., Gius, D., Schumacker, P. T., Surmeier, D. J., and Ma, Y. C. (2017). Sirt3 protects dopaminergic neurons from mitochondrial oxidative stress. Hum. Mol. Genet. 26 (10), 1915–1926. doi:10.1093/hmg/ddx100
Shi, T., Wang, F., Stieren, E., and Tong, Q. (2005). SIRT3, a mitochondrial sirtuin deacetylase, regulates mitochondrial function and thermogenesis in Brown adipocytes. J. Biol. Chem. 280 (14), 13560–13567. doi:10.1074/jbc.M414670200
Shin, D., Kim, E. H., Lee, J., and Roh, J. L. (2018). Nrf2 inhibition reverses resistance to GPX4 inhibitor-induced ferroptosis in head and neck cancer. Free Radic. Biol. Med. 129, 454–462. doi:10.1016/j.freeradbiomed.2018.10.426
Sidorova-Darmos, E., Sommer, R., and Eubanks, J. H. (2018). The role of SIRT3 in the brain under physiological and pathological conditions. Front. Cell Neurosci. 12, 196. doi:10.3389/fncel.2018.00196
Sosa, V., Moliné, T., Somoza, R., Paciucci, R., Kondoh, H., and Me, L. L. (2013). Oxidative stress and cancer: An overview. Ageing Res. Rev. 12 (1), 376–390. doi:10.1016/j.arr.2012.10.004
Spangler, B., Morgan, C. W., Fontaine, S. D., Vander Wal, M. N., Chang, C. J., Wells, J. A., et al. (2016). A reactivity-based probe of the intracellular labile ferrous iron pool. Nat. Chem. Biol. 12 (9), 680–685. doi:10.1038/nchembio.2116
Stockwell, B. R. (2022). Ferroptosis turns 10: Emerging mechanisms, physiological functions, and therapeutic applications. Cell 185 (14), 2401–2421. doi:10.1016/j.cell.2022.06.003
Stockwell, B. R., Friedmann Angeli, J. P., Bayir, H., Bush, A. I., Conrad, M., Dixon, S. J., et al. (2017). Ferroptosis: A regulated cell death nexus linking metabolism, redox biology, and disease. Cell 171 (2), 273–285. doi:10.1016/j.cell.2017.09.021
Su, G., Yang, W., Wang, S., Geng, C., and Guan, X. (2021). SIRT1-autophagy axis inhibits excess iron-induced ferroptosis of foam cells and subsequently increases IL-1Β and IL-18. Biochem. Biophys. Res. Commun. 561, 33–39. doi:10.1016/j.bbrc.2021.05.011
Sui, S., Zhang, J., Xu, S., Wang, Q., Wang, P., and Pang, D. (2019). Ferritinophagy is required for the induction of ferroptosis by the bromodomain protein BRD4 inhibitor (+)-JQ1 in cancer cells. Cell Death Dis. 10 (5), 331. doi:10.1038/s41419-019-1564-7
Sundriyal, S., Moniot, S., Mahmud, Z., Yao, S., Di Fruscia, P., Reynolds, C. R., et al. (2017). Thienopyrimidinone based sirtuin-2 (SIRT2)-Selective inhibitors bind in the ligand induced selectivity pocket. J. Med. Chem. 60 (5), 1928–1945. doi:10.1021/acs.jmedchem.6b01690
Suzuki, T., Khan, M. N., Sawada, H., Imai, E., Itoh, Y., Yamatsuta, K., et al. (2012). Design, synthesis, and biological activity of a novel series of human sirtuin-2-selective inhibitors. J. Med. Chem. 55 (12), 5760–5773. doi:10.1021/jm3002108
Tang, X., Li, X., Zhang, D., and Han, W. (2022). Astragaloside-IV alleviates high glucose-induced ferroptosis in retinal pigment epithelial cells by disrupting the expression of miR-138-5p/Sirt1/Nrf2. Bioengineered 13 (4), 8240–8254. doi:10.1080/21655979.2022.2049471
Tao, R., Coleman, M. C., Pennington, J. D., Ozden, O., Park, S. H., Jiang, H., et al. (2010). Sirt3-mediated deacetylation of evolutionarily conserved lysine 122 regulates MnSOD activity in response to stress. Mol. Cell 40 (6), 893–904. doi:10.1016/j.molcel.2010.12.013
Teixeira, C. S. S., Cerqueira, N., Gomes, P., and Sousa, S. F. (2020). A molecular perspective on sirtuin activity. Int. J. Mol. Sci. 21 (22), 8609. doi:10.3390/ijms21228609
Tissenbaum, H. A., and Guarente, L. (2001). Increased dosage of a sir-2 gene extends lifespan in Caenorhabditis elegans. Nature 410 (6825), 227–230. doi:10.1038/35065638
Trapp, J., Meier, R., Hongwiset, D., Kassack, M. U., Sippl, W., and Jung, M. (2007). Structure-activity studies on suramin analogues as inhibitors of NAD+-dependent histone deacetylases (sirtuins). ChemMedChem 2 (10), 1419–1431. doi:10.1002/cmdc.200700003
Vakhrusheva, O., Smolka, C., Gajawada, P., Kostin, S., Boettger, T., Kubin, T., et al. (2008). Sirt7 increases stress resistance of cardiomyocytes and prevents apoptosis and inflammatory cardiomyopathy in mice. Circ. Res. 102 (6), 703–710. doi:10.1161/CIRCRESAHA.107.164558
van der Meer, A. J., Scicluna, B. P., Moerland, P. D., Lin, J., Jacobson, E. W., Vlasuk, G. P., et al. (2015). The selective sirtuin 1 activator SRT2104 reduces endotoxin-induced cytokine release and coagulation activation in humans. Crit. Care Med. 43 (6), e199–e202. doi:10.1097/CCM.0000000000000949
Vaquero, A. (2009). The conserved role of sirtuins in chromatin regulation. Int. J. Dev. Biol. 53 (2-3), 303–322. doi:10.1387/ijdb.082675av
Viswanathan, M., and Guarente, L. (2011). Regulation of Caenorhabditis elegans lifespan by sir-2.1 transgenes. Nature 477 (7365), E1–E2. doi:10.1038/nature10440
Wang, C., Liu, T., Tong, Y., Cui, R., Qu, K., Liu, C., et al. (2021). Ulinastatin protects against acetaminophen-induced liver injury by alleviating ferroptosis via the SIRT1/NRF2/HO-1 pathway. Am. J. Transl. Res. 13 (6), 6031–6042.
Wang, D., Yin, K., Zhang, Y., Lu, H., Hou, L., Zhao, H., et al. (2023). Novel pathways of fluoride-induced hepatotoxicity: P53-dependent ferroptosis induced by the SIRT1/FOXOs pathway and Nrf2/HO-1 pathway. Comp. Biochem. Physiol. C Toxicol. Pharmacol. 264, 109526. doi:10.1016/j.cbpc.2022.109526
Wang, F., Wang, K., Xu, W., Zhao, S., Ye, D., Wang, Y., et al. (2017). SIRT5 desuccinylates and activates pyruvate kinase M2 to block macrophage IL-1β production and to prevent DSS-induced colitis in mice. Cell Rep. 19 (11), 2331–2344. doi:10.1016/j.celrep.2017.05.065
Wang, J., Liu, Y., Wang, Y., and Sun, L. (2021). The cross-link between ferroptosis and kidney diseases. Oxid. Med. Cell Longev. 2021, 6654887. doi:10.1155/2021/6654887
Wang, L., Jiang, X. L., Zhang, W. M., Li, F., Khan, A. A., Liu, X., et al. (2017). Homo-aro-cholestane, furostane and spirostane saponins from the tubers of Ophiopogon japonicus. Phytochemistry 136, 125–132. doi:10.1016/j.phytochem.2017.01.006
Wang, L., Sun, M., Cao, Y., Ma, L., Shen, Y., Velikanova, A. A., et al. (2020). miR-34a regulates lipid metabolism by targeting SIRT1 in non-alcoholic fatty liver disease with iron overload. Arch. Biochem. Biophys. 695, 108642. doi:10.1016/j.abb.2020.108642
Wang, M., and Lin, H. (2021). Understanding the function of mammalian sirtuins and protein lysine acylation. Annu. Rev. Biochem. 90, 245–285. doi:10.1146/annurev-biochem-082520-125411
Wang, X., Yang, Y., Xiao, A., Zhang, N., Miao, M., Wang, Z., et al. (2022). A comparative study of the effect of a gentle ketogenic diet containing medium-chain or long-chain triglycerides on chronic sleep deprivation-induced cognitive deficiency. Food Funct. 13 (4), 2283–2294. doi:10.1039/d1fo04087a
Wang, X. X., Wang, X. L., Tong, M. M., Gan, L., Chen, H., Wu, S. S., et al. (2016). SIRT6 protects cardiomyocytes against ischemia/reperfusion injury by augmenting FoxO3α-dependent antioxidant defense mechanisms. Basic Res. Cardiol. 111 (2), 13. doi:10.1007/s00395-016-0531-z
Wang, Y., Wang, S., Xin, Y., Zhang, J., Wang, S., Yang, Z., et al. (2021). Hydrogen sulfide alleviates the anxiety-like and depressive-like behaviors of type 1 diabetic mice via inhibiting inflammation and ferroptosis. Life Sci. 278, 119551. doi:10.1016/j.lfs.2021.119551
Wang, Y. P., Zhou, L. S., Zhao, Y. Z., Wang, S. W., Chen, L. L., Liu, L. X., et al. (2014). Regulation of G6PD acetylation by SIRT2 and KAT9 modulates NADPH homeostasis and cell survival during oxidative stress. EMBO J. 33 (12), 1304–1320. doi:10.1002/embj.201387224
Wortmann, M., Schneider, M., Pircher, J., Hellfritsch, J., Aichler, M., Vegi, N., et al. (2013). Combined deficiency in glutathione peroxidase 4 and vitamin E causes multiorgan thrombus formation and early death in mice. Circ. Res. 113 (4), 408–417. doi:10.1161/CIRCRESAHA.113.279984
Xiao, Q., Xiong, Z., Yu, C., Zhou, J., Shen, Q., Wang, L., et al. (2019). Antidepressant activity of crocin-I is associated with amelioration of neuroinflammation and attenuates oxidative damage induced by corticosterone in mice. Physiol. Behav. 212, 112699. doi:10.1016/j.physbeh.2019.112699
Xie, Y., Hou, W., Song, X., Yu, Y., Huang, J., Sun, X., et al. (2016). Ferroptosis: Process and function. Cell Death Differ. 23 (3), 369–379. doi:10.1038/cdd.2015.158
Yagoda, N., von Rechenberg, M., Zaganjor, E., Bauer, A. J., Yang, W. S., Fridman, D. J., et al. (2007). RAS-RAF-MEK-dependent oxidative cell death involving voltage-dependent anion channels. Nature 447 (7146), 864–868. doi:10.1038/nature05859
Yan, N., and Zhang, J. (2019). Iron metabolism, ferroptosis, and the links with Alzheimer's disease. Front. Neurosci. 13, 1443. doi:10.3389/fnins.2019.01443
Yang, L., Ma, X., Yuan, C., He, Y., Li, L., Fang, S., et al. (2017). Discovery of 2-((4,6-dimethylpyrimidin-2-yl)thio)-N-phenylacetamide derivatives as new potent and selective human sirtuin 2 inhibitors. Eur. J. Med. Chem. 134, 230–241. doi:10.1016/j.ejmech.2017.04.010
Yang, W. S., SriRamaratnam, R., Welsch, M. E., Shimada, K., Skouta, R., Viswanathan, V. S., et al. (2014). Regulation of ferroptotic cancer cell death by GPX4. Cell 156 (1-2), 317–331. doi:10.1016/j.cell.2013.12.010
Yang, X., Liu, Q., Li, Y., Tang, Q., Wu, T., Chen, L., et al. (2020). The diabetes medication canagliflozin promotes mitochondrial remodelling of adipocyte via the AMPK-Sirt1-Pgc-1α signalling pathway. Adipocyte 9 (1), 484–494. doi:10.1080/21623945.2020.1807850
Yang, X., Park, S. H., Chang, H. C., Shapiro, J. S., Vassilopoulos, A., Sawicki, K. T., et al. (2017). Sirtuin 2 regulates cellular iron homeostasis via deacetylation of transcription factor NRF2. J. Clin. Invest. 127 (4), 1505–1516. doi:10.1172/JCI88574
Yoo, A., Narayan, V. P., Hong, E. Y., Whang, W. K., and Park, T. (2017). Scopolin ameliorates high-fat diet induced hepatic steatosis in mice: Potential involvement of SIRT1-mediated signaling cascades in the liver. Sci. Rep. 7 (1), 2251. doi:10.1038/s41598-017-02416-6
Yoshida, M., Minagawa, S., Araya, J., Sakamoto, T., Hara, H., Tsubouchi, K., et al. (2019). Involvement of cigarette smoke-induced epithelial cell ferroptosis in COPD pathogenesis. Nat. Commun. 10 (1), 3145. doi:10.1038/s41467-019-10991-7
Yung, H. W., Alnæs-Katjavivi, P., Jones, C. J., El-Bacha, T., Golic, M., Staff, A. C., et al. (2016). Placental endoplasmic reticulum stress in gestational diabetes: The potential for therapeutic intervention with chemical chaperones and antioxidants. Diabetologia 59 (10), 2240–2250. doi:10.1007/s00125-016-4040-2
Zeng, Y., Cao, G., Lin, L., Zhang, Y., Luo, X., Ma, X., et al. (2023). Resveratrol attenuates sepsis-induced cardiomyopathy in rats through anti-ferroptosis via the sirt1/nrf2 pathway. J. Invest. Surg. 36 (1), 2157521. doi:10.1080/08941939.2022.2157521
Zhang, G., Yuan, C., Su, X., Zhang, J., Gokulnath, P., Vulugundam, G., et al. (2022). Relevance of ferroptosis to cardiotoxicity caused by anthracyclines: Mechanisms to target treatments. Front. Cardiovasc Med. 9, 896792. doi:10.3389/fcvm.2022.896792
Zhang, L., Yang, X., Li, X., Li, C., Zhao, L., Zhou, Y., et al. (2015). Butein sensitizes HeLa cells to cisplatin through the AKT and ERK/p38 MAPK pathways by targeting FoxO3a. Int. J. Mol. Med. 36 (4), 957–966. doi:10.3892/ijmm.2015.2324
Zhang, S., Xin, W., Anderson, G. J., Li, R., Gao, L., Chen, S., et al. (2022). Double-edge sword roles of iron in driving energy production versus instigating ferroptosis. Cell Death Dis. 13 (1), 40. doi:10.1038/s41419-021-04490-1
Zhang, X., Song, T., Zhao, M., Tao, X., Zhang, B., Sun, C., et al. (2022). Sirtuin 2 alleviates chronic neuropathic pain by suppressing ferroptosis in rats. Front. Pharmacol. 13, 827016. doi:10.3389/fphar.2022.827016
Zhou, B., Liu, J., Kang, R., Klionsky, D. J., Kroemer, G., and Tang, D. (2020). Ferroptosis is a type of autophagy-dependent cell death. Semin. Cancer Biol. 66, 89–100. doi:10.1016/j.semcancer.2019.03.002
Zhou, Q., and Ruan, D. (2022). SIRT1-autophagy axis may inhibit oxidative stress-induced ferroptosis in human nucleus pulposus cells. Med. Hypotheses 159, 110757. doi:10.1016/j.mehy.2021.110757
Zhou, Z., Ye, T. J., DeCaro, E., Buehler, B., Stahl, Z., Bonavita, G., et al. (2020). Intestinal SIRT1 deficiency protects mice from ethanol-induced liver injury by mitigating ferroptosis. Am. J. Pathol. 190 (1), 82–92. doi:10.1016/j.ajpath.2019.09.012
Zi, Y., Wang, X., Zi, Y., Yu, H., Lan, Y., Fan, Y., et al. (2023). Cigarette smoke induces the ROS accumulation and iNOS activation through deactivation of Nrf-2/SIRT3 axis to mediate the human bronchial epithelium ferroptosis. Free Radic. Biol. Med. 200, 73–86. doi:10.1016/j.freeradbiomed.2023.03.002
Keywords: sirtuins, ferroptosis, iron metabolism, lipid peroxidation, reactive oxygen species
Citation: Zeng J, Guo J, Huang S, Cheng Y, Luo F, Xu X, Chen R, Ma G and Wang Y (2023) The roles of sirtuins in ferroptosis. Front. Physiol. 14:1131201. doi: 10.3389/fphys.2023.1131201
Received: 24 December 2022; Accepted: 10 April 2023;
Published: 20 April 2023.
Edited by:
Nitish R. Mahapatra, Indian Institute of Technology Madras, IndiaReviewed by:
Prasanna Kumar Reddy Allu, Joslin Diabetes Center and Harvard Medical School, United StatesNinitha Asirvatham-Jeyaraj, Indian Institute of Technology Madras, India
Copyright © 2023 Zeng, Guo, Huang, Cheng, Luo, Xu, Chen, Ma and Wang. This is an open-access article distributed under the terms of the Creative Commons Attribution License (CC BY). The use, distribution or reproduction in other forums is permitted, provided the original author(s) and the copyright owner(s) are credited and that the original publication in this journal is cited, in accordance with accepted academic practice. No use, distribution or reproduction is permitted which does not comply with these terms.
*Correspondence: Guoda Ma, c2loYW4xMTA3QDEyNi5jb20=; Yajun Wang, eWFqdW53QGdkbXUuZWR1LmNu