- 1Faculty of Sport and Health Sciences, University of Jyväskylä, Jyväskylä, Finland
- 2Paavo Nurmi Centre & Unit for Health and Physical Activity, University of Turku, Turku, Finland
- 3Finnish Institute of High Performance Sport KIHU, Jyväskylä, Finland
- 4Central Finland Healthcare District, Department of Medicine, Jyväskylä, Finland
- 5Department of Medicine, Institute of Clinical Medicine, University of Eastern Finland, Kuopio, Finland
Introduction: This is one of the first intervention studies to examine how low- (LIT) and high-intensity endurance training (HIT) affect durability, defined as ‘time of onset and magnitude of deterioration in physiological-profiling characteristics over time during prolonged exercise’.
Methods: Sedentary and recreationally active men (n = 16) and women (n = 19) completed either LIT (average weekly training time 6.8
Results: When all three factors were averaged the durability was improved similarly (time x group p = 0.42) in both groups (LIT: p = 0.03, g = 0.49; HIT: p = 0.01, g = 0.62). In the LIT group, magnitude of average of drifts and their onset did not reach statistically significance level of p < 0.05 (magnitude: 7.7
Conclusion: Durability improved similarly by both LIT and HIT based on reduced physiological drifts, their postponed onsets, and changes in physiological strain. Despite durability enhanced among untrained people, a 10-week intervention did not alter drifts and their onsets in a large amount, even though it attenuated physiological strain.
1 Introduction
Recently, the term durability has been defined as ‘time of onset and magnitude of deterioration in physiological-profiling characteristics during prolonged exercise’ (Maunder et al., 2021). Durability can be seen as a form of fatigue resistance. However, fatigue resistance is usually connected to neuromuscular fatigue during maximal and short performances. Durability, on the other hand, is usually linked to gradual fatiguing process in prolonged (i.e. several hours) submaximal exercise with usually physiological or psychological origin. Durability may be estimated directly by evaluating the magnitudes and onsets of drifts, defined as gradual changes in key physiological variables during prolonged submaximal exercise (Smyth et al., 2022). Durability may also be assessed by measuring a maximal performance capacity immediately after prolonged exercise (Maunder et al., 2021; Van Erp et al., 2021). Decreased absolute physiological responses, e.g. lactate and heart rate level, during submaximal exercise after a training period may also represent attenuated physiological responses and thus improved durability.
Many physiological drifts occur during prolonged low-intensity exercise, e.g. increased energy expenditure (EE) (Rønnestad et al., 2011; Hopker et al., 2017), which is coupled with upward drifts of ventilation (VE) (Ekelund, 1967; Martin et al., 1981; Hopker et al., 2017), rate of perceived exertion (RPE) (Rønnestad et al., 2011; Hopker et al., 2017; Wills et al., 2019), and heart rate (HR) (Ekelund, 1967; Rønnestad et al., 2011; Mullins et al., 2015). HR drift is the most widely used drift when studying durability (Maunder et al., 2021; Smyth et al., 2022), and it is also related to neuromuscular fatigue (Coyle and González-Alonso, 2001). Inversely, stroke volume (SV) (Ekelund, 1967) and linear heart rate variability (HRV) indices (Moreno et al., 2013) form downward drifts.
High durability, i.e., ability to resist physiological changes, has clear advantages. For example, a marathoner with high durability can resist the inevitable performance deteriorating changes and maintain performance capacity for prolonged periods of time. In line with this, lower HR drift in marathon running is associated with prolonged onset of drift and faster relative speed (Smyth et al., 2022) and greater HR drift is associated with decrease in running speed at the later stage of the race (Billat et al., 2020) as well as worse total time (Billat et al., 2020; Smyth et al., 2022). Larger HR drift predicts also decreased maximal oxygen uptake (VO2max) at fatigued state (Wingo et al., 2005). EE drift has been strongly associated with a decrease in 5 min maximum performance in fatigued state (Passfield and Doust, 2000). Lately, it has been demonstrated that power profile does not decline after exhausting exercise (1500–2000 kJ) as much in successful professional cyclists compared to lesser successful ones (Van Erp et al., 2021). In addition, durability is a required feature in military training, because physical and mental functionality of soldiers should be maintained at high levels also in a fatigued state (Rudzki, 1989; Wills et al., 2019).
The most effective training methods to improve durability are not known. In some studies, strenuous (Hurley et al., 1986; Coggan et al., 1993) and high-volume moderate-intensity (Phillips et al., 1996; Carter et al., 2001) endurance training has led to reduced physiological drifts during prolonged exercise among untrained individuals. Well-trained athletes have not increased durability with endurance training alone (Hausswirth et al., 2010; Rønnestad et al., 2011), although the amount of low-intensity training (LIT) may be linked to increased durability (Spragg et al., 2022). Concurrent strength and endurance training has had a positive effect among athletes (Rønnestad et al., 2011).
There are no reports on intervention studies focusing on durability. Therefore, we wanted to understand how much and in which methods durability can be improved. Our main aim was to study durability before and after high- (HIT) and low-intensity endurance training of 10 weeks in recreationally active participants.
2 Materials and methods
2.1 Study design and protocol
Based on VO2 drifts in interventions (Coggan et al., 1993; Carter et al., 2001) and our pilot studies, we estimated that intervention would cause an average 3 p. p. (percentage point) reduction in EE drift in the HIT group, and 6 p. p. reduction in the LIT group with 3 p. p. standard deviation. Based on these findings, power calculations give group sample size of 16 (
The study included 6 visits to the laboratory (Figure 1): three before the training intervention, one during intervention, and two after intervention. All subjects provided written informed consent, and the study was approved by the Ethical Committee of the Central Finland Healthcare District (8U/2020), complying with the Declaration of Helsinki.
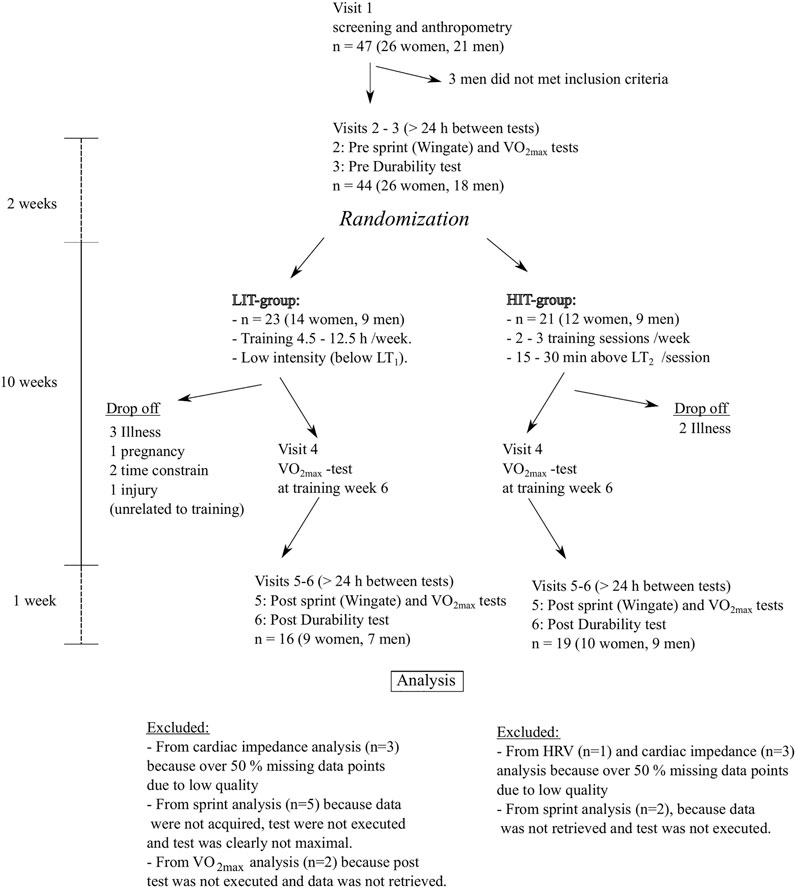
FIGURE 1. Flow chart and study design. Performance tests included VO2max and sprint tests, and durability. LIT Low intensity training. HIT High intensity training. HRV heart rate variability. VO2max maximal oxygen uptake. LT1 First lactate threshold. LT2 Second lactate threshold.
2.2 Subjects
Healthy sedentary or recreationally active (endurance exercise less than 6 h/wk) adults aged 18–40 years were recruited through social and print media announcements. Additionally, participants were not included if they had hypertension, pregnancy, or were nursing, or they had been diagnosed an upper respiratory infection or other acute illnesses within 2-weeks before the first laboratory visit. A total of 47 subjects were invited to the screening visit which included assessments of body height, resting ECG, blood pressure, health status, and medication (Figure 1: flow chart). Body mass and fat free mass were measured by bioelectrical impedance (InBody 770, Biospace Ltd., Seoul, Korea) in the morning after at least 8 h fasting. Forty-four subjects met the inclusion criteria and started the training intervention. Finally, 35 subjects (basic characteristics in Table 1) finished the entire study.
2.3 Laboratory tests and analyses
Visit 2, 4, & 5: The sprint (Wingate) and VO2max were performed individually at the same time of the day (±2 h). Visit 4 included only the VO2max-test. The subjects were advised to refrain from caffeine and alcohol 24 h before the tests and eating 3 h prior. Before each performance test, body mass was recorded (seca 719, seca GmbH & Co. KG., Hamburg, Germany) with cycling clothes on and shoes removed, then 300 g were subtracted as the weight of clothes.
15 s Wingate test (sprinting ability) was used to measure a fatigue of the neuromuscular system after a durability test. A 10-min warming up at 50 W followed by 10 s countdown phase to reach maximum pedaling rate with the load (7.5% of body weight) followed by 15 s all out maximal cycling (Monark 894E, Monark Exercise AB, Sweden). Subjects were verbally encouraged by researchers. Subjects were familiarized with the Wingate test on a separate day before the test.
Incremental VO2max cycling test. After the Wingate test, subjects remained sitting for 10 min, followed by a 25-min active recovery (walking around or 50 W cycling). Exactly 35 min after termination of the Wingate test, a step-incremental cycling test (Monark LC4, Monark Exercise AB, Sweden) was initiated. Initial power was 40 W for women and 50 W for men with increments of 20—25 W (women) and 30 W (men) at 3-min intervals. Subjects were verbally encouraged during the last stages by researchers. The ergometer (Monark LC4) was calibrated at the beginning of the study. Calibration was checked weekly with a 4000 g weight, following instructions by the manufacturer. Gas exchange was measured breath-by-breath (Jaeger Vyntus TM CPX, CareFusion Germany 234 GmbH, Hoechberg, Germany) and HR was monitored with a Garmin Forerunner 945 (Garmin Ltd., Taiwan). Means from the last minute of every stage were used in analysis. In the last minute of each state, blood lactate was measured by fingertip sampling (EKF-diagnostic GmbH Ebendorfer Chaussee 3, Germany) and RPE (Borg Scale 0–10) was recorded. VO2max was defined as the highest continuous 60 s mean oxygen consumption. Maximal aerobic power (Pmax) was defined as the weighted mean of the last 3 min of the test: power of last completed stage (W) + [time (s) of unfinished state]/(180 s)
Durability is defined as ‘time of onset and magnitude of deterioration in physiological-profiling characteristics over time during prolonged exercise’ (Maunder et al., 2021). In this study, the original definition of durability was interpreted through three different factors:
1) The magnitude of physiological drifts (defined as a gradual change in physiological variables during exercise): Change in EE, HR, RPE, VE, imputed left ventricular ejection time (LVET), and imputed stroke volume (SV) between 30 min and 180 min in the durability test.
2) The time of onset of a drift, which was defined to be the time when the value of the drift has risen (or fallen) a predetermined amount. The thresholds were arbitrarily chosen, as in (Smyth et al., 2022), and they were +5% (HR, VE), +2.5% (EE), +2 steps (RPE), and -1.5% (imputed LVET).
3) The physiological strain, which was defined as the absolute levels of HR, HRV, RPE, and blood lactate from the durability test. The effect of prolonged exercise on sprinting performance was done by inspecting the sprinting fatigue ratio:
To get a wider overall picture, durability was not understood as a single variable, but rather as a phenomenon which is illustrated by all these three different factors together.
Visit 3 and 6: Durability tests were performed individually for each subject at the same time of the day (±2 h). The subjects were advised to have a standard meal 2.5–3 h before entering the laboratory. They were instructed to document the timing and contents of the meal, and to repeat it before the second durability test. Before the durability test, body mass was measured (Seca 719), then followed by a rest period on bench while being prepared for the non-invasive impedance cardiography (Physioflow, 2016) (PhysioFlow PF-07 Enduro, Manatec Biomedical, France) with PF50 PhysioFlow electrodes to measure SV and LVET.
Thereafter, the subjects cycled for 3 h (Monark LC4) with a predetermined power, 50% VO2max, measured on incremental test, but not more than 95% LT1 to ensure that each participant cycled at low intensity zone. In pre-test the realization was 48 ± 4% VO2max and 87
Hydration (0.3% NaCl solution) was available ad libitum. Water intake during 3 h tests was measured and in the LIT group, it was 1.2 (0.4) l and 1.1 (0.4) l in pre- and post-tests, respectively. In the HIT group, the respective values were 1.3 (0.4) l and 1.1 (0.4) l. During the test, carbohydrates were given as a 2:1:1 mixture containing maltodextrin, fructose, and glucose dissolved into 1 dl of 0.3% NaCl. Carbohydrate intake was individualized, and the amount was related to the cycling power, calculated to cover 50% of theoretical energy expenditure where 18% gross efficiency was assumed (Ettema and Loras, 2009). A maximum of 75 g of carbohydrates were given per hour to minimize gastrointestinal problems (Jeukendrup, 2014). The carbohydrate intake was identical in pre- and post-tests (mean ± SD: 51
Energy expenditure (EE) was calculated using equation
Heart rate variability (HRV), cardiac impedance, and multiple imputations. In the HRV analysis, low frequency (0.04–0.15 Hz) and high frequency (0.15–1.1 Hz) bands were used. The higher than usual frequency limit of 1.1 Hz was chosen to include the respiratory frequency during exercise. HR and HRV data were collected with Garmin HRM-Pro or HRM-dual belt (Garmin Ltd., Taiwan), with 1000 Hz resolution frequency, and analyzed with Kubios HRV Premium (Version 3.5.0, Kubios Oy, Kuopio, Finland). Medium automatic quality detection with a 5% acceptance threshold and an automatic beat correction method were used. Low- and high-frequency spectrums were calculated applying Lomb-Scargle periodogram with 0.02 Hz smoothing window. The HRV samples were 8-min subintervals within 10-min measurement intervals of the durability test. Only data points with effective data length at least 95% were included in the final analysis.
In the analysis, after removing all subjects with more than 50% missing data points (six from cardiac impedance measurements and one from HRV), 7.0% of HRV and 13.2% of cardiac impedance data were missing. Multiple imputations were used to fill in missing data. Drifts in imputed LVET and SV were interpolated from regression lines.
2.4 LIT and HIT training
Subjects were randomized into two training groups, applying minimization method (Hu and Hu, 2012) after the first three visits to laboratory. Training zones were determined based on lactate thresholds. The subjects were instructed to continue their daily physical activities (commuting, non-physical hobbies, etc.), but all strenuous exercise in addition to LIT or HIT was not allowed. The exact training programs are given in Supplementary Material 1.
LIT consisted of cycling under LT1-power. The weekly 5–6 training days included long (1.5–4 h), medium (1–1.5 h), and short (45–60 min) exercises. Weekly training hours progressed individually (see Progression-paragraph below) based on perceived exertion from 4.5 up to maximum 12.5 h. Subjects did their training mostly outdoors with their own bicycles with Rally RK200 dual-sensing power meters (Garmin Ltd., Taiwan). A possibility for indoors cycling with trainer or Wattbike Trainer (Wattbike Ltd., Nottingham, UK) was given. Three (out from 16) subjects did their training completely indoors, and the others did 3% of their training indoors.
HIT consisted of 2—3 weekly indoor training sessions with Wattbike Trainers, or indoor trainer with their own bicycles with Rally RK200 dual-sensing power meters. Training consisted of high-intensity work intervals 3–7 min long with recovery periods ¾ of the work interval duration. In the first training week, there were 15 min of cumulative high-intensity time in a training session, and it progressed individually (see Progression-paragraph below) up to 30 min per session. Each session included 10 min warm up and cool down. These, as well as recovery periods, were done with power <60 W, and high-intensity segments were initially 110% LT2 power (
In both groups, RPE (0–10) was reported from each training session. HR from each session was recorded with the Garmin HRM-Pro heart belt. In the LIT group, power data were recorded with power meters (Rally RK200), and in the HIT group, power data were collected from the Wattbike Trainer or power meters (Rally RK200). All data was transferred after the session to AthleteMonitoring app (AthleteMonitoring, FITSTATS Technologies, Inc., Moncton, Canada), from which training realization was actively monitored weekly by the first author. In the HIT group, if all weekly training had RPE
Daily physical activity was gathered by measuring heart rate from wrist continuously by Garmin Forerunner 945 for two to 4 weeks before the start of the intervention. This was done to estimate the baseline endurance training before training intervention.
Training zones and load. Training was monitored by distributing cycling power output to five zones (Cejuela-Anta and Esteve-Lanao, 2011): Z1 (below LT1—10 W); Z2 (LT1—10 W to LT1 + 10 W); Z3 (LT1 + 10 W to LT2—10 W); Z4 (LT2—10 W to LT2 + 10 W); Z5 (above LT2 + 10 W). For each zone, a weighting factor was linked (in an ascending order: 1, 2, 3, 4, 7.5) and training load was calculated by multiplying the factor by the time spent in the zone (Cejuela-Anta and Esteve-Lanao, 2011).
Targeted power of LIT was designed to be at Z1 and Z2, and HIT at Z4 and Z5. Heart rate was divided into three zones (HR Zones 1—3) separated by LT1 and LT2. In addition, for the LIT group, it was calculated how many times their 30 s -average power exceeded Z2 -zone. For baseline physical activity before the start of the training intervention, Z0 was defined to be a zone below halfway between resting HR and LT1, and Z1b above Z0 and below LT1. During physical activity heart rate measurement part of the data was dismissed, 12
Training progression. Both groups had 10 training weeks. Weeks 3 and 7 were load reduction weeks for enhancing recovery. Subjects’ training progression was linked to the perceived exertion. After each training week (excluding load reduction weeks), subjects were asked ‘How much the training has strained your week on a scale of 0–10?’, and the training time was increased more with lower exertion (see Supplementary Digital Content 1). For those finishing the study, adherence rate in training sessions was 98.4%. The weekly training intensity distribution is shown in Figure 2 and training realization in Table 2.
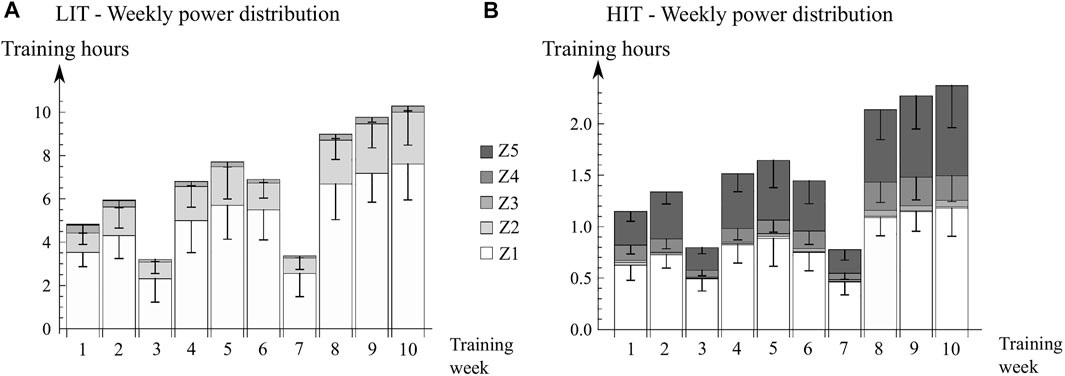
FIGURE 2. Mean weekly training power distribution for (A) LIT, SD for zones Z1, Z2, Z3; (B) HIT, SD for zones Z1, Z4, and Z5. Observe the different y-axis in figures (A) and (B). The small reduction in hours in week 6 was caused by replacing one session by VO2max-test. Mean weekly training hours in the LIT group was 4.4 (range 4.0—4.8) times than that of the HIT group (excluding load reduction weeks). LIT Low intensity training. HIT High intensity training. Power zones Z1 (below LT1—10 W); Z2 (between LT1—10 W to LT1 + 10 W); Z3 (between LT1 + 10 W to LT2—10 W); Z4 (between LT2—10 W to LT2 + 10 W); Z5 (above LT2 + 10 W). LT1 First lactate threshold. LT2 Second lactate threshold.
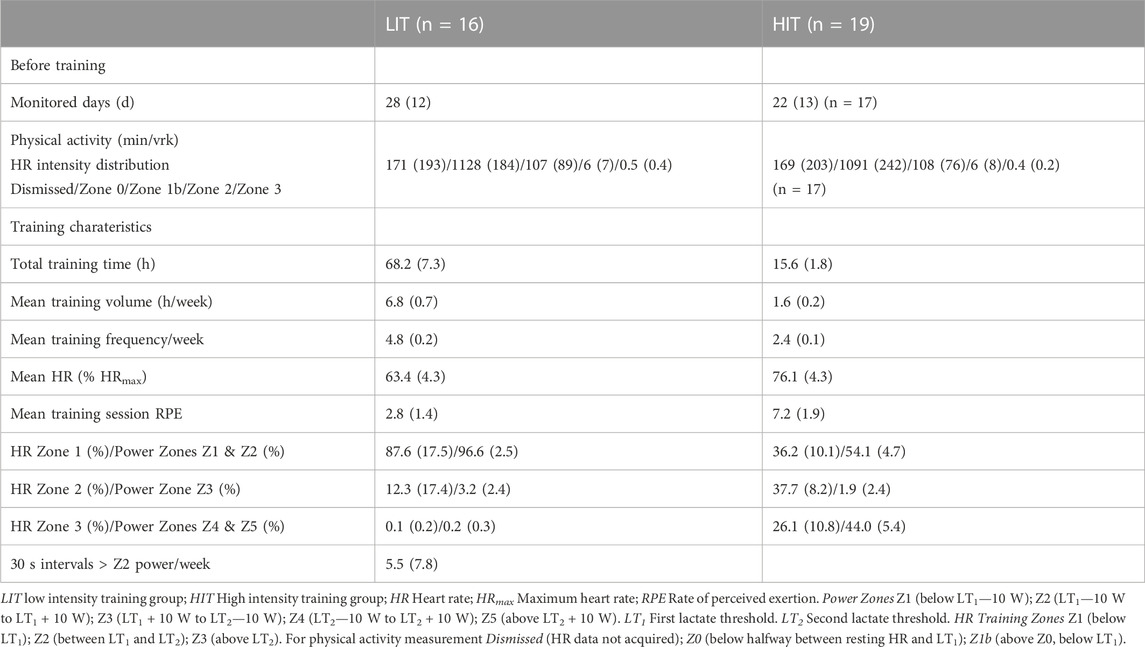
TABLE 2. Training realization, mean (SD), in LIT and HIT groups during 10- week training. Before training intervention there were no differences between the groups in HR distribution (p > 0.28).
2.5 Statistical analyses
Data are presented as mean (standard deviation). Statistical tests were calculated by SPSS 26.0 (SPSS Inc, Chicago, IL, United States) and Mathematica 13.0 (Wolfram Research, United States). Missing data on heart rate variability and cardiac impedance were handled by multiple imputation. To be conservative, 50 imputations were used (Licht, 2010). Pooled p-values were calculated applying z-method previously suggested (Licht, 2010).
Before performing the final analysis, it was determined if the magnitude of change in variables differed between the sexes (Kruskal-Wallis test) in 3 h test. Of all drifts and their onsets and physiological strains (88 tests), the only differences were detected in the RPE onset (p = 0.03) and lactate at 60 min time point (p = 0.04) in the HIT. As they were the only exceptions, it was decided to analyze females and males in a combined group. The Shapiro-Wilk test was used to examine normality.
If results were not normally distributed, group size was small (n < 10), or values were categorial (i.e. RPE, onset of drifts), the non-parametric Wilcoxon signed-rank-test was used for comparison between time points, and Mann-Whitney U-test for between-group comparison. When normality assumptions failed, but covariance matrices were homogenous by Box’s test, Pillai’s trace was utilized in ANOVA. If covariance assumption was not met, Kruskal-Wallis test was used. To calculate the average drifts and their onsets their weighted mean was calculated. In physiological strain, HRV responses were first averaged as a single variable. A harmonic mean p-value technique was applied to estimate the combined change in durability from all examined factors (drifts, their onset, and physiological strain) given all parameters weights proportional to their sample size. Post hoc correlations were done using Spearman correlations with RPE and Pearson correlations otherwise.
The effect size (ES) of differences for the main variables were calculated with a corrected effect size Hedge’s g, for which a non-central 95% confidence interval (CI) was calculated (Goulet-Pelletier and Cousineau, 2018). Effect size for between-group differences was calculated by subtracting within-group effect sizes from each other (Morris and DeShon, 2002). After non-parametric tests, effect size was calculated by a formula
where
In figures, the statistical values of the durability test were pooled: p-values were changed to z-scores which were averaged, similar to the methodology of multiple imputation (Licht, 2010). Effect sizes were pooled following the suggestion by (Rosenthal and DiMatteo, 2001): Cohen di ES
3 Results
All factors combined. When magnitude and onset of drifts and physiological strain were all averaged together, durability was improved in both LIT and HIT groups (LIT: combined harmonic p = 0.03, averaged Hedge’s g ES = 0.49, CLES = 68%; HIT: p = 0.01, ES = 0.62, CLES = 70%) with no difference between groups (time x group combined harmonic p = 0.42).
Magnitudes of drifts. There was no time x group difference in magnitude of drifts (combined harmonic p = 0.28). The averaged drifts from pre-to post-tests decreased (Table 3) in HIT (8.8
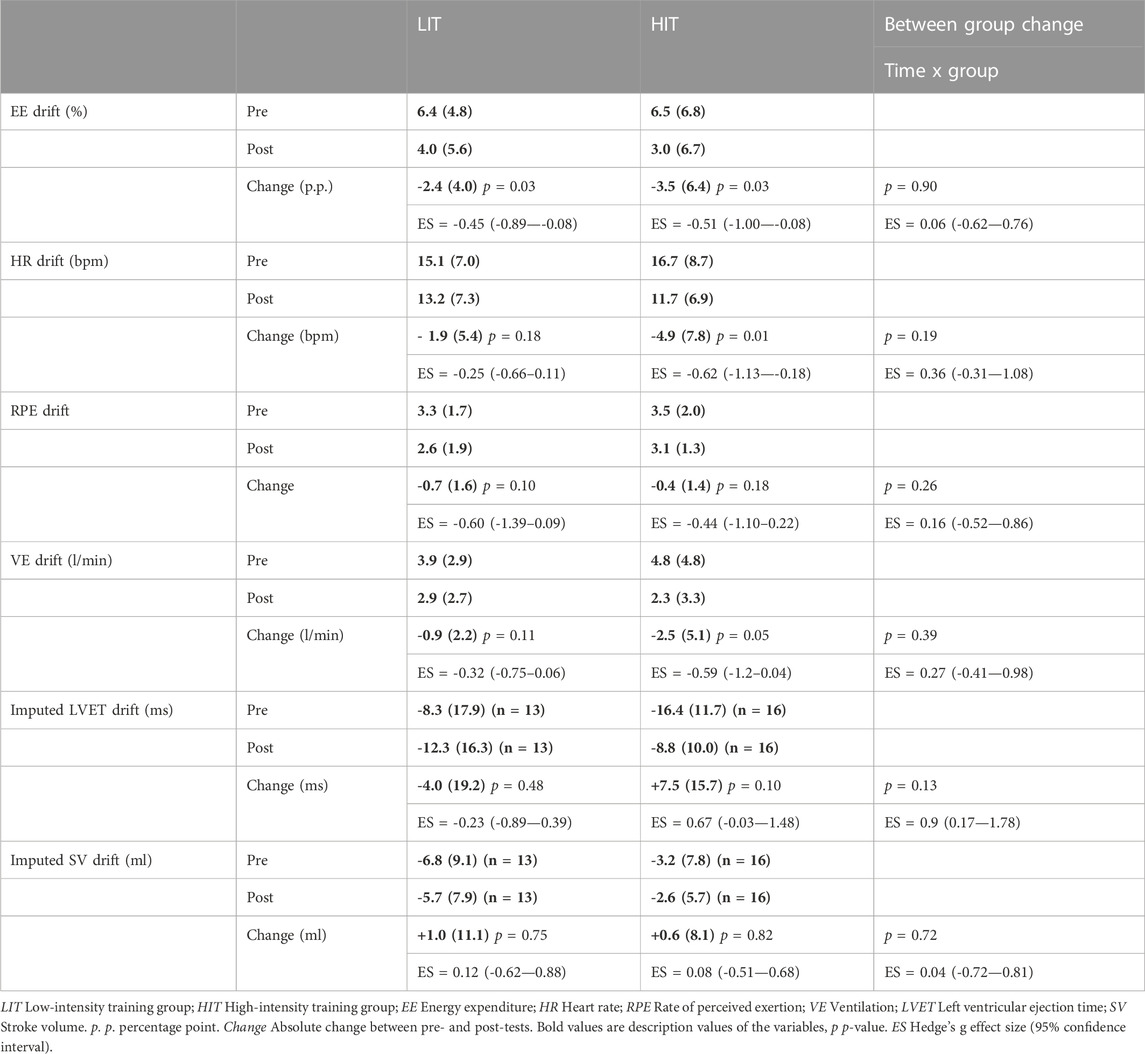
TABLE 3. Mean (SD) magnitude of the drifts of physiological variables during 3 h durability test. Drifts of the imputed LVET and SV are extrapolated from regression lines.
Onset of drifts. There were no time x group difference onset of drifts (combined harmonic p = 0.44). The average onset of drifts from pre-to post-tests prolonged (Table 4) in HIT (108
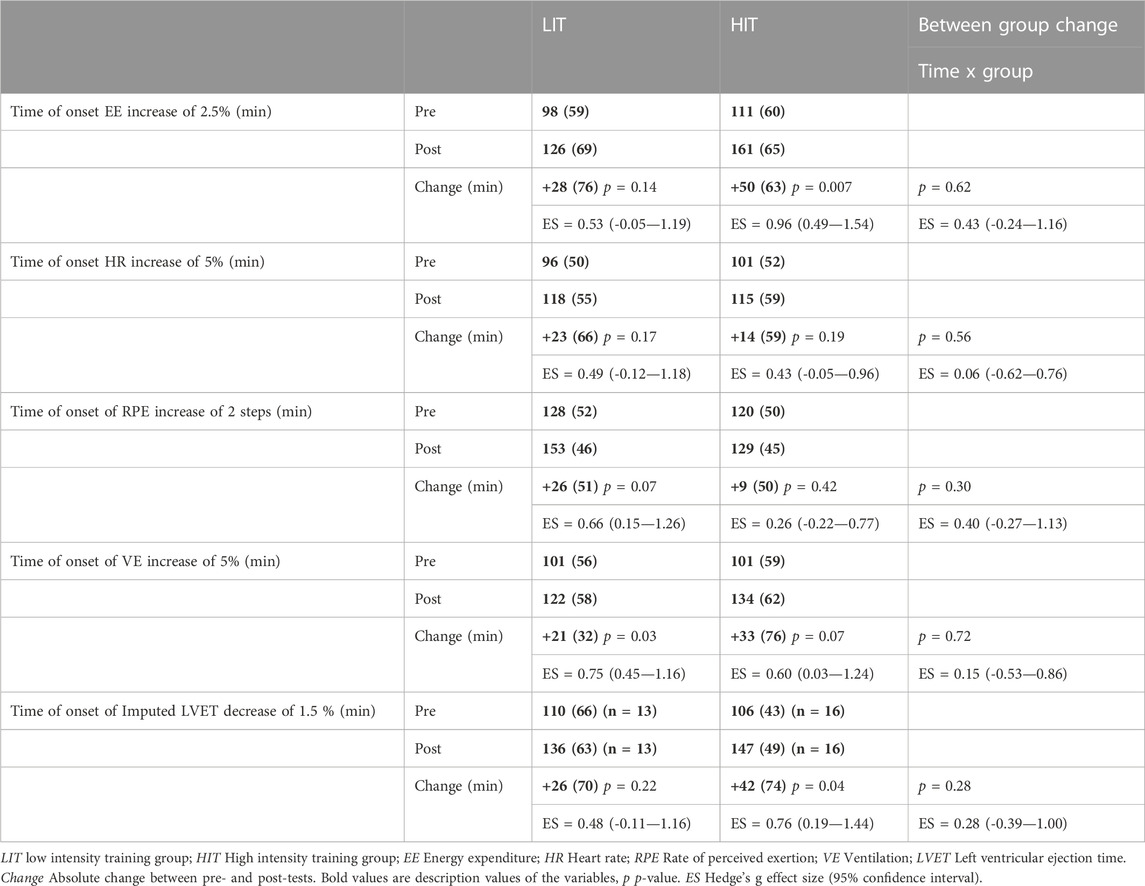
TABLE 4. Mean (SD) time of onset of the physiological drifts: EE (2.5% increase), HR (5% increase), RPE (2 steps), VE (5% increase), LVET (1.5% decrease).
Physiological strain. The averaged change in physiological strain (Figure 3; Figure 4) was large both in LIT (p = 0.01, ES = 0.60, CLES = 73%) and HIT (p = 0.005, ES = 0.78, CLES = 76%) The responses were similar on both groups (harmonic time x group p = 0.73).
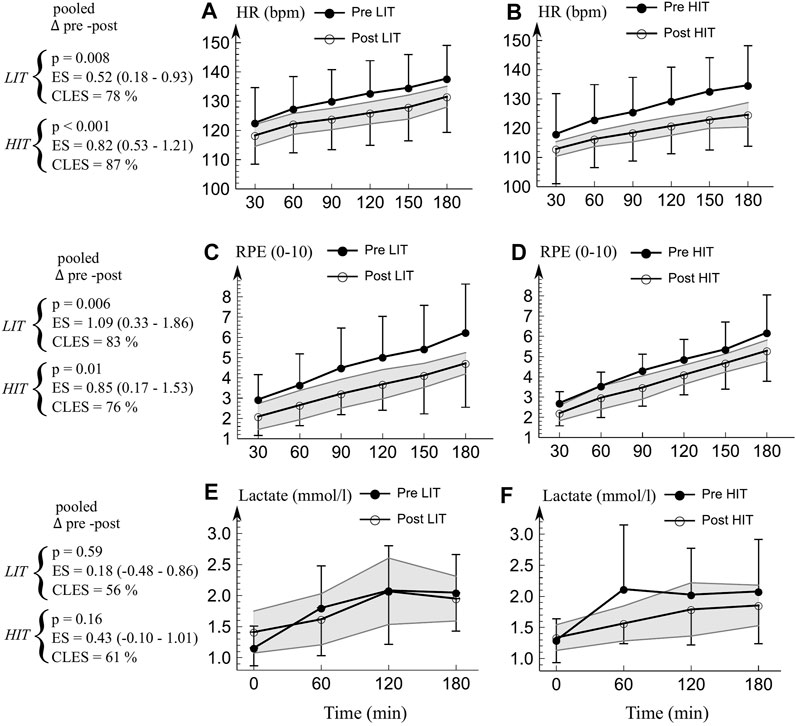
FIGURE 3. (A–B) Mean (SD) heart rate, (C–D) RPE, and (E–F) blood lactate concentration during durability test. Pooled p-values, ES (95% CI), and CLES were averaged from the time points of 30–180 min (60–180 min with lactate) to represent the average change. The gray area represents 95% confidence interval for the change in variable (i.e. if pre-value is outside gray area, the change in that time point is significant at p = 0.05 level). There were no time x group differences on average between the groups in HR, RPE, or lactate (p > 0.25). LIT Low intensity training group, HIT High intensity training group. HR Heart rate. RPE Rate of perceived exertion. P p-value. ES Effect size (Hedge’s g). CLES Common language effect size.
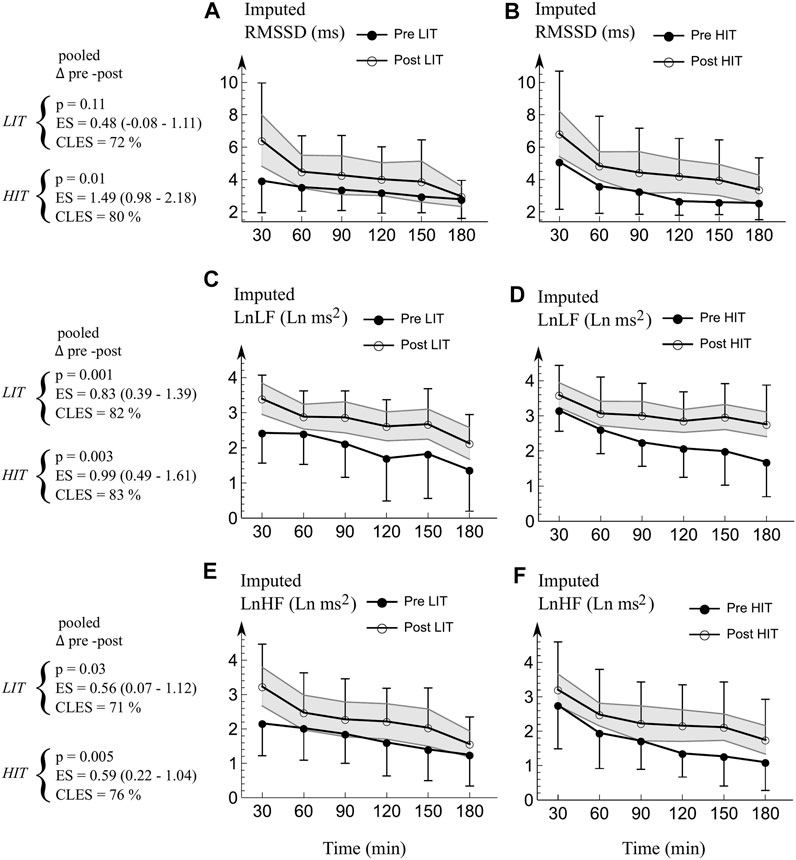
FIGURE 4. Mean (SD) imputed HRV responses to 3 h durability test. (A,B) RMSSD. (C,D) LnLF. (E,F) LnHF. Pooled p-values, ES (95% CI), and CLES were averaged from the time points of 30—180 min to represent the average change. The gray area represents 95% confidence interval for the change in variable (i.e. if pre-value is outside gray area, the change in that time point is significant at p = 0.05 level). ANOVA revealed no time x group differences (p > 0.10). LIT Low Intensity training group, HIT High Intensity training group. HR Heart rate. RMSSD Root mean square of successive RR interval differences. LnLF Natural logarithm of absolute power of the low-frequency band (0.04–0.15 Hz). LnHF Natural logarithm of absolute power of the high-frequency band (0.15–1.1 Hz). p p-value. ES Effect size (Hedge’s g). CLES Common language effect size.
VO2max and sprinting fatigue ratio. There was a large time x group difference [p < 0.001, ES = 1.51 (0.80—2.43), CLES = 83%] in VO2max(l/min), which improved only in HIT (Table 5) [LIT: n = 14,
There was no time x group difference [p = 0.91, ES = 0.33 (-0.44—1.16), CLES = 51%] in the sprinting fatigue ratio (Table 5). It increased in the HIT group, but no change was observed in the LIT group [LIT: n = 11,
Post-hoc correlations. When both groups were studied together in terms of training induced changes between the drifts, VO2max, and power at LT1, the only noteworthy correlations were observed between
Description of durability test. RER did not change during 3 h durability test from 30 min to 180 min: from 0.93 (0.03) to 0.92 (0.02) during pre-test and from 0.92 (0.04) to 0.92 (0.02) during post-test (n = 35, both groups combined) with no differences between the groups. Body mass during the 3 h durability test was unchanged: +0.2 (0.6) kg in pre-test, and -0.1 (0.4) kg in post-test (n = 28, both groups combined) with no differences between the groups. Also, the water intake was similar in pre- and post-tests (p = 0.07, g = 0.59, groups combined). EE, VO2, Imputed LVET and SV during durability test are shown in Supplementary Digital Content 2.
4 Discussion
Our main finding was that there was no difference between improvement in durability after 10 weeks of both LIT and HIT based on averaged value consisted of reduced magnitude of the physiological drifts (-1.2 and -2.4 p. p. on average), postponed time of onset (25 and 29 min on average) and attenuated physiological strain. No adverse effects, i.e. increased drifts, shortened onset of drifts or amplified physiological strain, were detected at the group level in any individual variable.
4.1 Results compared to literature
Our EE drift (6%) was consistent with earlier studies. Recalculated from average values from tables and figures, VO2 drift between 4% and 7% has been reported during 90–180 min low-to moderate-intensity endurance exercise (Coggan et al., 1993; Carter et al., 2001; Rønnestad et al., 2011), but also values as high as 16% were reported (Hurley et al., 1986). In these studies, VO2 drift was reduced by endurance alone or concurrent endurance and strength training by 2–4 p. p., which is equal to our 3 p. p. decrease in EE drift. In these same studies, initial HR drift of 10%—20% was reduced by 3 – 9 p. p. in endurance only or concurrent training. In our study, HR drift was initially 14%. HR drift was reduced by 4 p. p. in the HIT group, while it was not changed after LIT. It should be noted, however, that training does not necessarily lead to enhanced durability. Concurrent training did not affect durability (physiological drifts) during a 1 h load carriage task (Wills et al., 2019), and endurance training in well-trained athletes did not lead to enhanced durability (Hausswirth et al., 2010; Rønnestad et al., 2011), nor did concurrent training (Hausswirth et al., 2010).
4.2 Durability and relative reduction in intensity
Durability can usually be increased by endurance training in recreationally active subjects (Hurley et al., 1986; Coggan et al., 1993; Phillips et al., 1996; Carter et al., 2001). However, as training simultaneously increases VO2max, the relative intensity during the prolonged tests in these studies were lower after the intervention. It is possible that after the intervention the first lactate threshold may have risen above tested intensity, thereby possibly moving the tested intensity to a different exercise zone. This provokes the question of whether durability itself is enhanced or the drifts are more restrained because of decreased relative intensity.
In our study, we assured that the intensity was in both test occasions initially at low intensity zone so that the origin of fatigue was similar at both times. Similar lactate concentrations in pre- and post-tests confirms the similar exercising zones. VO2max increased only in the HIT group and the relative intensity was -6 p. p. lower (49% vs. 43% VO2max) in post-test compared to pre-test, while it remained unchanged in the LIT group. Hence, for the LIT group, durability was affected without increase in VO2max. This supports the idea that factors affecting VO2max and durability may be different, or that durability can be affected both by improving VO2max as well as by improving submaximal metabolism.
In a previous study (Carter et al., 2001), subjects cycled both the same relative and absolute intensities after a high-volume training intervention. Based on the study (Carter et al., 2001), one can deduce (after recalculations) that physiological drifts and strain are diminished by reduced relative intensity alone, and that increased durability (2.4 p. p. reduction in VO2 drift) might be possible when the same relative power is repeated. Further, concurrent endurance and heavy strength training has also shown that enhanced durability (reduced HR and VO2 drifts from recalculations) is possible with only a small simultaneous increase (+3%) in VO2max (Rønnestad et al., 2011). Finally, power profile in a fatigued state changes in competitive cyclists during the competition season (Spragg et al., 2022), with only minor changes in maximum exercise performance.
4.3 Decreased physiological strain
Formerly, the RPE response has been reduced after training during prolonged exercise (Rønnestad et al., 2011; Wills et al., 2019), which is consistent with the present findings. We observed that there was a decrease in submaximal exercise HR, which usually is reported to stem from increased plasma and total blood volume (Green et al., 1990). Structural or functional adaptations within the myocardium take longer and have not been found after a few weeks of endurance training (Bonne et al., 2014). Acute plasma volume expansion decreases HR considerably, up to 10–15 bpm (Green et al., 1990). However, it does not alter, and hence explain changes in, HR drift (Green et al., 1990; Grant et al., 1997).
In creased HRV indices may also be a consequence of plasma expansion (Frank et al., 2001). Further, catecholamine responses to regular exercise in a stable trained state are reduced (Phillips et al., 1996; Carter et al., 2001). Hence, reduced sympathetic effect on the heart may lead to increased HRV, which indicates positive changes in balance of the autonomic nervous system.
4.4 Underlying mechanistic reasons to drifts
The six physiological drifts were investigated to gain a wide physiological drift profile. EE represents the overall physiological strain of exercise, and RPE represents perceived strain. HR is the most utilized marker of relative intensity during prolonged exercise, and with SV, they completely represent the circulating blood flow. LVET illustrates the sympathetic activity of the heart. VE, in addition to being strongly linked to CO2 output, also portrays complex exercise hyperpnea.
EE drift is related to depletion of glycogen stores in slow-twitch muscle fibers of agonist muscles, after which the body needs to recruit less efficient co-agonists and fast-twitch fibers to maintain the constant power output (Krustrup et al., 2004). Also elevated O2 cost of fatigued muscle (Hopker et al., 2017) is nominated to cause EE drift. Thus, it is presumable that at least one of these processes was slowed during our intervention. Glycogen content of a muscle is increased by endurance training (Murray and Rosenbloom, 2018), as well as fat oxidation capacity and efficiency (Phillips et al., 1996). Hence, after the training, the need to activate less efficient and highly fatigable fast-twitch fibers is postponed, leading to reduced EE drift. Additionally, endurance training alters type II fibers by augmenting fatigue-resistance to act more like the type I fibers (Seene et al., 2007), so their mechanical efficiency might not drop so considerably during prolonged exercise. As endurance training also enhances mitochondrial mass and function (Bishop et al., 2014), energy production can be spread through larger mass, inflicting smaller oxidative damage during prolonged cycling so that O2 -cost is not elevated as greatly.
Due to the exercise intervention of the present study, HR drift was reduced in the HIT group. An increased sympathetic activation, core temperature, and fatigue of the neuromuscular system have been reported to be the reasons for HR drift (Coyle and González-Alonso, 2001). Core temperature was not measured in our study, and neuromuscular fatigue was negligible, as measured with 15 s maximum sprint. While HRV is a marker for autonomic nervous system balance, LVET and pre-ejection period can indicate chronotropic sympathetic activity (Thayer and Uijtdehaage, 2001). As the LVET drift was reduced in the HIT group in trained state, and its onset was postponed, it is possible that dampened sympathetic activity during durability tests may play a role in reducing HR drift. This observation is supported by the positive correlation between change in HR and LVET drifts. Increased HRV responses may further support our interpretation.
Durability can be seen as a form of fatigue resistance. However, the term ‘fatigue resistance’ has evolved greatly to different directions during its history. Its interpretations include, for example, an ability to resist fall in potentiated twitch force (Ducrocq et al., 2021) or in force during electrical stimulation (Morris et al., 2008), time to exhaustion (Lindsay et al., 1996), and a fraction of VO2max used during a time trial (Hawley et al., 1997). Fatigue resistance is usually connected to neuromuscular fatigue during maximal and short performances. Durability, in turn, is a specifically defined way to study fatigue resistance associated to prolonged and submaximal performances using physiological variables.
4.5 Training induced changes
The groups had similar baseline physical activity habits consisting in mostly low intensity activity. Training intervention induced changes in measured responses in our study in both groups pointed toward improved durability, indicating improved durability with the exception of imputed LVET in the LIT group. Based on effect sizes, LIT and HIT did not seemed to increase drifts or their onset in large amount, despite that the subjects had not undertaken systematic endurance training and a majority of them had not engaged in 3 h exercise before. This is quite a contrast to other endurance capacity markers such as VO2max (Wen et al., 2019) and time trial ability (Rosenblat et al., 2021), which are usually quite distinctly enhanced in untrained subjects. Indeed, VO2max was also distinctly elevated in our HIT group with very large effect size.
Keeping power below LT1 in the LIT group resulted in a mean HR of 63% HRmax. The sufficient exercise intensity to improve endurance capacity is at least 3 metabolic equivalents (Bull et al., 2020) which, in the case of the LIT group, corresponds to 58 (9)% HRmax in pre-test. As training did not induce changes in VO2max in the LIT group, it is possible that the intensity was not high enough for these subjects (Swain and Franklin, 2002; Laursen, 2010), which leads to a conclusion that intensity to improve VO2max could be higher than the intensity required for durability improvements.
It was surprising that high-volume LIT, which mimics very closely the durability test and challenges the same origins of fatigue (Black et al., 2017; Burnley and Jones, 2018), did not induce larger increases in durability. Earlier, 65% VO2max-intensity training produced quite clear changes in the VO2 drifts (Phillips et al., 1996; Carter et al., 2001), suggesting that durability may also be intensity dependent and one cannot simply compensate lower intensity with longer exercise bouts. Other reason might be that although the origins of fatigue are different in low and high intensity zones, high intensity zone could nevertheless stimulate similarly central neuromuscular function as can be deduced by similar M-wave decrease after low and high intensity exercise (Black et al., 2017) and they both deplete glycogen storages (Gollnick et al., 1974). Hence, they both could challenge similar abilities to resist fatigue at durability test. In our durability test constant energy intake was present, which prevented the influence of the possible enhanced fat oxidation capacity.
4.6 Limitations of our study
It seems that test-retest reliability measures have not been undertaken, although multiple studies have been conducted on physiological drifts in prolonged submaximal exercise (Ekelund, 1967; Phillips et al., 1996; Carter et al., 2001; Coyle and González-Alonso, 2001; Rønnestad et al., 2011; Mullins et al., 2015; Wills et al., 2019; Smyth et al., 2022). Hence, it is not known how sensitive physiological drifts are to changes in environment and preparation.
Nutrition was not controlled during the training, although the relevance of carbohydrate ingestion was stressed to participants during the training. Ingesting carbohydrates during the durability test reduced the importance of nutrition status, but it does not eliminate it completely (Tsintzas et al., 2001).
Change in plasma volume during 3 h durability test was not monitored. However, the body mass was not reduced, and the plasma volume reduction in prolonged low intensity exercise at lower than 50% VO2max intensity is minimal (Grant et al., 1997). Therefore, it can be deduced that acute plasma volume change during 3 h test had minimal effect to the outcomes.
There are sex differences in physiological responses to exercise (e.g. fat oxidation) (Purdom et al., 2018). As such, it might be that there are sex-specific optimal training protocols to improve durability. Our sample size was not planned to assess possible sex differences in the studied variables. Menstrual cycle was not controlled, but ingesting carbohydrates during the durability test minimized its effect (Campbell et al., 2001).
Our sample size (n = 35) was quite favorable as compared to previous intervention studies (n = 8–20) (Coggan et al., 1993; Phillips et al., 1996; Carter et al., 2001; Frank et al., 2001; Hausswirth et al., 2010; Rønnestad et al., 2011; Bonne et al., 2014; Wills et al., 2019). Nevertheless, a higher number of subjects may have enabled us to observe differences between the study groups and/or training protocols.
4.7 Future directions
The existing research on durability has mostly been reported by observational studies using existing training databases (Van Erp et al., 2021; Smyth et al., 2022; Spragg et al., 2022). Although that allows one to reach a high number of subjects, carefully conducted laboratory studies are needed to deepen the understanding of durability, its links to other possible physiological sources (e.g. endocrinal, body temperature, and muscle activity patterns drifts), and adaptability to training.
5 Conclusion
Our study was one of the first interventions on focusing durability, and it showed that 10-week high- and low-intensity training programs increased durability among untrained subjects, and no differences between the training groups were noticed. Increased VO2max facilitated 3 h cycling only in the high-intensity training group, although the change in VO2max did not correlate with the change in durability. Hence, it seems that durability and VO2max are not strongly related with each other and are driven by different physiological mechanisms. Despite durability enhanced among untrained people, a 10-week intervention did not alter drifts and their onsets in a large amount, even though it attenuated physiological strain.
Data availability statement
The raw data of this article is not yet available due to the agreement with the collaborative company and further papers will also be written from the same data. However, the corresponding author is willing to give further information in this regard.
Ethics statement
The studies involving human participants were reviewed and approved by Ethical Committee of the Central Finland Healthcare District. The patients/participants provided their written informed consent to participate in this study.
Author contributions
PM, OH, AN, and HK designed the study. PM, E-PA, and LP evaluated the practical implementation of the design and carried out the data collection. PM did the statistical analyses and initiated the writing. All authors revised the manuscript critically and approved the final version.
Funding
Heart rate monitors, power meters, and salary for two research assistants were received from Firstbeat Analytics. Firstbeat Analytics received data from the research measurements, and it does not benefit from the results of the present study.
Acknowledgments
The authors would like to thank Susanna Luoma and Tanja Toivanen for their assistance during the data collection. Insightful conversations with colleagues are always beneficial; Helpful comments from Olli-Pekka Nuuttila, Mika Simola, Mika Tarvainen, Jaakko Matomäki, Johanna Ihalainen, Paula Poikonen and Laura Karavirta among others are acknowledged. Meghan Tanel is acknowledged for language editing.
Conflict of interest
The authors declare that the research was conducted in the absence of any commercial or financial relationships that could be construed as a potential conflict of interest.
Publisher’s note
All claims expressed in this article are solely those of the authors and do not necessarily represent those of their affiliated organizations, or those of the publisher, the editors and the reviewers. Any product that may be evaluated in this article, or claim that may be made by its manufacturer, is not guaranteed or endorsed by the publisher.
Supplementary Material
The Supplementary Material for this article can be found online at: https://www.frontiersin.org/articles/10.3389/fphys.2023.1128111/full#supplementary-material
References
Billat, V. L., Palacin, F., Correa, M., and Pycke, J. R. (2020). Pacing strategy affects the sub-elite marathoner’s cardiac drift and performance. Front. Psychol. 10, 3026. doi:10.3389/fpsyg.2019.03026
Bishop, D. J., Granata, C., and Eynon, N. (2014). Can we optimise the exercise training prescription to maximise improvements in mitochondria function and content? Biochim. Biophys. Acta 1840, 1266–1275. doi:10.1016/j.bbagen.2013.10.012
Black, M. I., Jones, A. M., Blackwell, J. R., Bailey, S. J., Wylie, L. J., J McDonagh, S. T., et al. (2017). Muscle metabolic and neuromuscular determinants of fatigue during cycling in different exercise intensity domains. J. Appl. Physiol. 122, 446–459. doi:10.1152/japplphysiol.00942.2016
Bonne, T. C., Doucende, G., Fluck, D., Jacobs, R. A., Nordsborg, N. B., Robach, P., et al. (2014). Phlebotomy eliminates the maximal cardiac output response to six weeks of exercise training. AJP Regul. Integr. Comp. Physiology 306, R752–R760. doi:10.1152/ajpregu.00028.2014
Bull, F. C., Al-Ansari, S. S., Biddle, S., Borodulin, K., Buman, M. P., Cardon, G., et al. (2020). World Health Organization 2020 guidelines on physical activity and sedentary behaviour. Br. J. Sports Med. 54, 1451–1462. doi:10.1136/bjsports-2020-102955
Burnley, M., and Jones, A. M. (2018). Power–duration relationship: Physiology, fatigue, and the limits of human performance. Eur. J. Sport Sci. 18, 1–12. doi:10.1080/17461391.2016.1249524
Caldwell, A., and Vigotsky, A. D. (2020). A case against default effect sizes in sport and exercise science. PeerJ 8, e10314–e10319. doi:10.7717/peerj.10314
Campbell, S. E., Angus, D. J., and Febbraio, M. A. (2001). Glucose kinetics and exercise performance during phases of the menstrual cycle: Effect of glucose ingestion. Am. J. Physiol. Endocrinol. Metab. 281, 817–825. doi:10.1152/ajpendo.2001.281.4.e817
Carter, S. L., Rennie, C., and Tarnopolsky, M. A. (2001). Substrate utilization during endurance exercise in men and women after endurance training. Am. J. Physiol. Endocrinol. Metab. 280, E898–E907. doi:10.1152/ajpendo.2001.280.6.e898
Cejuela-Anta, R., and Esteve-Lanao, J. (2011). Training load quantification in triathlon. J. Hum. Sport Exerc. 6, 218–232. doi:10.4100/jhse.2011.62.03
Coggan, A. R., Spina, R. J., Kohrt, W. M., and Holloszy, J. O. (1993). Effect of prolonged exercise on muscle citrate concentration before and after endurance training in men. Am. J. Physiol. Endocrinol. Metab. 264, E215–E220. doi:10.1152/ajpendo.1993.264.2.e215
Coyle, E. F., and González-Alonso, J. (2001). Cardiovascular drift during prolonged exercise: New perspectives. Exerc Sport Sci. Rev. 29, 88–92. doi:10.1097/00003677-200104000-00009
Ducrocq, G. P., Hureau, T. J., Bøgseth, T., Meste, O., and Blain, G. M. (2021). Recovery from fatigue after cycling time trials in elite endurance athletes. Med. Sci. Sports Exerc 53, 904–917. doi:10.1249/MSS.0000000000002557
Ekelund, L. -G. (1967). Circulatory and respiratory adaptation during prolonged exercise of moderate intensity in the sitting position. Acta Physiol. Scand. 69, 327–340. doi:10.1111/j.1748-1716.1967.tb03529.x
Ettema, G., and Loras, H. W. (2009). Efficiency in cycling: A review. Eur. J. Appl. Physiol. 106, 1–14. doi:10.1007/s00421-009-1008-7
Faude, O., Kindermann, W., and Meyer, T. (2009). Lactate threshold concepts: How valid are they? Sports Med. 39, 469–490. doi:10.2165/00007256-200939060-00003
Frank, A., Belokopytov, M., Moran, D., Shapiro, Y., and Epstein, Y. (2001). Changes in heart rate variability following acclimation to heat. J. Basic Clin. Physiol. Pharmacol. 12, 19–32. doi:10.1515/JBCPP.2001.12.1.19
Gollnick, P. D., Piehl, K., and Saltin, B. (1974). Selective glycogen depletion pattern in human muscle fibres after exercise of varying intensity and at varying pedalling rates. J. Physiol. 241, 45–57. doi:10.1113/jphysiol.1974.sp010639
Goulet-Pelletier, J.-C., and Cousineau, D. (2018). A review of effect sizes and their confidence intervals, Part I: The Cohen’s d family. Quant. Method Psychol. 14, 242–265. doi:10.20982/tqmp.14.4.p242
Grant, S. M., Green, H. J., Phillips, S. M., and Sutton, J. R. (1997). Effects of acute expansion of plasma volume on cardiovascular and thermal function during prolonged exercise. Eur. J. Appl. Physiol. Occup. Physiol. 76, 356–362. doi:10.1007/s004210050261
Green, H. J., Jones, L. L., and Painter, D. C. (1990). Effects of short-term training on cardiac function during prolonged exercise. Med. Sci. Sports Exerc 22, 488–493. doi:10.1249/00005768-199008000-00012
Hausswirth, C., Argentin, S., Bieuzen, F., Le Meur, Y., Couturier, A., and Brisswalter, J. (2010). Endurance and strength training effects on physiological and muscular parameters during prolonged cycling. J. Electromyogr. Kinesiol. 20, 330–339. doi:10.1016/j.jelekin.2009.04.008
Hawley, J. A., Myburgh, K. H., Noakes, T. D., and Dennis, S. C. (1997). Training techniques to improve fatigue resistance and enhance endurance performance. J. Sports Sci. 15, 325–333. doi:10.1080/026404197367335
Hopker, J. G., O’Grady, C., and Pageaux, B. (2017). Prolonged constant load cycling exercise is associated with reduced gross efficiency and increased muscle oxygen uptake. Scand. J. Med. Sci. Sports 27, 408–417. doi:10.1111/sms.12673
Hu, Y., and Hu, F. (2012). Balancing treatment allocation over continuous covariates: A new imbalance measure for minimization. J. Probab. Stat. 2012, 1–13. Article ID 842369. doi:10.1155/2012/842369
Hurley, B. F., Nemeth, P. M., Martin, W. H., Hagberg, J. M., Dalsky, G. P., and Holloszy, J. O. (1986). Muscle triglyceride utilization during exercise: Effect of training. J. Appl. Physiol. 60, 562–567. doi:10.1152/jappl.1986.60.2.562
Jeukendrup, A. (2014). A step towards personalized sports nutrition: Carbohydrate intake during exercise. Sports Med. 44, S25–S33. doi:10.1007/s40279-014-0148-z
K. L. Keskinen, K. Häkkinen, and M. Kallinen (Editors) (2004). Kuntotestauksen Käsikirja (trans. Handbook of fitness testing) (Finland: Liikuntatieteellinen seura).
Krustrup, P., Söderlund, K., Mohr, M., and Bangsbo, J. (2004). Slow-twitch fiber glycogen depletion elevates moderate-exercise fast-twitch fiber activity and O2 uptake. Med. Sci. Sports Exerc 36, 973–982. doi:10.1249/01.MSS.0000128246.20242.8B
Laursen, P. B. (2010). Training for intense exercise performance: High-intensity or high-volume training? Scand. J. Med. Sci. Sports 20, 1–10. doi:10.1111/j.1600-0838.2010.01184.x
Licht, C. (2010). New methods for generating significance levels from multiply-imputed data [dissertation]. Germany: Otto-Friedrich-Universität Bamberg.
Lindsay, F. H., Hawley, J. A., Myburgh, K. H., Schomer, H. H., Noakes, T. D., and Dennis, S. C. (1996). Improved athletic performance in highly trained cyclists after interval training. Med. Sci. Sports Exerc 28, 1427–1434. doi:10.1097/00005768-199611000-00013
Martin, B. J., Morgan, E. J., Zwillich, C. W., and Weil, J. V. (1981). Control of breathing during prolonged exercise. J. Appl. Physiol. Respir. Environ. Exerc Physiol. 50, 27–31. doi:10.1152/jappl.1981.50.1.27
Maunder, E., Seiler, S., Mildenhall, M. J., Kilding, A. E., and Plews, D. J. (2021). The importance of ‘durability’ in the physiological profiling of endurance athletes. Sports Med. 51, 1619–1628. doi:10.1007/s40279-021-01459-0
McGrath, R. E., and Meyer, G. J. (2006). When effect sizes disagree: The case of r and d. Psychol. Methods 11, 386–401. doi:10.1037/1082-989X.11.4.386
Moreno, I. L., Pastre, C. M., Ferreira, C., de Abreu, L. C., Valenti, V. E., and Vanderlei, L. C. M. (2013). Effects of an isotonic beverage on autonomic regulation during and after exercise. J. Int. Soc. Sports Nutr. 10, 2. doi:10.1186/1550-2783-10-2
Morris, M. G., Dawes, H., Howells, K., Scott, O. M., and Cramp, M. (2008). Relationships between muscle fatigue characteristics and markers of endurance performance. J. Sports Sci. Med. 7, 431–436.
Morris, S. B., and DeShon, R. P. (2002). Combining effect size estimates in meta-analysis with repeated measures and independent-groups designs. Psychol. Methods 7, 105–125. doi:10.1037/1082-989X.7.1.105
Mullins, A. K., Annett, L. E., Drain, J. R., Kemp, J. G., Clark, R. A., and Whyte, D. G. (2015). Lower limb kinematics and physiological responses to prolonged load carriage in untrained individuals. Ergonomics 58, 770–780. doi:10.1080/00140139.2014.984775
Murray, B., and Rosenbloom, C. (2018). Fundamentals of glycogen metabolism for coaches and athletes. Nutr. Rev. 76, 243–259. doi:10.1093/NUTRIT/NUY001
Passfield, L., and Doust, J. H. (2000). Changes in cycling efficiency and performance after endurance exercise. Med. Sci. Sports Exerc 32, 1935–1941. doi:10.1097/00005768-200011000-00018
Phillips, S. M., Green, H. J., Tarnopolsky, M. A., Heigenhauser, G. J. F., Hill, R. E., and Grant, S. M. (1996). Effects of training duration on substrate turnover and oxidation during exercise. J. Appl. Physiol. 81, 2182–2191. doi:10.1152/jappl.1996.81.5.2182
Purdom, T., Kravitz, L., Dokladny, K., and Mermier, C. (2018). Understanding the factors that effect maximal fat oxidation. J. Int. Soc. Sports Nutr. 15, 3. doi:10.1186/s12970-018-0207-1
Rønnestad, B. R., Hansen, E. A., and Raastad, T. (2011). Strength training improves 5-min all-out performance following 185 min of cycling. Scand. J. Med. Sci. Sports 21, 250–259. doi:10.1111/j.1600-0838.2009.01035.x
Rosenblat, M. A., Lin, E., da Costa, B. R., and Thomas, S. G. (2021). Programming interval training to optimize time-trial performance: A systematic review and meta-analysis. Sports Med. 51, 1687–1714. doi:10.1007/s40279-021-01457-2
Rosenthal, R., and DiMatteo, M. R. (2001). Meta-analysis: Recent developments in quantitative methods for literature reviews. Annu. Rev. Psychol. 52, 59–82. doi:10.1146/annurev.psych.52.1.59
Rudzki, S. J. (1989). Weight-load marching as a method of conditioning Australian Army recruits. Mil. Med. 154, 201–205. doi:10.1093/milmed/154.4.201
Seene, T., Alev, K., Kaasik, P., and Pehme, A. (2007). Changes in fast-twitch muscle oxidative capacity and myosin isoforms modulation during endurance training. J. Sports Med. Phys. Fit. 47, 124–132.
Smyth, B., Maunder, E., Meyler, S., Hunter, B., and Muniz, D. (2022). Decoupling of internal and external workload during a marathon: An analysis of durability in 82303 recreational runners. Sports Med. 52, 2283–2295. doi:10.1007/s40279-022-01680-5
Spragg, J., Leo, P., and Swart, J. (2022). The relationship between training characteristics and durability in professional cyclists across a competitive season. Eur. J. Sport Sci. 22, 1–10. doi:10.1080/17461391.2022.2049886
Swain, D. P., and Franklin, B. A. (2002). VO2 reserve and the minimal intensity for improving cardiorespiratory fitness. Med. Sci. Sports Exerc 34, 152–157. doi:10.1097/00005768-200201000-00023
Thayer, J. F., and Uijtdehaage, S. H. J. (2001). Derivation of chronotropic indices of autonomic nervous system activity using impedance cardiography. Biomed. Sci. Instrum. 37, 331–336.
Tsintzas, K., Williams, C., Constantin-Teodosiu, D., Hultman, E., Boobis, L., Clarys, P., et al. (2001). Phosphocreatine degradation in type I and type II muscle fibres during submaximal exercise in man: Effect of carbohydrate ingestion. J. Physiology 537, 305–311. doi:10.1111/j.1469-7793.2001.0305k.x
Van Erp, T., Sanders, D., and Lamberts, R. P. (2021). Maintaining power output with accumulating levels of work done is a key determinant for success in professional cycling. Med. Sci. Sports Exerc 53, 1903–1910. doi:10.1249/MSS.0000000000002656
Wen, D., Utesch, T., Wu, J., Robertson, S., Liu, J., Hu, G., et al. (2019). Effects of different protocols of high intensity interval training for VO2max improvements in adults: A meta-analysis of randomised controlled trials. J. Sci. Med. Sport 22, 941–947. doi:10.1016/j.jsams.2019.01.013
Wills, J. A., Saxby, D. J., Glassbrook, D. J., and Doyle, T. L. A. (2019). Load-carriage conditioning elicits task-specific physical and psychophysical improvements in males. J. Strength Cond. Res. 33, 2338–2343. doi:10.1519/JSC.0000000000003243
Keywords: durability, low intensity training, high intensity training, perceived exertion, cardiovascular drift
Citation: Matomäki P, Heinonen OJ, Nummela A, Laukkanen J, Auvinen E-P, Pirkola L and Kyröläinen H (2023) Durability is improved by both low and high intensity endurance training. Front. Physiol. 14:1128111. doi: 10.3389/fphys.2023.1128111
Received: 20 December 2022; Accepted: 31 January 2023;
Published: 16 February 2023.
Edited by:
Danilo Iannetta, The University of Utah, United StatesReviewed by:
Hugo A. Kerhervé, University of Rennes 2—Upper Brittany, FranceRafael de Almeida Azevedo, University of São Paulo, Brazil
Copyright © 2023 Matomäki, Heinonen, Nummela, Laukkanen, Auvinen, Pirkola and Kyröläinen. This is an open-access article distributed under the terms of the Creative Commons Attribution License (CC BY). The use, distribution or reproduction in other forums is permitted, provided the original author(s) and the copyright owner(s) are credited and that the original publication in this journal is cited, in accordance with accepted academic practice. No use, distribution or reproduction is permitted which does not comply with these terms.
*Correspondence: Pekka Matomäki, cG1hdG9tYWtpQGdtYWlsLmNvbQ==