- 1Department of Gastroenterology, Tongji Hospital of Tongji Medical College, Huazhong University of Science and Technology, Wuhan, Hubei, China
- 2Institute of Liver and Gastrointestinal Diseases, Tongji Hospital of Tongji Medical College, Huazhong University of Science and Technology, Wuhan, Hubei, China
- 3Department of Gastroenterology, Union Hospital, Tongji Medical College, Huazhong University of Science and Technology, Wuhan, Hubei, China
BTB and CNC homologous (BACH) proteins, including BACH1 and BACH2, are transcription factors that are widely expressed in human tissues. BACH proteins form heterodimers with small musculoaponeurotic fibrosarcoma (MAF) proteins to suppress the transcription of target genes. Furthermore, BACH1 promotes the transcription of target genes. BACH proteins regulate physiological processes, such as the differentiation of B cells and T cells, mitochondrial function, and heme homeostasis as well as pathogenesis related to inflammation, oxidative-stress damage caused by drugs, toxicants, or infections; autoimmunity disorders; and cancer angiogenesis, epithelial-mesenchymal transition, chemotherapy resistance, progression, and metabolism. In this review, we discuss the function of BACH proteins in the digestive system, including the liver, gallbladder, esophagus, stomach, small and large intestines, and pancreas. BACH proteins directly target genes or indirectly regulate downstream molecules to promote or inhibit biological phenomena such as inflammation, tumor angiogenesis, and epithelial-mesenchymal transition. BACH proteins are also regulated by proteins, miRNAs, LncRNAs, labile iron, and positive and negative feedback. Additionally, we summarize a list of regulators targeting these proteins. Our review provides a reference for future studies on targeted drugs in digestive diseases.
Introduction
Bric-a-brac-Tramtrack-Broad (BTB) and cap “n” collar (CNC) homologue (BACH) transcription factors, including BACH1 and BACH2, are members of the basic leucine zipper (bZIP) family (Oyake et al., 1996). BACH transcription factors comprise a bZIP domain, BTB domain, cysteine–proline (CP) motifs, cytoplasmic localization signal (CLS), and intrinsically disordered regions (IDRs) (Igarashi et al., 2017). The structure of the BACH proteins and the functions corresponding to each structure are shown in Figure 1.
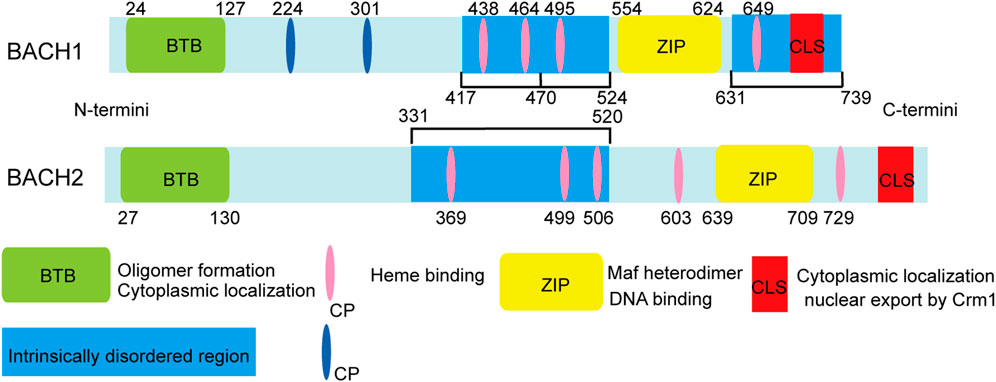
FIGURE 1. Structure and function of mouse BACH1 and BACH2. The amino acid positions of cysteines are indicated by numbers. The intrinsically disordered heme binding region of BACH1 can be divided into three functional parts: the first part (417–470), including two CP motifs, affects activator recruitment by binding to the local conformation of the five-coordinate heme and is involved in other protein-protein interactions; the second part (471–524), including one CP motif, participates in dissociation from DNA; and the last part (331–520), which contains one CP motif, may participate in destabilization of the BACH1/MAFK heterodimer and subsequent DNA dissociation.
The bZIP domain of BACH transcription factors mediates heterodimer formation with small musculoaponeurotic fibrosarcoma (MAF) proteins MAFF, MAFG, and MAFK, and controls DNA binding to repress the transcription of the target gene (Zhou et al., 2016). BACH transcription factors can identify and bind to MAF recognition elements, which are well conserved in both mouse and human gene promoters (Igarashi and Watanabe-Matsui, 2014). Consequently, BACH1 can block the transcription of several oxidative stress-response genes, including heme oxygenase-1 (HO-1/HMOX1), and NADPH and quinone oxidoreductase 1 (NQO1). Accumulated nuclear factor erythroid 2-Like 2 (NRF2) competes with BACH1 to form a heterodimer with MAF proteins. In this way, NRF2 activates the transcription of genes, such as H O -1 which encodes an anti-oxidant defense enzyme. HO-1 degrades heme into ferrous iron, biliverdin, and carbon monoxide (Zhang X. et al., 2018). Figure 2 illustrates the regulation of HO-1 via the BACH1/NRF2 pathway. In addition to repressing HO-1 transcription, BACH2 and bZIP ATF-like transcription factor form heterodimer that binds to the activator protein 1 (AP-1) motif, which contains regulatory regions of Th2 cytokine gene loci (Kuwahara et al., 2016).
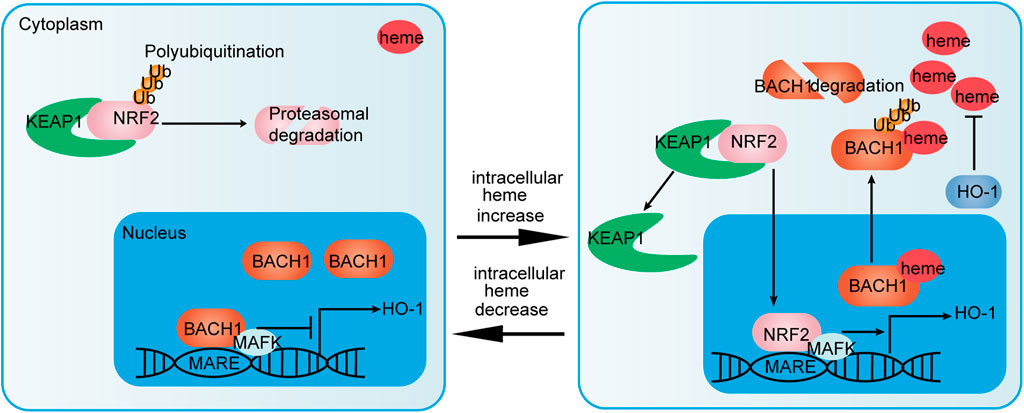
FIGURE 2. NRF2/BACH1 pathway that regulates HO-1 expression. NRF2/MAFK heterodimers activate HO-1 expression. Activation of HO-1 expression is inhibited by BACH1/MAFK heterodimers at low heme concentrations. Meanwhile, NRF2 is polyubiquitinated and degraded by interacting with Kelch-like ECH-associated protein 1 (KEAP1) (left). At high heme concentrations, heme binds to BACH1. Then, BACH1 dissociates from MAF recognition elements (MARF), is exported to the cytoplasm and ubiquitinated for degradation. NRF2 nuclear accumulation via heme-mediated disruption of NRF2/KEAP1 complexes promotes the formation of NRF2/MAFK heterodimers (right). In this way, the NRF2/BACH1 pathway affects the expression of HO-1 and regulates heme homeostasis.
The BTB domain, also known as the pox virus and zinc finger domain, is located at the N-terminus of BACH proteins (Oyake et al., 1996). The analysis of BTB proteins from 17 eukaryotic species revealed that the structure of BTB is highly conserved (Stogios et al., 2005). The BTB domain has a unique three-dimensional fold with a large protein–protein interaction surface and highly variable exposed residues, thereby allowing dimerization and oligomerization, as well as interaction with several other proteins (Perez-Torrado et al., 2006). BACH1 inhibits angiogenesis and the enhancer activity of locus control region in a BTB domain-dependent manner. Moreover, the BTB domain of BACH1 is necessary for the formation of large-looped DNA between two distant binding sites in vitro. The formation of BACH1/MAFK heterodimers through the BTB domain generates a multivalent DNA binding complex (Yoshida et al., 1999; Jiang L. et al., 2020). The BTB domain of BACH2 coordinates the interaction with HDAC3-containing co-repressor complexes to regulate the protein levels of Blimp-1 via epigenetic modifications in B cells (Tanaka et al., 2016).
The activity of the CLS, which is a highly conserved structure at the C-terminus of the BACH proteins, depends on a segment of nonhydrophobic amino acids. Oxidative stress disrupts the activity of CLS to induce the nuclear accumulation of BACH2 (Hoshino et al., 2000). Cadmium induces HO-1 expression and promotes the CLS-mediated nuclear export of BACH1 and BACH2 (Suzuki et al., 2003). Furthermore, the nuclear export of BACH1 depends on the activities of extracellular signal-regulated kinase-1/2 (ERK1/2) and exporter chromosome maintenance 1 (Crm1/Exportin-1) (Suzuki et al., 2003). Xpo1, also known as Crm1, binds to BACH1 and exports it to the cytoplasm, which promotes the formation of transcriptional complexes with NRF2 and small MAF proteins (Suzuki et al., 2004).
BACH1 consists of six CP motifs, whereas BACH2 consists of five CP motifs. BACH1 and BACH2 directly bind to heme through the C-terminal region with four and five CP motifs, respectively (Ogawa et al., 2001; Watanabe-Matsui et al., 2011). BACH proteins consist of two modes of heme binding: five- and six-coordination modes (Hira et al., 2007; Watanabe-Matsui et al., 2011). The five-coordinate mode is unique to BACH1 CP motifs, whereas six-coordination mode binding occurs nonspecifically (Segawa et al., 2019b). Heme binds multiple CP motifs within the IDRs of the BACH proteins, thereby inhibiting their DNA-binding activity and inducing nuclear export, polyubiquitination, and degradation of the BACH proteins (Zhou et al., 2016; Segawa et al., 2022). BACH1 degradation is induced by the heme binding of E3 ubiquitin ligase Hoil-1, Fbxl17 and Fbxo22. However, the specific mechanisms through which heme induces BACH2 polyubiquitination and degradation remain unknown (Zenke-Kawasaki et al., 2007; Tan et al., 2013; Lignitto et al., 2019).
IDRs generally lack long hydrophobic amino acid sequences, resulting in their inability to form a well-organized hydrophobic core comprising a structural domain, but they still perform certain biological activities (Oldfield and Dunker, 2014). Furthermore, IDRs not only recognize and recruit partners, such as proteins, but also participate in conformational changes, and post-translational modifications (van der Lee et al., 2014). Heme binds to BACH1 C-terminal IDRs through a conformational change (Segawa et al., 2019a). A recent study showed that different CP motifs in the heme-bound BACH1 IDRs play roles in co-activator recruitment, dissociation from DNA, and heterodimer destabilization (Segawa et al., 2022). In intrinsically-disordered heme binding regions of BACH2, heme binding induces disordered conformational alterations and more compact BACH2 IDRs conformations (Watanabe-Matsui et al., 2015; Suenaga et al., 2016).
BACH proteins were identified in 1996, and since then a growing number of studies have shown that BACH transcription factors are widely expressed in human tissues. These transcription factors regulate the development and function of innate and adaptive immune systems; progression, and metabolism of many cancers; infection; apoptosis; angiogenesis; lymphangiogenesis; oxidative stress injury; and senescence, and pluripotency of embryonic stem cells (Igarashi et al., 2017; Zhang X. et al., 2018; Cohen et al., 2020; Niu et al., 2021; Padilla and Lee, 2021; Chu et al., 2022). For instance, BACH2 controls CD4+ and stem-like CD8+ T-cell differentiation (Watanabe-Matsui et al., 2011; Yao et al., 2021). Both BACH1 and BACH2 inhibit the myeloid program to promote B cell development (Itoh-Nakadai et al., 2014). In this review, we summarize the pathophysiological role of BACH proteins in digestive system diseases including hepatitis C virus (HCV) infection, nonalcoholic steatohepatitis (NASH), hepatic and intestinal injury induced by drugs and other causes, immune-mediated intestinal diseases [inflammatory bowel disease (IBD) and celiac diseas], biliopancreatic diseases, and digestive system cancers (Table 1). The relationship between BACH proteins and the above diseases is shown in Figure 3. We find that BACH1 aggravates benign diseases mainly by inhibiting the expression of downstream molecules, such as transcriptional inhibition of HO-1, and promotes cancer progression of the digestive system mainly by upregulating the expression of downstream molecules, such as transcriptional activation of IGF1R. In addition, BACH1 has different regulatory effects in different cells or different microenvironments of the same cells. BACH2 affects tumor progression and immune-related benign diseases by regulating the differentiation and function of immune cells.
BACH proteins and benign diseases of the digestive system
Hepatobiliary diseases
Hepatitis C virus
The incidence of HCV has been decreasing; however, there was still approximately 56.8 million HCV infections worldwide in 2020 (Polaris Observatory HCV Collaborators, 2022). One study found that reduced BACH1 and elevated HO-1 levels played critical roles in reducing the cytotoxicities of HCV proteins in hepatocellular carcinoma (HCC) cells (Ghaziani et al., 2006). BACH1 is involved in the mechanism of action of miR-let-7c, miR-196, and miR-122 inhibiting HCV replication in vitro. Both miR-let-7c and miR-196 suppress BACH1 expression by directly acting on the 3′-UTR of BACH1 mRNA to downregulate the levels of HCV protein. A decline in BACH1 expression reduces HCV replication by promoting HO-1 expression to inhibit the activity of viral protease and stimulate an antiviral interferon response. Whether miR-122 suppresses HCV replication through the above mechanism remains to be investigated (Shan et al., 2007; Hou et al., 2010; Chen et al., 2019). Decreased HO-1 expression in patients with HIV/HCV co-infection may indicate a worse prognosis. However, HO-1 expression is positively correlated with BACH1 and miR-122 expression in patients with HCV (Jabłonowska et al., 2014). The expression of hepatic NRF2 and BACH1, and serum HO-1 result in the destructive increase in acetaminophen (APAP)-induced liver injury (Abo El-Magd and Eraky, 2020). HO-1 expression in patients with HCV may be affected by NRF2, which competes with BACH1 for binding to the HO-1 gene. Further, Legalon-SIL (LS) and statins decreased HCV replication and influenced the NRF2/BACH1/HO-1 pathway (Mehrab-Mohseni et al., 2011; Wuestenberg et al., 2014). These findings suggest that BACH1 regulates HCV replication and cytotoxicity of HCV proteins, and may affect drug efficacy.
Nonalcoholic steatohepatitis
Nonalcoholic fatty liver disease, which is the leading cause of chronic liver disease, affects the health of more than one billion people. NASH, a severe form of nonalcoholic fatty liver disease, can progress to cirrhosis, HCC, and death (Younossi et al., 2019). A methionine-choline deficient (MCD) diet was fed to wild type (WT) and BACH1(−/−) mice and the WT mice were shown to develop evident hepatic steatosis with a six-fold increase in hepatic triglyceride content; however, BACH1(−/−) mice exhibited negligible hepatic steatosis. Additionally, alanine aminotransferase (ALT) plasma concentrations and hepatic malondialdehyde (MDA) levels increased and peroxisome proliferator-activated receptor α and microsomal triglyceride transfer protein mRNA levels were downregulated in WT mice fed an MCD diet; however, no significant changes were observed for these parameters in BACH1(−/−) mice (Inoue et al., 2011). BACH1 also plays a role in hepatocyte-specific Sirt6-knockout (KO) and WT mice fed a high-fat and high-fructose diet. KO mice presented with increased steatohepatitis and fibrosis via elevated BACH1 expression, because Sirt6 interacts with BACH1, thereby inducing its detachment from the HO-1 promoter (Ka et al., 2017). These studies suggest that BACH1 deficiency plays a hepatoprotective role in NASH.
Drug-induced liver injury
Drug-induced liver injury (DILI) is the most common cause of acute liver failure in the United States and the United Kingdom (Lee, 2003; Bernal and Wendon, 2013). DILI is one of the most challenging liver diseases faced by gastroenterologists, as it has a wide variety of clinicopathological phenotypes and lacks specific biomarkers (European Association for the Study of the Liver et al., 2019). Two studies have investigated the role of Bach1 single nucleotide polymorphisms (SNPs) in anti-tuberculosis drug-induced hepatotoxicity (ATDH) in 870 Chinese and 100 Japanese patients. These studies revealed that Bach1 SNPs were significantly associated with ATDH; however, this was observed with different tagSNPs (rs372883 and rs1153285 in the Chinese study, and rs2070401 in the Japanese study). The differences in Bach1 tagSNPs could be attributed to the different tagSNP selection criteria or different allele or genotype frequencies in different patients (Nanashima et al., 2012; Zhang H. et al., 2018). Additionally, XPO1 encodes the protein Xpo1, which can bind to BACH1 to indirectly influence its antioxidative activity. Patients with the tagSNP rs4430924A in XPO1 were at a higher risk of developing ATDH than those with rs4430924G (He et al., 2019). SNPs are potential biomarkers for the diagnosis of ATDH.
APAP overdose is a common cause of acute liver injury (Ghanem et al., 2016). Serum HO-1, hepatic BACH1, and NRF2 levels and the nuclear export of NRF2 and BACH1 increase in patients with APAP-induced liver injury. Both vitamin D and omega-3 fatty acids protect against APAP-induced liver injury by decreasing serum HO-1 and hepatic NRF2 and BACH1 levels. Furthermore, omega-3 fatty acids showed preventive effects by promoting the nuclear export and accumulation of BACH1 and NRF2, respectively (Abo El-Magd and Eraky, 2020; Eraky and Abo El-Magd, 2020). These findings indicate that the massive and destructive elevation of BACH1 may contribute to APAP-induced liver injury and decreased BACH1 expression and nuclear translocation may alleviate the extent of liver injury.
Sepsis-induced liver injury
Sepsis-induced liver injury is an important independent risk factor for mortality in the intensive care units (Sun J. et al., 2020). Some clinical features of sepsis are attributed to an endotoxin-induced increase in vascular endothelial permeability; therefore, endotoxemia is often used to mimic the hyperinflammation associated with early sepsis and aid the understanding of the molecular mechanisms of sepsis (Aird, 2003; Dickson and Lehmann, 2019). Tanioka et al. (2021) used lipopolysaccharides (LPS) to establish an endotoxin-induced liver-injury rat model. Nuclear BACH1 proteins showed a significant but transient decline by 1 h after LPS injection, followed by a rapid increase to baseline levels by 3 h Bach1 mRNA elevated shortly after the transient decrease in nuclear BACH1 proteins, then rapidly returned to baseline levels by 24 h. D-galactosamine and LPS were used to establish the endotoxin-induced hepatic injury model in WT and BACH1(−/−) mice. Plasma ALT and aspartate aminotransferase (AST) activities were reduced in BACH1(−/−) mice (Iida et al., 2009). Sepsis was induced by cecal ligation and puncture in WT and BACH1(−/−) mice. In septic mice, knockout BACH1 resulted in increased hepatic HO-1 expression as well as hepatic and pulmonary blood flow, but also attenuated oxidative stress, inflammation, hepatic injury (including liver mitochondrial dysfunction), and mortality. Inhibiting HO-1 activity worsened organ function in KO mice following sepsis (Cai et al., 2022). In summary, BACH1 is a potential therapeutic target in sepsis-induced liver injury.
Other types of liver injury
Carbon tetrachloride (CCl4) is a toxic agent commonly used to induce acute or chronic liver injury (Unsal et al., 2021). BACH1 expression was upregulated in liver fibrosis and CCl4-induced liver injury. San Wei Gan Jiang San, an ancient medicine, alleviates CCl4-induced chronic liver injury and fibrosis by reducing BACH1 expression as well as increasing BACH1 nuclear export and NRF2 expression to regulate oxidative stress, including increasing GST, GSH-Px, and HO-1 levels and GSH/GSSG ratio, and reducing MDA levels (Chen et al., 2020). In pulmonary fibrosis, BACH1 expression is upregulated and BACH1 knockdown ameliorates fibrosis and inflammation by inhibiting the ERK signal pathway (Liu Y. et al., 2021). In addition, decreased nuclear BACH1 levels plays a protective role against acute CCl4-induced liver injury, hepatitis, and oxidative damage of hepatocytes (Cai et al., 2021). Those suggest that BACH1 contribute to acute and chronic liver injury.
Aflatoxin B1 (AFB1), a mycotoxin that affects humans and animals, is a group 1 human carcinogen; however, the mechanism of toxicity is poorly understood (Marchese et al., 2018). BACH1 deficiency reduces AFB1-induced liver oxidative damage by upregulating antioxidant genes. A kind of small molecule inhibitor of BACH1, named 1-Piperazineethanol, α-[(1,3-benzodioxol-5-yloxy) methyl]-4-(2-methoxyphenyl) (M2), ameliorates AFB1-induced human cell death in vitro and liver injury in vivo (Zhang et al., 2022). These findings suggest that BACH1 contributes to acute and chronic liver injury.
Arsenic accumulates in organs, particularly in the liver where it may cause liver injury and increase the risk of liver cancer (Palma-Lara et al., 2020). Transcription repressor BACH1 nuclear export increased and the expression of the downstream target genes HO-1, NADPH, and NQO1 increased in inorganic arsenic-induced liver injury samples, whereas BACH1 expression levels showed no change after organic arsenic treatment (Liu D. et al., 2013; Liu D. et al., 2021). Despite these findings, the functions of BACH1 in arsenic-induced liver injury warrants further study.
BACH proteins are associated with aryl hydrocarbon receptor (AhR)-mediated hepatotoxicity, liver ischemia-reperfusion (IR) injury, and radiation induced cytotoxicity. Hepatic BACH1 expression was upregulated in an AhR-mediated hepatotoxic mouse model (Fader et al., 2017). The reduction of BACH1 mediated by miR-27a-5p, increased Bcl-2 and decreased caspase-3 to alleviate apoptosis in liver IR injury (Xing et al., 2018). Microarray analysis showed that the liver is the main organ affected by radiation therapy and BACH2 expression is upregulated during radiotherapy (Karim et al., 2016).
Cholestasis
Cholestasis is a disease caused by mechanical obstruction of bile transport or changes in hepatocyte function to produce bile (Salas-Silva et al., 2019). Excess estrogen decreases bile flow, which results in estrogen-induced cholestasis (Zu et al., 2021). Rats given ethinylestradiol for 5 days exhibited cholestasis. HO-1 levels were induced by heme 24 h prior to ethinylestradiol administration. The results showed that heme promoted HO-1 via the NRF2/BACH1 pathway; therefore, cholestasis was reduced in two ways: by stimulating hepatic Mrp3 expression to promote bile flow and increasing urinary bile-acid clearance by up-regulating renal Mrp2/Mrp4 expression or down-regulating renal Mrp3 expression (Muchova et al., 2015). These findings indicate that BACH1 may be a contributing factor for estrogen-induced cholestasis.
Primary sclerosing cholangitis
Primary sclerosing cholangitis (PSC) is a relatively rare disease characterized by multifocal biliary strictures with bile duct fibrosis. IBD is present in 70% of patients with PSC and there is a high risk of cholangiocarcinoma and colorectal cancer (CRC) (Dyson et al., 2018). Immunochip analysis of 3,789 PSC cases and 25,079 population controls showed that SNPs of the BACH2 locus, rs56258221, are significantly associated with susceptibility to PSC. This association exhibits a low to moderate linkage disequilibrium (LD) with a BACH2 variant of type 1 diabetes (T1D) and Crohn’s disease (Liu J. Z. et al., 2013). These findings suggest that rs56258221 may serve as a biomarker for PSC and patients with PSC and IBD may be closely associated with the LD of BACH2.
Intestinal diseases
Inflammatory bowel disease
IBDs, including ulcerative colitis (UC) and Crohn’s disease (CD), are chronic and progressive disorders associated with genetic, environmental, microbial and immune factors (Agrawal et al., 2021). Bioinformatic analysis predicted BACH1 to be the key transcription factor regulating nine hub genes, which were correlated with Mayo scores in patients with UC (Ding et al., 2021). In addition to decreasing disease activity index, BACH1 depletion dramatically increases HO-1 expression to exert an intestinal mucosal protective effect in a DSS-induced colitis mouse model (Takagi et al., 2018; Dun et al., 2021). Furthermore, BACH1-deficient macrophages exhibit distinctly increased HO-1 levels and manifest the M2 macrophage marker to suppress TNBS-induced colitis (Harusato et al., 2013). BACH1 deficiency in mouse macrophages affects mitochondrial function, including regulation of mitochondrial energy metabolism (increased glycolysis and decreased oxidative phosphorylation), elevated mitochondrial membrane potential and the levels of mitochondrial reactive oxygen species (ROS), and decreased PINK1/Parkin-mediated mitophagy levels. Downregulated expression of BACH1 not only promotes NLRP3 inflammasome activation by regulating inflammatory factor IL-1β expression, but also increases pro-inflammatory cytokines IL-6 and tumor necrosis factor α (TNFα) (Pradhan et al., 2022). Studies indicated that BACH1 may be a candidate gene for target therapy of IBD.
Bach2 (rs1847472) is a UC susceptibility locus (Romani et al., 2021). Christodoulou et al. identified a potentially deleterious variation of Bach2 in pediatric patients with UC (Christodoulou et al., 2013). BACH2 is upregulated in UC and significantly increases in signaling by interacting with interleukins. Additionally, target genes of BACH2, including MMP7, MMP9, AKT3 and GNGT2, are involved in estrogen signaling. The overexpression of BACH2 probably influences UC by interleukins or estrogen signaling (Ding et al., 2021). Genome-wide meta-analysis demonstrated that rs1847472 of Bach2 is also a confirmed CD susceptibility locus and is associated with T1D and celiac disease (Franke et al., 2010). Furthermore, rs1847472-C in Bach2 resulted in an increased risk of nonmelanoma skin cancers (Cushing et al., 2022). A clinical cohort study showed that rs1847472 was also a CD recurrence susceptibility locus after intestinal resection. The rs1847472 may increase the risk of a hyperinflammatory response to gut microbiota after bowel resection (Laffin et al., 2018). Emerging evidence suggests that mucosal immunity plays a central role in intestinal immune homeostasis. IgA production by B cells is a central role in mucosal immunity (Graham and Xavier, 2013). BACH2 promotes immunoglobulin class switch by repressing the regulatory network of plasma-cell gene in B cells (Muto et al., 2010). The function of BACH2 in IBD may be associated with the induction of immunoglobulin class switch. BACH2 forms a transcriptional network with RORγt, FOSL2, AP-1, and RUNX1 to control wound healing. The downregulation of BACH2 regulates the production of CD161+ regulatory T (Treg) cells which enhances wound healing of the colorectal epithelium and is associated with reduced inflammation in IBD (Povoleri et al., 2018). Although BACH2 is closely related to the pathogenesis of UC and CD, more studies are needed to elucidate its roles.
Celiac disease
Coeliac disease (CeD) is a relatively common and overlooked immune-mediated disorder that occurs in genetically susceptible individuals. The gluten-free diet is currently the only available management plan, but it is not curative or universally effective (Pinto-Sanchez et al., 2021). A study showed that rs10806425 of Bach2 is strongly implicated in CeD pathogenesis (Medrano et al., 2019). BACH2 expression is significantly reduced in CD4+ T cells isolated from the blood of patients with CeD. Differentially expressed genes regulated by BACH2 are highly enriched and over-represented in the above CD4+ T cells (Quinn et al., 2015). As a main factor regulating CD4+ T cell differentiation, BACH2 controls the efficient formation of Treg cells and inhibits Treg-dependent lethal inflammation. Mild and incompletely penetrating bowel inflammation appeared in some BACH2(−/−) mice (Roychoudhuri et al., 2013). Decreased BACH2 expression has been shown to promote a pro-inflammatory response through lack of efficient Treg cell formation and may contribute to partial CeD and IBD.
Other intestinal diseases
Non-steroidal anti-inflammatory drugs (NSAIDs) are used worldwide with the most common side effect including gastrointestinal damage called NSAID-associated enteropathy (Scarpignato and Hunt, 2010). In addition to suppressing apoptosis, disruption of BACH1 effectively inhibits inflammatory chemokines such as keratinocyte chemoattractant (KC), MIP1α, and MCP1 to ameliorate indomethacin-induced intestinal injury in mice (Harusato et al., 2009; Harusato et al., 2011).
Intestinal ischemia-reperfusion (IR) injury is a complex disease with high morbidity and mortality (Ding et al., 2020). The downregulation of BACH1 expression distinctly reduces the levels of myeloperoxidase, hemoglobin, KC, luminal protein, and TNFα in intestinal IR injury mice. Simultaneously, this decreases PMN infiltration into the small intestine and attenuates the activation of NF-κB to reduce the levels of adhesion proteins, E-selectin and ICAM-1. Attenuation of myeloperoxidase and luminal protein expression can be reversed by reducing HO-1 expression (Katada et al., 2022). A similar mechanism involving BACH1 has been observed in cerebral IR injury (Yu et al., 2020). These findings reveal that BACH1 deficiency alleviates intestinal IR injury by increasing HO-1 expression. BACH2 deletion facilitates intestinal epithelial regeneration by promoting DNA repair of intestinal crypt cells in radiation-induced intestinal injury (Li et al., 2021).
Pancreatic diseases
Chronic pancreatitis
Chronic pancreatitis (CP) is a multifactorial fibroinflammatory syndrome of the exocrine pancreas. CP incidence has been increasing gradually worldwide, with no effective curative therapy (Beyer et al., 2020). BACH2 expression downregulates in the pancreas tissue and circulation of patients with CP. The repression of BACH2 expression leads to the polarization of T-helper cells toward Th17 cells and increased inflammation in patients with CP. Additionally, the rs9111-TT genotype in the 5′-UTR region of BACH2 decreases the expression of BACH2 and is associated with clinical features of advanced CP (Sasikala et al., 2018). This suggests that BACH2 plays an important role in protecting from CP by regulating immunity.
Conclusion of benign diseases of the digestive system
BACH proteins play an indispensable role in inhibition of HCV replication, intestinal mucosal protection, inflammation regulation, alleviation of steatohepatitis and fibrosis, and digestive-system damage caused by drugs, poisons, or ischemia-reperfusion. BACH proteins affect benign diseases mainly through oxidative stress and inflammation (Figures 4A, B).
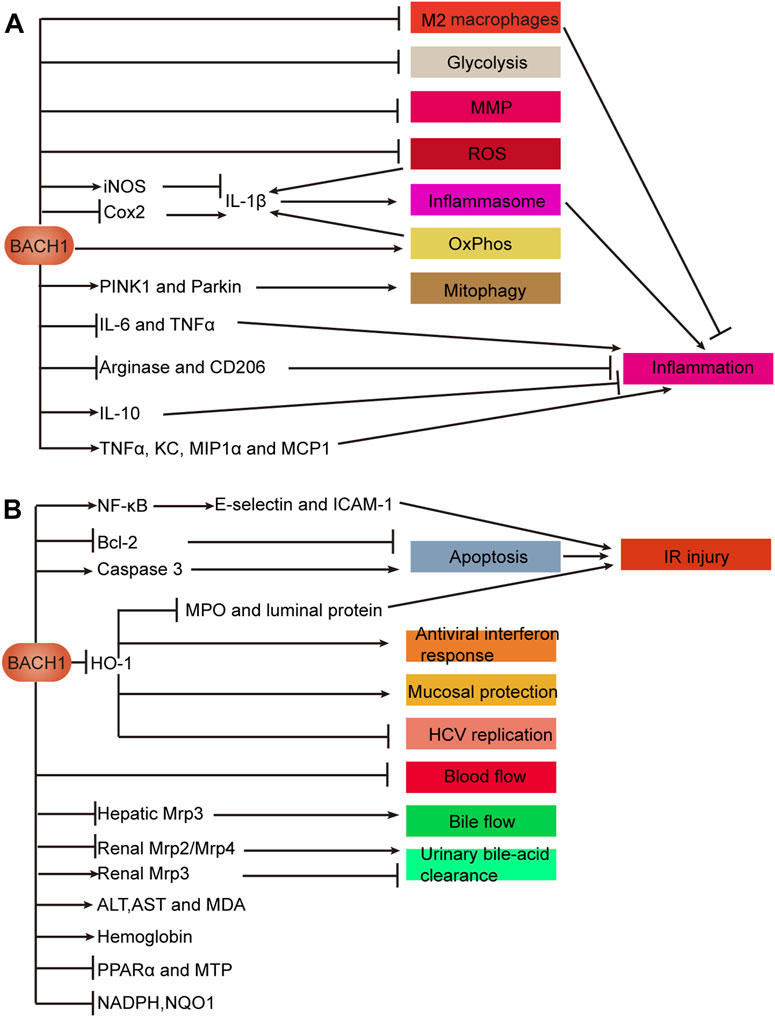
FIGURE 4. Mechanisms of BACH1 in benign diseases of the digestive system. (A) BACH1 regulates inflammation. (B) Effects of BACH1 on biological processes such as oxidative stress. A In macrophages, BACH1 inhibits glycolysis, MMP, and ROS and induces mitophagy via the PINK1/Parkin pathway. BACH1 decreases the number of M2 macrophages to promote inflammation. Inflammatory factor IL-1β is regulated by BACH1 in a variety of ways, as illustrated. IL-1β promotes the activation of the NLRP3 inflammasome to promote inflammation. Furthermore, BACH1 regulates inflammation by promoting or inhibiting anti-inflammatory markers, including CD206, arginase, and IL-10 and pro-inflammatory cytokines, including IL-6, TNF-α, KC, MIP1α, and MCP1. B BACH1 promotes IR injury involving the NF-κB pathway, apoptosis, and HO-1-mediated protection. BACH1 plays a role in viral replication, anti-interferon response, mucosal protection, and IR injury through transcriptional repression of HO-1. In addition to inhibiting urinary bile-acid clearance and bile and blood flow, BACH1 affects the levels of some proteins and metabolites that reflect liver function. Abbreviations: keratinocyte chemoattractant (KC); ischemia and reperfusion (IR); mitochondrial membrane potential (MMP); reactive oxygen species (ROS); oxidative phosphorylation (OxPhos); microsomal triglyceride transfer protein (MTP); peroxisome proliferator-activated receptor α (PPARα); ischemia-reperfusion (IR).
In HCV, DILI, cholestasis, IBD, IR injury and other diseases, BACH1 transcriptionally inhibits HO-1 to mediate oxidative stress response, which causes aggravated organ function damage and a rise in related indicators. In addition to affecting multiple diseases through similar mechanisms, BACH1 affects the same disease through multiple mechanisms. IR injury is regulated by three BACH1-mediated pathways: HO-1 transcriptional repression, NF-Κb activation, and promotion of apoptosis.
BACH1 regulates the biological function of macrophages and affects the expression of anti-inflammatory and pro-inflammatory factors, thereby inhibiting or promoting inflammation in IBD and other inflammation-related diseases. Interestingly, BACH1 deficiency promotes pro-inflammatory cytokine TNFα expression in bone marrow macrophages but suppresses TNFα expression in intestinal macrophages. The regulation of TNFα by BACH1 may be affected by the microenvironment in which macrophages are located. BACH2 regulates inflammation and the differentiation of T cells which affect inflammation or wound healing in IBD, CeD and CP.
BACH proteins and cancers of the digestive system
By 2040, the global cancer burden is expected to reach 28.4 million cases (Sung et al., 2021). BACH transcription factors, especially BACH1, are involved in the proliferation, metastasis, angiogenesis, metabolism, and chemotherapy resistance of many cancers (Padilla and Lee, 2021). In this section, we focus on discussing the roles of BACH proteins in digestive-system cancers.
Esophageal cancer
Esophageal cancer ranks seventh as the most prevalent type of cancer and sixth in mortality worldwide. Esophageal squamous cell carcinoma (ESCC) is a common histological subtype of esophageal cancer (Sung et al., 2021). BACH1 is involved in LncRNA SNHG8/miR-1270/BACH1 axis in esophageal cancer. LncRNA SNHG8 decreases the level of miR-1270 to increase the expression of BACH1, thereby promoting cancer progression (Wu et al., 2022). BACH1 expression is increased in ESCC tissues compared with that of healthy esophageal epithelial tissues. BACH1 induces epithelial-mesenchymal transition (EMT) by activating CDH2, SNAI2, and vimentin, and facilitates angiogenesis by upregulating the transcriptional activity of vascular endothelial growth factor C, thereby promoting the proliferation and metastasis of ESCC cells (Zhao et al., 2021). BACH1 inhibits Wnt/β-catenin pathway by recruiting HDAC1 to affect transcription of TCF4-targeted genes and impairing the interaction between β-catenin and p300/CBP or TCF4. In this way, BACH1 suppresses angiogenesis after ischemic injury (Jiang et al., 2015). BACH1 also inhibits angiogenesis in pancreatic cancer (Huang et al., 2018). It is suggested that the effect of BACH1 on angiogenesis is different in different diseases. BACH1 inhibits the biosynthesis of monounsaturated fatty acids (MUFAs), especially oleic acid, by transcriptionally inhibiting the SCD1 gene in ESCC cells. In this way, BACH1 induces ferroptosis. Thus, BACH1 suppresses hematogenous metastasis in vivo due to high levels of iron ions and oxidative stress in the blood. BACH1 promotes lymph node metastasis in vivo because lymph is rich in MUFAs such as oleic acid. The concentration gradient of MUFA between the primary lesion and the lymph resulted in the chemoattraction of ESCC cells to induce metastasis. In addition, MUFAs act as a protective coating around ESCC cells, protecting them from ferroptosis (Xie et al., 2023). Further mechanistic studies are required to elucidate the function of BACH1 in esophageal cancer.
Gastric cancer
Gastric cancer (GC) is the common malignant tumor and cause of cancer-related death (Smyth et al., 2020). Fang et al. showed using bioinformatics analyses that BACH1 overexpression indicated good prognosis in patients with GC (Fang and Lu, 2020). A recent study revealed that BACH1 facilitates the polarization of macrophages towards the M2 phenotype by activating Wnt1 and promotes macrophage-dependent GC progression (Yang et al., 2022). These somewhat contradictory results suggest that the role of BACH1 in GC is complex and may be related to the tumor immune microenvironment.
Bioinformatics analyses showed that BACH2 increased and was positively correlated with short survival time in patients with GC of microsatellite instability-high status (Tian et al., 2020). Methylation of the BACH2 promoter was reported to be increased in approximately half of patients with GC and associated with reduced expression of BACH2. Downregulated expression of BACH2 occurred in significantly less frequencies in the diffuse-type gastric cancers compared with that of the intestinal-type gastric cancers. Moreover, BACH2 deficiency has been shown to promote GC cell proliferation in vitro (Haam et al., 2014). These findings indicate that BACH2 is expressed at different levels and may play a positive or negative role in different GC subtypes. Therefore, BACH proteins are worthy of further study to reveal their role in gastric tumorigenesis.
Colorectal cancer
CRC is the second most common cause of cancer-related death, after lung cancer, with more than 1.9 million cases and 930,000 deaths annually worldwide. CRC accounts for approximately one in ten cancer cases and deaths globally. The primary cancer site of CRC is mainly the colon (Sung et al., 2021). A study showed that Bach1 mRNA levels were more upregulated in CRC than in paracancerous tissues (Chang et al., 2016; Chang et al., 2020). Immunohistochemical results showed that BACH1 expression was decreased in CRC tissues compared with that of adjacent healthy tissues, whereas no significant difference was observed in the expression of BACH1 in CRC tissues compared with that of distant healthy tissues (Chang et al., 2013). Another study demonstrated that BACH1 expression was lowest in adenomas, high in colon cancer tissues, and highest in normal tissues adjacent to colon cancer tissues. Increased BACH1 levels were positively associated with tumor progression in colon cancer (El-Deek et al., 2019). BACH1 expression is dynamically altered and likely to be epigenetically modified in colorectal malignant transformation and tumor progression.
In colon cancer, BACH1 expression increases and promotes cancer cell migration by increasing the expression of metastasis-related genes including MMP-1, MMP-9, MMP-13, SNAIL1, CXCR4, and HMGA2. The increased metastasis-related genes may be due to the BACH1-mediated decrease in miR-34a and let-7a (Davudian et al., 2016). The overexpression of BACH1 enhances CRC cell proliferation, migration, and invasion and may be associated with upregulated levels of CD31, vimentin, and CRC4 (Zhu et al., 2018). BACH1 knockdown inhibits STARD8 and TIAM2 expression at the transcriptional level, thereby suppressing CRC metastasis (Chen et al., 2022). Long non-coding RNA NEAT1 directly binds to let-7g-5p, which targets and upregulates BACH1 to facilitate EMT and cell growth of colon cancer (Gao et al., 2021). However, one study showed that the proliferative capacity of colon cancer cells was not affected by BACH1 knockdown in vitro (Davudian et al., 2016). More studies are needed to clarify the role of BACH1 in CRC proliferation. The association between BACH1 expression and mitochondrial function is different in CRC with or without metastasis (Chang et al., 2020). BACH1 negatively regulates mitochondrial metabolism in cancer cells by affecting transcription of mitochondrial respiratory chain genes and glucose utilization in the citric acid cycle (Lee et al., 2019). These findings indicate that BACH1 promotes metastasis by potentially affecting mitochondrial function. In addition, circBACH1 augmented the proliferation, migration, and metastasis of CRC and suppressed apoptosis by inhibiting et-7a-5p, which targets CREB5 and increased CREB5 expression (Li et al., 2020).
Gastrin releasing peptide (GRP) and its receptor (GRPR) are morphogenetic factors, which keep tumor cells at high degree of differentiation and reduce tumor metastasis ability. BACH2 expression is specifically downregulated when GRP/GRPR are aberrantly upregulated in colon cancer (Ruginis et al., 2006). BACH2 proteins promote immune homeostasis and long-term tumor immunosuppression by driving the quiescence and durable maintenance of resting Treg cells. In this way, BACH2 promotes tumor growth in colorectal adenocarcinoma (Grant et al., 2020). In addition to affecting adaptive immunity, deficiency of BACH2 expression promotes natural killer (NK) cell maturation, regulates its function, and suppresses tumor metastasis in mice (Li et al., 2022). In summary, both BACH1 and BACH2 play promoting roles in the progression of CRC.
Liver cancer
The global incidence of liver cancer is projected to exceed 1 million cases by 2025. Hepatocellular carcinoma (HCC) accounts for approximately 80% of the total liver-cancer cases (Forner et al., 2018). The expression of BACH1 was increased in HCC tissues and positively correlated with worse prognosis. Increased BACH1 expression facilitated the proliferation and metastasis of HCC by directly targeting IGF1R and PTK2. Furthermore, IGF2, which can interact with IGF1R, promotes BACH1 expression upregulation via the ERK1/2/ETS1 signaling pathway to form a positive feedback loop in HCC progression (Xie et al., 2022). BACH1 also facilitated transcription of HK2 which promotes glycolysis-induced HCC metastasis (Zhao et al., 2022). A recent study showed that BACH1 promoted cellular inflammatory factors and suppressed autophagy in HCC by negatively regulating the p53 pathway (Sun et al., 2021). In contrast to BACH1, upregulated BACH2 expression may enhance apoptosis in NASP-depleted HCC cells (Kang et al., 2018).
BACH1 is an essential component of Ten-eleven translocation 1 (TET1)/miR-34a/BACH1 axis, through which TET1 negatively modulates BACH1 by demethylating and activating miR-34a (Sun et al., 2021). BACH1 proteins facilitate HCC proliferation, migration, invasion, EMT progress, and cell cycle. LncRNA TRG-AS and Lnc712 interact with miR-4500 and miR-142-3p, respectively, to induce an increase in BACH1 expression (Sun X. et al., 2020; Cui and Ni, 2020). Paradoxically, BACH1 regulated by miR-25-3p and let-7a-5p induces the accumulation of ROS while suppressing cell proliferation (Wang et al., 2020). BACH1 binds to the two identified binding motifs (13271nt and 19595nt) in TKT to inhibiting TKT expression. The knockdown of TKT expression affects HCC cells in multiple ways; it sensitizes HCC cells to sorafenib, suppresses cell proliferation and lung metastasis, decreases glucose uptake in vitro, alters glutathione metabolism, increases intracellular ROS levels, and induces oxidative stress-related G1 phase arrest (Xu et al., 2016).
One study reported that circBACH1 accelerated cell cycle progression to promote HCC growth by interacting with HuR to block the translation of p27, which regulates cell cycle (Liu et al., 2020). CircBACH1 was also reported to promote HBV replication and HCC development by sponging miR-200a-3p to downregulate MAP3K2 expression (Du et al., 2022). Collectively, circBACH1 is an oncogenic factor of HCC and BACH1 plays an important role in HCC progression, metabolism, oxidative stress, and chemoresistance.
Cholangiocarcinoma (CCA)
CCA is a highly aggressive and chemoresistant malignant tumor of the digestive system (Rodrigues et al., 2021). A recent bioinformatics analysis showed that BACH1 expression decreased in CCA (Liu Z. et al., 2022). Increased BACH1 expression, mediated by the nuclear accumulation of FOXO1, represses the transcription of genes encoding proteasome subunits. Decreased PTEN expression downregulates the nuclear translocation of FOXO1 via the PI3K/AKT pathway, resulting in dependency on the proteasome for CCA cell growth and survival and sensitivity to proteasome inhibitors. This mechanism may be widespread in many cancers (Jiang T.-Y. et al., 2020). This study suggests that BACH1 is a potential biomarker and therapeutic target for resistance to proteasome inhibitor chemotherapy in CCA and other cancers.
Pancreatic cancer
Pancreatic cancer is a clinically challenging cancer. The prognosis of pancreatic cancer is very poor, with a median survival of less than a year after treatment (Wood et al., 2022). Therefore, there is an urgent need for efficient treatment methods, such as molecular targeted therapy.
BACH1 is mainly found in islet cells of the healthy human pancreas, acinar and ductal cells of CP, and ductal and stromal cells of pancreatic ductal adenocarcinoma (PDAC). Additionally, BACH1 is present in urine exosomes. BACH1 is dynamically expressed in cancer development from the early lesions to invasive stages and are more highly expressed in cancer tissues than in normal tissues. Moreover, BACH1 levels are inversely correlated with prognosis in patients with PDAC (Kim et al., 2021). BACH1 increases labile iron by inhibiting the expression of the ferritin subunit genes FTH1 and FTL. On the one hand, labile iron promotes EMT by inhibiting the expression of the epithelial gene E-cadherin. On the other hand, labile iron promotes BACH1 degradation by promoting Fbox22 expression. BACH1 and labile iron form negative feedback regulation (Liu et al., 2022). BACH1 has been shown to promote cancer metastasis by promoting EMT. BACH1 promotes EMT by suppressing the expression of E-cadherin, FOXA1, and tight junction proteins (OCLN, CLDN3, CLDN4) and promoting the expression of vimentin and SNAI2. ChIP-sequencing analysis showed that BACH1 directly inhibited the expression of CLDN3, CLDN4, FOXA1, and PKP2, whereas it directly activated SNAI2 expression (Sato et al., 2020). The expression of E-cadherin is induced by FOXA1 and inhibited by SNAI2 (Song et al., 2010; Carpenter et al., 2015). In vitro and in vivo experiments showed that BACH1 deficiency had no effect on proliferation (Sato et al., 2020). Nevertheless, one study showed that BACH1 expression decreased in patients with PDAC and BACH1 deficiency facilitated the growth of PDAC cells and angiogenesis, most likely by upregulating HO-1 to increase the expression of eNOS, VEGF, and HIF1A, evoke oncogenic AKT and ERK signaling, and inhibit PTEN expression. BACH1 promotes the expression of epithelial cell markers E-cadherin and ZO-1, and inhibits the expression of mesenchymal cell markers ZEB1, vimentin, and Slug, as well as stemness markers ABCG2 and ALDH1. This study also demonstrated that the rs372883C allele in the 3′-untranslated region (3′-UTR) of Bach1 resulted in evidently higher BACH1 levels than the rs372883T allele in both cancer and normal tissues. Additionally, patients with PDAC carrying the rs372883T allele were more resistant to gemcitabine and had worse overall survival compared with those carrying the rs372883C allele. It is suggested that patients with BACH1 deficiency were resistant to gemcitabine and had shorter survival times (Huang et al., 2018). Interestingly, BACH1 deficiency promotes E-cadherin expression in AsPC−1 (derived from ascitic fluid of pancreatic cancer) and SW1990 cells (derived from splenic metastases of pancreatic cancer), but inhibits E-cadherin expression in CFPAC-1 (derived from liver metastases of pancreatic cancer) and BXPC-3 cells (derived from orthotopic pancreatic cancer) (Huang et al., 2018; Sato et al., 2020). It indicated that BACH1 may have different effects on EMT-related genes in pancreatic cancer cell lines of different origins. Further mechanistic studies are needed to elucidate the function of BACH1 in pancreatic cancer.
Conclusion of cancers of the digestive system
BACH proteins regulate ferroptosis, EMT, angiogenesis, stemness, autophagy, chemotherapy resistance, metabolism, the immune microenvironment, proliferation, and metastasis by binding to the promoter of the target gene to affect gene expression or indirectly affecting gene expression (Figure 5). We focus on the mechanism of BACH1 in EMT, angiogenesis, proliferation, and metastasis. BACH1 regulates EMT by controlling the expression of EMT key genes such as CLDN3 or inhibiting the expression of ferritin subunit genes to decrease E-cadherin expression. The effect of BACH1 on EMT-related genes may be quite different in cell lines of different origins. For example, BACH1 inhibits EMT by decreasing vimentin expression in pancreatic cancer but promotes EMT by increasing vimentin expression in esophageal cancer. Angiogenesis is inhibited by the BACH1/HO-1 axis and promoted by the BACH1/VEGFC pathway. BACH1 regulates cancer proliferation by targeting IGF1R and PTK2 and affecting angiogenesis and cell cycle. Cell cycle is promoted by BACH1 and TKT which is transcriptionally suppressed by BACH1. BACH1 promotes metastasis by inducing metastasis-related gene expression, HK2-mediated glycolysis, Wnt1-mediated M2 macrophage polarization, EMT, angiogenesis, and ferroptosis. BACH2 maintains immune homeostasis and durable tumor immunosuppression and induces cancer progression by regulating Treg and NK cells in gastrointestinal tumors.
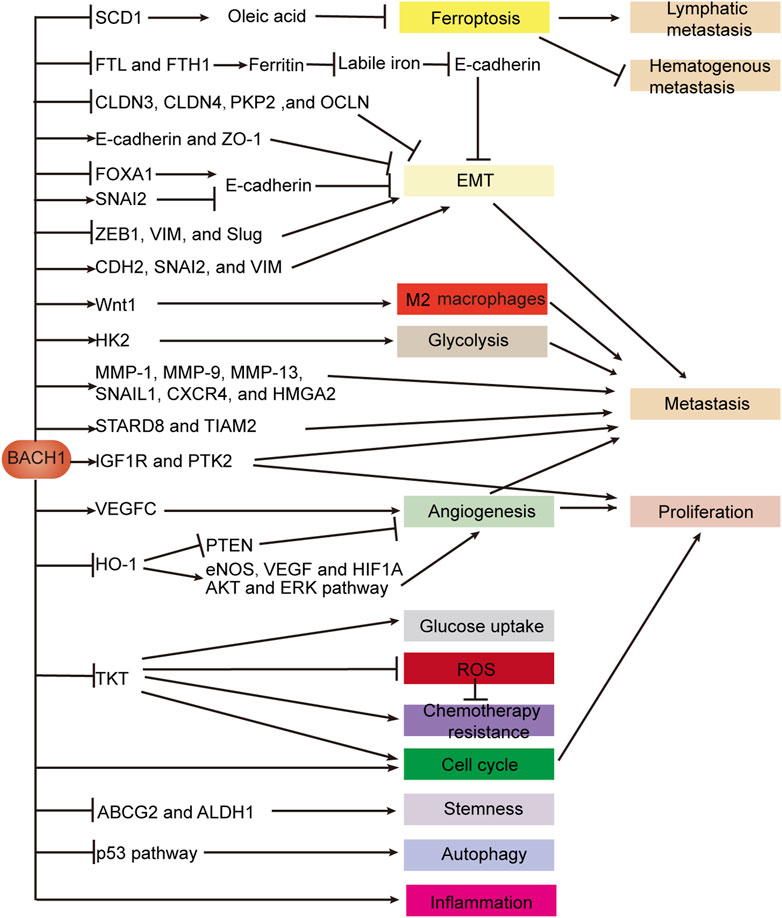
FIGURE 5. BACH1 regulates gene expression in digestive system cancers. BACH1 regulates EMT by activating or silencing genes which are critical for epithelial or mesenchymal cell structure. BACH1 also targets genes encoding ferritin subunits and indirectly promotes EMT by increasing labile iron. BACH1 induces ferroptosis to drive lymphatic metastasis and inhibit hematogenous metastasis by directly suppressing the transcription of SCD1, which is essential for oleic acid production. The expression of metastasis-related genes is indirectly promoted or transcriptionally activated by BACH1. BACH1 not only promotes tumor angiogenesis through VEGFC, but also transcriptionally inhibits HO-1 to inhibit tumor angiogenesis. BACH1 regulates multiple biological steps by directly targeting genes such as TKT and HK2 or indirectly regulating pathways such as the p53 pathway. Abbreviations: vimentin (VIM); epithelial-mesenchymal transition (EMT).
Conclusion
BACH transcription factors are important regulators of pathophysiology in the digestive system. The regulatory role of BACH proteins is dual in a variety of processes such as inflammation, EMT, angiogenesis, cell cycle, and macrophage polarization. For example, BACH1 knockdown promotes M2 macrophage polarization to alleviate colitis and inhibits M2 macrophage polarization to inhibit GC metastasis. ROS production is promoted by BACH1 in cancer cells but inhibited by BACH1 in macrophages. Different cellular functions regulated by BACH proteins are interconnected. For example, BACH1-induced ferrroptosis promotes lymphatic metastasis and inhibits hematogenous metastasis. In inflammation, EMT, and other conditions, BACH proteins regulate the same molecule differently in different cell tissues. For example, BACH1 increases the expression of hepatic Mrp3 and decreases the expression of renal Mrp3. The BACH1/HO-1 pathway plays a role in both benign and malignant diseases of the digestive system. In addition to inhibiting HCV replication and IR injury and promoting mucosal protection and antiviral response, BACH1-mediated HO-1 upregulates the expression of VEGF and other molecules and downregulates PTNE expression to regulate tumor angiogenesis. Although BACH2 has been reported to affect CP, IBD, and cancer by regulating adaptive immunity, the specific functions of BACH2 are unclear.
BACH protein expression levels appear to be related to the severity of IBD, CeD, CP, and the prognosis of patients with cancer. Therefore, these proteins may serve as biomarkers and therapeutic targets for digestive diseases, such as IBD, and tumor progression and prognosis. In addition to BACH expression levels, SNPs in Bach genes are susceptibility loci for IBD, CeD, PSC, ADTH, CP, and PDAC and may serve as biomarkers at the genetic level. Currently, Bach2 SNPs are associated with autoimmune diseases. However, the causal genetic variants within each susceptibility locus are unclear. Mouri et al. demonstrated an efficient method to preferentially select variants and study their relevant roles (Mouri et al., 2022). This will be useful to better characterize the effect of these SNPs on disease.
Pharmacological studies have identified some regulators of BACH. For example, hemin specifically degrades BACH1 with negligible toxicity, inhibits breast cancer growth, and is used to treat patients with acute porphyria (Sun et al., 2002; Chiabrando et al., 2014; Bissell et al., 2017; Lee et al., 2019; Lignitto et al., 2019). Table 2 summarizes some novel molecular compounds capable of modifying the expression and activities of BACH, as well as their function in related diseases. Bach1 gene is transcriptionally repressed by FOXO1 and EST1. BACH1 mRNA and protein levels are affected by miRNAs, labile iron, LncRNAs, TBK1 protein, and TET1 protein. BACH1 protein is also subject to post-translational regulation by TBK1 and labile iron. In addition, BACH1 forms positive feedback with PTEN or IGF1R and negative feedback with labile iron (Figure 6). These findings provide new ideas for the development of agonists or antagonists targeting BACH in the digestive system.
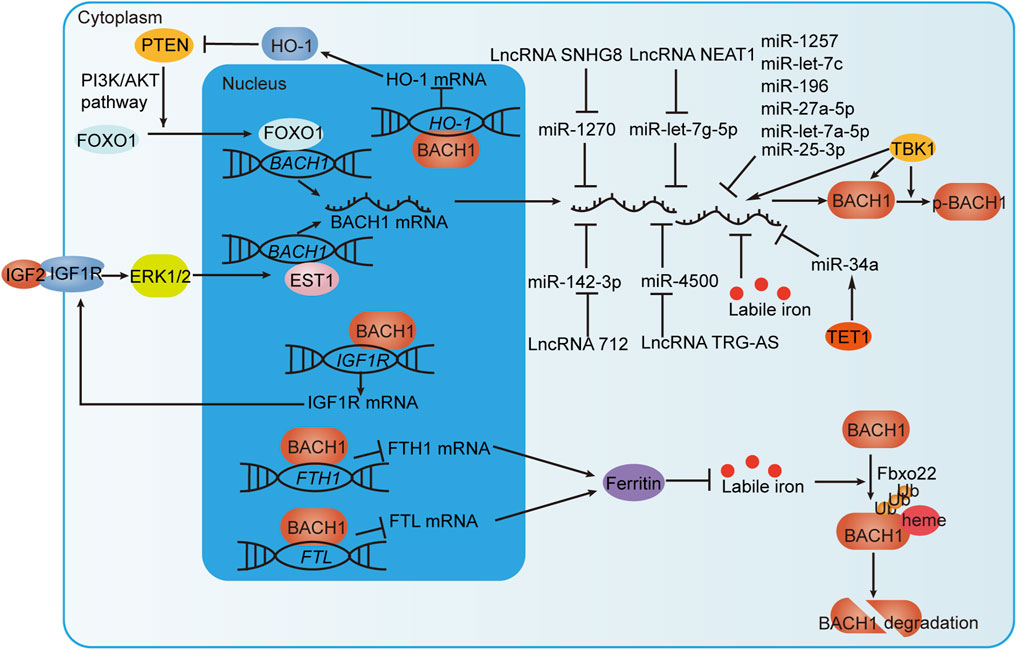
FIGURE 6. BACH1 regulation by transcriptional, post-transcriptional and post-translational mechanisms. IGF1R interacts with IGF2 to promote the transcription of BACH1 via the ERK1/2/EST1 pathway. In addition, BACH1 targets the IGF1R gene to form a positive feedback loop that continuously promotes BACH1 expression. BACH1 increases PNET expression by suppressing HO-1 expression at the transcriptional level. PNET induces BACH1 transcription through transcription factor FOXO1. BACH1 and PNET also form positive feedback. BACH1 mRNA can be directly negatively regulated by miRNAs but also indirectly regulated by TET1 protein or LncRNAs. TBK1 phosphorylates BACH1 and increases BACH1 mRNA and protein levels. Labile iron inhibits the level of BACH1 mRNA and promotes FBXO22-dependent BACH1 degradation. Moreover, BACH1, which targets the FTH1 and FTL genes encoding ferritin subunits, reduces ferritin expression to increase the level of labile iron. In this way, BACH1 and iron form negative feedback.
There has been some progress in understanding BACH proteins. However, many important questions remain. For example, both BACH1 and BACH2 are expressed in the same tissues such as gut, but their temporal and spatial relationships have not been clearly defined. In digestive diseases and tumors, further studies are required to elucidate the mechanisms of action of BACH proteins and aid in the search for valuable regulators targeting BACH proteins to treat diseases.
Author contributions
QS wrote the manuscript. XM and MJ collected the literature. WY and YF revised the manuscript. WY and YF are the co-corresponding authors. All authors contributed to the article and approved the submitted version.
Funding
This study was funded by the Natural Science Foundation of China No. 81974383 (WY) and No. 82273321 (WY).
Conflict of interest
The authors declare that the research was conducted in the absence of any commercial or financial relationships that could be construed as a potential conflict of interest.
Publisher’s note
All claims expressed in this article are solely those of the authors and do not necessarily represent those of their affiliated organizations, or those of the publisher, the editors and the reviewers. Any product that may be evaluated in this article, or claim that may be made by its manufacturer, is not guaranteed or endorsed by the publisher.
References
Abo El-Magd, N. F., and Eraky, S. M. (2020). The molecular mechanism underlining the preventive effect of vitamin D against hepatic and renal acute toxicity through the NrF2/BACH1/HO-1 pathway. Life Sci. 244, 117331. doi:10.1016/j.lfs.2020.117331
Agrawal, M., Spencer, E. A., Colombel, J.-F., and Ungaro, R. C. (2021). Approach to the management of recently diagnosed inflammatory bowel disease patients: A user's guide for adult and pediatric gastroenterologists. Gastroenterology 161 (1), 47–65. doi:10.1053/j.gastro.2021.04.063
Ahuja, M., Ammal Kaidery, N., Attucks, O. C., McDade, E., Hushpulian, D. M., Gaisin, A., et al. (2021). Bach1 derepression is neuroprotective in a mouse model of Parkinson's disease. Proc. Natl. Acad. Sci. U. S. A. 118 (45), e2111643118. doi:10.1073/pnas.2111643118
Aird, W. C. (2003). The role of the endothelium in severe sepsis and multiple organ dysfunction syndrome. Blood 101 (10), 3765–3777. doi:10.1182/blood-2002-06-1887
Attucks, O. C., Jasmer, K. J., Hannink, M., Kassis, J., Zhong, Z., Gupta, S., et al. (2014). Induction of heme oxygenase I (HMOX1) by HPP-4382: A novel modulator of Bach1 activity. PloS One 9 (7), e101044. doi:10.1371/journal.pone.0101044
Bernal, W., and Wendon, J. (2013). Acute liver failure. N. Engl. J. Med. 369 (26), 2525–2534. doi:10.1056/NEJMra1208937
Beyer, G., Habtezion, A., Werner, J., Lerch, M. M., and Mayerle, J. (2020). Chronic pancreatitis. Lancet (London, Engl. 396 (10249), 499–512. doi:10.1016/S0140-6736(20)31318-0
Bissell, D. M., Anderson, K. E., and Bonkovsky, H. L. (2017). Porphyria. N. Engl. J. Med. 377 (9), 862–872. doi:10.1056/NEJMra1608634
Cai, L., Arbab, A. S., Lee, T. J., Sharma, A., Thomas, B., Igarashi, K., et al. (2022). BACH1-Hemoxygenase-1 axis regulates cellular energetics and survival following sepsis. Free Radic. Biol. Med. 188, 134–145. doi:10.1016/j.freeradbiomed.2022.06.005
Cai, Y., Li, B., Peng, D., Wang, X., Li, P., Huang, M., et al. (2021). Crm1-Dependent nuclear export of Bach1 is involved in the protective effect of hyperoside on oxidative damage in hepatocytes and CCl4-induced acute liver injury. J. Inflamm. Res. 14, 551–565. doi:10.2147/JIR.S279249
Carpenter, R. L., Paw, I., Dewhirst, M. W., and Lo, H. W. (2015). Akt phosphorylates and activates HSF-1 independent of heat shock, leading to Slug overexpression and epithelial-mesenchymal transition (EMT) of HER2-overexpressing breast cancer cells. Oncogene 34 (5), 546–557. doi:10.1038/onc.2013.582
Casares, L., Moreno, R., Ali, K. X., Higgins, M., Dayalan Naidu, S., Neill, G., et al. (2022). The synthetic triterpenoids CDDO-TFEA and CDDO-Me, but not CDDO, promote nuclear exclusion of BACH1 impairing its activity. Redox Biol. 51, 102291. doi:10.1016/j.redox.2022.102291
Casares, L., Unciti-Broceta, J. D., Prados, M. E., Caprioglio, D., Mattoteia, D., Higgins, M., et al. (2020). Isomeric O-methyl cannabidiolquinones with dual BACH1/NRF2 activity. Redox Biol. 37, 101689. doi:10.1016/j.redox.2020.101689
Chang, L.-C., Fan, C.-W., Tseng, W.-K., Chein, H.-P., Hsieh, T.-Y., Chen, J.-R., et al. (2016). The ratio of hmox1/nrf2 mRNA level in the tumor tissue is a predictor of distant metastasis in colorectal cancer. Dis. Markers 2016, 8143465. doi:10.1155/2016/8143465
Chang, L.-C., Fan, C.-W., Tseng, W.-K., Chen, J.-R., Chein, H.-P., Hwang, C.-C., et al. (2013). Immunohistochemical study of the Nrf2 pathway in colorectal cancer: Nrf2 expression is closely correlated to Keap1 in the tumor and Bach1 in the normal tissue. Appl. Immunohistochem. Mol. Morphol. AIMM 21 (6), 511–517. doi:10.1097/PAI.0b013e318282ac20
Chang, L.-C., Fan, C.-W., Tseng, W.-K., and Hua, C.-C. (2020). Associations between the Nrf2/Keap1 pathway and mitochondrial functions in colorectal cancer are affected by metastasis. Cancer Biomarkers Sect. A Dis. Markers 27 (2), 163–171. doi:10.3233/CBM-190828
Chen, T., Zhang, X., Ding, X., Feng, J., Zhang, X., Xie, D., et al. (2022). Ryanodine receptor 2 promotes colorectal cancer metastasis by the ROS/BACH1 axis. Mol. Oncol. 17, 695–709. doi:10.1002/1878-0261.13350
Chen, W.-C., Wei, C.-K., and Lee, J.-C. (2019). MicroRNA-let-7c suppresses hepatitis C virus replication by targeting Bach1 for induction of haem oxygenase-1 expression. J. Viral Hepat. 26 (6), 655–665. doi:10.1111/jvh.13072
Chen, Z., Zhao, Y., Song, C., Li, N., Liao, J., Luo, X., et al. (2020). SanWeiGanJiang San relieves liver injury via Nrf2/Bach1. J. Ethnopharmacol. 251, 112445. doi:10.1016/j.jep.2019.112445
Chiabrando, D., Vinchi, F., Fiorito, V., Mercurio, S., and Tolosano, E. (2014). Heme in pathophysiology: A matter of scavenging, metabolism and trafficking across cell membranes. Front. Pharmacol. 5, 61. doi:10.3389/fphar.2014.00061
Christodoulou, K., Wiskin, A. E., Gibson, J., Tapper, W., Willis, C., Afzal, N. A., et al. (2013). Next generation exome sequencing of paediatric inflammatory bowel disease patients identifies rare and novel variants in candidate genes. Gut 62 (7), 977–984. doi:10.1136/gutjnl-2011-301833
Chu, X., Subramani, K., Thomas, B., Terry, A. V., Fulzele, S., and Raju, R. P. (2022). Juvenile plasma factors improve organ function and survival following injury by promoting antioxidant response. Aging Dis. 13 (2), 568–582. doi:10.14336/AD.2021.0830
Cohen, B., Tempelhof, H., Raz, T., Oren, R., Nicenboim, J., Bochner, F., et al. (2020). BACH family members regulate angiogenesis and lymphangiogenesis by modulating VEGFC expression. Life Sci. Alliance 3 (4), e202000666. doi:10.26508/lsa.202000666
Cui, D., and Ni, C. (2020). LncRNA Lnc712 promotes tumorigenesis in hepatocellular carcinoma by targeting miR-142-3p/bach-1 Axis. Cancer Manag. Res. 12, 11285–11294. doi:10.2147/CMAR.S254950
Cushing, K. C., Du, X., Chen, Y., Stetson, L. C., Kuppa, A., Chen, V. L., et al. (2022). Inflammatory bowel disease risk variants are associated with an increased risk of skin cancer. Inflamm. Bowel Dis. 28 (11), 1667–1676. doi:10.1093/ibd/izab336
Davudian, S., Shajari, N., Kazemi, T., Mansoori, B., Salehi, S., Mohammadi, A., et al. (2016). BACH1 silencing by siRNA inhibits migration of HT-29 colon cancer cells through reduction of metastasis-related genes. Biomed. Pharmacother. = Biomedecine Pharmacother. 84, 191–198. doi:10.1016/j.biopha.2016.09.021
Dickson, K., and Lehmann, C. (2019). Inflammatory response to different toxins in experimental sepsis models. Int. J. Mol. Sci. 20 (18), 4341. doi:10.3390/ijms20184341
Ding, H., Liu, X.-C., Jian-Ming, X., and Qiao, M. (2021). Identification of crucial genes and related transcription factors in ulcerative colitis. Ann. Clin. Laboratory Sci. 51 (2), 245–254.
Ding, R., Wu, W., Sun, Z., and Li, Z. (2020). AMP-activated protein kinase: An attractive therapeutic target for ischemia-reperfusion injury. Eur. J. Pharmacol. 888, 173484. doi:10.1016/j.ejphar.2020.173484
Du, N., Li, K., Wang, Y., Song, B., Zhou, X., and Duan, S. (2022). CircRNA circBACH1 facilitates Hepatitis B virus replication and hepatoma development by regulating the miR-200a-3p/MAP3K2 axis. Histology Histopathol. 37 (9), 863–877. doi:10.14670/HH-18-452
Dun, S., Wang, X., Ma, Y., Hao, J., Jinshen, W., Yongqu, L., et al. (2021). Nrf2-induced miR-23a-27a-24-2 cluster modulates damage repair of intestinal mucosa by targeting the Bach1/HO-1 axis in inflammatory bowel diseases. Free Radic. Biol. Med. 163, 1–9. doi:10.1016/j.freeradbiomed.2020.11.006
Dyson, J. K., Beuers, U., Jones, D. E. J., Lohse, A. W., and Hudson, M. (2018). Primary sclerosing cholangitis. Lancet (London, Engl. 391 (10139), 2547–2559. doi:10.1016/S0140-6736(18)30300-3
El-Deek, H. E. M., Ahmed, A. M., and Mohammed, R. A. A. (2019). Aberration of Nrf2-Bach1 pathway in colorectal carcinoma; role in carcinogenesis and tumor progression. Ann. Diagnostic Pathology 38, 138–144. doi:10.1016/j.anndiagpath.2018.11.003
Eraky, S. M., and Abo El-Magd, N. F. (2020). Omega-3 fatty acids protect against acetaminophen-induced hepatic and renal toxicity in rats through HO-1-Nrf2-BACH1 pathway. Archives Biochem. Biophysics 687, 108387. doi:10.1016/j.abb.2020.108387
European Association for the Study of the LiverClinical Practice Guideline Panel: Chair:Panel membersEASL Governing Board representative: (2019). EASL clinical practice guidelines: Drug-induced liver injury. J. Hepatology 70 (6), 1222–1261. doi:10.1016/j.jhep.2019.02.014
Fader, K. A., Nault, R., Kirby, M. P., Markous, G., Matthews, J., and Zacharewski, T. R. (2017). Convergence of hepcidin deficiency, systemic iron overloading, heme accumulation, and REV-ERBα/β activation in aryl hydrocarbon receptor-elicited hepatotoxicity. Toxicol. Appl. Pharmacol. 321, 1–17. doi:10.1016/j.taap.2017.02.006
Fang, D., and Lu, G. (2020). Expression and role of nuclear receptor-interacting protein 1 (NRIP1) in stomach adenocarcinoma. Ann. Transl. Med. 8 (20), 1293. doi:10.21037/atm-20-6197
Forner, A., Reig, M., and Bruix, J. (2018). Hepatocellular carcinoma. Lancet (London, Engl. 391 (10127), 1301–1314. doi:10.1016/S0140-6736(18)30010-2
Franke, A., McGovern, D. P. B., Barrett, J. C., Wang, K., Radford-Smith, G. L., Ahmad, T., et al. (2010). Genome-wide meta-analysis increases to 71 the number of confirmed Crohn's disease susceptibility loci. Nat. Genet. 42 (12), 1118–1125. doi:10.1038/ng.717
Gao, Z., Shi, Y., Wang, J., Li, W., Bao, Y., Wu, D., et al. (2021). “Long non-coding RNA NEAT1 absorbs let-7 g-5p to induce epithelial-mesenchymal transition of colon cancer cells through upregulating BACH1,” in Digestive and liver disease: Official journal of the Italian society of Gastroenterology and the Italian association for the study of the liver. doi:10.1016/j.dld.2021.04.031
Ghanem, C. I., Pérez, M. J., Manautou, J. E., and Mottino, A. D. (2016). Acetaminophen from liver to brain: New insights into drug pharmacological action and toxicity. Pharmacol. Res. 109, 119–131. doi:10.1016/j.phrs.2016.02.020
Ghaziani, T., Shan, Y., Lambrecht, R. W., Donohue, S. E., Pietschmann, T., Bartenschlager, R., et al. (2006). HCV proteins increase expression of heme oxygenase-1 (HO-1) and decrease expression of Bach1 in human hepatoma cells. J. Hepatology 45 (1), 5–12. doi:10.1016/j.jhep.2005.12.020
Graham, D. B., and Xavier, R. J. (2013). From genetics of inflammatory bowel disease towards mechanistic insights. Trends Immunol. 34 (8), 371–378. doi:10.1016/j.it.2013.04.001
Grant, F. M., Yang, J., Nasrallah, R., Clarke, J., Sadiyah, F., Whiteside, S. K., et al. (2020). BACH2 drives quiescence and maintenance of resting Treg cells to promote homeostasis and cancer immunosuppression. J. Exp. Med. 217 (9), e20190711. doi:10.1084/jem.20190711
Haam, K., Kim, H.-J., Lee, K.-T., Kim, J.-H., Kim, M., Kim, S.-Y., et al. (2014). Epigenetic silencing of BTB and CNC homology 2 and concerted promoter CpG methylation in gastric cancer. Cancer Lett. 351 (2), 206–214. doi:10.1016/j.canlet.2014.05.009
Harusato, A., Naito, Y., Takagi, T., Uchiyama, K., Mizushima, K., Hirai, Y., et al. (2013). BTB and CNC homolog 1 (Bach1) deficiency ameliorates TNBS colitis in mice: Role of M2 macrophages and heme oxygenase-1. Inflamm. Bowel Dis. 19 (4), 740–753. doi:10.1097/MIB.0b013e3182802968
Harusato, A., Naito, Y., Takagi, T., Uchiyama, K., Mizushima, K., Hirai, Y., et al. (2011). Suppression of indomethacin-induced apoptosis in the small intestine due to Bach1 deficiency. Free Radic. Res. 45 (6), 717–727. doi:10.3109/10715762.2011.574287
Harusato, A., Naito, Y., Takagi, T., Yamada, S., Mizushima, K., Hirai, Y., et al. (2009). Inhibition of Bach1 ameliorates indomethacin-induced intestinal injury in mice. J. Physiology Pharmacol. Official J. Pol. Physiological Soc. 60 (7), 149–154.
He, X., Zhang, H., Tao, B., Yang, M., Chen, H., Lu, L., et al. (2019). The A/A genotype of XPO1 rs4430924 is associated with higher risk of antituberculosis drug-induced hepatotoxicity in Chinese patients. J. Clin. Pharmacol. 59 (7), 1014–1021. doi:10.1002/jcph.1398
Hira, S., Tomita, T., Matsui, T., Igarashi, K., and Ikeda-Saito, M. (2007). Bach1, a heme-dependent transcription factor, reveals presence of multiple heme binding sites with distinct coordination structure. IUBMB Life 59 (8-9), 542–551. doi:10.1080/15216540701225941
Hoshino, H., Kobayashi, A., Yoshida, M., Kudo, N., Oyake, T., Motohashi, H., et al. (2000). Oxidative stress abolishes leptomycin B-sensitive nuclear export of transcription repressor Bach2 that counteracts activation of Maf recognition element. J. Biol. Chem. 275 (20), 15370–15376. doi:10.1074/jbc.275.20.15370
Hou, W., Tian, Q., Zheng, J., and Bonkovsky, H. L. (2010). MicroRNA-196 represses Bach1 protein and hepatitis C virus gene expression in human hepatoma cells expressing hepatitis C viral proteins. Hepatol. Baltim. Md 51 (5), 1494–1504. doi:10.1002/hep.23401
Huang, X., Zheng, J., Li, J., Che, X., Tan, W., Tan, W., et al. (2018). Functional role of BTB and CNC Homology 1 gene in pancreatic cancer and its association with survival in patients treated with gemcitabine. Theranostics 8 (12), 3366–3379. doi:10.7150/thno.23978
Igarashi, K., Kurosaki, T., and Roychoudhuri, R. (2017). BACH transcription factors in innate and adaptive immunity. Nat. Rev. Immunol. 17 (7), 437–450. doi:10.1038/nri.2017.26
Igarashi, K., and Watanabe-Matsui, M. (2014). Wearing red for signaling: The heme-bach axis in heme metabolism, oxidative stress response and iron immunology. Tohoku J. Exp. Med. 232 (4), 229–253. doi:10.1620/tjem.232.229
Iida, A., Inagaki, K., Miyazaki, A., Yonemori, F., Ito, E., and Igarashi, K. (2009). Bach1 deficiency ameliorates hepatic injury in a mouse model. Tohoku J. Exp. Med. 217 (3), 223–229. doi:10.1620/tjem.217.223
Inoue, M., Tazuma, S., Kanno, K., Hyogo, H., Igarashi, K., and Chayama, K. (2011). Bach1 gene ablation reduces steatohepatitis in mouse MCD diet model. J. Clin. Biochem. Nutr. 48 (2), 161–166. doi:10.3164/jcbn.09-122GFR
Itoh-Nakadai, A., Hikota, R., Muto, A., Kometani, K., Watanabe-Matsui, M., Sato, Y., et al. (2014). The transcription repressors Bach2 and Bach1 promote B cell development by repressing the myeloid program. Nat. Immunol. 15 (12), 1171–1180. doi:10.1038/ni.3024
Jabłonowska, E., Wójcik, K., Szymańska, B., Omulecka, A., Cwiklińska, H., and Piekarska, A. (2014). Hepatic HMOX1 expression positively correlates with Bach-1 and miR-122 in patients with HCV mono and HIV/HCV coinfection. PloS One 9 (4), e95564. doi:10.1371/journal.pone.0095564
Jiang, L., Jia, M., Wei, X., Guo, J., Hao, S., Mei, A., et al. (2020a). Bach1-induced suppression of angiogenesis is dependent on the BTB domain. EBioMedicine 51, 102617. doi:10.1016/j.ebiom.2019.102617
Jiang, L., Yin, M., Wei, X., Liu, J., Wang, X., Niu, C., et al. (2015). Bach1 represses wnt/β-catenin signaling and angiogenesis. Circulation Res. 117 (4), 364–375. doi:10.1161/CIRCRESAHA.115.306829
Jiang, T.-Y., Pan, Y.-F., Wan, Z.-H., Lin, Y.-K., Zhu, B., Yuan, Z.-G., et al. (2020b). PTEN status determines chemosensitivity to proteasome inhibition in cholangiocarcinoma. Sci. Transl. Med. 12 (562), eaay0152. doi:10.1126/scitranslmed.aay0152
Jiang, X., Cao, M., Wu, J., Wang, X., Zhang, G., Yang, C., et al. (2022). Protections of transcription factor BACH2 and natural product myricetin against pathological cardiac hypertrophy and dysfunction. Front. Physiology 13, 971424. doi:10.3389/fphys.2022.971424
Ka, S.-O., Bang, I. H., Bae, E. J., and Park, B.-H. (2017). Hepatocyte-specific sirtuin 6 deletion predisposes to nonalcoholic steatohepatitis by up-regulation of Bach1, an Nrf2 repressor. FASEB J. Official Publ. Fed. Am. Soc. For Exp. Biol. 31 (9), 3999–4010. doi:10.1096/fj.201700098RR
Kang, X., Feng, Y., Gan, Z., Zeng, S., Guo, X., Chen, X., et al. (2018). NASP antagonize chromatin accessibility through maintaining histone H3K9me1 in hepatocellular carcinoma. Biochimica Biophysica Acta Mol. Basis Dis. 1864 (10), 3438–3448. doi:10.1016/j.bbadis.2018.07.033
Karim, S., Mirza, Z., Chaudhary, A. G., Abuzenadah, A. M., Gari, M., and Al-Qahtani, M. H. (2016). Assessment of radiation induced therapeutic effect and cytotoxicity in cancer patients based on transcriptomic profiling. Int. J. Mol. Sci. 17 (2), 250. doi:10.3390/ijms17020250
Katada, K., Takagi, T., Iida, T., Ueda, T., Mizushima, K., Fukui, A., et al. (2022). Role and potential mechanism of heme oxygenase-1 in intestinal ischemia-reperfusion injury. Antioxidants (Basel, Switz. 11 (3), 559. doi:10.3390/antiox11030559
Kim, J., Ekstrom, T., Yang, W., Donahue, G., Grygoryev, D., Ngo, T. T. M., et al. (2021). Longitudinal analysis of human pancreatic adenocarcinoma development reveals transient gene expression signatures. Mol. Cancer Res. MCR 19 (11), 1854–1867. doi:10.1158/1541-7786.MCR-21-0483
Kuwahara, M., Ise, W., Ochi, M., Suzuki, J., Kometani, K., Maruyama, S., et al. (2016). Bach2-Batf interactions control Th2-type immune response by regulating the IL-4 amplification loop. Nat. Commun. 7, 12596. doi:10.1038/ncomms12596
Laffin, M. R., Fedorak, R. N., Wine, E., Dicken, B., and Madsen, K. L. (2018). A BACH2 gene variant is associated with postoperative recurrence of Crohn's disease. J. Am. Coll. Surg. 226 (5), 902–908. doi:10.1016/j.jamcollsurg.2018.01.052
Lee, J., Yesilkanal, A. E., Wynne, J. P., Frankenberger, C., Liu, J., Yan, J., et al. (2019). Effective breast cancer combination therapy targeting BACH1 and mitochondrial metabolism. Nature 568 (7751), 254–258. doi:10.1038/s41586-019-1005-x
Lee, W. M. (2003). Acute liver failure in the United States. Seminars Liver Dis. 23 (3), 217–226. doi:10.1055/s-2003-42641
Li, J., Tang, Q., Dong, W., and Wang, Y. (2020). CircBACH1/let-7a-5p axis enhances the proliferation and metastasis of colorectal cancer by upregulating CREB5 expression. J. Gastrointest. Oncol. 11 (6), 1186–1199. doi:10.21037/jgo-20-498
Li, S., Bern, M. D., Miao, B., Fan, C., Xing, X., Inoue, T., et al. (2022). The transcription factor Bach2 negatively regulates murine natural killer cell maturation and function. ELife 11, e77294. doi:10.7554/eLife.77294
Li, Y., Rao, X., Tang, P., Chen, S., Guo, Q., Fu, G., et al. (2021). Bach2 deficiency promotes intestinal epithelial regeneration by accelerating DNA repair in intestinal stem cells. Stem Cell Rep. 16 (1), 120–133. doi:10.1016/j.stemcr.2020.12.005
Lignitto, L., LeBoeuf, S. E., Homer, H., Jiang, S., Askenazi, M., Karakousi, T. R., et al. (2019). Nrf2 activation promotes lung cancer metastasis by inhibiting the degradation of Bach1. Cell 178 (2), 316–329. doi:10.1016/j.cell.2019.06.003
Liu, B., Yang, G., Wang, X., Liu, J., Lu, Z., Wang, Q., et al. (2020). CircBACH1 (hsa_circ_0061395) promotes hepatocellular carcinoma growth by regulating p27 repression via HuR. J. Cell. Physiology 235 (10), 6929–6941. doi:10.1002/jcp.29589
Liu, D., Duan, X., Dong, D., Bai, C., Li, X., Sun, G., et al. (2013a). Activation of the Nrf2 pathway by inorganic arsenic in human hepatocytes and the role of transcriptional repressor Bach1. Oxidative Med. Cell. Longev. 2013, 984546. doi:10.1155/2013/984546
Liu, D., Xu, G., Bai, C., Gu, Y., Wang, D., and Li, B. (2021a). Differential effects of arsenic species on Nrf2 and Bach1 nuclear localization in cultured hepatocytes. Toxicol. Appl. Pharmacol. 413, 115404. doi:10.1016/j.taap.2021.115404
Liu, J. Z., Hov, J. R., Folseraas, T., Ellinghaus, E., Rushbrook, S. M., Doncheva, N. T., et al. (2013b). Dense genotyping of immune-related disease regions identifies nine new risk loci for primary sclerosing cholangitis. Nat. Genet. 45 (6), 670–675. doi:10.1038/ng.2616
Liu, L., Matsumoto, M., Matsui-Watanabe, M., Ochiai, K., Callens, B. K. K., Nguyen, L. C., et al. (2022). BACH1 expression is promoted by tank binding kinase 1 (TBK1) in pancreatic cancer cells to increase iron and reduce the expression of E-cadherin. Antioxidants (Basel, Switz. 11 (8), 1460. doi:10.3390/antiox11081460
Liu, Y., Wang, Y., Lu, F., Wang, L., Miao, L., and Wang, X. (2021b). BTB and CNC homology 1 inhibition ameliorates fibrosis and inflammation via blocking ERK pathway in pulmonary fibrosis. Exp. Lung Res. 47 (2), 67–77. doi:10.1080/01902148.2020.1849448
Liu, Z., Wang, J., Chen, H., Wu, Z., Liao, F., Wang, S., et al. (2022b). Uncovering BTB and CNC Homology1 (BACH1) as a novel cancer therapeutic target. Front. Genet. 13, 920911. doi:10.3389/fgene.2022.920911
Lu, L., Liu, G., Lin, C., Li, K., He, T., Zhang, J., et al. (2021). Mitochondrial metabolism targeted nanoplatform for efficient triple-negative breast cancer combination therapy. Adv. Healthc. Mater. 10 (20), e2100978. doi:10.1002/adhm.202100978
Marchese, S., Polo, A., Ariano, A., Velotto, S., Costantini, S., and Severino, L. (2018). Aflatoxin B1 and M1: Biological properties and their involvement in cancer development. Toxins 10 (6), 214. doi:10.3390/toxins10060214
Medrano, L. M., Pascual, V., Bodas, A., López-Palacios, N., Salazar, I., Espino-Paisán, L., et al. (2019). Expression patterns common and unique to ulcerative colitis and celiac disease. Ann. Hum. Genet. 83 (2), 86–94. doi:10.1111/ahg.12293
Mehrab-Mohseni, M., Sendi, H., Steuerwald, N., Ghosh, S., Schrum, L. W., and Bonkovsky, H. L. (2011). Legalon-SIL downregulates HCV core and NS5A in human hepatocytes expressing full-length HCV. World J. Gastroenterology 17 (13), 1694–1700. doi:10.3748/wjg.v17.i13.1694
Moreno, R., Casares, L., Higgins, M., Ali, K. X., Honda, T., Wiel, C., et al. (2022). Biotinylation of an acetylenic tricyclic bis(cyanoenone) lowers its potency as an NRF2 activator while creating a novel activity against BACH1. Free Radic. Biol. Med. 191, 203–211. doi:10.1016/j.freeradbiomed.2022.08.041
Mouri, K., Guo, M. H., de Boer, C. G., Lissner, M. M., Harten, I. A., Newby, G. A., et al. (2022). Prioritization of autoimmune disease-associated genetic variants that perturb regulatory element activity in T cells. Nat. Genet. 54 (5), 603–612. doi:10.1038/s41588-022-01056-5
Muchova, L., Vanova, K., Suk, J., Micuda, S., Dolezelova, E., Fuksa, L., et al. (2015). Protective effect of heme oxygenase induction in ethinylestradiol-induced cholestasis. J. Cell. Mol. Med. 19 (5), 924–933. doi:10.1111/jcmm.12401
Muto, A., Ochiai, K., Kimura, Y., Itoh-Nakadai, A., Calame, K. L., Ikebe, D., et al. (2010). Bach2 represses plasma cell gene regulatory network in B cells to promote antibody class switch. EMBO J. 29 (23), 4048–4061. doi:10.1038/emboj.2010.257
Nanashima, K., Mawatari, T., Tahara, N., Higuchi, N., Nakaura, A., Inamine, T., et al. (2012). Genetic variants in antioxidant pathway: Risk factors for hepatotoxicity in tuberculosis patients. Tuberc. Edinb. Scotl. 92 (3), 253–259. doi:10.1016/j.tube.2011.12.004
Niu, C., Wang, S., Guo, J., Wei, X., Jia, M., Chen, Z., et al. (2021). BACH1 recruits NANOG and histone H3 lysine 4 methyltransferase MLL/SET1 complexes to regulate enhancer-promoter activity and maintains pluripotency. Nucleic Acids Res. 49 (4), 1972–1986. doi:10.1093/nar/gkab034
Ogawa, K., Sun, J., Taketani, S., Nakajima, O., Nishitani, C., Sassa, S., et al. (2001). Heme mediates derepression of Maf recognition element through direct binding to transcription repressor Bach1. EMBO J. 20 (11), 2835–2843. doi:10.1093/emboj/20.11.2835
Oldfield, C. J., and Dunker, A. K. (2014). Intrinsically disordered proteins and intrinsically disordered protein regions. Annu. Rev. Biochem. 83, 553–584. doi:10.1146/annurev-biochem-072711-164947
Oyake, T., Itoh, K., Motohashi, H., Hayashi, N., Hoshino, H., Nishizawa, M., et al. (1996). Bach proteins belong to a novel family of BTB-basic leucine zipper transcription factors that interact with MafK and regulate transcription through the NF-E2 site. Mol. Cell. Biol. 16 (11), 6083–6095. doi:10.1128/mcb.16.11.6083
Padilla, J., and Lee, J. (2021). A novel therapeutic target, BACH1, regulates cancer metabolism. Cells 10 (3), 634. doi:10.3390/cells10030634
Palma-Lara, I., Martínez-Castillo, M., Quintana-Pérez, J. C., Arellano-Mendoza, M. G., Tamay-Cach, F., Valenzuela-Limón, O. L., et al. (2020). Arsenic exposure: A public health problem leading to several cancers. Regul. Toxicol. Pharmacol. RTP 110, 104539. doi:10.1016/j.yrtph.2019.104539
Perez-Torrado, R., Yamada, D., and Defossez, P.-A. (2006). Born to bind: The BTB protein-protein interaction domain. BioEssays News Rev. Mol. Cell. Dev. Biol. 28 (12), 1194–1202. doi:10.1002/bies.20500
Pinto-Sanchez, M. I., Silvester, J. A., Lebwohl, B., Leffler, D. A., Anderson, R. P., Therrien, A., et al. (2021). Society for the Study of Celiac Disease position statement on gaps and opportunities in coeliac disease. Nat. Rev. Gastroenterology Hepatology 18 (12), 875–884. doi:10.1038/s41575-021-00511-8
Polaris Observatory HCV Collaborators (2022). Global change in hepatitis C virus prevalence and cascade of care between 2015 and 2020: A modelling study. Lancet. Gastroenterology Hepatology 7 (5), 396–415. doi:10.1016/S2468-1253(21)00472-6
Povoleri, G. A. M., Nova-Lamperti, E., Scottà, C., Fanelli, G., Chen, Y.-C., Becker, P. D., et al. (2018). Human retinoic acid-regulated CD161+ regulatory T cells support wound repair in intestinal mucosa. Nat. Immunol. 19 (12), 1403–1414. doi:10.1038/s41590-018-0230-z
Pradhan, P., Vijayan, V., Cirksena, K., Buettner, F. F. R., Igarashi, K., Motterlini, R., et al. (2022). Genetic BACH1 deficiency alters mitochondrial function and increases NLRP3 inflammasome activation in mouse macrophages. Redox Biol. 51, 102265. doi:10.1016/j.redox.2022.102265
Quinn, E. M., Coleman, C., Molloy, B., Dominguez Castro, P., Cormican, P., Trimble, V., et al. (2015). Transcriptome analysis of CD4+ T cells in coeliac disease reveals imprint of BACH2 and IFNγ regulation. PloS One 10 (10), e0140049. doi:10.1371/journal.pone.0140049
Rodrigues, P. M., Olaizola, P., Paiva, N. A., Olaizola, I., Agirre-Lizaso, A., Landa, A., et al. (2021). Pathogenesis of cholangiocarcinoma. Annu. Rev. Pathology 16, 433–463. doi:10.1146/annurev-pathol-030220-020455
Romani, P., Valcarcel-Jimenez, L., Frezza, C., and Dupont, S. (2021). Crosstalk between mechanotransduction and metabolism. Nat. Rev. Mol. Cell Biol. 22 (1), 22–38. doi:10.1038/s41580-020-00306-w
Roychoudhuri, R., Hirahara, K., Mousavi, K., Clever, D., Klebanoff, C. A., Bonelli, M., et al. (2013). BACH2 represses effector programs to stabilize T(reg)-mediated immune homeostasis. Nature 498 (7455), 506–510. doi:10.1038/nature12199
Ruginis, T., Taglia, L., Matusiak, D., Lee, B.-S., and Benya, R. V. (2006). Consequence of gastrin-releasing peptide receptor activation in a human colon cancer cell line: A proteomic approach. J. Proteome Res. 5 (6), 1460–1468. doi:10.1021/pr060005g
Salas-Silva, S., Simoni-Nieves, A., Lopez-Ramirez, J., Bucio, L., Gómez-Quiroz, L. E., Gutiérrez-Ruiz, M. C., et al. (2019). Cholangiocyte death in ductopenic cholestatic cholangiopathies: Mechanistic basis and emerging therapeutic strategies. Life Sci. 218, 324–339. doi:10.1016/j.lfs.2018.12.044
Sasikala, M., Ravikanth, V. V., Murali Manohar, K., Deshpande, N., Singh, S., Pavan Kumar, P., et al. (2018). Bach2 repression mediates Th17 cell induced inflammation and associates with clinical features of advanced disease in chronic pancreatitis. United Eur. Gastroenterology J. 6 (2), 272–282. doi:10.1177/2050640617716596
Sato, M., Matsumoto, M., Saiki, Y., Alam, M., Nishizawa, H., Rokugo, M., et al. (2020). BACH1 promotes pancreatic cancer metastasis by repressing epithelial genes and enhancing epithelial-mesenchymal transition. Cancer Res. 80 (6), 1279–1292. doi:10.1158/0008-5472.CAN-18-4099
Scarpignato, C., and Hunt, R. H. (2010). Nonsteroidal antiinflammatory drug-related injury to the gastrointestinal tract: Clinical picture, pathogenesis, and prevention. Gastroenterology Clin. N. Am. 39 (3), 433–464. doi:10.1016/j.gtc.2010.08.010
Segawa, K., Igarashi, K., and Murayama, K. (2022). The Cys-Pro motifs in the intrinsically disordered regions of the transcription factor BACH1 mediate distinct and overlapping functions upon heme binding. FEBS Lett. 596 (12), 1576–1585. doi:10.1002/1873-3468.14338
Segawa, K., Watanabe-Matsui, M., Matsui, T., Igarashi, K., and Murayama, K. (2019a). Functional heme binding to the intrinsically disordered C-terminal region of Bach1, a transcriptional repressor. Tohoku J. Exp. Med. 247 (3), 153–159. doi:10.1620/tjem.247.153
Segawa, K., Watanabe-Matsui, M., Tsuda, K., Matsui, T., Shirouzu, M., Igarashi, K., et al. (2019b). Biophysical characterization of heme binding to the intrinsically disordered region of Bach1. Eur. Biophysics J. EBJ 48 (4), 361–369. doi:10.1007/s00249-019-01364-5
Shan, Y., Zheng, J., Lambrecht, R. W., and Bonkovsky, H. L. (2007). Reciprocal effects of micro-RNA-122 on expression of heme oxygenase-1 and hepatitis C virus genes in human hepatocytes. Gastroenterology 133 (4), 1166–1174. doi:10.1053/j.gastro.2007.08.002
Smyth, E. C., Nilsson, M., Grabsch, H. I., van Grieken, N. C., and Lordick, F. (2020). Gastric cancer. Lancet (London, Engl. 396 (10251), 635–648. doi:10.1016/S0140-6736(20)31288-5
Song, Y., Washington, M. K., and Crawford, H. C. (2010). Loss of FOXA1/2 is essential for the epithelial-to-mesenchymal transition in pancreatic cancer. Cancer Res. 70 (5), 2115–2125. doi:10.1158/0008-5472.CAN-09-2979
Stogios, P. J., Downs, G. S., Jauhal, J. J. S., Nandra, S. K., and Privé, G. G. (2005). Sequence and structural analysis of BTB domain proteins. Genome Biol. 6 (10), R82. doi:10.1186/gb-2005-6-10-r82
Suenaga, T., Watanabe-Matsui, M., Uejima, T., Shima, H., Matsui, T., Ikeda-Saito, M., et al. (2016). Charge-state-distribution analysis of Bach2 intrinsically disordered heme binding region. J. Biochem. 160 (5), 291–298. doi:10.1093/jb/mvw035
Sun, J., Hoshino, H., Takaku, K., Nakajima, O., Muto, A., Suzuki, H., et al. (2002). Hemoprotein Bach1 regulates enhancer availability of heme oxygenase-1 gene. EMBO J. 21 (19), 5216–5224. doi:10.1093/emboj/cdf516
Sun, J., Zhang, J., Wang, X., Ji, F., Ronco, C., Tian, J., et al. (2020a). Gut-liver crosstalk in sepsis-induced liver injury. Crit. Care (London, Engl. 24 (1), 614. doi:10.1186/s13054-020-03327-1
Sun, X., Qian, Y., Wang, X., Cao, R., Zhang, J., Chen, W., et al. (2020b). LncRNA TRG-AS1 stimulates hepatocellular carcinoma progression by sponging miR-4500 to modulate BACH1. Cancer Cell Int. 20, 367. doi:10.1186/s12935-020-01440-3
Sun, X., Zhu, H., Cao, R., Zhang, J., and Wang, X. (2021). BACH1 is transcriptionally inhibited by TET1 in hepatocellular carcinoma in a microRNA-34a-dependent manner to regulate autophagy and inflammation. Pharmacol. Res. 169, 105611. doi:10.1016/j.phrs.2021.105611
Sung, H., Ferlay, J., Siegel, R. L., Laversanne, M., Soerjomataram, I., Jemal, A., et al. (2021). Global cancer statistics 2020: GLOBOCAN estimates of incidence and mortality worldwide for 36 cancers in 185 countries. CA a Cancer J. For Clin. 71 (3), 209–249. doi:10.3322/caac.21660
Suzuki, H., Tashiro, S., Hira, S., Sun, J., Yamazaki, C., Zenke, Y., et al. (2004). Heme regulates gene expression by triggering Crm1-dependent nuclear export of Bach1. EMBO J. 23 (13), 2544–2553. doi:10.1038/sj.emboj.7600248
Suzuki, H., Tashiro, S., Sun, J., Doi, H., Satomi, S., and Igarashi, K. (2003). Cadmium induces nuclear export of Bach1, a transcriptional repressor of heme oxygenase-1 gene. J. Biol. Chem. 278 (49), 49246–49253. doi:10.1074/jbc.M306764200
Takagi, T., Naito, Y., Mizushima, K., Hirai, Y., Harusato, A., Okayama, T., et al. (2018). Heme oxygenase-1 prevents murine intestinal inflammation. J. Clin. Biochem. Nutr. 63 (3), 169–174. doi:10.3164/jcbn.17-133
Tan, M.-K. M., Lim, H.-J., Bennett, E. J., Shi, Y., and Harper, J. W. (2013). Parallel SCF adaptor capture proteomics reveals a role for SCFFBXL17 in NRF2 activation via BACH1 repressor turnover. Mol. Cell 52 (1), 9–24. doi:10.1016/j.molcel.2013.08.018
Tanaka, H., Muto, A., Shima, H., Katoh, Y., Sax, N., Tajima, S., et al. (2016). Epigenetic regulation of the blimp-1 gene (Prdm1) in B cells involves Bach2 and histone deacetylase 3. J. Biol. Chem. 291 (12), 6316–6330. doi:10.1074/jbc.M116.713842
Tanioka, N., Shimizu, H., Omori, E., Takahashi, T., Yamaoka, M., and Morimatsu, H. (2021). Role of the transcription factor BTB and CNC homology 1 in a rat model of acute liver injury induced by experimental endotoxemia. Acta Medica Okayama 75 (3), 363–372. doi:10.18926/AMO/62232
Tian, R., Hu, J., Ma, X., Liang, L., and Guo, S. (2020). Immune-related gene signature predicts overall survival of gastric cancer patients with varying microsatellite instability status. Aging 13 (2), 2418–2435. doi:10.18632/aging.202271
Unsal, V., Cicek, M., and Sabancilar, İ. (2021). Toxicity of carbon tetrachloride, free radicals and role of antioxidants. Rev. Environ. Health 36 (2), 279–295. doi:10.1515/reveh-2020-0048
van der Lee, R., Buljan, M., Lang, B., Weatheritt, R. J., Daughdrill, G. W., Dunker, A. K., et al. (2014). Classification of intrinsically disordered regions and proteins. Chem. Rev. 114 (13), 6589–6631. doi:10.1021/cr400525m
Wada, S., Kanzaki, H., Katsumata, Y., Yamaguchi, Y., Narimiya, T., Attucks, O. C., et al. (2020). Bach1 inhibition suppresses osteoclastogenesis via reduction of the signaling via reactive oxygen species by reinforced antioxidation. Front. Cell Dev. Biol. 8, 740. doi:10.3389/fcell.2020.00740
Wang, D., Jiang, Y., Sun-Waterhouse, D.-X., Zhai, H., Guan, H., Rong, X., et al. (2020). MicroRNA-based regulatory mechanisms underlying the synergistic antioxidant action of quercetin and catechin in H2O2-stimulated HepG2 cells: Roles of BACH1 in Nrf2-dependent pathways. Free Radic. Biol. Med. 153, 122–131. doi:10.1016/j.freeradbiomed.2020.04.018
Watanabe-Matsui, M., Matsumoto, T., Matsui, T., Ikeda-Saito, M., Muto, A., Murayama, K., et al. (2015). Heme binds to an intrinsically disordered region of Bach2 and alters its conformation. Archives Biochem. Biophysics 565, 25–31. doi:10.1016/j.abb.2014.11.005
Watanabe-Matsui, M., Muto, A., Matsui, T., Itoh-Nakadai, A., Nakajima, O., Murayama, K., et al. (2011). Heme regulates B-cell differentiation, antibody class switch, and heme oxygenase-1 expression in B cells as a ligand of Bach2. Blood 117 (20), 5438–5448. doi:10.1182/blood-2010-07-296483
Wood, L. D., Canto, M. I., Jaffee, E. M., and Simeone, D. M. (2022). Pancreatic cancer: Pathogenesis, screening, diagnosis, and treatment. Gastroenterology 163 (2), 386–402.e1. doi:10.1053/j.gastro.2022.03.056
Wu, Y., Liang, Y., Li, M., and Zhang, H. (2022). Knockdown of long non-coding RNA SNHG8 suppresses the progression of esophageal cancer by regulating miR-1270/BACH1 axis. Bioengineered 13 (2), 3384–3394. doi:10.1080/21655979.2021.2021064
Wuestenberg, A., Kah, J., Singethan, K., Sirma, H., Keller, A. D., Rosal, S. R. P., et al. (2014). Matrix conditions and KLF2-dependent induction of heme oxygenase-1 modulate inhibition of HCV replication by fluvastatin. PloS One 9 (5), e96533. doi:10.1371/journal.pone.0096533
Xie, M., Sun, M., Ji, X., Li, D., Chen, X., Zhang, B., et al. (2022). Overexpression of BACH1 mediated by IGF2 facilitates hepatocellular carcinoma growth and metastasis via IGF1R and PTK2. Theranostics 12 (3), 1097–1116. doi:10.7150/thno.65775
Xie, X., Tian, L., Zhao, Y., Liu, F., Dai, S., Gu, X., et al. (2023). BACH1-induced ferroptosis drives lymphatic metastasis by repressing the biosynthesis of monounsaturated fatty acids. Cell Death Dis. 14 (1), 48. doi:10.1038/s41419-023-05571-z
Xing, Y., Li, J., Li, S.-P., Xi, J., Ma, N., Liu, L., et al. (2018). MiR-27a-5p regulates apoptosis of liver ischemia-reperfusion injury in mice by targeting Bach1. J. Cell. Biochem. 119 (12), 10376–10383. doi:10.1002/jcb.27383
Xu, I. M.-J., Lai, R. K.-H., Lin, S.-H., Tse, A. P.-W., Chiu, D. K.-C., Koh, H.-Y., et al. (2016). Transketolase counteracts oxidative stress to drive cancer development. Proc. Natl. Acad. Sci. U. S. A. 113 (6), E725–E734. doi:10.1073/pnas.1508779113
Yang, B., Su, K., Sha, G., Bai, Q., Sun, G., Chen, H., et al. (2022). LINC00665 interacts with BACH1 to activate Wnt1 and mediates the M2 polarization of tumor-associated macrophages in GC. Mol. Immunol. 146, 1–8. doi:10.1016/j.molimm.2022.03.120
Yao, C., Lou, G., Sun, H.-W., Zhu, Z., Sun, Y., Chen, Z., et al. (2021). BACH2 enforces the transcriptional and epigenetic programs of stem-like CD8+ T cells. Nat. Immunol. 22 (3), 370–380. doi:10.1038/s41590-021-00868-7
Yoshida, C., Tokumasu, F., Hohmura, K. I., Bungert, J., Hayashi, N., Nagasawa, T., et al. (1999). Long range interaction of cis-DNA elements mediated by architectural transcription factor Bach1. Genes Cells Devoted Mol. Cell. Mech. 4 (11), 643–655. doi:10.1046/j.1365-2443.1999.00291.x
Younossi, Z., Tacke, F., Arrese, M., Chander Sharma, B., Mostafa, I., Bugianesi, E., et al. (2019). Global perspectives on nonalcoholic fatty liver disease and nonalcoholic steatohepatitis. Hepatol. Baltim. Md 69 (6), 2672–2682. doi:10.1002/hep.30251
Yu, S., Zhai, J., Yu, J., Yang, Q., and Yang, J. (2020). Downregulation of BACH1 protects AGAINST cerebral ischemia/reperfusion injury through the functions of HO-1 and NQO1. Neuroscience 436, 154–166. doi:10.1016/j.neuroscience.2020.04.014
Zenke-Kawasaki, Y., Dohi, Y., Katoh, Y., Ikura, T., Ikura, M., Asahara, T., et al. (2007). Heme induces ubiquitination and degradation of the transcription factor Bach1. Mol. Cell. Biol. 27 (19), 6962–6971. doi:10.1128/MCB.02415-06
Zhang, H., Qian, H., Tao, B., Yang, M., Gong, J., Yi, H., et al. (2018a). The association between BACH1 polymorphisms and anti-tuberculosis drug-induced hepatotoxicity in a Chinese cohort. Infect. Genet. Evol. J. Mol. Epidemiol. Evol. Genet. Infect. Dis. 66, 217–221. doi:10.1016/j.meegid.2018.10.006
Zhang, J., Hu, S., Zhao, C., Zhou, Y., Zhang, L., Liu, H., et al. (2022). Genome-scale CRISPR knockout screening identifies BACH1 as a key regulator of aflatoxin B1-induced oxidative damage. Antioxidants (Basel, Switz. 11 (9), 1787. doi:10.3390/antiox11091787
Zhang, X., Guo, J., Wei, X., Niu, C., Jia, M., Li, Q., et al. (2018b). Bach1: Function, regulation, and involvement in disease. Oxidative Med. Cell. Longev. 2018, 1347969. doi:10.1155/2018/1347969
Zhao, L., Kang, M., Liu, X., Wang, Z., Wang, Y., Chen, H., et al. (2022). UBR7 inhibits HCC tumorigenesis by targeting Keap1/Nrf2/Bach1/HK2 and glycolysis. J. Exp. Clin. Cancer Res. CR 41 (1), 330. doi:10.1186/s13046-022-02528-6
Zhao, Y., Gao, J., Xie, X., Nan, P., Liu, F., Sun, Y., et al. (2021). BACH1 promotes the progression of esophageal squamous cell carcinoma by inducing the epithelial-mesenchymal transition and angiogenesis. Cancer Med. 10 (10), 3413–3426. doi:10.1002/cam4.3884
Zhou, Y., Wu, H., Zhao, M., Chang, C., and Lu, Q. (2016). The bach family of transcription factors: A comprehensive review. Clin. Rev. Allergy & Immunol. 50 (3), 345–356. doi:10.1007/s12016-016-8538-7
Zhu, G.-D., Liu, F., OuYang, S., Zhou, R., Jiang, F.-N., Zhang, B., et al. (2018). BACH1 promotes the progression of human colorectal cancer through BACH1/CXCR4 pathway. Biochem. Biophysical Res. Commun. 499 (2), 120–127. doi:10.1016/j.bbrc.2018.02.178
Keywords: BACH1, BACH2, cancer, digestive system diseases, transcription factor
Citation: Song Q, Mao X, Jing M, Fu Y and Yan W (2023) Pathophysiological role of BACH transcription factors in digestive system diseases. Front. Physiol. 14:1121353. doi: 10.3389/fphys.2023.1121353
Received: 11 December 2022; Accepted: 26 April 2023;
Published: 09 May 2023.
Edited by:
Catia Sternini, University of California, Los Angeles, United StatesReviewed by:
Sifeng Chen, Fudan University, ChinaNirmala Mavila, Cedars Sinai Medical Center, United States
Copyright © 2023 Song, Mao, Jing, Fu and Yan. This is an open-access article distributed under the terms of the Creative Commons Attribution License (CC BY). The use, distribution or reproduction in other forums is permitted, provided the original author(s) and the copyright owner(s) are credited and that the original publication in this journal is cited, in accordance with accepted academic practice. No use, distribution or reproduction is permitted which does not comply with these terms.
*Correspondence: Yu Fu, ZnV0dXJleXVAaHVzdC5lZHUuY24=; Wei Yan, eWFud2VpQHRqaC50am11LmVkdS5jbg==