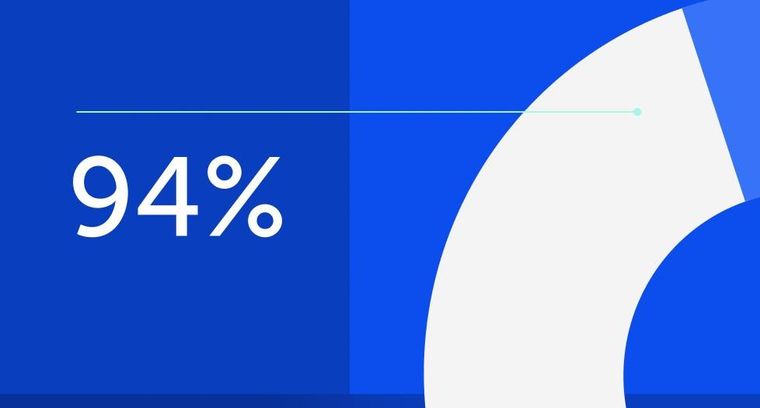
94% of researchers rate our articles as excellent or good
Learn more about the work of our research integrity team to safeguard the quality of each article we publish.
Find out more
ORIGINAL RESEARCH article
Front. Physiol., 02 February 2023
Sec. Membrane Physiology and Membrane Biophysics
Volume 14 - 2023 | https://doi.org/10.3389/fphys.2023.1117352
This article is part of the Research TopicThe Calcium-Sensing Receptor: from Physiology to PharmacologyView all 7 articles
Whether GPCRs support the sensing of temperature as well as other chemical and physical modalities is not well understood.
Introduction: Extracellular Ca2+ concentration (Ca2+o) modulates core body temperature and the firing rates of temperature-sensitive CNS neurons, and hypocalcemia provokes childhood seizures. However, it is not known whether these phenomena are mediated by Ca2+o-sensing GPCRs, including the calcium-sensing receptor (CaSR). In favor of the hypothesis, CaSRs are expressed in hypothalamic regions that support core temperature regulation, and autosomal dominant hypocalcemia, due to CaSR activating mutations, is associated with childhood seizures.
Methods: Herein, we tested whether CaSR-dependent signaling is temperature sensitive using an established model system, CaSR-expressing HEK-293 cells.
Results: We found that the frequency of Ca2+o-induced Ca2+i oscillations but not the integrated response was linearly dependent on temperature in a pathophysiologically relevant range. Chimeric receptor analysis showed that the receptor’s C-terminus is required for temperature-dependent modulation and experiments with the PKC inhibitor GF109203X and CaSR mutants T888A and T888M, which eliminate a key phosphorylation site, demonstrated the importance of repetitive phosphorylation and dephosphorylation.
Discussion and Conclusion: CaSRs mediate temperature-sensing and the mechanism, dependent upon repetitive phosphorylation and dephosphorylation, suggests that GPCRs more generally contribute to temperature-sensing.
The calcium-sensing receptor (CaSR) is a class C G protein-coupled receptor (GPCR) that acts as a master regulator of calcium homeostasis with key actions in parathyroid, kidney and bone (Brown and MacLeod, 2001). It is also expressed widely in non-calcitropic tissues (i.e., tissues not directly involved in calcium metabolism) (Hannan et al., 2018) and, depending on the compartment (Conigrave et al., 2000a), acts as a multi-modal, multi-metabolic sensor that responds to endogenous ionic, nutrient and other chemical activators including Mg2+ (Brown et al., 1993), l-amino acids (Conigrave et al., 2000b; Conigrave et al., 2004; Liou et al., 2011; Wang et al., 2011) and glutathione and analogs (Wang et al., 2006; Broadhead et al., 2011), inhibitors including inorganic phosphate ions (Centeno et al., 2019), and key physicochemical modalities including ionic strength and pH (Quinn et al., 1998; Quinn et al., 2004; Campion et al., 2015). It is not known, however, whether the CaSR might also sense and respond to changes in temperature in the pathophysiological range as suggested by its expression in anterior preoptic neurons of the hypothalamus (Yano et al., 2004) and by the observation that activating mutations of the CaSR in type-I Autosomal Dominant Hypocalcemia (ADH) are associated with childhood febrile convulsions (Papadimitriou et al., 2001).
In response to small, physiologically relevant increases in Ca2+o concentration, the CaSR mediates oscillations in cytoplasmic free Ca2+ (Ca2+i) in various cell types including transfected HEK-293 cells that translate CaSR mRNA (Breitwieser and Gama, 2001), parathyroid cells (Miki et al., 1995; Ridefelt et al., 1995), and colonic epithelial cells (Rey et al., 2010). In CaSR-expressing HEK-293 cells, elevated Ca2+o induces low frequency (1—4 min−1) Ca2+i oscillations (Young and Rozengurt, 2002) (review: (Breitwieser, 2006)) that are supported by the cyclical phosphorylation and dephosphorylation of the CaSR’s primary protein kinase-C (PKC) site at Thr-888 (T888), in its intracellular C-terminus (Bai et al., 1998; Jiang et al., 2002; McCormick et al., 2010). HEK-293 cells transfected with either of the CaSR mutants T888A or T888M, which eliminate the PKC dependent phosphorylation site, exhibit enhanced Ca2+o sensitivity and support sustained increases in Ca2+i rather than Ca2+i oscillations (Davies et al., 2007; Lazarus et al., 2011).
Previously it was reported that the frequency of Ca2+i oscillations was sensitive to a large change in temperature in CaSR-expressing HEK-293 cells (Breitwieser and Gama, 2001; Young et al., 2002); however, the possibility that the CaSR might act as a temperature-sensor has not been formally investigated. We considered whether the CaSR might exhibit temperature-sensitive properties that arise from the dual control of T888 phosphorylation via PKC and protein phosphatase activity, both of which are temperature sensitive (Sugiura et al., 2002; Kim et al., 2005; Chemin et al., 2007; Sohn et al., 2007). Thus, in the present study, we investigated whether the CaSR has temperature-sensing properties, as revealed by changes in the frequency and/or amplitude of Ca2+o-induced Ca2+i oscillations in CaSR-expressing HEK-293 cells. We observed temperature-sensitive modulation of the frequency but not the amplitude of CaSR-mediated Ca2+i oscillations together with roles for PKC and CaSR C-terminal residue T888 in supporting the mechanism.
The wild-type (WT) human CaSR cDNA was cloned between the Kpn I and Xba I sites of pcDNA3.1 (+). All mutants were generated in pcDNA3.1(+)-WT CaSR. The Quikchange II site-directed mutagenesis protocol was used to introduce the point mutations T888A and T888M, as described previously (Lazarus et al., 2011): briefly, pairs of complementary or overlapping primers (30–40 bases) were designed to encode the required mutation with flanking wild-type sequences of ∼15–20 bases. The template DNA was amplified for 18 cycles with Pfu Ultra II HS DNA polymerase (Agilent Technologies, USA). Following digestion of parent DNA with Dpn I, amplified DNA was transformed into DH5α Escherichia coli cells.
The hCaSR/rmGluR1/rmGluR1 chimeric receptor construct was described previously (Mun et al., 2004). It encodes a class C GPCR protein that contains the hCaSR VFT domain (residues 1–540) appended to the rat mGluR1alpha Cysteine-rich domain, heptahelical domain, and intracellular C-terminus (residues 524–1199).
The identities of all mutants were confirmed by DNA sequencing (Australian Genome Research Facility, Sydney, NSW, Australia).
HEK-293 cells stably expressing the human CaSR (HEK-CaSR cells) or the hCaSR/rmGluR1/rmGluR1 (HEK-C/G/G) chimeric receptor were cultured in DMEM (Invitrogen, USA) supplemented with 10% fetal bovine serum (FBS) as described previously (Mun et al., 2004; Szekely et al., 2009). Cells were grown on 15-mm diameter glass coverslips in 24-well plates for microfluorimetry experiments.
For experiments with transiently expressing CaSR constructs, when the cells had reached 85%–95% confluency they were transfected with XtremeGENE HP transfection reagent (Roche, Germany): briefly, 0.5 μg samples of WT or mutant DNA and 3 μL of transfection reagent were diluted with 100 μL of DMEM and allowed to complex at RT for 15 min. The transfection solution was added to the cell cultures to a final concentration of 9.1% (v/v).
HEK-CaSR, HEK-C/G/G or transiently transfected HEK-293 cells were cultured on coverslips in 24-well plates and then loaded in the dark with Fura-2AM (5 μM) in physiological saline solution (PSS): 125 mM NaCl, 4 mM KCl, 0.1% (w/v) D-glucose, 1 mM MgCl2, 20 mM HEPES-NaOH (pH 7.45) that contained 1 mM CaCl2, 0.8 mM NaH2PO4, and 1 mg ml−1 BSA for 2 h at 37°C. The cells were stored in loading solution prior to experiments. Fura-2-loaded HEK-CaSR cells were then placed in a perifusion chamber in the light path of a Zeiss Axiovert 200M fluorescence microscope (×63 objective) and perifused with PSS containing various concentrations of Ca2+o. The PKC inhibitor GF109203X (Sigma-Aldrich) was used at a final concentration of 250 nM.
Fura-2-loaded HEK-CaSR cells were excited using a Lambda DG-4 150 Watt xenon light source (Sutter Instruments), with alternating wavelengths of 340 and 380 nm at 0.5s intervals, and the fluorescent cells were imaged at 510 nm. Each data set consisted of 10 regions of interest each containing an individual cell and digital images were captured using an AxioCam camera controlled by Stallion SB.4.1.0 PC software (Carl Zeiss).
The temperature in the perifusion chamber of the fluorescent microscope and therefore of fura-2-loaded cells was set by 1) a recirculating heat exchange system that delivered solutions of the desired temperature into the chamber via a peristaltic pump (Gilson Minipuls 2) and; 2) a Warner TC-344B Dual Channel Heat Controller that controlled the temperature of the microscope stage. To prevent heat losses, the inlet tubing to the microscope chamber was passed through a wide-bore 1.5 cm diameter tube containing water of the desired temperature that re-circulated via the external circuit of a thermostatically controlled water-bath. Using this system, the required perifusion solution temperatures were achieved by adjusting the demand temperatures of the water bath and the microscope stage as demonstrated by the temperatures measured in the effluent solutions (Supplementary Material). When the set temperature was adjusted, re-equilibration times of 1–2 min were observed.
Human parathyroid cells were prepared by collagenase digestion of surgical specimens of normal parathyroid tissue as described previously (Conigrave et al., 2004; McCormick et al., 2010) according to ethics protocol 11_067 (Human Research Ethics Committee, St Vincent’s Health Network, Darlinghurst NSW 2010). Tissue samples were collected from the Mater Hospital (North Sydney) into MEM (Gibco #11575032; Ca2+ 1.2 mM) and transported to the laboratory on ice. After isolation and washing to remove collagenase by centrifugation (110 X g, 2 min), aliquots of cells (40,000—200,000) were loaded into columns to lie above a layer of Biogel P4 (Biorad #1504124) and below a layer of Sephadex G-25 medium (Sigma-Aldrich #G-25150) as described previously and perifused with physiological saline solution (NaCl 125 mM, KCl 4 mM, HEPES 20 mM, Na2HPO4 0.8 mM, glucose 1.0 g L−1, MgCl2 1.0 mM, pH 7.4) that contained bovine serum albumin 1 mg/ml (Sigma-Aldrich #A3059; protease-free) and various Ca2+ concentrations at 37°C as required. Perifusion columns were housed in metal holders that enabled full immersion and connections to be established upstream to the perifusion solutions and downstream to a peristaltic pump. The metal holders also protected the columns during transfer between waterbaths (see below). Samples were collected on ice and transferred to dry ice prior to storage at -80°C. Analysis for PTH was subsequently performed on a Siemens Immulite autoanalyzer (Royal Prince Alfred Hospital, NSW Health Pathology). To permit studies of the effect of temperature on Ca2+o-regulated PTH secretion, two water baths (one equilibrated at 35°C and one equilibrated at 38°C) were placed in close proximity and identical pre-equilibrated PSS-albumin solutions (i.e., containing the same Ca2+o concentrations) were immersed in both baths to allow prompt temperature exchange (∼30 s) when required. To enable temperature changeovers, the perifusion pump was stopped briefly (20–30s) and perifusion columns were transferred to the neighboring bath at the new temperature (38°C or back to 35°C) where its upstream connections were established to the new temperature pre-equilibrated solution, and the pump restarted.
Single-cell Ca2+ i mobilization data consisted of excitation ratios (F340/F380) plotted against experiment time (min). Where indicated, Ca2+ i responses from single cells were integrated with respect to time according to the ‘trapezoidal rule’, using the following equation:
where Δx is time interval between measurements (0.5 s) and y is F340/F380, to provide results in the form of integrated response units (IRUs) that were corrected for the baseline observed at control Ca2+o (0.5 mM), as described previously (Brennan et al., 2015).
The fluorescence records from individual cells were processed to estimate the Ca2+i oscillation frequency using wavelet analysis in a Mathematica 9.0 script, as described previously (Szekely et al., 2009; Brennan et al., 2015). Frequency estimates from individual cells are expressed as means ± SEM.
Statistical analyses were performed in GraphPad Prism version 8.0 using: Student’s unpaired t-test for direct comparisons between individual groups; one-way ANOVA with Dunnett’s post-test for comparisons between multiple groups, Linear Regression Analysis to evaluate the nature of the relationship between temperature and Ca2+i oscillation frequency, and Analysis of Co-Variance (ANCOVA) for comparisons between regression lines.
In pilot experiments fura-2 loaded HEK-CaSR cells were perifused with PSS in the presence of a control sub-physiological temperature (34 °C) at low-baseline Ca2+o (0.5 mM) and then exposed to an abrupt step-wise increase in Ca2+o to a near-maximally effective Ca2+o concentration, 5.0 mM. Once stable Ca2+i oscillations had been established, the temperature was lowered to 14°C for 10–15 min and subsequently restored to the baseline temperature (34°C). At baseline temperature (34°C) and at 5.0 mM Ca2+o, the Ca2+o-induced Ca2+i frequency was around 3 min−1 (Figure 1). As the temperature was lowered, however, we observed a substantial reduction in Ca2+o-induced Ca2+i frequency to around 1 min−1 that promptly recovered to the control level as the temperature was restored to 34°C (Figure 1). Thus, an acute change in temperature induced a reversible change in Ca2+o-induced Ca2+i frequency.
FIGURE 1. Reduced temperature reversibly lowers Ca2+i oscillation frequency in single HEK-CaSR cells exposed to elevated Ca2+o. HEK-CaSR cells were loaded with fura-2 AM then transferred to a microfluorescence apparatus and perifused with physiological saline solution (PSS) as described in the Methods section. After equilibration at control Ca2+o (0.5 mM) the cells were exposed to a near-maximal Ca2+o concentration (5.0 mM) at 34 °C and the temperature was then lowered progressively to 14 °C then restored to 34°C. Ca2+o was then returned to the control level (0.5 mM).
We next determined the effects of stepwise changes in temperature in the pathophysiological range 31°C–41°C on fura-2 loaded HEK-CaSR cells that were exposed to increases in Ca2+o from 0.5 mM to 4.0 mM. These Ca2+o levels were selected based on the known Ca2+o concentration-response behaviour of HEK-CaSR cells (Conigrave et al., 2000b; Brennan et al., 2015). The experiments were performed in two series with overlapping temperature ranges: 1) 31°C—37°C (Figure 2A); and 2) 35°C—41°C (Figure 2B). For the determination of Ca2+i oscillation frequency at distinct temperatures, ratiometric traces obtained in individual cells were processed using wavelet analysis (73—157 cells from 8—17 individual experiments) and the data were pooled.
FIGURE 2. Impact of temperature on the frequency of Ca2+o-induced Ca2+i oscillations in the absence or presence of CaSR positive modulator L-Phe. HEK-CaSR cells were loaded with fura-2 AM then transferred to a microfluorescence apparatus and perifused with physiological saline solution (PSS). After a brief period of equilibration at control Ca2+o (0.5 mM), Ca2+o was increased acutely to either (i) 4.0 mM or (ii) 2.0 mM in the absence or presence of 10 mM L-Phe. The temperature was adjusted as required using a customised heating system (see Methods). In (A, B), Ca2+o was increased acutely from 0.5 mM to 4.0 mM and the temperature was adjusted in the ranges 31°C–37°C and 35°C–41°C respectively. The dotted lines indicate 2 min periods of temperature adjustment. In (C), the cells were exposed initially to 31°C at control Ca2+o (0.5 mM) and the Ca2+o level was then increased to 2.0 mM followed by the addition of L-Phe (10 mM). Panel (D) presents the combined results of 3-4 experiments in each case. Temperature-dependent changes in Ca2+i frequency exhibited linear relationships for 4.0 mM Ca2+o (open squares) and 2.0 mM Ca2+o in the absence (open triangles) or presence (filled triangles) of 10 mM L-Phe.
At the control Ca2+o concentration (0.5 mM) all cells exhibited stable baseline Ca2+i levels. When Ca2+o was increased to 4.0 mM, however, around 95% of HEK-CaSR cells exhibited prompt oscillations in Ca2+i across the temperature range 31°C–41°C. At normal physiological temperature (37°C) the Ca2+i oscillation frequency was 4.21 ± 0.13 min−1 consistent with previous reports (Breitwieser and Gama, 2001; Brennan et al., 2015). In addition as temperature increased, Ca2+i oscillation frequency increased progressively from 2.72 ± 0.26 min−1 at 31°C to 5.62 ± 0.54 min−1 at 41°C. The Q10 temperature co-efficient was around 2.1 and a linear relationship was observed between temperature and Ca2+i oscillation frequency (Figure 2D) with a slope of 0.29 ± 0.03 min−1°C−1 (Table 1).
TABLE 1. Linear relationships between temperature and Ca2+i frequency in HEK-CaSR cells exposed to Ca2+o in the absence or presence of L-Phe Temperature: Ca2+i frequency data were obtained in HEK-CaSR cells exposed to 1) near-maximal Ca2+o (4.0 mM) or 2) submaximal Ca2+o (2.0 mM) concentrations in the absence or presence of the amino acid L-Phe (positive modulator; 10 mM). The data were subjected to Linear Regression Analysis (GraphPad Prism 8). The slopes of the temperature: Ca2+i frequency relationships are presented ±SE, together with the associated r2 values. p1 values represent the probabilities that the calculated slopes were the same as zero. p2 values represent the probabilities that the calculated slopes for 2 mM Ca2+o in the absence or presence of 10 mM L-Phe were the same as those observed in cells exposed to 4.0 mM Ca2+o. In all cases, the p-values demonstrate that the null hypotheses (no difference) were false.
We further investigated the effect of temperature on Ca2+i frequency at 2.0 mM Ca2+o, which lies below the concentration at which the CaSR is half-maximally activated (Conigrave et al., 2000b; Brennan et al., 2015), in the absence or presence of an endogenous L-amino acid modulator, L-Phenylalanine (L-Phe). As in the case of 4.0 mM Ca2+o, we observed linear relationships between temperature and Ca2+i oscillation frequency at 2.0 mM Ca2+o in both the absence and presence of L-Phe (10 mM), and L-Phe increased Ca2+i oscillation frequency at all temperatures tested, without changing the slope of the temperature—Ca2+i frequency relationship (Figures 2C, D; Table 1). Thus, Ca2+i oscillation frequencies at 2.0 mM Ca2+o exhibited a step-wise increase upon the addition of L-Phe but the calculated slope of the frequency-temperature relationship was unchanged (Figure 2D; Table 1). The Q10 temperature co-efficients were around 2.7.
We next investigated whether temperature affected the integrated Ca2+i responses to fixed Ca2+o concentrations. Thus, HEK-CaSR cells were exposed to 4.0 mM Ca2+o at different temperatures in the range 31°C–41°C (Figure 3). The Ca2+i responses were integrated using data from 10 single cells per experiment. No significant differences were observed between the different temperatures. Wavelet analysis, however, revealed that the Ca2+i frequency in each single cell was temperature dependent as described above, increasing from 2.99 ± 0.48 min−1 at 31°C, to 4.84 ± 0.70 min−1 at 37 °C, and to 6.56 ± 0.07 min−1 at 41°C. The results indicate that temperature modulated Ca2+o-induced Ca2+i oscillation frequency but had no effect on the amplitudes of the Ca2+i responses.
FIGURE 3. Lack of effect of temperature on integrated Ca2+i responses in HEK-CaSR cells. HEK-CaSR cells were loaded with fura-2 AM at baseline Ca2+o (0.5 mM) and adjusted to various set temperatures in the range 31°C–41°C. They were then exposed to elevated Ca2+o (4.0 mM) for 5 min prior to return to baseline. Panels (A—F) show time-courses for the mean responses (solid black lines) as well as responses for means plus one SD and means minus one SD (grey lines) for 10 individual cells in each case. Temperature had no effect on the integrated Ca2+i responses under the conditions of these experiments.
Cyclical phosphorylation and dephosphorylation of residue T888 in the CaSR’s intracellular C-terminus is required for Ca2+o-induced Ca2+i oscillations (Davies et al., 2007; McCormick et al., 2010). In assessing the role of the CaSR C-terminus in temperature-dependent modulation of Ca2+o-induced Ca2+i oscillations, we first tested the behavior of the chimeric receptor CaSR/mGluR1/mGluR1, which retains Ca2+o sensing properties (Mun et al., 2004) but in which the CaSR’s Cysteine-rich domain, heptahelical domain and C-terminus are replaced by the corresponding domains of its class C receptor homolog, mGluR1α, which does not support Ca2+i oscillations (Kawabata et al., 1998). In HEK-293 cells stably transfected with CaSR/mGluR1/mGluR1, we observed low frequency Ca2+i transients as Ca2+o increased from 0.5 mM to 4.0 mM but these responses did not exhibit temperature-dependent modulation (Figure 4).
FIGURE 4. Effect of temperature on Ca2+o-induced Ca2+i frequency in HEK-293 cells transfected with CaSR/mGluR1/mGluR1 chimeras. HEK-293 cells that stably expressed CaSR/mGluR1/mGluR1 chimeric receptors (HEK-C/G/G cells) or control HEK-CaSR cells were loaded with fura-2 AM and then exposed to 4.0 mM Ca2+o as described in Materials and Methods. The temperature was adjusted as required in the range 31°C–37 °C. (A) Representative single cell Ca2+i response to 4.0 mM Ca2+o in the range 31°C–37°C in HEK-C/G/G cells. (B) Linear relationships between temperature and Ca2+i frequency for HEK-CaSR (circles) and HEK-C/G/G cells (triangles) at 4.0 mM Ca2+o; several error bars are contained within the data points.
We next investigated whether temperature-dependent modulation of CaSR-mediated Ca2+i oscillations requires CaSR residue T888. We transiently transfected HEK-293 cells with either wild-type CaSR or with one of the CaSR mutants T888A or T888M, in which the PKC-dependent phosphorylation site was eliminated. HEK-293 cells that were transiently transfected with wild-type CaSR were loaded with fura-2 then exposed to 4.0 mM Ca2+o at various temperatures in the range 33°C—39°C. At 37°C the Ca2+i oscillation frequency was 4.41 ± 0.74 min−1 (n = 4) emulating the responses observed in HEK-CaSR cells, in which the CaSR was expressed stably (Suppl. Figure 1). In addition, HEK-293 cells transiently transfected with the wild-type CaSR exhibited temperature-dependent modulation similar to that previously observed for HEK-CaSR cells (Figure 5A).
FIGURE 5. Effect of T888 mutants on temperature-dependent control of Ca2+o-induced Ca2+i oscillations in transiently transfected HEK293 cells. HEK-293 cells were transiently transfected for 48 h with either the WT CaSR or CaSR mutants T888A or T888M then loaded with fura-2 AM and after equilibration to control Ca2+o (0.5 mM) exposed to 4.0 mM Ca2+o in a micro-fluorescence apparatus. Temperature was adjusted as described in the Methods section. Panels (A, B) show representative traces from single cells for WT and T888A. Panel (C) shows the relationships between temperature and Ca2+i frequency for all three constructs. Temperature-dependent modulation of Ca2+i oscillation frequency was not observed in cells transfected with either T888A or T888M. The results in C were obtained in four experiments in each case.
In HEK-293 cells that were transiently transfected with the activating mutations T888A (Figures 5B, C) or T888M (Figure 5C), the frequencies of Ca2+o-induced Ca2+i oscillations were markedly suppressed. Thus, at 37°C in the presence of 4 mM Ca2+o the Ca2+i frequency in HEK-293 cells transiently transfected with T888M was 1.1 ± 0.28 min−1 (n = 7; p < 0.05), or with T888A was 1.03 ± 0.26 min−1 (n = 3; p < 0.05).
In cells that had been transiently transfected with T888M, an increase in Ca2+o from 0.5 to 4.0 mM induced Ca2+i oscillations in around 65% of cells (45/70 individual cells) and this response was independent of temperature over the range 31°C–41°C. Similar results were obtained with HEK-293 cells that were transiently transfected with T888A. Thus, temperature had a positive effect on the frequency of Ca2+o-induced Ca2+i oscillations in HEK-293 cells transiently transfected with the wild-type CaSR but had no effect on Ca2+i frequency in HEK-293 cells transiently transfected with either T888M or T888A (Figure 5C). In particular, slopes of the temperature-dependent responses observed in HEK-293 cells transiently transfected with WT, T888M and T888A and subsequently exposed to 4.0 mM Ca2+o were, respectively: 0.36 ± 0.06 min−1°C−1; 0.01 ± 0.01 min−1°C−1; and 0.04 ± 0.05 min−1°C−1 (p < 0.01 for WT with respect to either T888M or T888A).
Since phosphorylation of CaSR residue T888 is dependent on PKC (Bai et al., 1998), we next investigated the impact of GF109203X, a potent inhibitor of both α and β1 isoforms of PKC, which was previously reported to convert Ca2+o-induced Ca2+i oscillations to sustained elevations in Ca2+i in HEK-CaSR cells (Davies et al., 2007). Exposure of HEK-CaSR cells to 4.0 mM Ca2+o at 37°C induced Ca2+i oscillations in approximately 95% of cells as observed previously and GF109203X (250 nM) transformed the Ca2+i responses from stable oscillations to sustained increases in ∼60% of cells, presumably as a consequence of the unopposed action of protein phosphatase activity (McCormick et al., 2010) (Type 1 response, Figure 6A2) 32 of 54 individual cells, n = 6). Ca2+i oscillations continued in the remaining ∼40% of cells (Type 2 response, Figure 6A ii; 22 of 54 individual cells, n = 6) and the frequencies of Ca2+i oscillations were lower than control at all temperatures tested (Figures 6B, C). Thus, in cells that continued to support Ca2+i oscillations at 41°C in the presence of GF109203X, the Ca2+i frequency was 2.18 ± 0.29 min−1 compared with 4.95 ± 0.29 min−1 (p < 0.0001) during the control period (Figures 6B, C). Similarly, in cells that continued to support Ca2+i oscillations at 33°C, the Ca2+i frequency was 1.42 ± 0.21 min−1 compared with 2.94 ± 0.53 min−1 (Figures 6B, C). Linear regression analysis demonstrated that GF109203X (250 nM) significantly reduced the slope of the relationship between temperature and Ca2+i oscillation frequency from 0.25 ± 0.05 min−1°C−1 to 0.10 ± 0.01 min−1°C−1 (p < 0.001).
FIGURE 6. Effect of protein kinase C inhibitor on temperature-dependent modulation of the frequency of Ca2+o-induced Ca2+i oscillations. HEK-CaSR cells were loaded with fura-2 AM then exposed to 4.0 mM Ca2+o in the absence or presence of the PKC inhibitor GF109203X and the temperature was adjusted in the range 33°C—41°C. At the physiological control temperature, 37°C, GF109203X (250 nM) either (i) converted Ca2+i oscillations into sustained responses (Type 1 response, ∼60% of cells; Panel (Ai, ii) reduced the frequency of Ca2+i oscillations (Type 2 response, ∼40% of cells; Panel (Aii). Panel (B) shows a representative trace from a single cell that was exposed to 4.0 mM Ca2+o and three distinct temperatures (33, 37°C and 41°C). Ca2+o-induced Ca2+i oscillations persisted upon exposure to GF109203X permitting analysis of the effect of the inhibitor as the temperature was re-adjusted to the control level. Panel (C) shows the relationships between temperature and Ca2+i oscillation frequency for HEK-CaSR cells exposed to 4.0 mM Ca2+o in the absence (circles) or presence (triangles) of GF109203X (250 nM). The data were obtained in six independent experiments.
The CaSR is a key multi-modal sensor that mediates responses to multiple metabolic signals including activating divalent cations Ca2+ and Mg2+, L-amino acids, polyamines, small peptides, and pH (Conigrave et al., 2000a; Hofer and Brown, 2003; Feng et al., 2010). It is also inhibited by ionic strength (Quinn et al., 1998) and inorganic phosphate (Centeno et al., 2019). In the present study, we have demonstrated that CaSR-mediated signalling is sensitive to an additional physical modality, temperature via modulation of the frequency of Ca2+o-induced Ca2+i oscillations. In HEK-CaSR cells we demonstrated that relatively small, stepwise increases in temperature over the pathophysiological range 31°C–41°C induced increases in the frequency of Ca2+o-induced Ca2+i oscillations, but not in the amplitudes of the Ca2+i responses or in their integrated values. Ca2+i oscillation frequency was dependent on both Ca2+o concentration and temperature, and the slope of the temperature-Ca2+i frequency relationship increased as Ca2+o increased from 2.0 to 4.0 mM. The Q10 values as temperature increased were around 2–3 (see below).
Unlike the wild-type CaSR, the CaSR/mGluR1/mGluR1 (C/G/G) chimeric receptor, which is composed of the human CaSR’s VFT domain fused to the rat mGluR1α cysteine-rich domain, heptahelical domain and intracellular C-terminus, exhibited high Ca2+o-induced low frequency Ca2+i spikes (<1 min−1) that were insensitive to temperature (Figure 4). Although mGluR1 has a moderate degree of homology to the CaSR (∼30% identity in amino acid sequence) (Hu and Spiegel, 2007) mGluR1-mediated Ca2+i responses do not take the form of PKC-dependent stable oscillations (Kawabata et al., 1998). The results demonstrate that the CaSR VFT domain is not sufficient for temperature-sensing, unlike its roles in nutrient-sensing (Mun et al., 2004) and implicate instead the receptor’s C-terminus.
The highly conserved PKC phosphorylation site T888 in the CaSR’s C-terminus is required for CaSR-mediated Ca2+i oscillations (Bai et al., 1998; Young et al., 2002; Davies et al., 2007; McCormick et al., 2010). To investigate the role of T888 in temperature-sensing we used two non-phosphorylatable mutants, T888M and T888A. T888M was identified previously in a human kindred with autosomal dominant hypocalcemia (ADH) providing evidence that phosphorylation of T888 impairs 1) sustained elevations in Ca2+i and 2) CaSR-mediated suppression of PTH secretion (Lazarus et al., 2011). Both T888M and T888A were shown previously to exhibit left-ward shifts in the Ca2+o concentration response curves for Ca2+i mobilization relative to WT (Bai et al., 1998; Lazarus et al., 2011). A small subset of cells that expressed T888M or T888A exhibited low frequency Ca2+i oscillations, apparently due to an inability to reach the threshold frequency required for the transition from Ca2+i oscillations to sustained elevations in Ca2+i (Bai et al., 1998; Lazarus et al., 2011). As predicted, cells transfected with T888M or T888A exhibited reduced frequencies of high Ca2+o (4.0 mM)-induced Ca2+i oscillations at 37°C and Ca2+i frequency was not modulated by temperature (Figure 5).
Since PKC catalyses T888 phosphorylation we investigated the impact on temperature modulation of the potent, broad-spectrum PKC inhibitor GF109203X, whose spectrum of activity includes PKC-α, the isoform that mediates inhibitory control of Ca2+i oscillations (Young et al., 2014). Consistent with a role for PKC in the control of Ca2+i oscillations (Bai et al., 1998; Davies et al., 2007), GF109203X (250 nM) converted high Ca2+o-induced Ca2+i oscillations to sustained elevations in Ca2+i in around 60% of cells, presumably due to the unopposed action of protein phosphatase activity (McCormick et al., 2010). Approximately 40% of cells, however, retained Ca2+i oscillations in the presence of GF109203X (Figure 6). In this subset of cells, the frequency of Ca2+o-induced Ca2+i oscillations was significantly decreased and their sensitivity to temperature was also markedly reduced (Figure 6).
It is possible that mechanisms relating to the management of intracellular Ca2+ fluxes may contribute to temperature-related alterations in CaSR-dependent Ca2+i oscillation frequency. Thus, store-operated Ca2+ channels including STIM1/Orai-1 (Parekh and Penner, 1997; Xiao et al., 2011), the endoplasmic reticulum Ca2+-ATPase (Shigekawa et al., 1976) and L-type voltage-gated Ca2+ channels (Peloquin et al., 2008) have all been reported to exhibit temperature sensitivity. In the present study, the loss of temperature dependence in cells transfected with the CaSR/mGluR1/mGluR1 chimeric receptor or T888 mutants, which disrupt the receptor’s primary PKC site, suggests that contributions from these effectors to CaSR-mediated temperature sensing are small.
The role of T888 phosphorylation and the impact of PKC inhibitor GF109203X points to a key role for PKC and possibly also protein phosphatase activity in CaSR-mediated temperature sensitivity. PKC and various protein phosphatases have been reported to exhibit temperature sensitivity (Sugiura et al., 2002; Kim et al., 2005; Chemin et al., 2007). Based on these considerations, any receptor that controls Ca2+i oscillation frequency in a phosphorylation-dependent manner would be expected to adjust Ca2+i frequency-dependent signaling outputs and attendant cell responses to the prevailing temperature. A phenomenon of this type operates in mesangial cells, in which activation of V1 vasopressin receptors (Jard et al., 1987) induces PKC-dependent Ca2+i oscillations modulated by temperature over the range 24°C–37°C, with a Q10 value of around 3 (Hajjar and Bonventre, 1991), comparable to that observed for the CaSR in the present study. Interestingly, V1 vasopressin receptors were shown recently to modulate temperature sensing in the hypothalamus (Xu et al., 2018).
The present findings demonstrate that the CaSR contributes to temperature-dependent changes in cell function and are consistent with a role for the CaSR in mediating Ca2+o-dependent modulation of thermoregulation in vivo. Consistent with this idea, the CaSR is expressed at high levels in the anterior preoptic nucleus of the hypothalamus (Yano et al., 2004), which participates in central thermoregulation and is sensitive to acute changes in core and local temperatures (Schmid and Pierau, 1993; Boulant, 2000; Tabarean, 2018). In addition, Ca2+o (0.9–1.5 mM) negatively modulates core temperature in various species (Hanegan and Williams, 1973; Nielsen, 1974; Myers et al., 1976), and also negatively modulates the firing rates and temperature coefficients of anterior preoptic neurons (Schmid and Pierau, 1993). Finally, in the context of type-1 Autosomal Dominant Hypocalcemia (ADH-1), arising from activating mutations of the CaSR ((Pollak et al., 1994); review: (Egbuna and Brown, 2008)) and in which there is enhanced Ca2+o-induced inhibition of Ca2+ reabsorption and attendant hypercalciuria, there is an increased risk of febrile convulsions in childhood, raising the possibility that acute drops in CNS extracellular fluid Ca2+o due to acute exacerbations of hypercalciuria might disable CaSR-mediated negative control of core body temperature, either inducing a fever or exacerbating a co-incident fever resulting in disordered CNS neuronal activity and attendant convulsions. Renal hypercalciuria arising from other genetic disorders has also been linked to febrile convulsions in childhood (Papadimitriou et al., 2001; Gorabi et al., 2018), presumably secondary to acute drops in plasma Ca2+ concentration.
In considering appropriate models for investigating the pathophysiological significance of our findings, in preliminary experiments we screened a human parathyroid cell preparation, which expresses the CaSR endogenously, for secretory responses to temperature. We observed that switching from physiological temperature (37°C) to a low temperature (30°C) reversibly lowered PTH secretion, as expected for enzyme-dependent exocytosis from membrane-bound vesicles, and also exhibited an apparent, low temperature-dependent impairment of the CaSR-mediated inhibitory response, as Ca2+o was raised from 0.8 mM to 1.2 mM. Conversely, when the temperature was raised from 35°C to 38°C, we observed enhanced PTH secretion at a Ca2+o concentration of 0.8 mM, together with enhanced suppression of PTH secretion at a Ca2+o concentration of 1.1 mM. However, the cell preparation failed to recover to its baseline PTH secretory rate upon return to the control temperature (Supplementary Figure S2). Although the results are consistent with the temperature-sensing properties we observed in the present study, the failure of the parathyroid cell preparation to recover normal secretory control following large excursions in temperature leads us to conclude that animal rather than cell models are likely to be more informative. One such model would be a tissue-selective CaSR-null mouse in which upon deletion of the CaSR from the anterior preoptic nucleus, loss of Ca2+o-dependent negative modulation of core temperature would be expected.
In the absence of readily available animal models, it is feasible, nevertheless, to identify the CaSR as a novel, functionally relevant, thermoregulatory sensor based on three key conditions (Wang and Siemens, 2015): 1) the candidate protein should exhibit thermoregulatory properties; 2) the candidate protein should be expressed in temperature-sensing CNS organs such as the anterior preoptic nucleus; and 3) thermoregulatory mechanisms should be impaired in one or more disorders related to the candidate gene/protein. Based on the outcomes of the present study and on results reported in the literature, the CaSR satisfies all three criteria. Thus, it exhibits thermoregulatory properties (Criterion-i), it is expressed in a key temperature-sensing organ, the anterior preoptic nucleus (Yano et al., 2004) (Criterion-ii), and it has been linked to a genetic disorder of thermoregulation (infantile febrile convulsions) in human cases of ADH (review: (Egbuna and Brown, 2008)) (Criterion-iii).
The Q10 temperature coefficient values for the CaSR in the current experimental system lay in the range 2.0—3.0, consistent with the behavior of enzymes (Kotov et al., 2007), and point to control by temperature-sensitive protein kinases and/or phosphatases, as noted above. This raises the possibility that the CaSR modulates the behavior of other temperature sensors. Temperature sensing has been ascribed previously to several transient receptor potential (TRP) ion channels. These ThermoTRPs include four heat-activated channels (TRPV 1—4) and three cold-activated channels (TRPM2, TRPM8 and TRPA1) (Islas and Emir, 2017). Several mammalian TRP channels have Q10 temperature coefficient values (defined by the fold-change of effector output as temperature increases by 10°C) > 5 (warm sensitive) or <0.2 (cold sensitive) (Wang and Siemens, 2015). Furthermore, some high temperature-sensitive TRP channels, such as TRPV3 and TRPV4, exhibit Q10 values around 20 (Baylie and Brayden, 2011). Whether the CaSR modulates the activation states of one or more ThermoTRP channels is unknown, however, TRPV4, which is sensitive to the temperature range 25°C–34°C (Almeida et al., 2015), is co-expressed along with the CaSR in the anterior preoptic hypothalamus and the CaSR interacts with and activates TRPV4/TRPC1 heteromeric channels (Greenberg et al., 2017), as well as TRPC1 in the parathyroid as reported recently (Onopiuk et al., 2020).
The identification of the CaSR’s temperature-sensing properties extends its repertoire of physical and chemical sensing properties (reviews: (Conigrave et al., 2000a; Conigrave and Hampson, 2006; Conigrave and Hampson, 2010; Feng et al., 2010). The results underscore the role of the receptor in systemic homeostasis and in the metabolism of specific local compartments.
In conclusion, we have demonstrated that the CaSR responds to changes in temperature in the pathophysiological range 31°C–41°C. Temperature positively and reversibly modulated Ca2+i oscillation frequency, and these responses were supported by PKC-dependent phosphorylation and, presumably, dephosphorylation of the CaSR C-terminal residue T888. Expression of the CaSR in thermoregulatory organs of the hypothalamus, observations that Ca2+o negatively modulates core body temperature together with the firing rates of thermoregulatory neurons, the existence of interactions between Ca2+o and the CaSR on the behavior of thermoregulatory ion channels, and the recognised impact of genetic disorders of calcium metabolism and/or the CaSR on thermoregulation and CNS function are all consistent with the hypothesis that the CaSR modulates thermal regulation in vivo.
The datasets presented in this study can be found in online repositories. The names of the repository/repositories and accession number(s) can be found below: https://researchdata.edu.au/effect-temperature-calcium-oscillations-dataset/267924.
The studies involving human participants were reviewed and approved by Ethics Protocol 11_067 Human Research Ethics Committee, St Vincent’s Health Network, Darlinghurst NSW 2010. The patients/participants provided their written informed consent to participate in this study.
SB assisted with the development of the main hypothesis and performed the experiments on CaSR-transfected HEK-293 cells. She also drafted multiple sections of the manuscript including key elements of the Introduction and Discussion. H-CM assisted with the experimental work including the parathyroid cell experiments. He also contributed to the editing and proof-reading of the MS. LD is an endocrine surgeon at the University of Sydney, who provided access to parathyroid tissue according to the Human Ethics protocol described in the MS. He contributed to the study design and to editing and proof-reading of the MS. PK is a medical biochemist at the University of Sydney. He developed the software in Mathematica used to estimate the frequencies of the Ca2+o oscillations observed under different experimental conditions and contributed to the editing and proof-reading of the MS. AC is a Molecular and Clinical Endocrinologist based at the University of Sydney and Royal Prince Alfred Hospital. He conceived the primary hypothesis, assisted with the experiments, drafted multiple sections of the manuscript and took overall responsibility for editing and proof-reading.
The authors declare that the research was conducted in the absence of any commercial or financial relationships that could be construed as a potential conflict of interest.
The handling editor EK declared a past co-authorship with the author SCB.
All claims expressed in this article are solely those of the authors and do not necessarily represent those of their affiliated organizations, or those of the publisher, the editors and the reviewers. Any product that may be evaluated in this article, or claim that may be made by its manufacturer, is not guaranteed or endorsed by the publisher.
The Supplementary Material for this article can be found online at: https://www.frontiersin.org/articles/10.3389/fphys.2023.1117352/full#supplementary-material
Almeida M., Vizin R., Carrettiero D. (2015). Current understanding on the neurophysiology of behavioral thermoregulation. Temp. (Austin) 2, 483–490. doi:10.1080/23328940.2015.1095270
Bai M., Trivedi S., Lane C. R., Yang Y., Quinn S. J., Brown E. M. (1998). Protein kinase C phosphorylation of threonine at position 888 in Ca2+o-sensing receptor (CaR) inhibits coupling to Ca2+ store release. J. Biol. Chem. 273, 21267–21275. doi:10.1074/jbc.273.33.21267
Baylie R., Brayden J. (2011). TRPV channels and vascular function. Acta Physiol. (Oxf). 203, 99–116. doi:10.1111/j.1748-1716.2010.02217.x
Boulant J. (2000). Role of the preoptic-anterior hypothalamus in thermoregulation and fever. Clin. Infect. Dis. 31, S157–S161. doi:10.1086/317521
Breitwieser G. (2006). Calcium sensing receptors and calcium oscillations: Calcium as a first messenger. Curr. Top. Dev. Biol. 73, 85–114. doi:10.1016/S0070-2153(05)73003-9
Breitwieser G. E., Gama L. (2001). Calcium-sensing receptor activation induces intracellular calcium oscillations. Am. J. Physiol. 280, C1412–C1421. doi:10.1152/ajpcell.2001.280.6.C1412
Brennan S., Mun H., Leach K., Kuchel P., Christopoulos A., Conigrave A. (2015). Receptor expression modulates calcium-sensing receptor mediated intracellular Ca2+ mobilization. Endocrinology 156, 1330–1342. doi:10.1210/en.2014-1771
Broadhead G., Mun H., Avlani V., Jourdon O., Church W., Christopoulos A., et al. (2011). Allosteric modulation of the calcium-sensing receptor by gamma-glutamyl peptides: Inhibition of PTH secretion, suppression of intracellular cAMP levels, and a common mechanism of action with L-amino acids. J. Biol. Chem. 286, 8786–8797. doi:10.1074/jbc.M110.149724
Brown E. M., Gamba G., Riccardi D., Lombardi M., Butters R., Kifor O., et al. (1993). Cloning and characterization of an extracellular Ca2+-sensing receptor from bovine parathyroid. Nat. Lond. 366, 575–580. doi:10.1038/366575a0
Brown E. M., MacLeod R. J. (2001). Extracellular calcium sensing and extracellular calcium signaling. Physiol. Rev. 81, 239–297. doi:10.1152/physrev.2001.81.1.239
Campion K., McCormick W., Warwicker J., Khayat M., Atkinson-Dell R., Steward M., et al. (2015). Pathophysiologic changes in extracellular pH modulate parathyroid calcium-sensing receptor activity and secretion via a histidine-independent mechanism. J. Am. Soc. Nephrol. 26, 2163–2171. doi:10.1681/ASN.2014070653
Centeno P., Herberger A., Mun H., Tu C., Nemeth E., Chang W., et al. (2019). Phosphate acts directly on the calcium-sensing receptor to stimulate parathyroid hormone secretion. Nat. Commun. 10, 4693. doi:10.1038/s41467-019-12399-9
Chemin J., Mezghrani A., Bidaud I., Dupasquier S., Marger F., Barrère C., et al. (2007). Temperature-dependent modulation of CaV3 T-type calcium channels by protein kinases C and A in mammalian cells. J. Biol. Chem. 282, 32710–32718. doi:10.1074/jbc.M702746200
Conigrave A. D., Hampson D. R. (2010). Broad-spectrum amino acid-sensing class C G-protein coupled receptors: Molecular mechanisms, physiological significance and options for drug development. Pharmacol. Ther. 127, 252–260. doi:10.1016/j.pharmthera.2010.04.007
Conigrave A. D., Hampson D. R. (2006). Broad-spectrum L-amino acid sensing by class 3 G-protein-coupled receptors. Trends Endocrinol. Metab. 17, 398–407. doi:10.1016/j.tem.2006.10.012
Conigrave A. D., Mun H.-C., Delbridge L., Quinn S. J., Wilkinson M., Brown E. M. (2004). L-amino acids regulate parathyroid hormone secretion. J. Biol. Chem. 279, 38151–38159. doi:10.1074/jbc.M406373200
Conigrave A. D., Quinn S. J., Brown E. M. (2000). Cooperative multi-modal sensing and therapeutic implications of the extracellular Ca2+-sensing receptor. Trends Pharm. Sci. 21, 401–407. doi:10.1016/s0165-6147(00)01546-7
Conigrave A. D., Quinn S. J., Brown E. M. (2000). L-amino acid sensing by the extracellular Ca2+-sensing receptor. Proc. Natl. Acad. Sci. U. S. A. 97, 4814–4819. doi:10.1073/pnas.97.9.4814
Davies S. L., Ozawa A., McCormick W. D., Dvorak M. M., Ward D. T. (2007). Protein kinase C-mediated phosphorylation of the calcium-sensing receptor is stimulated by receptor activation and attenuated by calyculin-sensitive phosphatase activity. J. Biol. Chem. 282, 15048–15056. doi:10.1074/jbc.M607469200
Egbuna O., Brown E. (2008). Hypercalcaemic and hypocalcaemic conditions due to calcium-sensing receptor mutations. Best. Pract. Res. Clin. Rheumatol. 22, 129–148. doi:10.1016/j.berh.2007.11.006
Feng J., Petersen C. D., Coy D. H., Jiang J. K., Thomas C. J., Pollak M. R., et al. (2010). Calcium-sensing receptor is a physiologic multimodal chemosensor regulating gastric G-cell growth and gastrin secretion. Proc. Natl. Acad. Sci. U. S. A. 107, 17791–17796. doi:10.1073/pnas.1009078107
Gorabi V., Nikkhoo B., Faraji O., Mohammadkhani M., Mirzaee S., Rasouli M., et al. (2018). Hypercalciuria and febrile convulsion in children under 5 years old. Korean J. Pediatr. 61, 129–131. doi:10.3345/kjp.2018.61.4.129
Greenberg H., Carlton-Carew S., Khan D., Zargaran A., Jahan K., Vanessa Ho W., et al. (2017). Heteromeric TRPV4/TRPC1 channels mediate calcium-sensing receptor-induced nitric oxide production and vasorelaxation in rabbit mesenteric arteries. Vasc. Pharmacol. 96-98, 53–62. doi:10.1016/j.vph.2017.08.005
Hajjar R., Bonventre J. (1991). Oscillations of intracellular calcium induced by vasopressin in individual fura-2-loaded mesangial cells. Frequency dependence on basal calcium concentration, agonist concentration, and temperature. J. Biol. Chem. 266, 21589–21594. doi:10.1016/s0021-9258(18)54679-2
Hanegan J., Williams B. (1973). Brain calcium: Role in temperature regulation. Science 181, 663–664. doi:10.1126/science.181.4100.663
Hannan F., Kallay E., Chang W., Brandi M., Thakker R. (2018). The calcium-sensing receptor in physiology and in calcitropic and noncalcitropic diseases. Nat. Rev. Endocrinol. 15, 33–51. doi:10.1038/s41574-018-0115-0
Hofer A. M., Brown E. M. (2003). Extracellular calcium sensing and signalling. Nat. Rev. Mol. Cell Biol. 4, 530–538. doi:10.1038/nrm1154
Hu J., Spiegel A. M. (2007). Structure and function of the human calcium-sensing receptor: Insights from natural and engineered mutations and allosteric modulators. J. Cell Mol. Med. 11, 908–922. doi:10.1111/j.1582-4934.2007.00096.x
Islas L. (2017). “Molecular mechanisms of temeprature gating in TRP channels,” in Neurobiology of TRP channels. Editor T. Emir (Boca Raton FL USA: CRC Press/Taylor & Francis).
Jard S., Lombard C., Marie J., Devilliers G. (1987). Vasopressin receptors from cultured mesangial cells resemble V1a type. Am. J. Physiol. 253, F41–F49. doi:10.1152/ajprenal.1987.253.1.F41
Jiang Y. F., Zhang Z., Kifor O., Lane C. R., Quinn S. J., Bai M. (2002). Protein kinase C (PKC) phosphorylation of the Ca2+o-sensing receptor (CaR) modulates functional interaction of G proteins with the CaR cytoplasmic tail. J. Biol. Chem. 277, 50543–50549. doi:10.1074/jbc.M205798200
Kawabata S., Kohara A., Tsutsumi R., Itahana H., Hayashibe S., Yamaguchi T., et al. (1998). Diversity of calcium signaling by metabotropic glutamate receptors. J. Biol. Chem. 273, 17381–17385. doi:10.1074/jbc.273.28.17381
Kim D., Park S., Kwon S., Youn S., Park E., Park K. (2005). Heat treatment decreases melanin synthesis via protein phosphatase 2A inactivation. Cell Signal 17, 1023–1031. doi:10.1016/j.cellsig.2004.11.020
Kotov N., Baker R., Dawidov D., Platov K., Valejev N., Skorinkin A., et al. (2007). A study of the temperature dependence of bienzyme systems and enzymatic chains. Comput. Math. Methods Med. 8, 93–112. doi:10.1080/17486700701371488
Lazarus S., Pretorius C., Khafagi F., Campion K., Brennan S., Conigrave A., et al. (2011). A novel mutation of the primary protein kinase C phosphorylation site in the calcium-sensing receptor causes autosomal dominant hypocalcemia. Eur. J. Endocrinol. 164, 429–435. doi:10.1530/EJE-10-0907
Liou A., Sei Y., Zhao X., Feng J., Lu X., Thomas C., et al. (2011). The extracellular calcium-sensing receptor is required for cholecystokinin secretion in response to L-phenylalanine in acutely isolated intestinal I cells. Am. J. Physiol. Gastrointest. Liver Physiol. 300, G538–G546. doi:10.1152/ajpgi.00342.2010
McCormick W. D., Atkinson-Dell R., Campion K. L., Mun H., Conigrave A. D., Ward D. T. (2010). Increased receptor stimulation elicits differential calcium-sensing receptor T888 dephosphorylation. J. Biol. Chem. 285, 14170–14177. doi:10.1074/jbc.M109.071084
Miki H., Maercklein P. B., Fitzpatrick L. A. (1995). Spontaneous oscillations of intracellular calcium in single bovine parathyroid cells may be associated with the inhibition of parathyroid hormone secretion. Endocrinology 136, 2954–2959. doi:10.1210/endo.136.7.7789320
Mun H., Franks A., Culverston E., Krapcho K., Nemeth E., Conigrave A. (2004). The Venus Fly Trap domain of the extracellular Ca2+-sensing receptor is required for L-amino acid sensing. J. Biol. Chem. 279, 51739–51744. doi:10.1074/jbc.M406164/200
Myers R., Simpson C., Higgins D., Nattermann R., Rice J., Redgrave P., et al. (1976). Hypothalamic Na+ and Ca++ ions and temperature set-point: New mechanisms of action of a central or peripheral thermal challenge and intrahypothalamic 5-HT, NE, PGEi and pyrogen. Brain Res. Bull. 1, 301–327. doi:10.1016/0361-9230(76)90102-7
Nielsen B. (1974). Effect of changes in plasma Na+ and Ca++ ion concentration on body temperature during exercise. Acta Physiol. Scand. 91, 123–129. doi:10.1111/j.1748-1716.1974.tb05664.x
Onopiuk M., Eby B., Nesin V., Ngo P., Lerner M., Gorvin C., et al. (2020). Control of PTH secretion by the TRPC1 ion channel. JCI Insight 5, e132496. doi:10.1172/jci.insight.132496
Papadimitriou A., Nicolaidou P., Garoufi A., Georgouli H., Karpathios T. (2001). Hypercalciuria in children with febrile convulsions. Pediatr. Int. 43, 231–234. doi:10.1046/j.1442-200x.2001.01386.x
Parekh A. B., Penner R. (1997). Store depletion and calcium influx. Physiol. Rev. 77, 901–930. doi:10.1152/physrev.1997.77.4.901
Peloquin J. B., Doering C. J., Rehak R., McRory J. E. (2008). Temperature dependence of Cav1.4 calcium channel gating. Neuroscience 151, 1066–1083. doi:10.1016/j.neuroscience.2007.11.053
Pollak M. R., Brown E. M., Estep H. L., McLaine P. N., Kifor O., Park J., et al. (1994). Autosomal dominant hypocalcaemia caused by a Ca2+-sensing receptor gene mutation. Nat. Genet. 8, 303–307. doi:10.1038/ng1194-303
Quinn S. J., Bai M., Brown E. M. (2004). pH sensing by the calcium-sensing receptor. J. Biol. Chem. 279, 37241–37249. doi:10.1074/jbc.M404520200
Quinn S. J., Kifor O., Trivedi S., Diaz R., Vassilev P., Brown E. M. (1998). Sodium and ionic strength sensing by the calcium receptor. J. Biol. Chem. 273, 19579–19586. doi:10.1074/jbc.273.31.19579
Rey O., Young S., Jacamo R., Moyer M., Rozengurt E. (2010). Extracellular calcium sensing receptor stimulation in human colonic epithelial cells induces intracellular calcium oscillations and proliferation inhibition. J. Cell Physiol. 225, 73–83. doi:10.1002/jcp.22198
Ridefelt P., Björklund E., Akerström G., Olsson M. J., Rastad J., Gylfe E. (1995). Ca(2+)-induced Ca2+ oscillations in parathyroid cells. Biochem. Biophys. Res. Commun. 215, 903–909. doi:10.1006/bbrc.1995.2549
Schmid H. A., Pierau F. K. (1993). Temperature sensitivity of neurons in slices of the rat PO/AH hypothalamic area: Effect of calcium. Am. J. Physiol. 264, R440–R448. doi:10.1152/ajpregu.1993.264.2.R440
Shigekawa M., Finegan J. A., Katz A. M. (1976). Calcium transport ATPase of canine cardiac sarcoplasmic reticulum. A comparison with that of rabbit fast skeletal muscle sarcoplasmic reticulum. J. Biol. Chem. 251, 6894–6900. doi:10.1016/s0021-9258(17)32919-8
Sohn J., Buhrman G., Rudolph J. (2007). Kinetic and structural studies of specific protein-protein interactions in substrate catalysis by Cdc25B phosphatase. Biochemistry 46, 807–818. doi:10.1021/bi061257y
Sugiura T., Tominaga M., Katsuya H., Mizumura K. (2002). Bradykinin lowers the threshold temperature for heat activation of vanilloid receptor 1. J. Neurophysiol. 88, 544–548. doi:10.1152/jn.2002.88.1.544
Szekely D., Brennan S., Mun H., Conigrave A., Kuchel P. (2009). Effectors of the frequency of calcium oscillations in HEK-293 cells: Wavelet analysis and a computer model. Eur. Biophys. J. 39, 149–165. doi:10.1007/s00249-009-0469-2
Tabarean I. (2018). Central thermoreceptors. Handb. Clin. Neurol. 156, 121–127. doi:10.1016/B978-0-444-63912-7.00007-2
Wang H., Siemens J. (2015). TRP ion channels in thermosensation, thermoregulation and metabolism. Temp. (Austin) 26, 178–187. doi:10.1080/23328940.2015.1040604
Wang M., Yao Y., Kuang D., Hampson D. R. (2006). Activation of family C G-protein-coupled receptors by the tripeptide glutathione. J. Biol. Chem. 281, 8864–8870. doi:10.1074/jbc.M512865200
Wang Y., Chandra R., Samsa L., Gooch B., Fee B., Cook J., et al. (2011). Amino acids stimulate cholecystokinin release through the Ca2+-sensing receptor. Am. J. Physiol. Gastrointest. Liver Physiol. 300, G528–G537. doi:10.1152/ajpgi.00387.2010
Xiao B., Coste B., Mathur J., Patapoutian A. (2011). Temperature-dependent STIM1 activation induces Ca²+ influx and modulates gene expression. Nat. Chem. Biol. 7, 351–358. doi:10.1038/nchembio.558
Xu J., Hou X., Tang Y., Luo R., Zhang J., Liu C., et al. (2018). Arginine vasopressin antagonizes the effects of prostaglandin E(2) on the spontaneous activity of warm-sensitive and temperature-insensitive neurons in the medial preoptic area in rats. Neurosci. Lett. 662, 59–64. doi:10.1016/j.neulet.2017.10.002
Yano S., Brown E. M., Chattopadhyay N. (2004). Calcium-sensing receptor in the brain. Cell Calcium 35, 257–264. doi:10.1016/j.ceca.2003.10.008
Young S. H., Rozengurt E. (2002). Amino acids and Ca2+ stimulate different patterns of Ca2+ oscillations through the Ca2+-sensing receptor. Am. J. Physiol. Cell Physiol. 282, C1414–C1422. doi:10.1152/ajpcell.00432.2001
Young S. H., Wu S. V., Rozengurt E. (2002). Ca2+-stimulated Ca2+ oscillations produced by the Ca2+-sensing receptor require negative feedback by protein kinase C. J. Biol. Chem. 277, 46871–46876. doi:10.1074/jbc.M207083200
Keywords: calcium sensing receptor, temperature sensing, intracellular calcium oscillations, protein kinase C, protein phosphatase, GPCR
Citation: Brennan SC, Mun H-c, Delbridge L, Kuchel PW and Conigrave AD (2023) Temperature sensing by the calcium-sensing receptor. Front. Physiol. 14:1117352. doi: 10.3389/fphys.2023.1117352
Received: 06 December 2022; Accepted: 16 January 2023;
Published: 02 February 2023.
Edited by:
Enikö Kallay, Medical University of Vienna, AustriaReviewed by:
Caroline Gorvin, University of Birmingham, United KingdomCopyright © 2023 Brennan, Mun, Delbridge, Kuchel and Conigrave. This is an open-access article distributed under the terms of the Creative Commons Attribution License (CC BY). The use, distribution or reproduction in other forums is permitted, provided the original author(s) and the copyright owner(s) are credited and that the original publication in this journal is cited, in accordance with accepted academic practice. No use, distribution or reproduction is permitted which does not comply with these terms.
*Correspondence: Arthur D. Conigrave, YXJ0aHVyLmNvbmlncmF2ZUBzeWRuZXkuZWR1LmF1
Disclaimer: All claims expressed in this article are solely those of the authors and do not necessarily represent those of their affiliated organizations, or those of the publisher, the editors and the reviewers. Any product that may be evaluated in this article or claim that may be made by its manufacturer is not guaranteed or endorsed by the publisher.
Research integrity at Frontiers
Learn more about the work of our research integrity team to safeguard the quality of each article we publish.