- 1Department of Physiotherapy, University of Granada, Granada, Spain
- 2Faculty of Health Sciences, Melilla, Spain
- 3PNI Europe, The Hague, Netherlands
- 4Chair of Clinical Psychoneuroimmunology, University of Granada and PNI Europe, Granada, Spain
Mitochondria play a key role in both health and disease. Their function is not limited to energy production but serves multiple mechanisms varying from iron and calcium homeostasis to the production of hormones and neurotransmitters, such as melatonin. They enable and influence communication at all physical levels through interaction with other organelles, the nucleus, and the outside environment. The literature suggests crosstalk mechanisms between mitochondria and circadian clocks, the gut microbiota, and the immune system. They might even be the hub supporting and integrating activity across all these domains. Hence, they might be the (missing) link in both health and disease. Mitochondrial dysfunction is related to metabolic syndrome, neuronal diseases, cancer, cardiovascular and infectious diseases, and inflammatory disorders. In this regard, diseases such as cancer, Alzheimer’s, Parkinson’s, amyotrophic lateral sclerosis (ALS), chronic fatigue syndrome (CFS), and chronic pain are discussed. This review focuses on understanding the mitochondrial mechanisms of action that allow for the maintenance of mitochondrial health and the pathways toward dysregulated mechanisms. Although mitochondria have allowed us to adapt to changes over the course of evolution, in turn, evolution has shaped mitochondria. Each evolution-based intervention influences mitochondria in its own way. The use of physiological stress triggers tolerance to the stressor, achieving adaptability and resistance. This review describes strategies that could recover mitochondrial functioning in multiple diseases, providing a comprehensive, root-cause-focused, integrative approach to recovering health and treating people suffering from chronic diseases.
1 Introduction
1.1 Mitochondria targets for root cause medicine
Over the course of evolution, the fates of mitochondria and the rest of the eukaryotic cells have become intricately intertwined. The selective advantage of this endosymbiotic relationship for the host is manyfold, and additional functions are discovered rapidly, adding insight into its significance and central role in human health. As such, dysfunctions related to mitochondria can result in detrimental health consequences. Nowadays, mitochondrial dysfunction is known to be related to a broad range of diseases. From pulmonary, urinary, and metabolic pathologies to neurological and proliferative diseases (Tian et al., 2022).
Mitochondria facilitated humans to adapt and evolve by enabling flexible physiological responses to new environments (Wallace, 2015). They are an intersection point for external experiences and biological stress responses. Reciprocally, acute physiological stressors have become a vocal point for mitochondrial functioning and health in general (Navarro-Ledesma et al., 2022). Protocols, including acute physiological stressors, show promising results in the prevention and treatment of diseases, especially those strategically combining different hormetic and evolutionary interventions (Bosma-Den Boer et al., 2012; Ruiz-Núñez et al., 2013; Lane and Martin, 2015; Pruimboom et al., 2015; Pruimboom et al., 2016; Pruimboom and Muskiet, 2018). Per the low-cost nature of these interventions, they have importance at the individual and public health levels.
This study provides a theoretical framework for the expanding field of mitochondrial functions, highlighting recent insights into multiple mitochondrial disorders and their influence on the development of different pathologies. We finally describe several treatment options based on the combinations of physiological hormetic stressors on mitochondrial and, thereby, overall health (Pinna et al., 2022). Mitochondria are true integrative hubs and might be the main connector of all biological domains involved in health and handling a plethora of chronic non-communicable diseases (CNCDs).
2 Part I: Molecular mechanism in which mitochondria are involved
As the literature states, “eukaryotes are special, and mitochondria are why” (Pinna et al., 2022). Hereafter, the various functions and characteristics of mitochondria will be discussed. The symbiosis between two prokaryotes produced the development of mitochondria and the start of all actual living organisms including plants Figure 1.
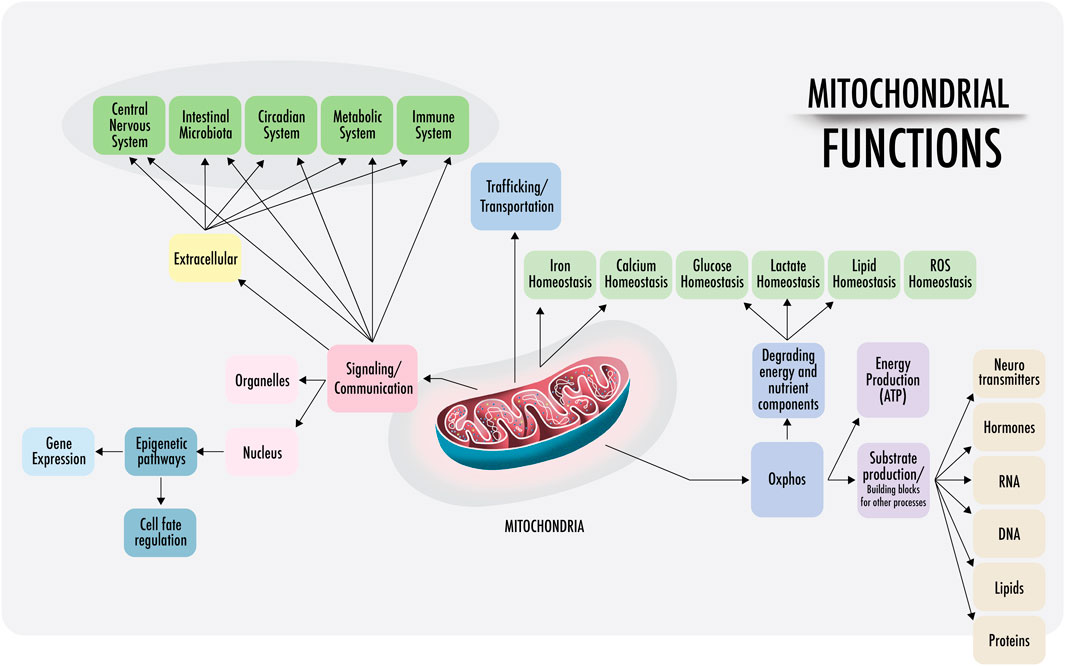
FIGURE 1. Mitochondrial functions: a visual representation of the functions of mitochondria discussed in this paper. Right: within the cell, mitochondria are anchored and transported across the cytoskeleton and cell membranes. Mitochondrial density is especially high at the perinuclear level and near the endoplasmic reticulum in most cells (also in the synaptic areas of neurons). The physical interactions of the structural domains known as mitochondria-associated membranes (MAMs) are physical contacts between organelles, such as the nucleus, lysosomes, endoplasmic reticulum, Golgi apparatus, that regulate messages through the transfer of ions and metabolites that act as a signaling center. This regulates the demands of substrate ATP and reactive oxygen species (ROS), among others. Left: intercellular communication. Cell-free mitochondria and their probable signaling functions.
2.1 ATP energy production
Figure 2 Cells require a constant supply of energy to generate and maintain the biological organization that keeps them alive and functioning. Adenosine triphosphate (ATP) is the source of energy for most cellular processes (Pinna et al., 2022). Mitochondria are the main energy production sites, converting substrates into ATP; the breakdown of nutrients into energy is called mitochondrial oxidative phosphorylation (OXPHOS) (Herzig and Shaw, 2018). Without mitochondria, humans would be dependent on the relatively energy-inefficient process of aerobic glycolysis (discussed below), a cytosolic process resulting in two ATPs per molecule of glucose. In aerobic glycolysis, glucose is converted into lactate through the reaction with nicotinamide adenine dinucleotide (NAD+). This reaction forms cytosolic reduced nicotinamide adenine dinucleotide (NADH) and lactate by lactate dehydrogenase (LDH) with a continuous reversal from lactate to pyruvate (Glancy et al., 2021). In contrast, mitochondrial OXPHOS activity yields an energy production that exceeds 30 molecules of ATP per molecule of glucose. As the body cannot easily store ATP, mitochondrial OXPHOS activity is essential for health and, therefore, should dominate cell metabolism most of the time (Bonora et al., 2012).
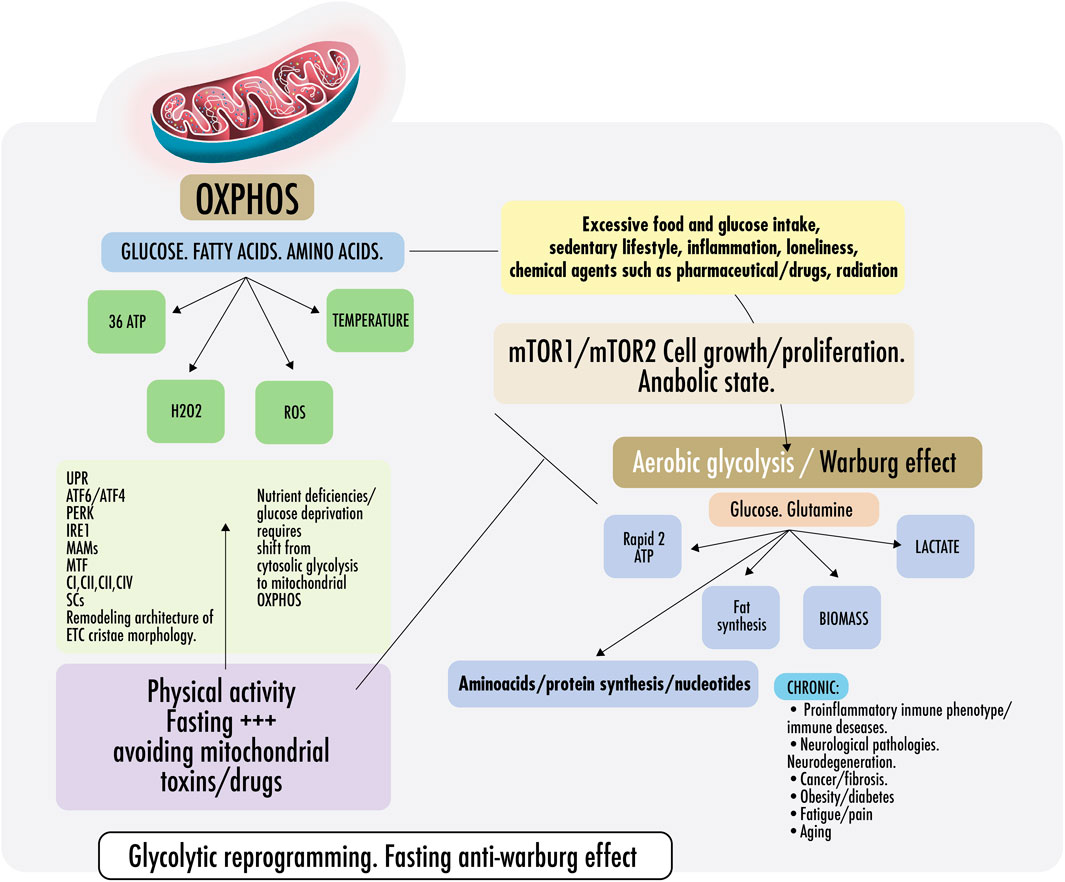
FIGURE 2. Warburg effect and anti-Warburg effect. Switch From OXPHOS to aerobic glycolysis. Warburg effect and anti-Warburg effect. Under physiological conditions, cells can change from mitochondrial respiration to cytosolic respiration. Mitochondria allow the efficient production of ATP and regulate temperature, producing ROS (OXPHOS). In the presence of nutrients, cytosolic glycolysis permits cell repair/proliferation without oxidation, which facilitates the production of amino acids, fats, and nucleotides, among others. It is important to keep these processes alternate and intermittent. Right: in pathological states, some risk factors are mentioned, such as inflammation, loneliness, glucose surplus, and some medications, which induce a metabolic change from OXPHOS to aerobic glycolysis directed by the mechanistic target of rapamycin if persistently maintained (mTORC1/mTORC2). Both continously activated result in failure of the lysosomal and autophagy mechanisms inducing persistent anabolic state, fibrosis, excess proliferation, and changes in the immunometabolic phenotype that could result in CNCDs. Left: to restore physiological states and recover OXPHOS, exercise and fasting achieve an anti-Warburg effect. During nutrient deprivation, cells demand OXPHOS to increase bioenergetic capacity by driving a decrease in fission and remodeling in the electron transport chain (ETC) or cristae morphology. It is not yet clear, but probably, ETC architecture remodeling is driven because of endoplasmic reticulum ER stress during fasting because of the disruption of protein folding and glycosylation. An imbalance in protein folding capacity starts the unfolded protein response (UPR) mechanism, activating transcription factor (ATF6/ATF4), protein kinase R- (PKR-) like ER kinase (PERK), and inositol-requiring enzyme (IRE1) to re-establish ER homeostasis and maintain protein folding. Physical contact sites termed mitochondria-ER-associated membranes (MAMs) regulate calcium homeostasis, mitochondrial fission, lipid metabolism, autophagy, and inflammasome activity. ER communicates with the OXPHOS system to increase ATP supply and promote protein homeostasis. Exercise also induced mitochondrial cristae remodeling or shaping, improving the activity of respiratory chain complexes (CI, CII, CIII, and CIV) in the inner membrane and mitochondrial respiratory efficiency. Exercise impacts the stoichiometry of the SCs, enhancing the efficiency of electron flux by segmentation of the CoQ, improving the stability of the individual respiratory complexes, and avoiding ROS excess.
Several important processes in metabolism are found within the mitochondrial matrix, including pyruvate decarboxylation by the pyruvate dehydrogenase complex (PDC), which converts pyruvate to acetyl coenzyme A (acetyl-CoA), and the same holds for fatty acid beta-oxidation enzymes and the production of acetyl-CoA from free fatty acids. All provide reducing equivalents to the tricarboxylic acid (TCA) cycle and OXPHOS (Herzig and Shaw, 2018).
The respiratory chain activity sequentially transfers electrons between four major multi-enzymatic complexes dispersed in the inner mitochondrial membrane (IMM) (Enriquez and Lenaz, 2014). Of recent interest are mitochondrial supercomplexes (SCs), evolutionarily conserved structures that are products of the association of mitochondrial respiratory chain (MRC) multi-heterodimeric complexes (I–IV). These structures are thought to provide functional advantages in the electron transfer process. SCs differ among species and tissues, depending on the metabolic and physiological conditions, as well as on the lipid content of the IMM. The most abundant SC in mammals is I + III2 + IV1, known as the respirasome and organized through phospholipid activity or the availability in the IMM. Stable SCs are essential for mitochondrial functioning, and phospholipids, such as cardiolipin and probably phosphatidylethanolamine, prevent the destabilization of SCs and possible mitochondrial dysfunctions (Lobo-Jarne and Ugalde, 2017; Nesci et al., 1103).
Other important participants in the respiratory chain are two mobile components acting as co-substrates: the lipophilic ubiquinone, coenzyme Q (CoQ), embedded in the membrane lipid bilayer, and the hydrophilic heme protein cytochrome c (cytc) located on the external surface of the IMM (Lobo-Jarne and Ugalde, 2017; Nesci et al., 1103).
Different theories exist on the organization of the respiratory chain and its components. Opposed to other models, the plasticity model describes the respiratory chain as a dynamic organization (Enriquez and Lenaz, 2014). Individual complexes and SCs are thought to participate in the electron transfer collectively and individually. Altogether, the knowledge about the way the respiratory chain functions is constantly increasing, and the same holds for the mechanisms of their dysfunction and their role in the development of (chronic) diseases and aging. Nevertheless, the exact structural organization of its components remains to be fully elucidated (Lobo-Jarne and Ugalde, 2017; Nesci et al., 1103).
2.2 Lactate shuttling
Recently, the role of lactate as an energy source has become more apparent, as well as the function of mitochondria in its metabolism. Lactate was considered a metabolic waste product with unfavorable effects. However, this view has changed, with mitochondrial L-lactate dehydrogenase (m-L-LDH) being of vital importance for the metabolism of many organs. It oxidizes lactate into pyruvate, enabling it to become a substrate for mitochondrial respiration and, thus, energy supply (Young et al., 2020).
Besides enabling lactate to become a metabolic substrate, mitochondria have a significant role in lactate shuttling. Lactate shuttling enables the exchange of lactate between producer (driver) cells and so-called consumer (recipient) cells (Brooks et al., 2022). The lactate shuttle is propelled from white fibers (drivers) to red fibers (lactate users) thanks to their high mitochondrial density (Brooks, 2002). An essential shuttle exists between muscle lactate produced during movement/exercise (driver) and its transfer to the heart and brain (receivers) that use lactate as an important energy source. Lactate from muscular cells can also be used in the liver, kidneys, lungs, sperm, and many other organs, making lactate an essential energy source, opposite to the common opinion that lactate is a toxic metabolite (Brooks, 2018; Brooks, 2020a; Brooks, 2020b; Brooks et al., 2022). Furthermore, the exchange between astrocytes and neurons is common, where lactate is metabolized and excreted by astrocytes and subsequently actively consumed and oxidized by neurons. In addition to intercellular shuttling, intracellular shuttling is possible. This refers to the exchange of lactate between cell constituents, such as the cytosol and the mitochondria or peroxisomes. Mitochondria are of essence here, as the shuttling depends on pH and concentration gradients. These are created by mitochondria in recipient (user) cells (Brooks, 2018; Brooks, 2020a; Brooks, 2020b; Brooks et al., 2022). Mitochondria contain a collection of proteins bound to the IMM and organic molecules, which electrons pass through in a series of redox reactions. The oxidation of NADH and FADH2 causes the release of energy, and the energy released forms a pH gradient of protons. Lactate transport is pH-sensitive and proton-dependent and subsequently occurs through facilitated exchange along the pH and concentration gradients, involving a family of proton-driven lactate transport proteins called monocarboxylate transporters (MCTs) (Payen et al., 2020).
Disorders in lactate metabolism are related to multiple diseases, of which perhaps cancer is the most evidenced. Lactate produced by aerobic glycolysis in cells suffering from chronic hypoxia is a so-called oncometabolite implicated in tumor epigenetic regulatory activity, tumor angiogenesis, and lipid metabolisms favoring anabolic and catabolic activity in the tumor (de la Cruz-López et al., 2019; Kes et al., 2020; Ippolito et al., 2022).
Cancer is conventionally considered a disease caused by genetic mutations, although some authors put this hypothesis in serious doubt, suggesting that in-depth knowledge of cancer metabolism is essential for the development of successful cancer therapies (Duraj et al., 2021; Seyfried and Chinopoulos, 2021; Sainero-Alcolado et al., 2022). Perhaps the most convincing evidence of cancer being a metabolic disease comes from studies conducted with cancer cells, in which the implantation of healthy mitochondria suppresses the further development of cancer cells, although the cell nucleus shows an abundant number of mutated oncogenes. Other studies showed that cancer develops when “cancerous” mitochondria are implanted in a healthy cell without any genetic mutations (Gyamfi et al., 2022). Many oncometabolites causing mitochondrial disorders and possibly initiating the cancer process have been identified, and as aforementioned, lactate is one of the most ubiquitous oncometabolites in cancer (Kes et al., 2020; Shegay et al., 2022). Aerobic glycolysis in tumor cells is important, but dynamic adjustment of mitochondrial RNA modification directly contributes to tumor malignancy and metastasis, shaping tumor metabolic plasticity (Delaunay et al., 2022). We will discuss the role of mitochondria and cancer in more depth in part II.
2.3 Maintenance of homeostasis
Mitochondria are highly mobile in vivo in neurons and in vitro in multiple cell lines (Green et al., 2022). The maintenance of mitochondrial motility is believed to be vital to cellular function. Trafficking ensures ATP supply at local sides of demand and calcium buffering. They are involved in cell differentiation and cell division to ensure proper inheritance (Debattisti et al., 2017), the efficiency of embryological development, and neurodevelopmental and immunological efficiency (Walker and Moraes, 2022). Mitochondria and their viability are primarily dependent on the level of production of cytosolic reactive oxygen species (ROS), the calcium balance, and adenosine monophosphate protein kinase (AMPK) signaling (Walker and Moraes, 2022). Within the cell, mitochondria are anchored and transported across the cytoskeleton and cell membranes. They use several anchoring proteins; for example, syntaphilin is an anchoring protein found in nerve cells and necessary for maintaining mitochondrial density and viability (Kraft and Lackner, 2018). Mitochondrial density is especially high at the perinuclear level and close to the endoplasmic reticulum (ER) in most cells. In neurons, mitochondrial density is high in synaptic areas to guarantee energy supply and efficiency of nerve transmission (Walker and Moraes, 2022). Chronic ROS stress on mitochondria can cause aging, affecting neuronal survival. Again, syntaphilin protects against malfunctioning by eliminating damaged mitochondria, and adequate removal of those mitochondria prevents axonal deterioration (Lin et al., 2017).
Homeostasis depends on the availability of sufficient energy (ATP). Mobile mitochondria can pause in regions that have a high metabolic demand. Mitochondrial distribution is often quite heterogeneous, showing enrichment at sites with high energy demand (Cunniff et al., 2016). Muscles are important regions whose ATP/ADP ratio affects contraction capacity, whereas ADP slowly dissociates from the motor to act as an inhibitor of motility (Debattisti et al., 2017). Thereby, active infiltration of mitochondria leads to the development of edge and protrusive cell structures supporting localized ATP production (Cunniff et al., 2016). ROS, either derived from an extracellular source or intracellularly generated, controls a dose-dependent mitochondrial distribution and function through the p38α pathway (Brooks et al., 2022). P38α specifically and reversibly decreases mitochondrial motility, and the role of atypical activation of p38α is part of the pathophysiology of retinal diseases, cardiovascular diseases, neurodegenerative diseases, diabetes, and cancer (Burton et al., 2021).
The fact that mitochondrial motility is induced by physiologically relevant doses of H2O2 and is further rapidly reversed by the removal of H2O2 supports the idea that ROS might work as a physiological regulator of mitochondrial distribution, temporally decelerating the migration of these organelles when and where it is required. In this context, H2O2 becomes a signaling molecule involving mitochondrial and cell motility through the dynamic rearrangement of actin networks. Therefore, it seems logical that H2O2 levels are increased in migrating cells compared to stationary ones (Pak et al., 2020). This process may enable the recruitment of additional mitochondria at the site of elevated ROS production and where they serve as scavengers and propagators and possibly protect against the development of pathophysiological conditions (Debattisti et al., 2017).
2.3.1 Calcium and its availability show a high impact on mitochondrial functioning
Diminished mitochondrial motility in the region of the Ca2+ rise promotes the recruitment of mitochondria to enhance local Ca2+ buffering (Yi et al., 2004). Both deficient as excessive Ca2+ uptake is related to different chronic disorders. Excessive mitochondrial Ca2+ uptake has been shown to induce cell death in patients with Alzheimer’s disease (AD) (Calvo-Rodriguez et al., 2020), whereas deficient Ca2+ uptake plays an important role in the pathophysiology of Parkinson’s and possibly amyotrophic lateral sclerosis (ALS) (Calvo-Rodriguez et al., 2020).
Mitochondria participate in intracellular Ca2+ signaling as modulators, buffers, and sensors (Calvo-Rodriguez et al., 2020). They can store and release Ca2+ and thus influence the shape, frequency, and amplitude of Ca2+ spikes in the cytosol (Wacquier et al., 2016).
Calcium handling by mitochondria is a key feature in cell life. The cell cycle control machinery must ensure perfect genome duplication and cell division, the basis for self-replication. Calcium-based signaling is a universal mechanism through which extracellular messengers modify the activity of target cells. Cells can decode Ca2+ signals based on the characteristics of intracellular changes in Ca2+ concentration (amplitude, duration, frequency, and location). Four fundamental, intimately related, and interdependent processes are responsible for cell life: survival, proliferation, differentiation, and death (Danese et al., 2021). Ca2+ is essential in each of these processes, especially the impact of Ca2+ homeostasis on cell death mechanisms (Contreras et al., 2010; Danese et al., 2021; Garbincius and Elrod, 2022). Mitochondrial Ca2+ uptake primarily depends on the mitochondrial Ca2+ uniporter (MCU). MCU-mediated effects drive cell cycle, ATP, and ROS production (Zhao and Pan, 2021). As aforementioned, disturbances of Ca2+ buffering can lead to many neurodegenerative disorders. Therefore, the regulation of Ca2+ by mitochondria should be an important target for the primary and secondary prevention of a wide range of neurodegenerative diseases (Rodríguez et al., 2022).
Trafficking also contributes to the cleansing and replenishment of mitochondria in the periphery (Schwarz, 2013). Postmitotic cells need to survive for the lifetime of an organism (Schwarz, 2013). Studies show that the lifetime of mitochondrial proteins varies from weeks to several years (Krishna et al., 2020). As this usually does not cover the lifetime of a cell, replenishment is necessary. Thus, constant, efficient turnover of mitochondria is important to maintain health during the entire cell life cycle. This includes the clearance of older, damaged components and the delivery of new materials (Schwarz, 2013).
A recent discovery is the existence of cell-free mitochondria. This discovery could have significant physiological consequences that need to be elucidated. Intact cell-free mitochondria oversee normal energy production and cellular metabolism. The free mitochondria may act as signals in cell-to-cell communication. An estimated 200,000–3,700,000 functional mitochondria were found per ml of extracted plasma. These are a new class of signaling organelles involved in complex regulatory activities and intercellular communication (Al Amir Dache et al., 2020). Whether functional blood-borne mitochondria serve as sentinels to maintain homeostatic metabolic activities and as a reserve for essential cellular functions remains to be confirmed. It has been suggested that these cell-free mitochondria present immunologically active proteins, such as programmed cell death-ligand 1 (PD-L1) and CD270, both associated with the upregulation of CD4 + T cells, CD8 + T cells, and reduced concentrations of pro-inflammatory cytokines (Stefano and Kream, 2022).
2.4 Building blocks and storehouses
Besides providing energy and the implications of their ability to move, mitochondria contribute to the production of multiple macromolecules, such as lipids, proteins, DNA, and RNA (Spinelli and Haigis, 2018). Mitochondria are an important site for the synthesis of steroid hormones and key neurotransmitters (Pak et al., 2020; Burton et al., 2021). Acetylcholine is an example of mitochondrial-produced neurotransmitters, the primary neurotransmitter of the autonomic system and the chief neurotransmitter of the parasympathetic nervous system (Skok, 2022). A second example is glutamate, a critical excitatory neurotransmitter in the central nervous system (CNS) and essential for multiple brain functions (Scalise et al., 2017a). Thereby, mitochondria play a critical role in the de novo synthesis of other key neurotransmitters, such as noradrenaline (NA), gamma-aminobutyric acid (GABA), and serotonin (Guo et al., 2017).
Even melatonin is produced in neuronal mitochondria (Carter et al., 2021). An interesting study, including 12 subjects, investigated the effects of lithium (Li), valproate (VPA), and lamotrigine (LTG) on humans (Danese et al., 2021). These are established therapies for bipolar disorder (BD) and other mood disturbances; however, their mechanisms of action have not yet unraveled. The results showed that the treatment with Li, VPA, and LTG led to enriched transcriptional signatures favoring the OXPHOS pathways. In addition to shared genes, they found that Li exposure was associated with 554 genes enriched for OXPHOS pathways and thermogenesis (Osete et al., 2021).
It is important to state that neurotransmitter and hormone production can only occur when mitochondria use OXPHOS as a metabolic pathway; mitochondrial dysfunction and loss of OXPHOS capacity can cause multiple hormonal and neurotransmitter dysfunctions and diseases (Suliman and Piantadosi, 2016; Danese et al., 2021).
Finally, mitochondria import iron and, in turn, store, traffic, and supply the cell with heme. Heme is necessary to assemble cytosolic and nuclear proteins that contain iron–sulfur groups (Dietz et al., 2021). Iron–sulfur groups are precursors for hemoglobin, necessary to bind oxygen in the bloodstream. Therefore, mitochondria have a significant role in iron homeostasis, strictly regulating cellular iron levels, heme, and intracellular ferritin level (Ward and Cloonan, 2019).
2.5 Mitochondria and biological rhythms
One of the most underestimated risk factors for the development of chronic diseases in the patient population is a disturbance of the circadian rhythm. Circadian misalignment is associated with numerous diseases, including neurodegenerative diseases (Sardon Puig et al., 2018; Abbott et al., 2020), cardiovascular disorders, and even cancer (Sardon Puig et al., 2018; Logan and McClung, 2019; Abbott et al., 2020). Sleep quantity and quality are influenced by multiple environmental factors, such as artificial light, meal timing, and socialization (Abbott et al., 2020). Mitochondrial functioning is much affected by the circadian rhythm, circadian misalignments affect mitochondrial viability, and mitochondrial dysfunction affects the circadian rhythm (Sardon Puig et al., 2018). The circadian timing system is hierarchically structured, with the suprachiasmatic nucleus (SCN) as a master pacemaker of all organs, including the brain. The SCN synchronizes subsidiary oscillators—clock proteins coded by clock genes—in almost every tissue and cell of the body. The oscillating chemical signals released by the SCN influence intracellular clock proteins and modulate almost all physiological processes in mammals (Garbincius and Elrod, 2022). The central mechanism responsible for the accurate function of clock genes is based on a transcription/translation negative and positive feedback loop (Acuña-Castroviejo et al., 2017a; Ashton et al., 2022).
The positive branch of rhythmic genes consists of circadian locomotor output cycles kaput (CLOCK) and brain and muscle ARNT-like/basic helix–loop–helix ARNT-like 1 (BMAL1). Other examples are neuronal PAS domain protein 2 (NPAS2) and aryl hydrocarbon receptor-like nuclear translocator (ARNTL)/BMAL1 or aryl hydrocarbon receptor-like nuclear translocator (ARNTL2)/basic helix–loop–helix-like RNAT 2 (BMAL2). Negative regulators include period (Per1, Per2, and Per3) and cryptochrome (Cry1 and Cry2). The PER and CRY proteins heterodimerize and repress their own transcription by interacting in a feedback loop with CLOCK/ARNTL complexes. In summary, PER1/2/3 and CRY1/2 are transcriptional repressors and interact with the CLOCK, NPAS2-ARNTL/BMAL1, or ARNTL2/BMAL2 heterodimer, inhibiting their activity (Acuña-Castroviejo et al., 2017a; Carmona et al., 2020; Thakur et al., 2020; Gabriel et al., 2021).
Most mechanisms maintaining an optimal circadian rhythm are related to normal mitochondrial functioning in the SNC (Aguilar-López et al., 2020), whereas the opposite is true, meaning that mitochondrial disorders affect sleep and circadian rhythm (Scrima et al., 2016; Sardon Puig et al., 2018). Mitochondria can regulate energy, subsequently allowing the regulation of the central and peripheral clocks.
Mitochondria produce melatonin in neuronal mitochondria only when energy is delivered by OXPHOS (Carter et al., 2021). The main function of melatonin is to provide a time cue to the SCN (Stefano and Kream, 2022). Furthermore, mitochondria produce NAD+, which is necessary to activate SIRT1 and SIRT3. Sirtuins have been reported to regulate central and peripheral clocks. Sirtuins further modulate the circadian epigenome and maintain specificity in transcriptional control (Stefano and Kream, 2022). Indeed, pharmacological inhibition of the mitochondrial OXPHOS system resulted in dramatic deregulation of the rhythmic clock-gene expression. A similar result was attained with mitochondrial DNA (mtDNA) depleted cells (Scrima et al., 2016).
Mitochondrial dynamics and changes in mitochondrial architecture are reported to influence circadian rhythmicity (Sardon Puig et al., 2018). Crosstalk between circadian clocks and mitochondria is given by the fact that circadian clocks regulate the biosynthesis of NAD+ and thus the mitochondrial capacity for energy production (Aguilar-López et al., 2020).
On a molecular level, there are several synchronization pathways between mitochondria and circadian molecular controllers. The clock protein CRY (Sirt1 and 3 dependent) influences mitochondrial activity, reduces gluconeogenesis, and favors substrates for OXPHOS through glycolysis, enhancing ATP production (Aguilar-López et al., 2020). AMPK detects the increase in energy and reduces the activity of CRY and the production of ATP by the mitochondria. Inactivation of CRY releases BMAL/CLOCK, which initiates a new cycle enhancing CRY and RORα expression, whereas the latter, with SIRT1/PGC1α, further increases BMAL1 expression and mitochondrial biogenesis. PGC-1α, the main regulator of mitogenesis, constitutes a link between clock genes and metabolism. SIRT1 and PGC-1α in the mitochondria regulate energy metabolism and activate mitochondrial transcription factor A (TFAM). This, in turn, regulates mtDNA copy number and transcriptional activity (Acuña-Castroviejo et al., 2017a; Poole and Ray, 2022). All resumed data show the important crosstalk between circadian rhythm and mitochondrial function, which is essential for understanding health and disease.
2.6 Communication: Mitochondria as an integrative hub
Evolutionary pressure led to multiple communication pathways between mitochondria and other organelles of the host cell and other cells. Most of those pathways are critical for cell homeostasis and are recognized as cellular and organismal signaling hubs (Shen et al., 2022a). The exemplary role of Ca2+ signaling and its interaction with circadian rhythms have been discussed earlier in this review.
Intracellular communication depends on circadian rhythm, metabolism, intestinal microbiota, and the immune system, but they are subordinate to mitochondrial functioning and communication. Mitochondria seem to regulate the back and forward trafficking of information from one domain to the other. As such, mitochondria might be a hub within the circadian clock-metabolism-intestinal-microbiota-immune system network (Aguilar-López et al., 2020). If these data are confirmed in vitro and in vivo, targeting mitochondria with evidenced-based interventions could be primary for the treatment of multiple chronic diseases related to this network. Those interventions could include exercise, regulation of gut microbiota, and cognitive training, all connected through mitochondrial functions (Clark and Mach, 2017; Zhu et al., 2022a).
Mitochondria are increasingly recognized as information hubs that sense cellular changes and transmit messages to other cellular components. They dynamically sense the constantly changing intra- and extracellular environmental milieu and relay messages to other subcellular compartments. One way of communication is facilitated by physical interactions of structural domains known as mitochondria-associated membranes (MAMs). These MAMS are physical contacts between organelles, such as nucleus, lysosomes, ER, and Golgi apparatus (Benayoun and Lee, 2019a; Wang et al., 2021a; Lv et al., 2022). They regulate messages by transferring ions and metabolites, acting as a signaling hub, and controlling many cellular functions. Alterations of these MAMS are associated with several pathologies (López-Crisosto et al., 2015; Annunziata et al., 2018; Eysert et al., 2020).
2.6.1 Mito-nuclear communication
At the moment in evolution when mitochondria became cellular endosymbionts, they transferred most of their genomes to the nucleus of the host cell (eukaryote), thereby massively reducing their number of genes (Walker and Moraes, 2022). Mitochondrial genes are encoded in the cell nucleus to produce the proteins of the outer and inner membrane, those of the intermembrane space, and most of the proteins of the mitochondrial matrix. These nuclear genes encode the proteins to be synthesized in the ribosomes. The precursor proteins are recognized by mitochondrial receptors, imported by multiple complex systems, assembled and translocated to integrate the internal–external membranes, or released into the matrix (Millichap et al., 2021).
Nuclear respiratory factors 1 and 2 (NRF1/NRF2) are transcription factors encoded in the nucleus signaling more than 250 genes. Many of them are mitochondrial genes. NRF1 regulates gene expression related to mitochondrial respiration, heme formation, and the import and assembly of mitochondrial proteins. NRF2 influences the transcription of genes related to the electron transport chain (ETC) activating complexes II (succinate dehydrogenase) and IV (cytochrome oxidase) (Millichap et al., 2021; Walker and Moraes, 2022).
A relevant function of NRF1/NRF2 is that they activate mitochondrial transcription factor A (TFAM) and peroxisome proliferator-activated receptor gamma coactivator 1α (PGC-1α). These are master regulators responsible for mitochondrial biogenesis and mitochondrial DNA stability (Benayoun and Lee, 2019a; Muri and Kopf, 2020; Calabrese and Kozumbo, 2021). NRF efficiency is necessary to regulate ROS signaling, and ROS is involved in the initiation and progression of any disease (Biagiotti et al., 2019; Bhandari et al., 2021a; Morris et al., 2021; Ulasov et al., 2021; Vashi and Patel, 2021).
Mitochondrial signals that activate nuclear responses are called “retrograde signals.” Their function is to improve energy efficiency, monitor the cell cycle, respond to cellular stress, and eliminate dysfunctional organelles, among others (Yang and Kim, 2019).
A variety of stimuli and pathways fall under the retrograde signaling umbrella. An example of the importance of mtDNA is given when depletion leads to calcium dysregulation and susceptibility to a range of diseases. mtDNA depletion syndromes are normally caused by mutations in mitochondrial genes and are very deleterious for patients suffering from mtDNA syndromes (El-Hattab and Scaglia, 2013). Calcium dysregulation can be responsible for the release of pro-inflammatory factors, cell proliferation factors, and anti-apoptotic factors (Patergnani et al., 2020). A subsequent increase in ROS, caused by mitochondrial damage or hypoxia, will induce a further hypoxic nuclear response to the increased respiration and mitochondrial and nuclear enzymatic antioxidant expression (Hernansanz-Agustín and Enríquez, 2021).
Mitochondrial products, such as acetyl CoA, α-ketoglutarate, succinate, fumarate, and FAD/FADH, will induce specific and targeted chromatin modifications and change transcriptional silencing or gene expression. NADH/NAD+, as a residue of metabolic activity, induces nuclear transcription for mitogenesis, fatty acid oxidation, DNA repair, and DNA modifications (Gyamfi et al., 2022).
Many cellular functions can be altered by mitochondrial signals. Activation of transcription factors by mitochondria leads to the translocation of transcription factors to the nucleus (Soledad et al., 2019; Walker and Moraes, 2022).
Mitochondrial-derived peptides (MDPs) are a newly recognized form of retrograde signaling substances contributing to the adaptive stress response; one of the MDPs, mitochondrial 12S ribosomal RNA type c open reading frame (MOTS-c) is a peptide with metabolic functions encoded within the mitochondrial 12S ribosomal RNA gene. MOTS-c reacts to ROS and low glucose by signaling nuclear genes to regulate cell balance (Benayoun and Lee, 2019b). Other important functions of MDPs are related to the acute stress response, for instance, caused by mitochondrial perturbation during exercise, showing protective functions and an increase in insulin sensitivity. Eight MDPs have been identified, and the use of three of them (humanin, MOTS-c, and SHLP2) (Merry et al., 2020) in rodents showed surprising positive effects related to insulin sensitivity and protection against aging processes (Merry et al., 2020). Murine animal models suggest that a decrease in MOTS is related to metabolic diseases. However, human studies show that a decrease in MOTS is differentially regulated in the skeletal muscle and plasma of healthy males during aging, suggesting that the changes in MOTS-c levels result from normal aging and do not indicate disease development (D'Souza et al., 2020). Further research on humans is necessary to show the relevance of MDPs in diseases such as type 2 diabetes and other metabolic disorders.
Mitochondrial-derived non-coding RNAs (ncRNAs) also fall under the retrograde signaling umbrella (Zhang et al., 2019). ncRNAs are a group of ribonucleic acids that are ubiquitous in the body and do not encode proteins. They have emerged as important regulatory factors in almost all biological processes. ncRNAs play a critical role in the epigenetic regulation of gene expression at the transcriptional and post-transcriptional levels. Recent evidence indicates that ncRNAs are messengers between the nucleus and the mitochondria (Vendramin et al., 2017). Common regulatory mechanisms of ncRNAs are the modulation of target genes, silencing mRNA translation or inhibition of mRNA transcription by targeting transposons, and inhibition of transcription by targeting specific loci. ncRNAs are modulators of the mitochondrial proteome. Mitochondria-localized miRNAs (mitomiRs) directly regulate mitochondrial gene expression. ncRNAs also hide small open reading frames (sORFS), encoding for small functional peptides called micro peptides (Gusic and Prokisch, 2020). Mitochondrial dysfunction leads to several diseases, such as cardiovascular diseases, cancers, and neurodegeneration. The cell/tissue-specific expression of mt-ncRNAs suggests that they could be important in mitochondria-related diseases. More in-depth knowledge about the ncRNA regulatory network would contribute to a better understanding of the etiology of mitochondrial-related diseases and lead to novel diagnostic and therapeutic approaches (Liu and Shan, 2021)
It seems clear that the complete transcriptome of mitochondria plays an essential role in the maintenance of mitochondrial viability and healthy aging. Further research is needed to see if interventions with the transcriptome as a target can help patients suffering from a wide range of chronic diseases related to mitochondrial dysfunction.
3 Part II: Mitochondrial dysfunction and its involvement in disease—Main dysregulated pathways in chronic diseases
3.1 Genetic alterations and mutations
Our review focuses on acquired dysfunctions of mitochondria and their influence on health and disease. Congenital mutations and their role in the development of chronic diseases fall out of the scope of this manuscript. Nevertheless, we will highlight some mutational changes to complete the knowledge for the reader. Mitochondrial dysfunctions are part of a group of multisystemic disorders with possible genetic alterations. Certain pathologies are linked to mutations in mtDNA, whereas others result from mutations in nuclear genes.
Mitochondrial DNA differs from nuclear DNA. Noteworthy is its maternal inheritance with a multicopy nature. There are thousands to hundreds of thousands of copies within each cell (polyplasmy). In a healthy situation, copies of mtDNA are identical (homoplasmy). Wild-type (wt) mtDNA and mutant mtDNA species frequently cohabitate in human cells, referred to as heteroplasmy. This phenomenon is commonly linked with pathological outcomes. Mutations of mtDNA can have an effect of 0%–100%, with an estimated mutation rate of 7–10 times higher than nuclear DNA. The phenotypic expression and the consequences of the mutations depend on the remaining amount of wt-mtDNA, the functionality of the mitochondria, the location in the cell, and their capacity to produce ATP in relation to the demand of the tissue. In humans, drastic changes can appear at the level of heteroplasmy in the next generation. These could either be mitochondrially transmitted adaptations or mtDNA copy numbers that flow from one generation to the following via the female germ line (Rai et al., 2018; Maude et al., 2019; Zeviani and Viscomi, 2022).
Heteroplasmy has significant implications for aging, can cause conflicts in metabolic regulation, and affects cellular functionality by altering retrograde signals. Aging, cancer, and many diseases are associated with heteroplasmy. In adult life, compared to early life, there is a downregulation of mitochondrial genes (increasing the likelihood of mitochondrial mutations) and an upregulation of genes associated with the innate immune response, the response to proteotoxicity, the response to oxidative stress, and purine biosynthesis. Compromised mitochondrial function is associated with increased ROS production, increased mitochondrial DNA fragments, and other mitochondrial peptides that activate inflammatory responses. Mitochondrial genomes do not recombine or only recombine very little (Tower, 2015; Maude et al., 2019). The question arises if mtDNA mutations will increase in frequency through the effect of human migration. If so, we can expect an increase in the number of people suffering from mitochondrial diseases caused by those mutations. In order to prevent this process, it is necessary to investigate the way mtDNA mutations are selected and which can or cannot be related to disturbances in OXPHOS (Stewart and Chinnery, 2015).
3.2 Aerobic glycolysis and chronic disease
Modern life puts a real burden on the normal functioning of mitochondria in multiple organs. Factors such as sitting time (Nogueira Silva Lima et al., 2021), high-calorie diet (Nogueira Silva Lima et al., 2021), sleep disturbance (Saner et al., 2021), and alcohol abuse (Wang et al., 2010) and factors that are hardly evitable such as environmental pollution (Grytting et al., 2022), light pollution (Grytting et al., 2022) diesel exhaust and the use of multiple medicines, including NAISDs (Tang et al., 2022), can produce a state of low-grade inflammation (LGI) associated with many chronic diseases (de Punder and Pruimboom, 2015a). LGI and mitochondrial dysfunction are two cross-connected mechanisms. Although mitochondrial dysfunction can induce low-grade inflammation (Zampino et al., 2020), LGI can cause mitochondrial dysfunction (Schmitt and Gaspar, 2022). OXPHOS defects involve energetic cost, hypermetabolism, and increased aging velocity in most, if not all, organs (Diaz-Vegas et al., 2020; Sturm et al., 2023). Mitochondria-induced inflammation can be highly detrimental to overall health. Therefore, several control mechanisms exist to prevent mitochondria-driven inflammation and mitochondria from becoming damage-associated molecular patterns (DAMPs) and even prevent autoreactivity and possible autoimmune diseases (Marchi et al., 2022).
The Baltimore Longitudinal Study of Aging, investigating a total number of 669 individuals with an average age of 67 years, showed that participants with lower mitochondrial oxidative capacity exhibited hallmarks of inflammation, specifically showing markedly higher levels of interleukin-6 and C-reactive protein, as well as increased erythrocyte sedimentation rate compared with participants with better oxidative capacity, independent of age and sex (Zampino et al., 2020). The authors of this study proposed that products of damaged mitochondria activate the immune system, which in turn causes “inflammaging,” whereas oxidative species cause inflammation and detrimental aging. The possibility of mitochondrial damage should be considered part of the fact that organisms live. Therefore, it seems logical that many mechanisms are in place to prevent mitochondrial damage and the subsequent possibility of systemic inflammation. Marchi et al. described the different protective pathways of mitochondria-induced inflammation, including mitophagy, autophagy, and cell apoptosis (Zampino et al., 2020; Marchi et al., 2022; Marchi et al., 2023). Although mitochondria-induced inflammation has been evidenced in multiple chronic diseases, interventions are scarce, and only one medicine, venetoclax, has been approved till now (Roca-Portoles et al., 2020; Olivas-Aguirre et al., 2021).
This review proposes that the use of physiological stress triggers, such as intermittent fasting, intermittent cold, and intermittent hypoxia, should be able to provide primary and secondary prevention of mitochondrial-induced systemic inflammation (the scope of this review).
As aforementioned, LGI also produces multiple metabolic disturbances in different organs through the switch from OXPHOS to aerobic glycolysis, firstly in the immune system (Pålsson-McDermott and O’Neill, 2020) and secondly in other organs through long-term insulin resistance and hyperglycemia (Guzmán-Ruiz et al., 2014).
Aerobic glycolysis in non-immune cells is responsible for the production of biomass. On the one hand, site aerobic glycolysis can protect against oxidative stress and serve as an anabolic pathway of cell repair, growth, and cell division (Brand, 1997; Yuan et al., 2019; Bell et al., 2020; Takahashi, 2021). On the other hand, long-term aerobic glycolysis, called the Warburg effect, leads to cell swelling, metabolic disturbances, lack of ATP, and, depending on the type of cell, cell death through apoptosis or necrosis and possibly cancer (Schwartz et al., 2017; Cassim et al., 2020). Modern life is responsible for the metabolic switch from OXPHOS to aerobic glycolysis through LGI, energy abundance caused by a high-calorie diet, lack of physical activity and sitting time, leading to chronic hyperglycemia and a surplus of fatty acids (Segovia et al., 2014; Barrea et al., 2017; Cuevas-Sierra et al., 2019; Milanova et al., 2019; Nicolaidis, 2019; Setayesh et al., 2019; Khan et al., 2021a; Drozdz et al., 2021; Keipert and Ost, 2021). We speculate that aerobic glycolysis in non-dividing and dividing cells should be considered the central pathway of most, if not all, chronic diseases, including most types of cancer (and its hallmarks) (Kaur et al., 2020; Kaur et al., 2022), neurodegenerative diseases, such as Alzheimer (Traxler et al., 2022), and cardiovascular diseases (Tran and Wang, 2019).
Long-term aerobic glycolysis can uncouple enzymes of normal OXPHOS, causing a chronic Warburg effect and accumulation of intracellular fatty acids, nucleotides, amino acids, and, through the activation of the polyol-mechanism, sorbitol and sorbitol-attracted water (Singh et al., 2021; Tigchelaar et al., 2022). Chronic accumulation of biomass through aerobic glycolysis and the polyol pathway is responsible for retinopathy, nephropathy, and neuropathy next to all aforementioned diseases (Balestri et al., 2022)
In line with the reviewed data, people suffering from metabolic syndrome (Mets) are susceptible to the development of many, if not all, CNCDs. MetS is characterized by numerous metabolic dysregulations, including insulin resistance, leptin resistance, dysregulated hypothalamus—hypophysis—suprarenal gland axis function, atherogenic dyslipidemia, vascular calcification, central obesity, mitochondrial dysfunction, and altered blood pressure. All these disrupted mechanisms lead to immune dysregulation and LGI, which are part of the hallmarks responsible for the development of CNCDs (Uzunlulu et al., 2016; Pruimboom and Muskiet, 2018; Drozdz et al., 2021; Khorshidian et al., 2021; Muriel et al., 2021; Fahed et al., 2022).
Many of the pathophysiological mechanisms that characterize MetS are related to mitochondrial dysfunction caused by excessive ROS production when suffering from metabolic disturbances. The oxidant/antioxidant gradient normally protects against oxidative damage. In case of oxidative pressure that exceeds anti-oxidative capacity, mitochondria and other organelles can be severely damaged. Several mechanisms and risk factors can lead to oxidative overload (Tian et al., 2022), including the upregulation of fatty acid oxidation that increases NADH and FADH2 load. Spilling some of these high-energy electrons off the TCA chain leads to excessive ROS production. The excessive amounts of glucose and free fatty acids in adipocytes activate NADPH oxidase, an enzyme that produces H2O2 (Annie-Mathew et al., 2021; Fahed et al., 2022; Wallace, 2015). Insulin-resistance induces an increase in the production of oxidative stress, reduced OXPHOS, and energy production (Wu et al., 2005; Oliveira et al., 2014; Navarro-Ledesma et al., 2022). A sedentary lifestyle reduces mitochondrial density, whereas mitochondrial biogenesis only functions in response to high-energy requirements (Magalhães et al., 2013; Pruimboom et al., 2016). The absence of a healthy dietary pattern (diversity in vegetables and spices, mushrooms, good healthy proteins and fats, fruits), which we often find in people suffering from MetS, leads to deficiency in a variety of polyphenols and micronutrients (Phillips et al., 2019).
Polyphenols are a group of substances with evidenced hormetic effects in healthy food. They are phytochemical compounds that improve metabolism, cell signaling, and mitochondrial health; the phenolic compound works as a hormetic trigger. The same mechanism characterizes the impact of the use of acute physiological stress strategies, such as intermittent fasting, therapeutic cold, therapeutic heat, and intermittent hypoxia. We speculate that the absence of hormetic triggers in modern life will decrease the transcription of NRF1 and NRF2, subsequently TFAM and mitochondrial transcription factor B2 (TFB2M), and ultimately leading to a decrease in mitochondrial mass (Kokura et al., 2007; Harvie and Howell, 2016; Chung et al., 2017; Alavi et al., 2021; Parsamanesh et al., 2021; Zeraattalab-Motlagh et al., 2021; Lavallee et al., 2022; Pak et al., 2022). The absence of hormetic triggers can also alter the activity of PGC-1α, which could result in metabolic dysfunction of tissues, leading to the development of various metabolic diseases (Vandenbeek et al., 2018).
3.3 The impact of modern life on the Warburg effect and mitochondrial dysfunction
Figure 3 States of nutrient abundance or nutrient deprivation are sensed and regulated by the “nutrient signaling” pathway, the growth axis, and regulation mechanisms of growth and division. The mechanistic target of rapamycin (mTOR) is seen as a central hub of nutrient signaling, cell growth, and division. It facilitates the metabolic switch between the use of OXPHOS and aerobic glycolysis (Liu and Sabatini, 2020a). It is also seen as a regulator of mitochondrial functions and is influenceable by acute stressors; therefore, it is of special interest in this review. mTOR controls biomass accumulation and metabolism by modulating key cellular processes, including protein synthesis and autophagy. mTOR is a 289-kDa serine/threonine protein kinase in the PI3K-related protein kinase (PIKK) family. It is made up of two complexes called mTORC1 and mTORC2. On cellular metabolic demand, mTORC1 and mTORC2 initiate biosynthetic cascades to support cell proliferation and anabolic state (Blagosklonny, 2013),
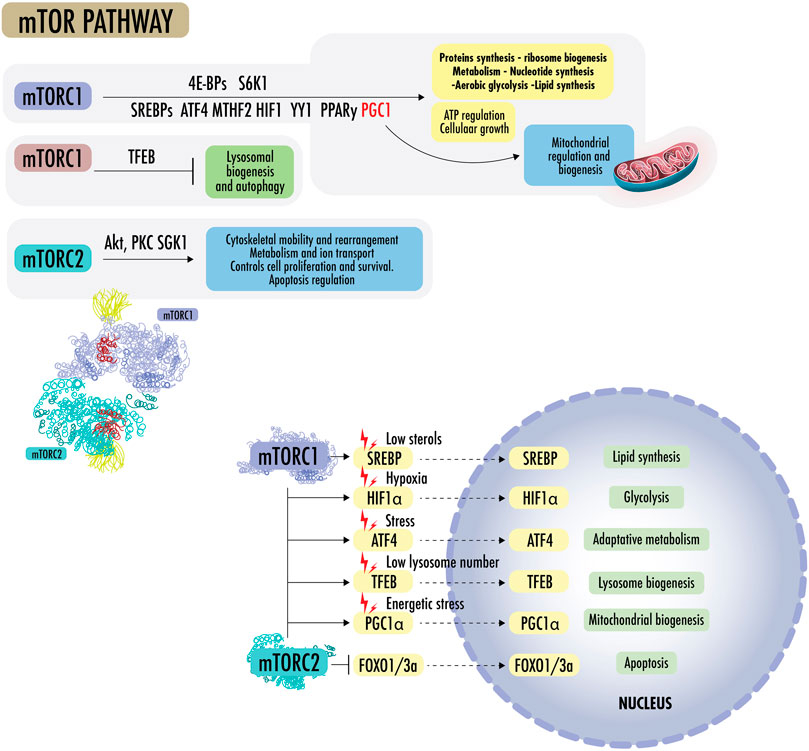
FIGURE 3. mTOR pathway: mTORC1 promotes protein synthesis by phosphorylating eukaryotic initiation factor 4E-binding proteins (4E-BPs) and p70 S6 kinase 1 (S6K1), increasing the production of ATP, nucleotides, and lipids. The lipid synthesis occurs primarily through the sterol regulatory element, protein-binding protein ½ (SREBP1/2), and peroxisome proliferator-activated receptor-γ (PPARγ). In the absence of sterols, SREBPs translocate to the nucleus to regulate genes for de novo cholesterol and other lipid synthesis. mTOR1 regulates the supply of one-carbon units for the biosynthesis of nucleotides for DNA/RNA replication, as it regulates methylenetetrahydrofolate dehydrogenase 2 (MTHFD2), the mitochondrial tetrahydrofolate cycle enzyme, carbamoyl-phosphate synthetase 2 (CPS2), aspartate transcarboxylase (ATCase), dihydroorotase (CAD), and the rate-limiting enzyme in pyrimidine biosynthesis, among others. mTORC1 regulates the transcription factor hypoxia-inducible factor 1α (HIF1α), which increases the expression of glycolytic enzymes and favors glycolysis over OXPHOS. mTORC1 activates mitochondrial transcripts through 4E-BP1 and stimulates mitochondrial biogenesis by driving PGC1α. mTORC1 can simultaneously activate SREBPs, transcription factor ATF4, HIF1, Yin Yang 1 (YY1), PPARy, and PGC1a to drive mitochondrial regulation, ATP regulation, macromolecules synthesis, and cellular growth, blocking lysosomal biogenesis through transcription factor EB (TFEB). mTORC2 effectors include protein kinase B (Akt/PKB) and protein kinase C alpha (PKCα), a member of the AGC family of protein kinases (PKA/PKG/PKC). mTOR2 plays a role in cytoskeletal rearrangement, actin regulation, chemotaxis, migration, and cell survival. Akt is a central early effector in the phosphatidylinositol 3-kinase-protein kinase B (PI3K) pathway, where it mediates the cellular response to insulin and promotes proliferation. Akt regulates metabolism to resist stressors through the transcription factors of FOXO1/3a and NAD kinase. In addition, Akt can mediate between mTORC1 and mTORC2 complexes by inactivating tuberous sclerosis complex 2 (TSC2), a strong inhibitor of mTORC1 activity.
mTORC1 promotes protein synthesis by phosphorylating eukaryotic initiation factor 4E-binding proteins (4E-BPs) and p70 S6 kinase 1 (S6K1), increasing the production of ATP, nucleotides, and lipids. The lipid synthesis occurs primarily through the sterol regulatory element, protein-binding protein ½ (SREBP1/2), and peroxisome proliferator-activated receptor-γ (PPARγ). In the absence of sterols, SREBPs translocate to the nucleus and regulate genes for de novo cholesterol and other lipid synthesis. mTOR1 regulates the supply of one-carbon units for the biosynthesis of nucleotides for DNA/RNA replication. It further regulates methylenetetrahydrofolate dehydrogenase 2 (MTHFD2), the mitochondrial tetrahydrofolate cycle enzyme, carbamoyl-phosphate synthetase 2 (CPS2), aspartate transcarboxylase (ATCase), dihydroorotase (CAD, the rate-limiting enzyme in pyrimidine biosynthesis), among others. mTORC1 regulates the transcription factor hypoxia-inducible factor 1α (HIF1α), which increases the expression of glycolytic enzymes and favors glycolysis over OXPHOS. mTORC1 activates mitochondrial transcripts through 4E-BP1 and stimulates mitochondrial biogenesis by driving PGC1α (Summer et al., 2019). mTORC1 can simultaneously activate SREBPs, transcription factor ATF4, HIF1, Yin Yang 1 (YY1), PPARy, and PGC1a to drive mitochondrial regulation, ATP regulation, macromolecule synthesis, and cellular growth, blocking lysosomal biogenesis through transcription factor EB (TFEB) (Liu and Sabatini, 2020b; Zhu et al., 2022b; Khan et al., 2022; Cui et al., 2023).
mTORC2 effectors include protein kinase B (Akt/PKB) and protein kinase C alpha (PKCα), which is a member of the AGC family of protein kinases (PKA/PKG/PKC). mTOR2 plays a role in cytoskeletal rearrangement, actin regulation, chemotaxis, migration, and cell survival. mTORC2 also collaborates with PDK1 to activate other AGC family kinases and the Akt oncogene. Akt is a central early effector in the phosphatidylinositol 3-kinase-protein kinase B (PI3K) pathway, where it mediates the cellular insulin response and promotes proliferation. Akt regulates metabolism to resist stressors through the transcription factors of FOXO1/3a and NAD kinase. In addition, Akt can mediate between mTORC1 and mTORC2 complexes by inactivating tuberous sclerosis complex 2 (TSC2), a strong inhibitor of mTORC1 activity, and phosphorylating mSin1, an obligate component of mTORC2. As the FOXO proteins are regulated by SGK1 and Akt, SGK can be the mTORC2 effector, whereas Akt appears to be a more subtle modulator (Liu and Sabatini, 2020a; Choi et al., 2020).
Macrophages exposed to an inflammatory stimulus switch their metabolism from OXPHOS to aerobic glycolysis, increasing glucose metabolism enzymes, activating transcription factors, such as mTOR and HIF1α, to support ATP production independently of the availability of oxygen and facilitating the synthesis of nucleotides, fatty acids, and proteins to support cellular function (Peruzzotti-Jametti et al., 2021). In the cells of the immune system, maintained glycolysis tends to switch immune cells to a pro-inflammatory phenotype. Upregulated glycolysis is observed in many immune cells, such as T cells, NK cells, B lymphocytes, and dendritic cells. Upregulated glycolysis could lead to immune activation with altered antibody production, lower self-tolerance, and increased cytokine release, resulting in transcriptional and post-transcriptional upregulated pro-inflammatory mediators affecting immune efficiency. This also leads to increased post-prandial inflammation responses, an important risk factor for LGI and its consequences (Teng et al., 2019; Kornberg, 2020).
In the situation of substrate surplus, adipose tissue also switches from OXPHOS to Warburg/glycolysis developing hypertrophy (increased adipocyte size), hyperplasia (increased numbers of adipocytes), or both (Choe et al., 2016). Hypertrophic adipose tissue is associated with immune cell recruitment, increased basal fatty acid release, pro-inflammatory cytokine release, hypoxia, necrotic-like abnormalities, fibrosis, decreased adiponectin, impaired insulin sensitivity, and insulin-dependent glucose uptake related to a defect in GLUT4 trafficking (Choe et al., 2016). The differentially hyperplasic adipose tissue shows increased adiponectin, decreased basal fatty acid release, and improved insulin sensitivity. It also releases pro-inflammatory cytokine and induces hypoxia and fibrosis but with fewer immune cells and a higher proportion of small adipocytes, leading to a healthier adipose tissue phenotype (Choe et al., 2016).
The adipocytes that determine a pro-inflammatory immune system are white adipocytes with low mitochondrial density. Beige and brown adipocytes characterized by high healthy mitochondrial density are metabolically efficient and maintain an anti-inflammatory and antitumoral phenotype (Corrêa et al., 2019; Symonds et al., 2019; Zoico et al., 2019). In particular, white hypertrophic adipose tissue acts as an endocrine regulator that uses many adipokines such as hormones (leptin and adiponectin); some peptides such as resistin, angiotensinogen, and apelin, among others; immune factors; and inflammatory cytokines such as interleukin-1, interleukin-4, interleukin-6, interleukin-8, interleukin-10, interleukin-18, monocyte chemoattractant protein-1, nerve growth factor, neuropeptide Y, retinol-binding protein-4, transforming growth factor-β, tumor necrosis factor- (TNF-) alpha, vascular endothelial growth factor (VEGF), visfatin, omentin, and chemerin. All of these oversee controlling orexigenic (hunger) or anorexigenic (satiety) stimuli (Coelho et al., 2013; Szewczyk-Golec et al., 2015; Tsatsanis et al., 2015; Katsiki et al., 2020)
Risk factors of Mets and switchers to glycolysis are excess sugar and unhealthy fats consumption, obesogens, sedentarism, consumption of processed food, higher intake of fructose (corn syrup, juices, soft drinks, and sweets), and many other processed foods (Dornas et al., 2015; Khorshidian et al., 2021; Muriel et al., 2021; Coronati et al., 2022; Muskiet et al., 2022). Moreover, pollution and exposure to obesogens such as endocrine-disrupting chemicals (EDC) will increase the fat amount, inflammation, and adipocyte dysfunction (Uzunlulu et al., 2016; Pruimboom and Muskiet, 2018; Heindel and Blumberg, 2019; Dietert, 2021; Khorshidian et al., 2021; Mohajer et al., 2021; Muriel et al., 2021).
Other less cited, modern factors are involved. Loneliness and chronic social isolation are also associated with upregulated lipid synthesis and a metabolic switch from OXPHOS to aerobic glycolysis and glycolytic pathway gene expression (Williams et al., 2009). Social interactions should be considered a basic need, just as other human needs, such as eating, breathing, and sleeping (Shen et al., 2022b).
The benefits of social interaction include better mental health, improved sleep quality, increased life expectancy, and improved immunological and metabolic health (Xiong et al., 2022). Related to metabolic changes observed in loneliness or chronic isolation, HPA axis chronic activation leads to the elevated secretion of cortisol, increased blood glucose, glycogenolysis, and insulin resistance that ends up in engaging in unhealthy habits and decreasing satiety signaling. A recent 20-year follow-up study including 24,024 participants found that loneliness was associated with a twofold risk of type 2 diabetes in participants who informed “very much” on experiencing loneliness than those who had not felt lonely (Henriksen et al., 2022).
New insights into the way mitochondria respond to social behavior include mitochondria-derived stress mediators (steroid hormones produced by mitochondria) and blood mitokines. An emerging circulating mitokine is cell-free mtDNA (cf-mtDNA) present in human blood, which activates immune receptors and triggers inflammatory responses. Acute psychological stress (Trumpff et al., 2019), major depression (Lindqvist et al., 2018), and intense physical activity (Stawski et al., 2017) modify mitokines production and increase circulating cf-mtDNA. Interdisciplinary approaches that involve mitochondrial signaling in resilience, aging, and metabolism are needed, and perhaps mitochondria should be defined as social organelles (Picard and Sandi, 2021).
Not only should the modern lifestyle be considered toxic for cell and mitochondrial functioning, but also medication can induce mitochondrial toxicity and a metabolic switch to glycolysis because of the impairment of OXPHOS. Many drugs have been reported to cause mitochondrial harm and damage, including benfluorex, rosiglitazone, celecoxib, ponatinib, etoricoxib, diclofenac, and remdesivir (Tang et al., 2022). Oncological drugs are reported to cause structural damage to mitochondria, including downregulated ferroptosis, accumulation of lipid peroxides, mitochondrial swelling, cristae disappearance, and matrix cavitation, as found in research with the oncological medicine doxorubicin (DOX) (Tadokoro et al., 2020; Tang et al., 2022). Related to mitochondrial complexes, zoniporide, naproxen, dronedarone, and mubritinib inhibit complex I (Tang et al., 2022). Complex II is compromised by propranolol and atenolol. Celecoxib suppresses complex IV, and As2O3 inhibits complexes I, III, and IV. Non-steroidal anti-inflammatory drugs (NSAIDs), such as nimesulide, meloxicam, and acetylsalicylate, also o inhibit OXPHOS.
Lipophilic drugs can damage phospholipids on the IMM, especially cardiolipin, or activate the mitochondrial permeability transition pore (mPTP) (Tang et al., 2022). Studies have demonstrated the presence of mitochondria-induced myopathies caused by reduced respiratory enzyme activity, calcium leakage, and oxidative stress in patients treated with statins in addition to rhabdomyolysis reported in 1 in 10,000 patients (Stoker et al., 2019; Xiang et al., 2021; Mima, 2022; Tang et al., 2022). Cancer radiation therapy in animal models induces aerobic glycolysis through ROS (Zhong et al., 2013). Chemical cancer therapies, antiviral or antiretroviral drugs, antibiotics, antidiabetic drugs, non-steroidal anti-inflammatory agents, anesthetics, and many others, impair healthy mitochondrial function altering the metabolism of many cell types, including those of the immune system (Stoker et al., 2019; Tang et al., 2022).
Almost all mentioned risk factors leading to the Warburg effect and long-term aerobic glycolysis are characterized by high blood glucose levels and an abundance of free fatty acids. The use of hormetic triggers could serve as an antidote against modern life because of a rerouting of cellular metabolism from cytosolic glycolysis to mitochondrial OXPHOS (Bianchi et al., 2015; Ng et al., 2022; Pak et al., 2022).
Lifestyle interventions, such as avoiding sedentarism, improving social connection, avoiding mitochondrial toxins/drugs (if possible), and lowering glucose intake, combined with fasting targets, could serve as an antidote against mTOR aging effects and glycolytic reprogramming. Intermittent fasting alone already induces an anti-Warburg effect (Bianchi et al., 2015; Choi et al., 2016; Mattson et al., 2017; de Cabo and Mattson, 2019; Kornberg, 2020; Locati et al., 2020; Cuevas-Cervera et al., 2022) (elaborated in PART III).
3.4 The focus on cancer as a “Warburg” disease
Cancer is considered a disease characterized by hallmarks such as aerobic glycolysis in most, if not all types of, cancers. Cancer manipulates its own metabolism and the metabolism of cells surrounding a tumor, making it a selfish-metabolic disease (Vaupel and Multhoff, 2021). Therefore, not only does the tumor cell itself depend on aerobic glycolysis for the initiation and progression of cancer, but also the tumor activates the Warburg effect in cells of the tumor microenvironment (TME), including immune cells and cancer-affected fibroblasts. By doing so, the tumors cells create a hyper-acidic, nutrient-deficient environment combined with changes in glutamine load, fatty acid metabolism, and hypoxic states that support tumor aggressivity and growth (Correnti et al., 2022; Zhu et al., 2022c; Salita et al., 2022; Wang and Abolhassani, 2022; Mora Barthelmess et al., 2023)
Different from what was earlier assumed, the acceleration of aerobic glycolysis is not a consequence of dysfunctional mitochondria perse and a compensation for the poor ATP yield per molecule of glucose. Instead, in most tumors, the Warburg effect is an essential part of selfish metabolic reprogramming. As discussed earlier, mitochondria play an important role in cell fate. The exclusion of mitochondria from the metabolism prevents the cell and its cancer from being killed by the cell fate mechanisms of mitochondria. The glycolytic switch is an early event in oncogenesis and primarily supports cell survival (Vaupel and Multhoff, 2021). The metabolic transformation leading to the Warburg effect we observe in cancer also underlies neuronal degeneration in sporadic AD (Traxler et al., 2022). Strategies intervening in this metabolic switch, inhibiting glycolysis and glutaminolysis, and promoting OXPHOS—keeping mitochondria healthy—could be interesting strategies in the fight against these conditions and others related to the Warburg effect (Manzi et al., 2015; Branco et al., 2016; Smyl, 2016; Cusso et al., 2019; Tran et al., 2020).
Besides preventing pro-apoptotic pathways mediated by mitochondria, aerobic glycolysis enables a list of other malignant progression and survival advantages for cancer cells. Examples are accelerated glycolytic fluxes, ATP generation, a backup and diversion of glycolytic intermediates, the biosynthesis of nucleotides, the production of non-essential amino acids, lipids and hexosamines, maintenance of cellular redox homeostasis, low ROS formation, inhibition of pyruvate entry into mitochondria, lactate accumulation, stimulating sustained proliferation and suppression of anti-tumor immunity, and extracellular acidosis, which accelerates malignant progression and drives resistance to conventional therapies (Vaupel and Multhoff, 2021). As the metabolic shift seems an important stone early in the domino effect of cancer initiation, preventing or intervening in this switch seems an important intervention for primary and secondary prevention of cancer.
The Warburg effect results from an interplay of different mechanisms and driving processes. HIF1 overexpression, oncogene activation (cMyc, K-ras- mTORC1, and Akt), activation of signaling pathways (PI3K/Akt/mTORC1, Ras/Raf/MEK/ERK), an increase in glucose (GLUT) and lactate (MCT4) transporters, and the activation of glycolytic enzymes (HK2, PFK1, ENO1, PKM2, and LDHA) (Wallace, 2005; Choudhury et al., 2020; Han et al., 2021; Mariani et al., 2021; Pluimakers et al., 2021; Shen et al., 2021; Vaupel and Multhoff, 2021; Zhu et al., 2022c) are all part of the metabolic change observed in cancer cells. Furthermore, the functions of tumor suppressors (mutant p53, mutant PTEN, and microRNAs 29, 143, and 144), Sirtuins 3 and 6, and the AMPK signaling pathway (Kumar et al., 2016; Alhebshi et al., 2021; Jazvinšćak Jembrek et al., 2021; Zhang et al., 2022a) are inhibited to prevent cell death and reduce metabolic stress of tumor cells. The metabolic changes belonging to the Warburg effect can be influenced by the known physiological hormetic triggers that could serve as primary and perhaps secondary preventive interventions (Jazvinšćak Jembrek et al., 2021). For instance, intermittent fasting programs could perhaps serve as Warburg antidote, blocking enzymatic pathways and creating amino-acid and glucose starvation (Lee et al., 2012; Harvie and Howell, 2016; Bauersfeld et al., 2018; Fassier et al., 2018; Lende et al., 2019; Tiwari et al., 2022). Glutamine executes multiple functions in cancer cells. Besides being an energy source, glutamine is a so-called anaplerotic molecule. Glutamine can replenish the TCA cycle with intermediates extracted for biosynthesis. In this regard, glutamine is an alternative source for the TCA cycle. Thereby, glutamine uptake by the energetically transformed cell contributes to the formation of nucleotides and fatty acids and has an important role in the homeostasis of ROS (Alhayaza et al., 2020). Another important oncometabolite is leucine, which, together with glutamine, can activate the mTOR complex (cell growth master) (Scalise et al., 2017b). As aforementioned, intermittent fasting could serve as an mTOR antidote.
3.4.1 A brief description of glutaminolysis in cancer
When glutamine is absorbed by metabolically transformed cells, it is converted into glutamate. Thereafter, glutamate is converted into α-ketoglutarate, which enters the TCA in the mitochondria, where the reaction is catalyzed by succinyl-CoA synthetase with the resulting production of ATP. In the above process, one of the five carbon atoms of glutamine is released as CO2. The remaining four carbon atoms of glutamine are exported to the cytosol as malate, which, in turn, can give rise to different metabolic pathways useful for cancer cells, including the conversion into pyruvate (Scalise et al., 2017c). Pyruvate can be converted into lactate for aerobic glycolysis and ATP production. The conversion of glutamine into glutamate is regulated by an oxidative reaction orchestrated by glutaminase (Zhang et al., 2022b). Alternatively, malate can enter the TCA cycle as a molecule with four carbon atoms, including asparagine alanine-serine cysteine-preferring transporter 2 (ASCT2) substrates (Scalise et al., 2018). Malate is converted into oxaloacetate via malate dehydrogenase and then into aspartate via aspartate aminotransferase (Scalise et al., 2018). The alternative output substrate of ASCT2, serine, can be derived from glucose (via phosphoglycerate dehydrogenase/phosphoserine aminotransferase/phosphoserine phosphatase). The enzymes mentioned serve as important targets in the development of anticancer therapies. The glutaminase enzyme is produced by two different genes: GLS1 and GLS2 (Scalise et al., 2017b; Zhang et al., 2022b). These genes are important therapeutic targets, and inhibition could serve as a promising cancer intervention.
Other targets of cancer preventive interventions are glucose and glutamine transporters and their increase in the cancer process. Cancer cells overexpress glucose transporters (GLUTs), sodium-dependent amino acid transporters such as ASCT2, and sodium-independent amino acid transporters for signaling, such as LAT1 (Scalise et al., 2017c; Scalise et al., 2020). Glutamine is also recognized at the plasma membrane by SLC receptors (including members, e.g., SLC1, SLC6, SLC7, SLC36, and SLC38). Within this family, the major and best-characterized glutamine transporter is SLC1A5. SLC1A5 is currently known as ASCT based on preliminary observations of substrate specificity, although the actually preferred substrate is now known to be glutamine (Scalise et al., 2017c). The most likely exchanged amino acids by SLC1A5 are asparagine, threonine, or serine, and their transport, together with glutamine, allows the entry of 1–2 carbon atoms into the cell, which can then be oxidized in the TCA with ATP production in the mitochondria. The increase in the plasmatic concentration of serine and threonine is well-described in cancer (Scalise et al., 2017c). It seems clear that amino acids are involved in cancer metabolic rewiring, and several are essential for the initiation and progress of cancer (Scalise et al., 2020; Wang et al., 2022a; Shen et al., 2022c)
As touched upon briefly, the metabolic transformation seen in tumor cells also enhances a favorable ROS environment. ROS accumulation can directly affect DNA integrity, and ROS-mediated DNA damage could favor the initiation stage of tumorigenesis. ROS have also been associated with epigenetic alterations that favor oncogenic transformation. ROS-induced hypermethylation of the promoter region of tumor suppressor genes has been shown to promote carcinogenesis. Cancer cells also need to keep ROS production under control, and glutamine converted in glutamate serves the synthesis of glutathione peroxidase as a major anti-oxidative enzyme from glutamine. Intervening in this pathway also seems important (Shi et al., 2022).
Another possible factor causing the metabolic transformation of cells into tumor cells is related to the presence of mutations in mitochondria caused by “modern life.” Enzymes produced by mutated mtDNA are related to cancer development and the use and production of several oncometabolites (Kes et al., 2020). Oncometabolites are signaling molecules derived from mitochondria dysfunction. Some examples found in various types of cancer are loss-of-function mutations of the iron-sulfur B subunit of the succinate dehydrogenase complex (SDHB) and fumarate hydratase (FH) with increased levels of fumarate and/or succinate (Haas and Nathanson, 2014; Gupta et al., 2019). Others are gain-of-function mutations in cytosolic and mitochondrial isocitrate dehydrogenase (IDH) isoforms 1 and 2 with the production of 2-hydroxyglutarate (2-HG). These combinations lead to the loss of α-ketoglutarate (α-KG) production and simultaneous gain of 2-HG that alters transcriptional patterns of histone methylation regulation and whole DNA methylation in favor of tumor growth (Cassim et al., 2020).
Pyruvate kinase M (PKM) is the glycolytic enzyme that converts phosphoenolpyruvate to pyruvate. The PKM gene codes for two isoforms, PKM1 and PKM2, which code for 22 different amino acids. PKM2 is the most common isoform of this enzyme in cancer (van Niekerk and Engelbrecht, 2018; Li et al., 2022a). The pathological isoform of PKM toward the cancer-associated PKM2 isoform causes metabolic and transcriptional changes. These alterations occur through the lack of metabolic activity of PKM2. PKM2 specifically interacts with the STAT3 and HIF1a transcription factors and enhances them, together exerting pro-oncogenic programs in which HIF1a is aberrantly activated despite a normoxic environment (Traxler et al., 2022).
Altogether, pro-carcinogenic changes have a solid metabolic basis, and changes in metabolism are a leading therapeutic target for the treatment of patients with cancer, which is among the leading causes of mortality and, in many countries, risk factor number one (Bray et al., 2018; Bray et al., 2021). As many processes are involved at the same time, primary and perhaps secondary prevention should be achieved by multiple lifestyle interventions. In the scope of this review, the use of physiological hormetic triggers possibly stops the domino cascade, leading to the development of neoplasms.
3.5 Infection and endotoxemia
Most CNCDs involve endotoxemia, LGI, and/or intestinal permeability. Intestinal barrier dysfunction results from food poisoning, dietary factors, and dysbiosis, and increased permeability leads to the translocation of sterile toxins and living or dormant microbes and lipopolysaccharides (LPS) into the bloodstream (de Punder and Pruimboom, 2013; de Punder and Pruimboom, 2015b).
The resulting endotoxemia leads to a sustained or low inflammatory and stress response. Stress induces corticotropin-releasing hormone (CRH), which stimulates mucosal cell cytokine release, which can, in turn, increase TNF that results in loose tight junctions and pathological gut permeability (Takakura and Pimentel, 2020; Michielan and D’Incà, 2015; Fasano, 2020; Woodhouse et al., 2018; Cristofori et al., 2021). Translocation of intestinal xenotoxins and micro-organisms should be considered one of the main pathways leading to low-grade inflammation (Laugerette et al., 2011; de Vries et al., 2014; Mohammad and Thiemermann, 2021). In the intestine only, there are more than 100 billion microbes, including archaea, bacteria, fungi, protozoa/parasites, and viruses. The immune, mitochondrial, and metabolic characteristics of the gut environment will depend on the enterotype (distribution taxonomic classification of microbial families, mucus quality, and diversity of each intestine) (Pimentel and Lembo, 2020). A gut pathological microbiome has been related to chronic disease, LGI, and mitochondrial dysfunction, and the so-called atopbiome in other tissues is frequently recognized as a risk factor for chronic diseases. Our industrialized society, with the presence of a wide range of toxic chemicals, metals, and antibiotics, has completely changed the microbiome, which affects mitochondrial functionality and organ function (Demeneix, 2019; Martel et al., 2022).
Modern life also comes with environmental exposure to many pathogenic microorganisms and resistant microbes exposed to insecticides or pesticides. Dysbiosis caused by pathobionts impairs mitochondrial integrity through multiple pathways, impairing healing mechanisms and promoting chronicity. Therefore, improving mitochondrial health is a way to prevent ROS overload and mtDNA deterioration and subsequently improve immune responses (Michielan and D’Incà, 2015; Fasano, 2020; Martel et al., 2022; Akdis, 2021; Rizzetto et al., 2018; Chopyk and Grakoui, 2020; Mou et al., 2022). In other words, strengthening mitochondria as much as possible helps us deal with the deteriorating consequences of modern life.
An acute infection induces dysregulation in mitochondria-nuclear communication, which is part of the resolving response when it is time-restricted. In an LGI, this dysregulation lasts long and should be considered one of the most important steps in the development of chronic diseases (Sureshbabu et al., 2018; Sun et al., 2019; Piotrowicz et al., 2021). Mitochondria are a major location for the production of ROS, which is necessary to fight infections. Evolutionary pressure made pathogens exploit the mitochondrial influence in killing and developed mechanisms to disturb mitochondrial–nucleus communication in fighting infections and increased the survival of the struggling pathogens (Andrieux et al., 2021).
The existence and optimal functioning of pattern-recognizing receptors (PRRs) are essential for optimal immune functionality. There are four families of PRRs distributed throughout all types of cells in the human body, namely, TOLL-like receptors (TLRs), NOD-like receptors (NLRs), retinoic acid-inducible gene I-like receptors RIG-I (RLRs), RIG-I receptors, and C-type lectin receptors (CLR). Each PRR can trigger a response through the nuclear transcription factor Kappa beta (nfKb) that allows the transcription of interferon and pro-inflammatory cytokines dependent on the pathogen that activated the PRR (Sandhir et al., 2017; Andrieux et al., 2021).
In the antiviral response, the mitochondrial antiviral signaling protein (MAVS)—located in the outer membrane of the mitochondria—is involved in the recognition and detection of viruses via the RLR receptor signaling pathway. Mitochondrial dynamics (fusion/fission) also regulate the RLR signaling pathway. The interaction between MAVS and the mitochondrial outer membrane protein mitofusin (MFN) and mitochondrial fusion are required in the RLR signaling pathway. In animal models with the deletion of MFN1 and MFN2, responses via RLR are reduced and impair the antiviral response. ROS are essential in antimicrobial signaling and efficiency because macrophages and dendritic cells eliminate microorganisms by phagocytosis. The proximity between the alpha-phagosomes and the mitochondria allows mtROS to cross the phagosome and eliminate the pathogen (Sandhir et al., 2017; Tan et al., 2017).
The NRLP3-dependent inflammasome is also an immune response that induces inflammatory cell death (pyroptosis) and has the function of eliminating intracellular bacteria/viruses before they proliferate too much. It allows the recruitment of other immune cells to improve anti-infective efficiency. The activation of the NLRP3 inflammasome is related to the triggering of TLRs and the production of pro-IL1b and pro-IL18 via caspase-1 activation in response to microbial infection and cellular damage (PAMP and DAMP signaling). Free mtDNA, ROS, and the MAVS pathway are required for the recruitment of NLRP3 to mitochondrial membranes. The phospholipid cardiolipin translocates from the inner to the outer membrane of the mitochondria to bind to NLRP3 and promote its activation. The accumulation of mtDNA in the cytosol results in an antiviral immune response, and the oxidation of mtDNA and cardiolipin leads to the activation of the inflammasome that ultimately induces a pro-inflammatory response that should resolve in time (Kelley et al., 2019; Deo et al., 2020; Wang et al., 2022b; Harapas et al., 2022).
Besides the necessity of mitochondrial involvement in eliminating pathogens, damaged mitochondria can be the cause of inflammation due to their prokaryotic (bacterial) origin. Damage causes free mtDNA, which is perceived as DAMP, and triggers inflammation. Therefore, infection is not the only cause of inflammation; mitochondrial damage could be a cause as well (Tan et al., 2017; Kelley et al., 2019; Deo et al., 2020; Alfarouk et al., 2021; Andrieux et al., 2021; Harapas et al., 2022).
The role of mitochondria in the antibacterial response is evidenced by their role in apoptotic regulation. Host cells and their mitochondria produce ROS to damage the lipids, proteins, and nucleic acids of some bacteria. In addition, mitochondrial fission via dynamin-related protein 1 (DRP1) maintains cell homeostasis during infection to prevent propagation by modulating cell apoptosis because if the cell dies, the infection can spread (Andrieux et al., 2021; Yang et al., 2022).
Pathogen infection changes the mitochondrial- metabolic- and oxidative profile of cells. Infected cells cause dysregulation of several nuclear genes through retrograde signaling. When pathogens exploit and eliminate mitochondrial defense mechanisms, they are much more efficient in bypassing immune mechanisms. Pathogens have learned to use pathways that avoid both immune and mitochondrial anti-infective effects. The aberrant/persistent activation of the NLRP3 inflammasome leads to chronic inflammatory disorders with LGI and endotoxemia. The treatment of mitochondrial damage and gut permeability and the restoration of gut equilibrium are promising targets for optimizing and resolving infection at the time. Mitochondria are vital in triggering the immune response related to RLR, NLR, or TLR against viruses, protozoa, bacteria, fungi, or damage (Kelley et al., 2019; Curi et al., 2020; Saleh et al., 2020; Andrieux et al., 2021).
The persistence of pathological microbes harms the integrity of the intestinal barrier, mediating immune and mitochondrial failure. Even biomechanical disorders, such as intervertebral discopathies, seem to be associated with disc infection caused by oral and skin-derived pathogens (Rajasekaran et al., 2020; Li et al., 2022b; Shanmuganathan et al., 2022). Besides the aforementioned mechanisms, dysbiosis reduces the supply of calcium to the bone and impairs the ability to manufacture vitamins K2 and D and other important immuno-metabolic substances. Therefore, dysbiosis and the resulting endotoxemia affect the integrity of bones (Radaic and Kapila, 2021; D’Amelio and Sassi, 2018). Dysbiosis mediates more aggressive immunological disorders in genetically predisposed individuals, and dysbiosis is related to Multiple immune pathologies. Examples are multiple sclerosis (Opazo et al., 2018; Kinashi and Hase, 2021; Mou et al., 2022), type 1 diabetes (Lin and Zhang, 2017; Kinashi and Hase, 2021), inflammatory bowel diseases (de Oliveira et al., 2017; Lin and Zhang, 2017; Opazo et al., 2018), rheumatoid arthritis (Horta-Baas et al., 2017; Jethwa and Abraham, 2017; Lin and Zhang, 2017; Nikitakis et al., 2017), autoimmune liver diseases (Virili and Centanni, 2017; Chopyk and Grakoui, 2020), thyroid diseases (Knezevic et al., 2022), celiac disease (Cenit et al., 2015; Videhult and West, 2016), systemic lupus erythematosus (Opazo et al., 2018; De Luca and Shoenfeld, 2019; Konig, 2020), and atopic dermatitis and allergies (McKenzie et al., 2017; Shu et al., 2019; Carucci et al., 2021). The interconnection between endotoxemia, mitochondrial dysfunction, LGI, and even non-resolved infection demands more integrative interventions. Lifestyle interventions and the use of physiological hormetic stress triggers could serve as interventions for primary and secondary prevention of chronic diseases, the scope of this review.
3.6 Neurological diseases. Mitochondria in the brain
The metabolic activity of brain neurons is, together with the activity in the lungs and kidneys, the highest in the human body. Neurodegeneration and neurodegenerative diseases (Alzheimer’s, Parkinson’s, and ALS) or neuroinflammatory disorders (myalgia encephalitis/chronic fatigue syndrome (CFS), and chronic pain) are all related to mitochondrial dysfunction (Fairbrother-Browne et al., 2021; Kumar et al., 2022; Rey et al., 2022).
The disruption of any of the mitochondrial dynamics or alteration of functions, such as energy production, ROS production, calcium homeostasis, control of epigenetic and nuclear transcriptional processes, immune defense, lipid regulation, and glucose regulation, can have deleterious effects on neurons and neuroglial cells (Fairbrother-Browne et al., 2021; Kumar et al., 2022; Rey et al., 2022).
Although neurological diseases vary in terms of their underlying risk factors and mechanisms, mitochondrial dysfunction is common in most, if not all, of them. Mitochondria are necessary for the energy supply of neurological cells, as their energy consumption is incredibly high, with the brain as one of the most specialized and metabolically active organs. Accordingly, neurons in the brain have a dense population of mitochondria consuming 20% of the total energy expenditure, whereas the brain weight is only 2% of the total body mass. The human brain is dependent on a continuous demand for energy in the form of glucose that is converted into lactate by Glia cells because neurons use 80%–90% of energy in the form of lactate. In basal conditions, neurons and astrocytes will use the same amount of energy sources. Although neurons synthesize ATP through OXPHOS, astrocytes are specialized in metabolizing glucose through aerobic glycolysis, resulting in an enormous amount of lactate and pyruvate generation from glucose that can be transported to neurons by MCTs and hydrocarboxylic acid receptors 1 (HCAR1) (Sharma et al., 2019; Mani et al., 2021; Bhatti et al., 2022; Wang et al., 2022c; Zeviani and Viscomi, 2022).
Parkinson’s disease (PD) could be considered a mitochondrial dysfunctional disease. One of the mitochondrial mechanisms involved in PD is related to the function DJ-1 protein. DJ-1 is an oxidative stress sensor that prevents neuronal death induced by oxidative stress and inhibits the aggregation of α-synuclein via its chaperone activity. Dj-1 mutation causes mitochondrial dysfunction and accumulation of α-synuclein, the hallmark of PD (Dolgacheva et al., 2019; Chia et al., 2020). This could also lead to complex I deficiency in muscular and immune cells, substantia nigra, and platelets, as seen in patients with PD (Rey et al., 2022). MAM and ER defects induce translocation of the MAM components, such as IP3R, VDAC, and MFN1 and MFN12. This can lead to disturbed calcium homeostasis and cause misfolded proteins with impaired autophagy, distorted mitochondrial dynamics, and cell death (Sunanda et al., 2021). Sirtuins are NAD+-dependent protein deacetylases, and seven sirtuin members can be involved (SIRT1–SIRT7) (Grünewald et al., 2019; Lautrup et al., 2019). Alterations in mitochondrial-located sirtuins (SIRT3, SIRT4, and SIRT5) induce mitochondrial transcriptional problems, which might result in pathologies (He et al., 2022; Kumar et al., 2022). Iron is a key element for mitochondrial function and homeostasis, which is also crucial for the maintenance of the neuronal system. However, too much iron promotes oxidative stress, immune response, and altered mitochondrial proteins. Patients with PD show an over-storage of iron compared with controls that could be caused by blood barrier dysfunction and subsequently lead to iron storage in the substantia nigra (Cheng et al., 2022). Another explanation can be an upregulation of some iron-storage proteins such as mitoferritin, lactoferrin, and transferrin or increased expression of DMT1 in dopamine neurons and ceruloplasmin dysfunction, observed in multiple neurodegenerative diseases (Zhang et al., 2021a; Cheng et al., 2022).
In ALS, mitophagy and increased ROS are markedly involved in its pathogenesis, resulting in a reduced number of phagosomes at the neuromuscular junction (Tsitkanou et al., 2016; Baek et al., 2022; Cheng et al., 2022). Mutations (acquired or congenital) in the genes for the production of mitochondrial proteins FUS, TDP-43, SOD1, and C9ORF72 have been reported (Wong and Venkatachalam, 2019; Rey et al., 2022). More than 170 SOD1 mutations are associated with ALS. SOD1 codes for Cu-Zn superoxide dismutase, which is responsible for neutralizing superoxide radicals by catalyzing molecular oxygen and hydrogen peroxide. Mutations in FUS, TDP-43, SOD1, and C9ORF72 increase DNA damage, alter mitochondria fusion and fission, alter calcium homeostasis, and reduce the activity of respiratory chain complexes II and IV (Kodavati et al., 2020; Rey et al., 2022). This leads to structural abnormalities, such as swollen mitochondria, impaired MAM functions, augmented mitochondrial fragmentation, progressive loss of membrane potential, increased ROS production, and defective mitochondrial axonal transport (Kodavati et al., 2020; Rey et al., 2022). Inhibition of the action of the GTPase involved in mitochondrial fission dynamin-related protein 1 (DRP1) and reduced levels of mitochondrial metabolic proteins lead to many neurodegenerative diseases through the accumulation of misfolded TAU proteins called “tauopathies.” Normally, TAU is responsible for microtubule attachment and cytoskeleton stability in the brain (Petrozziello et al., 2022). Dysregulations of the cytoskeletal network, mitochondrial localization, and homeostasis are involved pathways in the initial steps for the development of neurodegeneration. In ALS, mitochondrial dysfunction increases mtROS and decreases ATP production. Dysregulation of mitochondrial proteins that impair fission, fusion, and mitophagy is responsible for disruptions of axonal transport and defects of the cytoskeletal organization (Petrozziello et al., 2022). Furthermore, mitochondrial dysfunction leads to impaired binding of motor proteins to microtubules, altered activities of kinases, and destabilization of the motor cargo binding. ALS further shows dysregulated PKN1 activity (Petrozziello et al., 2022). The serine/threonine-protein kinase N1 encoded in the PKN1 gene is a regulator of synaptic transmission and maturation. PKN1 elevates excitatory amino acid transporter-3 (EAAT3) and other glutamate transporters. The resulting excitotoxic levels of glutamate disrupt the axonal trafficking of neurofilaments and may contribute to irreversible neurodegeneration (Petrozziello et al., 2022).
Mitochondrial dysfunction is also seen as a primary cause of AD. The brains of patients with AD show impaired glucose and oxygen metabolism and impaired activity of pyruvate dehydrogenase, ketoglutarate dehydrogenase, and cytochrome oxidase. Furthermore, mtDNA alterations, dysfunctional transmembrane amyloid beta, and tau protein accumulation are found, together with altered mitochondria morphology and respiratory chain dysfunction (Chen et al., 2021a; Castora et al., 2022; Rey et al., 2022).
Myalgic encephalitis (ME)/CFS are two other maladies associated with mitochondrial dysfunction. The two pathologies show common mechanisms with neuroinflammatory diseases, such as depression, fibromyalgia, and migraine. The co-occurrence of depression and pain is 30%–60% (Maletic and Raison, 2009). Some of the shared mechanisms are altered brain activity or morphology in several regions; alterations of the HPA axis; genetic susceptibilities; inflammatory signaling mediated by several cytokines, ROS, NRS, and chemokines; altered neuroglial cell functions (including indolamine dioxygenase and neurotrophic factors); dysregulation in monoamines; increase in substance P; altered galanin and opiate signaling; and excessive excitatory glutamatergic transmission and compromised GABA mediated inhibition (Maletic and Raison, 2009).
ME/CFS pathophysiological changes are strongly dependent on mitochondrial dysfunction. In particular, MAVS could protect patients with CFS from viral-related infections, which is essential in a progressive disease related to severe immune alteration (Rasa et al., 2018). The observed mitochondrial alterations are the suppression of the PDC, thereby lowering the conversion of pyruvate to acetyl-CoA and diminished mitochondrial ATP production from OXPHOS and glycolysis. In addition, the reduction in acetyl-CoA prevents melatonin-mediated cellular and mitochondrial protection. Acetyl-CoA is important for aralkylamine N-acetyltransferase (AANAT) activity, which converts serotonin to N- acetylserotonin (NAS) that is then converted to melatonin by acetyl-serotonin methyltransferase (ASMT) (Anderson and Maes, 2020). Melatonin has immune plus antioxidative effects and optimizes mitochondrial OXPHOS (Acuña-Castroviejo et al., 2017b; Anderson and Maes, 2020; McCarty et al., 2020). Other mitochondrial dysfunction mechanisms in patients suffering from ME/CFS include AMPK-altering protein recycling and fatty acid catabolism, mTORC1, and creatine kinase altering cell cycle and instability across different tissue and cell types (Khan et al., 2017; Missailidis et al., 2020). Heightened lactate production is evident in muscles, serum, and cerebrospinal fluid of ME/CFS patients following exercise, indicating significant dysregulation in exercise-induced metabolic activity, including an accelerated switching to aerobic glycolysis, increasing lactate production and suppressing ATP levels (Anderson and Maes, 2020).
Neuroinflammation is an important mechanism related to pain syndromes such as fibromyalgia and CFS. Various pathways are associated with neuroinflammation, including increased oxidative stress, peripheral inflammation, and changes in the gut microbiome. The long-lasting neuroinflammation leads to sensitization of the CNS, with chronic pain as a consequence (Chen et al., 2018; Brain et al., 2021; Chávez-Castillo et al., 2021).
Neuroinflammation in the CNS is mediated by neuroglia cells, found in high numbers in the brain and spinal cord. Glial cells (oligodendrocytes, astrocytes, microglia, and ependymal cells) can be activated by inappropriate dietary patterns, another possible cause of neuroinflammation. The most relevant diet-related risk factors for Glia-cell induced neuroinflammation are the imbalance of anti-inflammatory fatty acids (omega 3) and pro-inflammatory fatty acids (omega 6), energy-dense diets (excess sugar), a diet poor in micronutrients (vitamins and minerals), and diets low in polyphenols (Chen et al., 2018; Brain et al., 2021; Chávez-Castillo et al., 2021).
Microglia are macrophages residing in the CNS and responsible for the regulation of homeostasis. They interact dynamically with synapses and induce synaptic pruning in stages of healthy brain development. The pro-inflammatory activity microglia caused by several risk factors in modern life is an essential step in the development of neurodegenerative brain diseases, such as AD, PD, Huntington’s disease, multiple sclerosis, stroke recovery, neuropsychiatric diseases (depression and anxiety), pain, and neurodevelopmental diseases. Glia cells contribute to the pathogenesis of brain diseases through neuroinflammation. The role of mitochondrial dysfunction is central to Glial function and even development (Chen et al., 2018; Chávez-Castillo et al., 2021).
Shifts in mitochondrial metabolism are crucial in the regulation of glial immune cell phenotypes. Perhaps melatonin should be considered the main regulator of glia cell inflammatory activity. Many protective mechanisms against neuroinflammation have been attributed to melatonin functioning. Autocrine melatonin switches immune cells from a pro-inflammatory (M1) to an anti-inflammatory (M2) phenotype and produces a change in reactivity from cellular to humoral (Carrillo-vico et al., 2013; Hardeland et al., 2015; Chen et al., 2020a; Hardeland, 2021; Bitzer-Quintero et al., 2022). Besides the switch in phenotype, melatonin interacts in multiple ways with microglia. It acts as a protector and an antioxidant and, by this means, inhibits processes that cause, promote, or propagate oxidative stress and neurodegeneration, resulting in excitatory overactivity, toxicological aggressions, viral and bacterial infections, and inflammation (Carrillo-vico et al., 2013; Hardeland et al., 2015; Chen et al., 2020a; Hardeland, 2021; Bitzer-Quintero et al., 2022).
Melatonin also has several other mitochondrial mechanisms that interfere with pathways leading to chronic pain. Melatonin can eliminate mitochondrial free radicals and inhibit the activity of mitochondrial nitric oxide synthase. It restores mitochondrial calcium homeostasis, deacetylates and activates mitochondrial SIRT3, improves the integrity of the blood–brain barrier, and counteracts neuroinflammation and glutamate excitotoxicity (Morris et al., 2021). Furthermore, melatonin and its derivatives act as natural electron donors, being, therefore, highly efficient substances against oxidative stress (Manchester et al., 2015; Tan et al., 2015; Reiter et al., 2018; Salehi et al., 2019a).
The activation of the melatonin/PI3K/Akt/Bmal1-axis increases the production of neuroprotective and metabolic factors. Melatonin-induced Bmal1 drives the night-time dampening of immune cells. Under challenging conditions at night, when immune cell activity is required, an increase in pro-inflammatory cytokines suppresses pineal melatonin production, called the immune-pineal axis (Markus et al., 2021).
As already mentioned, dysbiosis is involved in many or all neurological pathologies. Dysbiosis can signal to the CNS through the production of neuromodulators, such as GABA, tryptophan, choline, serotonin, butyrate, and short-chain fatty acids (SCFA) (Rea et al., 2016; Wang and Wang, 2016; Alkasir et al., 2017; Bonaz et al., 2018; Chen et al., 2021b). Dysregulation of the gut–brain axis can contribute to the development of several neurodegenerative, neuropsychiatric, neurodevelopmental, and neuroinflammatory diseases (Mezzelani et al., 2015; Rosenfeld, 2015; Chen et al., 2016; Sampson et al., 2016; Scheperjans, 2016; Cox and Weiner, 2018; Bull-Larsen and Mohajeri, 2019). Gut microbiome-generated metabolites, such as colonic acid, D-lactic acid, D amino acids, and methyl metabolites, can pass the blood–brain barrier and modulate neuronal behavior. In this way, they influence neuronal development and brain mitochondrial dynamics. One positive pathway related to a “healthy” microbiome is via the production of SCFA that can upregulate PGC-1α as a master regulator of mitochondrial biogenesis (Petra et al., 2015; Bastings et al., 2019; Kobayashi, 2019; Theunissen et al., 2021; Taniguchi et al., 2022). Strategies to enhance melatonin production and or exogenous delivery are described in PART III.
4 Part III: Strategies to restore the mitochondria as a therapeutic approach for multiple chronic diseases
Figure 4 Understanding the different components of mitochondrial dynamics and their influence on health and disease enables the implementation of specific and targeted interventions. As mitochondria play a key role in health and disease, maintenance or recovery of mitochondrial function could or even should be considered a root cause prevention and root cause medicine.
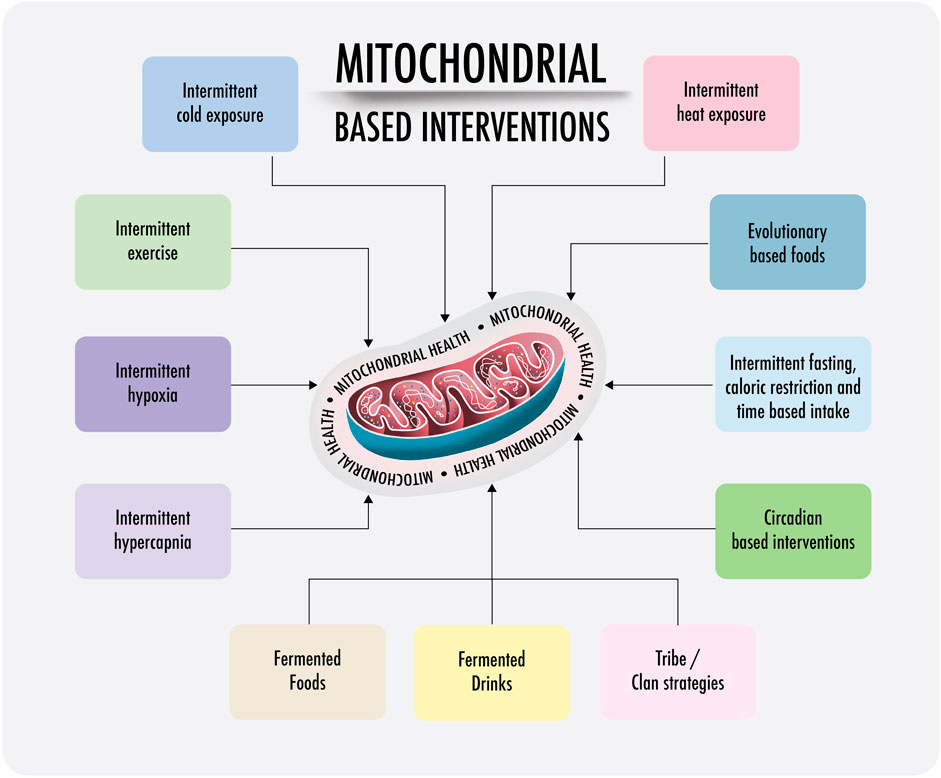
FIGURE 4. Visual representation of proposed strategies improving mitochondrial health: hormesis refers to the evolutionarily conserved adaptive responses of all living organisms to acute/temporary environmental mild physiological stress, nutritional or even voluntary challenges, through which the system modifies its tolerance to more dangerous stressors. Lifestyle interventions based on this principle are also known as hormetic strategies. At the mitochondrial level: mitohormetic strategies. Mitohormesis could support mitochondrial resilience and health, especially preventing CNCDs or premature and failed aging.
A phenomenon called hormesis is important in the maintenance of mitochondrial health. Hormesis refers to the evolutionary conserved adaptive responses of all living organisms to mild environmental, nutritional, or even voluntary challenges, through which the system amends its tolerance to more toxic stress factors (Pruimboom and Muskiet, 2018). Subsequently, treatments and lifestyle interventions based on this principle are also known as hormetic strategies (Lee and Lee, 2019; Bongiovanni et al., 2020; Morris et al., 2021) or mitohormetic strategies at the mitochondrial level. Substances producing hormetic responses are called hormetins (Gezer, 2018; Nunn et al., 2020; Calabrese and Kozumbo, 2021). The application of hormetic strategies and combination thereof might lead to increased lifespan and disease resistance, which is evidenced in different animal studies (Schmeisser et al., 2013; Wang et al., 2019; Mattson, 2008; Berry and López-Martínez, 2020; Proshkina et al., 2020; Calabrese and Kozumbo, 2021). Hormetic triggers follow the adage “what does not kill you makes you stronger” and “if you do not use it, you lose it”; both rules might be an expectation of evolution and even a prerequisite for maintaining (mitochondrial) health.
Hormesis is the result of an upregulation of stress response genes. Triggering or stressing these mitochondrial control systems enables the expression of certain genes and results in the production of protective substances and activation of longevity-related mechanisms (Blagosklonny, 2018; Lee and Lee, 2019; Calabrese and Kozumbo, 2021).
The positive impact of the use of mitohormetic stimuli has been shown in studies on animals in early life. Mitohormetic triggers applied in early life could support mitochondrial resilience and prevent early and unsuccessful aging (Yun and Finkel, 2014; Merry and Ristow, 2016; Bárcena et al., 2018). One of the mitochondrial stress responses is characterized by the induction of specific heat shock protein (HSP) genes in the nucleus and the subsequent effects of the HSPs on mitochondrial functioning, called the mitochondrial unfolded protein response (UPRmt) (Tower, 2015; Inigo and Chandra, 2022). HSPs induce mitophagy and thus influence mitochondrial dynamics that enable the breakdown and replacement of abnormal mitochondria, including those destructed or with mutated mitochondrial genomes (Tower, 2015). The activation of HSPs and the clearance of damaged mitochondria could be a possible solution for heteroplasmy explained earlier in this document. HSPs are involved in immune function, cell cycle regulation, and proteome homeostasis. They facilitate cell function by translocating proteins to other sites within the cell, escorting proteins across cell membranes, stabilizing various proteins and receptors, and identifying and repairing damaged proteins (Brunt and Minson, 2021). HSPs are also associated with other proteins in the ER and in close proximity to the plasma membrane, nucleus, cytosol, or regulation of mitochondrial proteostasis (Brunt and Minson, 2021).
Hormetins induce responses to HSP through the stimulation of kinases and transcription factors (Lee and Lee, 2019; Nunn et al., 2020). Examples of these are the co-transcription factors PGC1α, SIRT1, and SIRT3. They code for proteins that protect mitochondrial dynamics and thus functioning. They are known to prevent the transition from acute to chronic pathologies in kidney disease models (Aparicio-Trejo et al., 2020). Restored expression of PGC-1α in cells ameliorates defective FA oxidation and corrects ATP depletion by reversing changes in damaged tissues through mitochondrial recovery (Fontecha-Barriuso et al., 2020). In addition, mitochondrial protectors and antioxidants, such as N-acetyl cysteine, are frequently used to prevent kidney damage. Their efficacy is shown in models of folic acid-induced kidney damage. They are also a good example of interventions that could support mitochondrial health and induce the production of mitochondrial antioxidants for injury prevention (Aparicio-Trejo et al., 2020; Fontecha-Barriuso et al., 2020; Zhang et al., 2021b).
Hormetic triggers also influence retrograde signaling (nuclear–mitochondrial), a phenomenon discussed earlier. Retrograde signaling influences mitochondrial dynamics, replacement, and health. Important proteins here are HIF1, nuclear factor kappa B (NFkB), PPARs, NRF1, and NRF2. All of these promote the transcription of TFAM and PGC-1α responses. They migrate and bind to target genes, eliciting the expression of cytoprotective molecules (Pruimboom and Muskiet, 2018) and facilitating mitochondrial dynamics and mitochondrial health.
Examples of hormetins as hormetic triggers are exercise, controlled oxidative stress, calory restriction (CR), temperature stressors such as heat and cold, and the use of phytochemicals. These and their relation to mitochondrial health will be discussed hereafter.
4.1 Nutritional strategies: Fasting, phytochemicals, phytomelatonin, and fermented food
4.1.1 Fasting
Fasting is an effective way to recover mitochondrial efficiency, and multiple human studies have shown its ability to restore metabolic pathways in people suffering from chronic pathologies. Fasting regimens are promising primary and secondary prevention strategies in patients suffering from metabolic and cardiovascular diseases (Oliveira et al., 2022; Papakonstantinou et al., 2022; Vasim et al., 2022; Zang et al., 2022), pain, inflammation and immune conditions (Feng et al., 2022; Fitzgerald et al., 2022; Parveen and Alhazmi, 2022), cancer (Lv et al., 2014; Marinac et al., 2016; Taucher et al., 2022; Tiwari et al., 2022), and neurological diseases characterized by altered mood, sleep, pain, and cognition (Phillips, 2019; Brocchi et al., 2022; Caron et al., 2022; Feng et al., 2022; Lobo et al., 2022; Xu et al., 2022).
During nutrient deprivation, the fusion rate increases and the fission rate decreases, leading to increased bioenergetic efficiency (Cuevas-Sierra et al., 2019). CR, another way to create hormetic stress, induces remodeling of the ETC architecture and cristae morphology. This remodeling of the ECT architecture might be driven by ER stress (Balsa et al., 2019). Eukaryotic cells have evolved a conserved pathway called the unfolded protein response aimed to re-establish ER homeostasis. During this process, transcription factor ATF6/ATF4, protein kinase R- (PKR-) like ER kinase (PERK), and inositol-requiring enzyme (IRE1) are activated. These substances can re-establish ER homeostasis and support proper protein folding. A properly functioning ER enables the OXPHOS system to increase ATP supply and promote protein homeostasis (Balsa et al., 2019).
The combination of fasting and exercising can stimulate super complex formation through the ROS/UPR/PERK pathway. Glucose deprivation and its influence on the NADH/FADH2 gradient modulate supercomplexes, enhance mitochondrial respiration, improve all parts of the respiratory chain, and increase cristae density (Balsa et al., 2019; Cogliati et al., 2021)
Studies suggest that fasting supports the balance between the fusion and fission states and homeostasis in the mitochondrial network in multiple cells and organs (Liesa and Shirihai, 2013; Yu and Pekkurnaz, 2018). Research shows an increased expression of mitochondrial fusion-related proteins, MFN 1 and MFN 2. In an experimental model that studied the influence of CR on mitochondrial morphology and dynamics in muscle cells, it was reported that CR reduced fission (Cuevas-Cervera et al., 2022; Xu et al., 2022). CR can induce an activation of the autophagic/mitophagic machinery. As we explain above, fasting induces an ANTI-Warburg effect (Bianchi et al., 2015; Choi et al., 2016; Mattson et al., 2017; de Cabo and Mattson, 2019; Kornberg, 2020; Locati et al., 2020; Cuevas-Cervera et al., 2022) and prevents pathological accumulation or altered proteostasis that are part of the pathophysiology pathways leading to neurological and metabolic pathologies. Protein and biomass accumulation is treatable, improving autophagia, for example, with fasting (Gherardi et al., 2019; Hu et al., 2021; Lechado Terradas et al., 2021; Lizama and Chu, 2021; Roberts and Markby, 2021).
The positive effects of IF and CR and the timing of food intake on mitochondria are related to oxidative stress (Savencu et al., 2021). Numerous studies on animals and humans have shown the beneficial effects of diet interventions on mitochondria-related ROS production. Long-term exposure to CR can reduce not only oxidative stress but also the oxidative damage of the mitochondrial DNA (by 30%). Thus, CR and IF seem able to influence ROS generation and the antioxidative capacity of mitochondria (Cuevas-Cervera et al., 2022; Perez-Montilla et al., 2022).
4.1.2 Phytochemicals
Nuclear erythroid-related factor 2 (NRF2) is a transcription factor encoded by the NFE2L2 gene. Currently, the activation of the NRF2 system is considered a powerful cytoprotective strategy for the treatment of different pathologies, whose pathogenesis is based on oxidative stress, including viral infections, metabolic diseases, and neurological/neuropsychiatric diseases (Bousquet et al., 2020; Bousquet et al., 2021a; Alonso-Piñeiro et al., 2021; Bhandari et al., 2021b; Wang et al., 2021b; Chun et al., 2021; Lee et al., 2021). Phytochemicals seem to reverse conditions involved in extensive lipid peroxidation, protein oxidation and carbonylation, and oxidative damage to nuclear and mitochondrial DNA (Biagiotti et al., 2019; Bhandari et al., 2021a; Morris et al., 2021; Ulasov et al., 2021; Vashi and Patel, 2021).
Optimization of the activity of NRF1 and NRF2 is a very efficient strategy for rehabilitating mitochondrial function. NRF2 activation is inversely related to mtROS generation. It increases PINK/Parkin-mediated mitophagy and removes damaged mitochondria. PGC1α, in collaboration with NRF2, increases mitochondrial biogenesis to supply healthier mitochondria. NRF2 further supports the maintenance of the mitochondrial membrane potential, OXPHOS, ATP synthesis, fatty acid synthesis, and oxidation (Buttari et al., 2022).
Under stress conditions, cells activate the regulatory enhancer sequence of the NRF2 pathway—antioxidant response element (ARE) —to promote the expression of antioxidant genes. This decreases the expression of pro-inflammatory mediators and increases the detoxifying capacity of all cell types. ARE sequences (5′-RTGACnnnGC-3′) are present in more than 200 genes, such as those that express antioxidant enzymes, NAD(P)H quinone oxidoreductase 1 (NQO1), sulfiredoxin 1 (SRXN1), glutathione S-transferase (GST), health-preserving red blood cells heme oxygenase-1 (HMOX1), multidrug resistance-associated proteins (MRP), and UDP-glucuronosyltransferase (UGT) (Buttari et al., 2022).
Phytochemicals are involved in the regulation of NRF2. They are plant antinutrients and are present in the human diet. There are thousands of different phytochemicals in commonly consumed plants. These phytochemicals can be subdivided into four classes based on their chemical structure: phenols and polyphenols, terpenoids, alkaloids, and sulfur-containing compounds (Clifford et al., 2021).
Multiple dietary phytochemicals are useful in regulating NRF and the subsequent activation of cytoprotective effects (Cuadrado et al., 2019). Some useful phytochemicals investigated are naringenin in citrus fruits and tomatoes (Lim et al., 2013). Another example is sulforaphane present in broccoli, brussels sprouts, cabbage, and cauliflower (Santín-Márquez et al., 2019; Cardozo et al., 2021; Kiser et al., 2021; Liebman and Le, 2021) and diallyl disulfide in allium plants, such as garlic and its family members (Arzanlou, 2016; Silvestris et al., 2019; Bhatwalkar et al., 2021; Jayasuriya et al., 2021).
One very powerful phytochemical is resveratrol. It modulates inflammation, improves redox balance, controls cell death and survival, mobilizes kinases, changes mitochondrial function, regulates autophagy, and affects multiple receptors, transcription factors, and ion channels, depending on the dose (Cicero and Colletti, 2015; Brown, 2017; Shetty et al., 2018; Nunn et al., 2020; Li et al., 2021). Resveratrol improves parameters in metabolic and neurodegenerative diseases by increasing antioxidative capacity via the NRF2/HO-1 pathway (Yadav et al., 2022).
Curcumin is present in turmeric root. It exhibits anti-inflammatory, antioxidant (by the action of NRF2), and anticancer activity. Various analgesic mechanisms induced by curcumin effectively attenuate pain by modulating pain-related neurotransmitters by modulating the immune response or blocking transient receptor potential vanilloid type I (TRPV1), as well as modulating purinergic receptors and chemokines, which has been shown in animal and human studies (Lim et al., 2013; Yang et al., 2014; Lv et al., 2015; Wang et al., 2016; Sato et al., 2017; Teymouri et al., 2017; Fan et al., 2018; Liu et al., 2018; Ghasemi et al., 2019; Nunn et al., 2020; Clifford et al., 2021; Jayasuriya et al., 2021; Cianciulli et al., 2022; Dasuni Wasana et al., 2022).
Quercetin is a ubiquitous plant pigment (flavonoid). It is found in many plants and foods, such as onions, green tea, apples, berries, Ginkgo biloba, St. John’s wort, elderberry, and buckwheat tea. Meta-analyses show significant positive results with regard to physical resistance, lower mortality, fewer complications from respiratory viral infections, reduction in pro-inflammatory cytokines and chemokines, a decrease in the amount of ROS, and optimization of mucus production. Quercetin efficiently reduces triglycerides at doses greater than 50 mg/day. It has widely studied neuroprotective effects against neurotoxic chemicals and can also prevent the progression and development of neuronal injury and neurodegeneration (Kressler et al., 2011; Serban et al., 2016; Shi and Williamson, 2016; Sahebkar, 2017; Ho et al., 2018; Bousquet et al., 2020; Nunn et al., 2020; Zhang et al., 2020; Alasmari, 2021; Bhat, 2021; Brito et al., 2021; Ghafouri-Fard et al., 2021; Pinheiro et al., 2021; Soofiyani et al., 2021; Yi et al., 2021).
Ginsenosides in ginger roots in AD models activate NRF2 and suppress ROS/ASK-dependent mitochondrial apoptosis (Liu et al., 2019). Ginsenoside Rd inhibits proliferation and reverses resistance to cisplatin in cancer models (Chian et al., 2019). Pinostrobin protects against neurotoxins by activating NRF2/ARE in Parkinson’s models and ellagic acid in AD models. It has a neuroprotective effect through the NF-κb/NRF2/TLR4 pathway (Grilc et al., 2021).
The use of NAC, coenzyme Q10, resveratrol, and melatonin also increases the activity of NRF2 (Oppedisano et al., 2020; Morris et al., 2021). For improving brain health in neurological disorders, the intake of NAC is proposed and is associated with better brain mitochondrial function and decreased oxidative stress, reduced neuroinflammation, relief of ER stress, and regulation of the unfolded protein response (Morris et al., 2021). Q10 acts as a superoxide scavenger in neuroglial mitochondria, instigates mitohormesis, enhances lipid peroxidation in the IMM, activates uncoupling proteins, promotes mitochondrial biogenesis, is important to restore mitochondrial damage after statins consumption, and has positive effects on the plasma membrane redox system (Lee et al., 2013; Banach et al., 2015; Mohammadi-Bardbori et al., 2015; Morris et al., 2021; Pradhan et al., 2021; Rauchová, 2021).
4.1.3 Melatonin: Phytomelatonin
Melatonin can eliminate mitochondrial free radicals, inhibit mitochondrial nitric oxide synthase, restore mitochondrial calcium homeostasis, and further deacetylate and activate mitochondrial SIRT3 (Galano et al., 2018; Morris et al., 2021). Melatonin improves PGC-1α, Mfn2, and mitochondrial parameters in an animal model of fibromyalgia (Favero et al., 2019). Melatonin enhances the integrity of the blood–brain barrier and the integrity of the intestines and counteracts neuroinflammation and glutamate excitotoxicity (Morris et al., 2021).
We described the functions of melatonin as a mitochondrial protector in an earlier section. Blood melatonin concentrations increase significantly after the consumption of food containing melatonin (Salehi et al., 2019a). Melatonin can be found as a bioavailable substance in many foods. Animal products such as fish and eggs are relatively rich sources of melatonin (Meng et al., 2017a). In plant foods, nuts are an interesting source of melatonin. Mushrooms, berries, cherries, tomatoes, and cucumbers, among many other fruits and vegetables, also contain melatonin (Meng et al., 2017b; Salehi et al., 2019a). Studies on 108 herb species have revealed the presence of melatonin in concentrations ranging from a few to several thousand nanograms per gram of tissue, meaning they are potentially good natural sources of this molecule (Salehi et al., 2019b). Melatonin has also been detected and quantified in roots, shoots, leaves, flowers, fruits, and seeds, but its highest levels have been found in seeds (Salehi et al., 2019b). In the scientific literature, the term phytomelatonin sometimes appears, mainly applied to refer to endogenous melatonin synthesized by plants. Interestingly, in medicinal plants, including pyrethrum maruna (Tanacetum parthenium L.) and Hypericum perforatum L., melatonin levels are sometimes higher than those found in animals (Salehi et al., 2019b)
4.1.4 Fermented food items
As described earlier, intestinal permeability and dysbiosis are risk factors for mitochondrial dysfunction. One simple strategy to improve our gut microbial diversity and intestine integrity is the consumption of fermented food items (Tamang et al., 2016; Dimidi et al., 2019; Melini et al., 2019; Stiemsma et al., 2020). The ability of fermented foods to prevent disease may be related to the number of beneficial microbes, high content of sulforaphane (in, e.g., cabbage and its family), and capsaicinoids (a spicy substance in red pepper powder) (Kwak et al., 2014; Patra et al., 2016; Kim et al., 2018; Woo et al., 2018; Park et al., 2019; Han et al., 2020; Cantadori et al., 2022). The fermentation process increases the number of phenolic compounds (Le et al., 2020) and is a good source of micronutrients, GABA, and amino acids (Lin, 2013; Tamang et al., 2016; Lee et al., 2018; Bousquet et al., 2021b). Many active substances in fermented food items possess potent antibacterial, anticancer, and immune-enhancing capacities (Lin, 2013; Tamang et al., 2016; Lee et al., 2018; Bousquet et al., 2021b). Fermented vegetables and spices are NRF2-induced enzyme agonists. The synergy between fermented food items and spices shows activation effects on NRF2 and a potent antioxidative effect. At the same time, spicy foods are likely to desensitize TRP channels and act in synergy with exogenous polyphenols and flavonoids in plants that activate the NRF2 pathway (McKenzie et al., 2017; Kim et al., 2018; Lee et al., 2018; Woo et al., 2018; Dimidi et al., 2019; Park et al., 2019; Bousquet et al., 2020). Undoubtedly, the most important feature of kimchi, a fermented food item, is its extraordinary effect with regard to the improvement of the gut microbiome, improving the absorption and production of substances essential for health by the bacteria in the gastrointestinal system (Dimidi et al., 2019; Han et al., 2020; Stiemsma et al., 2020).
4.2 Exercise
Contraction of skeletal muscle during exercise activates many hormetic pathways, including improved nutrient sensor AMP kinase activity, mTOR regulation, intracellular PI3K-Akt activity, transcriptional PGC-1α, and sirtuin 1 signaling. All these mechanisms orchestrate the adaptions involved in response to exercise (Foster et al., 2015; Merry and Ristow, 2016; Roberts and Markby, 2021; Magaña et al., 2022). Exercise improves macro-autophagic and lysosomal activity and protects the balance between mitochondrial biogenesis, mitochondrial dynamics, and mitophagy (Sorriento et al., 2021). Mitochondrial response to exercise follows multiple mechanisms (Tian et al., 2022): increase in mitochondrial biogenesis (Wallace, 2015); improvement of expression and action of proteins involved in the mitochondrial dynamics through mitofusins (fusion and fission proteins, promoting a more fused, tumular network) such as OPA1 (Navarro-Ledesma et al., 2022); increase in mitochondrial turnover by the action of proteins related to autophagy and mitophagy (e.g., PINK1, parkin, nix, and Bnip3) and (Pruimboom et al., 2016) quality control supporting mechanisms through the degradation of damaged or dysfunction mitochondria; and mitochondrial cristae remodeling or shaping (Ruiz-Núñez et al., 2013), improving chain complexes and mitochondrial respiratory efficiency (Roberts and Markby, 2021; Sorriento et al., 2021; Lv et al., 2022). Exercise impacts the stoichiometry of the SCs, enhancing the efficiency of electron flux by segmentation of the CoQ pool, improving the stability of the individual respiratory complexes, and avoiding ROS excess (by CI and CIII) (Huertas et al., 2021; Roberts and Markby, 2021). Theoretically, exercise per its capacity to create ROS as a signaling molecule could also impact signaling pathways linked to quality and quantity control of mitochondria (Barbieri et al., 2015; Axelrod et al., 2019). Training volume strongly correlates positively with changes in mitochondrial volume. Therefore, a mix between high-intensity interval training (HIIT) and endurance training seems important (Bishop et al., 2014; Barbieri et al., 2015; Stawski et al., 2017).
4.3 Cold exposure
Cold exposure promotes increased expression of mitochondrial biogenesis. The use of short-lasting cold triggers has been shown to increase the number and activity of mitochondria and protection against oxidate damage (Saltykova, 2019). The influence of cold exposure on mitochondria has been mentioned in relation to the so-called browning of adipose tissue: the transition of the white adipose tissue into a beige or brown adipose tissue (BAT) (Saito et al., 2009). When white adipocytes are re-programmed to beige adipocytes, they show an increase in mitochondria. PGC-1α is considered the link in the transition from external physiological stimuli (e.g., cold) to an internal metabolic response, such as mitochondrial biogenesis (Ihsan et al., 2021). Cold exposure activates UCP1-mediated thermogenesis in adipose tissue, and UCP-1 is mainly found in adipocytes of BAT. Uncoupling proteins play a vital role in regulating mitochondrial membrane potential, preventing ROS production, and regulating calcium homeostasis, amongst others (Saito et al., 2009; Chung et al., 2017; Okamatsu-Ogura et al., 2020).
4.4 Heat exposure
Heat is typically used to improve heat tolerance, although it has also been shown to improve physical performance (Waldron et al., 2021).
Heat exposure is often investigated using a traditional wood, electric, or infrared sauna. Some heaters heat the air by raising the temperature. Traditional saunas use temperatures of 70°C–100°C. Infrared heaters emit thermal radiation, which heats the body directly using 45°C–60°C. Dry sauna humidity is 10%–20%, whereas the humidity levels in steam saunas are above 50% (Patrick and Johnson, 2021).
Exposure to high temperatures is a hormetic stress stimulus, a robust response that mainly affects the skin and the cardiovascular system. Cardiac output can increase by as much as 60%–70%. During sauna exposure, 50%–70% of the body’s circulation is redistributed to the skin to facilitate sweating, resulting in fluid losses at a rate of approximately 0.6–1.0 kg/h through sweating to cool the body and avoid rapid increases in its core temperature. It also detoxifies and eliminates heavy metals (Patrick and Johnson, 2021).
The molecular response to heat includes increased expression of HSPs, transcriptional regulators, such as NRF2, and pro- and anti-inflammatory factors (Patrick and Johnson, 2021).
In cancer models, at temperatures of 40°C, one sees an increase in the expression of the transcription factor protein NRF2. The same holds for SOD, catalase, heme oxygenase-1, glutamate cysteine ligase, and HSP70 (Glory and Averill-Bates, 2016). Increased NRF2 expression and catalase activity at 40°C were inhibited by the antioxidant PEG-catalase and the p53 inhibitor pifithrin-α, suggesting that mild thermotolerance (40°C) increases prooxidant levels, which in turn activates NRF2 (Glory and Averill-Bates, 2016).
Chronic high temperatures can cause the inactivation of protein synthesis and DNA repair processes. In that situation, cells die by apoptosis and/or necrosis or become sensitized to other cytotoxic modalities, such as radiation. Intermittent hyperthermia (41°C–45°C) activates apoptosis through the mitochondrial death receptor and ER pathways. Training cells at elevated temperatures induces thermotolerance (Glory et al., 2014). Both exercise and sauna use raise core body temperature and acutely increase plasma IL-6 and IL-10 levels. IL-6 exerts anti-inflammatory properties through the activation of IL-10 (Patrick and Johnson, 2021).
4.5 Breathing techniques
Many other strategies based on evolutionary challenges have been investigated. In particular, the strategies that involve gas changes, respiratory changes, or respiratory techniques, such as pranayama, are promising as interventions for primary and perhaps secondary prevention for the development of chronic diseases.
Studies on participants engaging in pranayama breathing have shown psychological and physiological benefits in cancer, cardiovascular diseases, and mainly pulmonary diseases (Jayawardena et al., 2020). Pranayama breathing also shows positive effects with regard to the improvement of exercise tolerance in patients with pulmonary diseases (Kaminsky et al., 2017). Similar effects are also shown in patients with asthma, improving their quality of life (Erdoğan Yüce and Taşcı, 2020; Das et al., 2022). Yoga respiratory practice significantly reduces the levels of anxiety and negative affect. Its effects are thought to be caused by the modulation of activity and connectivity in brain areas involved in attention, awareness, and emotion processing measured with functional MRI (Novaes et al., 2020). Slow types of yoga breathing techniques show beneficial effects on cardiovascular and autonomic variables, unlike fast breathing (Nivethitha et al., 2016). Different techniques will produce different effects, although the mechanisms are not yet fully understood (Nivethitha et al., 2016). Moreover, intermittent hypobaric hypoxia (IHH) and endurance training (ET) are protective strategies for improving stress resistance. Again, mitochondrial modulation is an important step in this process (Magalhães et al., 2013).
After hypoxia exposure, animal models show mitochondrial-resistant phenotypes and a protective hypoxia response against mitochondrial toxicity (Jain et al., 2016). Intermittent hypoxia training (IHT) in animal models significantly improved OXPHOS and α-ketoglutarate oxidation (Kurhaluk et al., 2013). Moreover, IHT reorganizes the mitochondrial energy metabolism in the liver (Kurhaluk et al., 2013). IHT induces an adaptative specific type of mitochondrial mitosis in cardiac and lung tissue depending on the duration (Rozova and Mankovska, 2012). IHT is often investigated in 20-day programs of 5–8 daily cycles of 5–10 min moderate intense hypoxia (9.5%–10% O2) and 4 min exposures to normoxia (21% O2), with each daily session totaling 45–98 min (Jung and Mallet, 2018). Proven cardiovascular function improvements are shown, as well as increases in resistance against toxins. It is further proposed as a powerful, non-invasive brain protective strategy (Jung and Mallet, 2018). Acute intermittent hypoxia (AIH) is one of the most promising approaches to improve recovery after spinal cord injury and other diseases with motoneurons alterations due to its effects on neuronal plasticity through increasing VEGF, brain-derived neurotrophic factor (BDNF), and phosphorylated and non-phosphorylated forms of the BDNF receptor tropomyosin-related kinase B (TrkB) (Hassan et al., 2018; Vose et al., 2022). AIH combined with task-specific training synergistically improves motor function (Welch et al., 2020). AIH improves cognitive and mitochondrial functions and protects against cerebrovascular malfunction in neurological diseases (Manukhina et al., 2016; Serebrovska et al., 2019; Burtscher et al., 2021).
5 Mitochondrial health, mitochondrial dynamics, mitophagy, and biogenesis
5.1 Mitochondrial health
The opposite of disease is health. Understanding what mitochondrial health means and how to maintain mitochondrial health enables the consistent execution of a myriad of functions and thus contributes to prevention, care, and cure in times of pathologies.
Mitochondria are highly dynamic organelles that undergo frequent structural and metabolic changes to fulfill cellular demands. Mitochondrial dynamics depend mainly on mitochondrial fission, fusion, biogenesis, and mitophagy. These processes coordinate the control of mitochondrial morphology, quantity, quality, turnover, and inheritance (Yapa et al., 2021; Green et al., 2022). Maintenance of both mitochondrial quantity and quality is strictly related to the conservation of an adequate concentration of several proteins, such as PGC-1α, TFAM, mitochondrial cristae biogenesis protein optic atrophy 1 (Opa1), Drp1, Mitofusin 1 (Mfn1), Mitofusin 2 (Mfn2), mitochondrial fission protein 1 (FIS1), PINK1, Parkin, VDAC1, Bnip3, and Nix, reunited in the process of proteostasis (Moreira et al., 2017; Wiese and Bannister, 2020; Lima et al., 2022).
5.2 Mitochondrial dynamics: Fusion and fission
A fine-tuned balance needs to exist between fusion and fission (Green et al., 2022). Fusion means the physical merging of mitochondrial segments of two originally distinct mitochondria. Amongst others, it takes care of the replenishment of damaged mitochondrial DNA. The main proteins responsible are Mfn1, Mfn2, and OPA1 in IMM and lipid components (e.g., cardiolipin) (Adebayo et al., 2021).
Conversely, fission is a division of mitochondria performed in the ER. It is required for mitochondrial motility and mitochondrial inheritance in the G2/M phase of the cell cycle. It influences the regulation of mitochondrial size and shape and the distribution of mitochondria throughout the cell body. The main proteins involved in fission are dynamin-related protein 1 (DRP1), dynamin-1 (DNM1), DNM2, and FIS1 (Quiles and Gustafsson, 2022). Some studies have found that upon aging, mitochondria tend to be more fragmented, suggesting that fusion is decreased and/or fission is increased (Wang et al., 2019). Fused mitochondria have indeed been suggested as being metabolically more active than fragmented mitochondria, as cells with a fused mitochondrial network seem to have a higher respiratory rate than cells with fragmented mitochondria (Westermann, 2012).
5.3 Mitophagy and biogenesis
Mitochondrial biogenesis, a cellular process enabling the production of new mitochondria, is mediated by different activators, regulators, and transcription factors, such as PGC-1a and NRF2. Damaged mitochondria are removed via a selective autophagosomal process called mitophagy. The major regulator for this is PGC1 (Ma et al., 2020; Choubey et al., 2021; Terešak et al., 2022). Aging has been associated with decreased mitophagy capacity and mitochondrial biogenesis, leading to the accumulation of mitochondrial damage (Chen et al., 2020b).
Although mitochondria are a source of ROS, they are vulnerable to oxidative stress. Therefore, endogenous anti-oxidative systems play an important role in cell survival under physiological and pathological conditions. NRF2 is important in this defense. The NRF2/ARE signaling pathway affects almost all mitochondrial processes and is, therefore, an important target in the protocols proposed to improve mitochondrial health (Khan et al., 2021b; Chen, 2021; Gilardini Montani et al., 2021; Herengt et al., 2021; Liebman and Le, 2021; Matsumaru and Motohashi, 2021; Syed et al., 2021; Thiruvengadam et al., 2021; Ulasov et al., 2021; Zgorzynska et al., 2021; Zhao et al., 2021). In order to cope with deleterious effects, mitochondria feature different mechanisms for quality control. One such mechanism is the mitochondrial unfolded protein response (UPRmt), which corresponds to the transcriptional activation of mitochondrial chaperones, proteases, and antioxidant enzymes to repair defective mitochondria (Inigo and Chandra, 2022).
Interventions explained above could serve the purpose of mitochondria anatomical and functional maintenance. This review describes a series of possible treatment options as primary and secondary preventive interventions in mitochondria dysfunction-related disorders and diseases.
6 Conclusion
Modern life has come with novel risk factors. Although our adapted brain produced the modern and novel environment, our immune system and metabolism have not yet adapted to those new circumstances. Many novel risk factors, such as sitting time, lack of physical activity, food abundance, sleep disturbances, and lack of micronutrients, and environmental factors, such as pollution, can produce severe mitochondrial stress, damage, and dysfunction. The metabolic disturbances caused by mitochondrial dysfunction and damage caused by the aforementioned risk factors are hallmarks of most, if not all, of the metabolic, immune, neurological, and infectious diseases and CNCDs in general. Risk factors and consequences can be prevented and/or treated, considering that one of the main pathways is the switch from OXPHOS to glycolysis directed by mTOR. The chronic Warburg effect leads to proliferative, inflammatory, or fibrotic states, increasing the intracellular abundance of biomass. In this study, we describe the immuno-metabolic mechanism that impedes healthy OXPHOS. Evolutionary medicine provides us with mitohormetic strategies. These are simple, low-risk, and economically cheap strategies based on enhancing human molecular responses through epigenetic mechanisms. Intermittently applied and in the right combination, they provide powerful tools to facilitate mitochondrial health through several pathways. As mitochondrial functioning is essential in understanding health and disease, the use of hormetic interventions, protecting or recovering normal mitochondrial functioning, could result in widespread health benefits and possibly be effectual in primary and secondary chronic disease prevention. These so-called hormetic strategies support immune system efficiency and an anti-inflammatory phenotype, along with metabolic and neurological plasticity. Avoiding metabolic glycolytic states and switching to OXPHOS is especially described in fasting (an anti—Warburg strategy). Other promising hormetic strategies are exposure to intermittent therapeutic cold or heat. Nutrition as medicine with regard to mitochondrial health is evidenced by numerous studies related to the beneficial effects of phytochemical-rich food and fermented food items. Breathing techniques, including therapeutic intermittent hypercapnia and hypoxia, can even serve as quick wins in people suffering from pain and fatigue. All these robust strategies trigger evolutionarily conserved mechanisms, which, in turn, make homo sapiens more resilient and thus resistant to the toxic effects of modern life. Mechanisms behind each hormetic trigger strategy are not fully understood, and further research is needed, not only in vitro and in vivo, but also in clinical settings with humans.
Author contributions
Conceptualization: AC, AW, SN-L, and LP. Methodology: AC and AW. Investigation: AC, AW, SN-L, and LP. Visualization: AC, AW, SN-L, and LP. Funding acquisition: SN-L and LP. Project administration: SN-L and LP. Supervision: SN-L and LP. Writing original draft: AC, AW, SN-L, and LP. Writing—review and editing: AC, AW, SN-L, and LP.
Funding
The study was funded by the chair of Clinical Psychoneuroimmunology (the University of Granada and PNI Europe)
Conflict of interest
LP is the head of PNI Europe and teaches about this research line. The remaining authors are also part of the PNI Europe team.
The remaining authors declare that the research was conducted in the absence of any commercial or financial relationships that could be construed as a potential conflict of interest.
Publisher’s note
All claims expressed in this article are solely those of the authors and do not necessarily represent those of their affiliated organizations or those of the publisher, the editors, and the reviewers. Any product that may be evaluated in this article, or claim that may be made by its manufacturer, is not guaranteed or endorsed by the publisher.
References
Abbott, S. M., Malkani, R. G., and Zee, P. C. (2020). Circadian disruption and human health: A bidirectional relationship. Eur. J. Neurosci. 51 (1), 567–583. doi:10.1111/ejn.14298
Acuña-Castroviejo, D., Rahim, I., Acuña-Fernández, C., Fernández-Ortiz, M., Solera-Marín, J., Sayed, R. K. A., et al. (2017). Melatonin, clock genes and mitochondria in sepsis. Cell Mol. Life Sci. 74 (21), 3965–3987. doi:10.1007/s00018-017-2610-1
Acuña-Castroviejo, D., Rahim, I., Acuña-Fernández, C., Fernández-Ortiz, M., Solera-Marín, J., Sayed, R. K. A., et al. (2017). Melatonin, clock genes and mitochondria in sepsis. Cell. Mol. Life Sci. 74 (21), 3965–3987. doi:10.1007/s00018-017-2610-1
Adebayo, M., Singh, S., Singh, A. P., and Dasgupta, S. (2021). Mitochondrial fusion and fission: The fine-tune balance for cellular homeostasis. FASEB J. 35, e21620. doi:10.1096/fj.202100067R
Aguilar-López, B. A., Moreno-Altamirano, M. M. B., Dockrell, H. M., Duchen, M. R., and Sánchez-García, F. J. (2020). Mitochondria: An integrative hub coordinating circadian rhythms, metabolism, the microbiome, and immunity. Front. Cell Dev. Biol. 8, 51. doi:10.3389/fcell.2020.00051
Akdis, C. A. (2021). Does the epithelial barrier hypothesis explain the increase in allergy, autoimmunity and other chronic conditions? Nat. Rev. Immunol. 21 (11), 739–751. doi:10.1038/s41577-021-00538-7
Al Amir Dache, Z., Otandault, A., Tanos, R., Pastor, B., Meddeb, R., Sanchez, C., et al. (2020). Blood contains circulating cell-free respiratory competent mitochondria. FASEB J. 34 (3), 3616–3630. doi:10.1096/fj.201901917RR
Alasmari, A. F. (2021). Cardioprotective and nephroprotective effects of Quercetin against different toxic agents. Eur. Rev. Med. Pharmacol. Sci. 25 (23), 7425–7439. doi:10.26355/eurrev_202112_27440
Alavi, M., Farkhondeh, T., Aschner, M., and Samarghandian, S. (2021). Resveratrol mediates its anti-cancer effects by Nrf2 signaling pathway activation. Cancer Cell Int. 21 (1), 579. doi:10.1186/s12935-021-02280-5
Alfarouk, K. O., Alhoufie, S. T. S., Hifny, A., Schwartz, L., Alqahtani, A. S., Ahmed, S. B. M., et al. (2021). Of mitochondrion and COVID-19. J. Enzyme Inhib. Med. Chem. 36 (1), 1258–1267. doi:10.1080/14756366.2021.1937144
Alhayaza, R., Haque, E., Karbasiafshar, C., Sellke, F. W., and Abid, M. R. (2020). The relationship between reactive oxygen species and endothelial cell metabolism. Front. Chem. 8, 592688. doi:10.3389/fchem.2020.592688
Alhebshi, H., Tian, K., Patnaik, L., Taylor, R., Bezecny, P., Hall, C., et al. (2021). Evaluation of the role of p53 tumour suppressor posttranslational modifications and ttc5cofactor in lung cancer. Int. J. Mol. Sci. 22 (24), 13198. doi:10.3390/ijms222413198
Alkasir, R., Li, J., Li, X., Jin, M., and Zhu, B. (2017). Human gut microbiota: The links with dementia development. Protein Cell 8 (2), 90–102. doi:10.1007/s13238-016-0338-6
Alonso-Piñeiro, J. A., Gonzalez-Rovira, A., Sánchez-Gomar, I., Moreno, J. A., and Durán-Ruiz, M. C. (2021). Nrf2 and heme oxygenase-1 involvement in atherosclerosis related oxidative stress. Antioxidants (Basel) 10, 1463. doi:10.3390/antiox10091463(9)
Anderson, G., and Maes, M. (2020). Mitochondria and immunity in chronic fatigue syndrome. Prog. Neuropsychopharmacol. Biol. Psychiatry 103, 109976. doi:10.1016/j.pnpbp.2020.109976
Andrieux, P., Chevillard, C., Cunha-Neto, E., and Nunes, J. P. S. (2021). Mitochondria as a cellular hub in infection and inflammation. Int. J. Mol. Sci. 22 (21), 11338. doi:10.3390/ijms222111338
Annie-Mathew, A. S., Prem-Santhosh, S., Jayasuriya, R., Ganesh, G., Ramkumar, K. M., and Sarada, D. V. L. (2021). The pivotal role of Nrf2 activators in adipocyte biology. Pharmacol. Res. 173, 105853. doi:10.1016/j.phrs.2021.105853
Annunziata, I., Sano, R., and d’Azzo, A. (2018). Mitochondria-associated ER membranes (MAMs) and lysosomal storage diseases. Cell Death Dis. 9 (3), 328. doi:10.1038/s41419-017-0025-4
Aparicio-Trejo, O. E., Avila-Rojas, S. H., Tapia, E., Rojas-Morales, P., León-Contreras, J. C., Martínez-Klimova, E., et al. (2020). Chronic impairment of mitochondrial bioenergetics and β-oxidation promotes experimental AKI-to-CKD transition induced by folic acid. Free Radic. Biol. Med. 154, 18–32. doi:10.1016/j.freeradbiomed.2020.04.016
Arzanlou, M. (2016). Inhibition of streptococcal pyrogenic exotoxin B using allicin from garlic. Microb. Pathog. 93, 166–171. doi:10.1016/j.micpath.2016.02.010
Ashton, A., Foster, R. G., and Jagannath, A. (2022). Photic entrainment of the circadian system. Int. J. Mol. Sci. 23 (2), 729. doi:10.3390/ijms23020729
Axelrod, C. L., Fealy, C. E., Mulya, A., and Kirwan, J. P. (2019). Exercise training remodels human skeletal muscle mitochondrial fission and fusion machinery towards a pro-elongation phenotype. Acta Physiol. (Oxf). 225 (4), e13216. doi:10.1111/apha.13216
Baek, Y., Woo, T. G., Ahn, J., Lee, D., Kwon, Y., Park, B. J., et al. (2022). Structural analysis of the overoxidized Cu/Zn-superoxide dismutase in ROS-induced ALS filament formation. Commun. Biol. 5, 1085. doi:10.1038/s42003-022-04017-0
Balestri, F., Moschini, R., Mura, U., Cappiello, M., and del Corso, A. (2022). In search of differential inhibitors of aldose reductase. Biomolecules 12, 485. doi:10.3390/biom12040485
Balsa, E., Soustek, M. S., Thomas, A., Cogliati, S., García-Poyatos, C., Martín-García, E., et al. (2019). ER and nutrient stress promote assembly of respiratory chain supercomplexes through the PERK-eIF2α Axis. Mol. Cell 74 (5), 877–890. doi:10.1016/j.molcel.2019.03.031
Banach, M., Serban, C., Sahebkar, A., Ursoniu, S., Rysz, J., Muntner, P., et al. (2015). Effects of coenzyme Q10 on statin-induced myopathy: A meta-analysis of randomized controlled trials. Mayo Clin. Proc. 90 (1), 24–34. doi:10.1016/j.mayocp.2014.08.021
Barbieri, E., Agostini, D., Polidori, E., Potenza, L., Guescini, M., Lucertini, F., et al. (2015). The pleiotropic effect of physical exercise on mitochondrial dynamics in aging skeletal muscle. Oxid. Med. Cell Longev. 2015, 917085. doi:10.1155/2015/917085
Bárcena, C., Mayoral, P., and Quiros, P. (2018). “Mitohormesis, an antiaging paradigm,” in International review of cell and molecular Biology.
Barrea, L., Savastano, S., di Somma, C., Savanelli, M. C., Nappi, F., Albanese, L., et al. (2017). Low serum vitamin D-status, air pollution and obesity: A dangerous liaison. Rev. Endocr. Metab. Disord. 18 (2), 207–214. doi:10.1007/s11154-016-9388-6
Bastings, J. J. A. J., van Eijk, H. M., Damink, S. W. O., and Rensen, S. S. (2019). D-Amino acids in health and disease: A focus on cancer. Nutrients 11 (9), 2205–2218. doi:10.3390/nu11092205
Bauersfeld, S. P., Kessler, C. S., Wischnewsky, M., Jaensch, A., Steckhan, N., Stange, R., et al. (2018). The effects of short-term fasting on quality of life and tolerance to chemotherapy in patients with breast and ovarian cancer: A randomized cross-over pilot study. BMC Cancer 18 (1), 476. doi:10.1186/s12885-018-4353-2
Bell, S. M., Burgess, T., Lee, J., Blackburn, D. J., Allen, S. P., and Mortiboys, H. (2020). Peripheral glycolysis in neurodegenerative diseases. Int. J. Mol. Sci. 21 (23), 8924. doi:10.3390/ijms21238924
Benayoun, B. A., and Lee, C. (2019). MOTS-c: A mitochondrial-encoded regulator of the nucleus. Bioessays 41 (9), e1900046. doi:10.1002/bies.201900046
Benayoun, B. A., and Lee, C. (2019). MOTS-c: A mitochondrial-encoded regulator of the nucleus. BioEssays 41 (9), 1900046. doi:10.1002/bies.201900046
Berry, R., and López-Martínez, G. (2020). A dose of experimental hormesis: When mild stress protects and improves animal performance. Comp. Biochem. Physiol. A Mol. Integr. Physiol. 242, 110658. doi:10.1016/j.cbpa.2020.110658
Bhandari, R., Kaur, J., Kaur, S., and Kuhad, A. (2021). The Nrf2 pathway in psychiatric disorders: Pathophysiological role and potential targeting. Expert Opin. Ther. Targets 25 (2), 115–139. doi:10.1080/14728222.2021.1887141
Bhandari, R., Khanna, G., Kaushik, D., and Kuhad, A. (2021). Divulging the intricacies of crosstalk between NF-kb and nrf2-keap1 pathway in neurological complications of COVID-19. Mol. Neurobiol. 58 (7), 3347–3361. doi:10.1007/s12035-021-02344-7
Bhat, I. U. H. (2021). Quercetin: A bioactive compound imparting cardiovascular and neuroprotective benefits: Scope for exploring fresh produce, their wastes, and by-products. Biol. (Basel) 10, 586. doi:10.3390/biology10070586(7)
Bhatti, G. K., Gupta, A., Pahwa, P., Khullar, N., Singh, S., Navik, U., et al. (2022). Targeting mitochondrial bioenergetics as a promising therapeutic strategy in metabolic and neurodegenerative diseases. Biomed. J. 45, 733–748. doi:10.1016/j.bj.2022.05.002
Bhatwalkar, S. B., Mondal, R., Krishna, S. B. N., Adam, J. K., Govender, P., and Anupam, R. (2021). Antibacterial properties of organosulfur compounds of garlic (allium sativum). Front. Microbiol. 12, 613077. doi:10.3389/fmicb.2021.613077
Biagiotti, S., Bianchi, M., Rossi, L., Chessa, L., and Magnani, M. (2019). Activation of NRF2 by dexamethasone in ataxia telangiectasia cells involves KEAP1 inhibition but not the inhibition of p38. PLoS One 14 (5), e0216668. doi:10.1371/journal.pone.0216668
Bianchi, G., Martella, R., Ravera, S., Marini, C., Capitanio, S., Orengo, A., et al. (2015). Fasting induces anti-Warburg effect that increases respiration but reduces ATP-synthesis to promote apoptosis in colon cancer models. Oncotarget 6, 11806–11819. doi:10.18632/oncotarget.3688
Bishop, D. J., Granata, C., and Eynon, N. (2014). Can we optimise the exercise training prescription to maximise improvements in mitochondria function and content? Biochimica Biophysica Acta (BBA) - General Subj. 1840 (4), 1266–1275. doi:10.1016/j.bbagen.2013.10.012
Bitzer-Quintero, O. K., Ortiz, G. G., Jaramillo-Bueno, S., Ramos-González, E. J., Márquez-Rosales, M. G., Delgado-Lara, D. L. C., et al. (2022). Psycho-neuro-endocrine-immunology: A role for melatonin in this new paradigm. Molecules 27 (15), 4888. doi:10.3390/molecules27154888
Blagosklonny, M. V. (2013). MTOR-Driven quasi-programmed aging as a disposable soma theory: Blind watchmaker vs. intelligent designer. Cell Cycle 12, 1842–1847. doi:10.4161/cc.25062
Blagosklonny, M. v. (2018). Rapamycin, proliferation and geroconversion to senescence. Cell Cycle 17 (24), 2655–2665. doi:10.1080/15384101.2018.1554781
Bonaz, B., Bazin, T., and Pellissier, S. (2018). The vagus nerve at the interface of the microbiota-gut-brain Axis. Front. Neurosci. 12, 49. doi:10.3389/fnins.2018.00049
Bongiovanni, T., Genovesi, F., Nemmer, M., Carling, C., Alberti, G., and Howatson, G. (2020). Nutritional interventions for reducing the signs and symptoms of exercise-induced muscle damage and accelerate recovery in athletes: Current knowledge, practical application and future perspectives. Eur. J. Appl. Physiol. 120 (9), 1965–1996. doi:10.1007/s00421-020-04432-3
Bonora, M., Patergnani, S., Rimessi, A., De Marchi, E., Suski, J. M., Bononi, A., et al. (2012). ATP synthesis and storage. Purinergic Signal 8 (3), 343–357. doi:10.1007/s11302-012-9305-8
Bosma-Den Boer, M. M., Van Wetten, M. L., and Pruimboom, L. (2012). Chronic inflammatory diseases are stimulated by current lifestyle: How diet, stress levels and medication prevent our body from recovering. Nutr. Metab. (Lond) 9 (1), 32. doi:10.1186/1743-7075-9-32
Bousquet, J., Anto, J. M., Czarlewski, W., Haahtela, T., Fonseca, S. C., Iaccarino, G., et al. (2021). Cabbage and fermented vegetables: From death rate heterogeneity in countries to candidates for mitigation strategies of severe COVID-19. Allergy 76 (3), 735–750. doi:10.1111/all.14549
Bousquet, J., Cristol, J. P., Czarlewski, W., Anto, J. M., Martineau, A., Haahtela, T., et al. (2020). Nrf2-interacting nutrients and COVID-19: Time for research to develop adaptation strategies. Clin. Transl. Allergy 10 (1), 58. doi:10.1186/s13601-020-00362-7
Bousquet, J., Czarlewski, W., Zuberbier, T., Mullol, J., Blain, H., Cristol, J. P., et al. (2021). Potential interplay between Nrf2, TRPA1, and TRPV1 in nutrients for the control of COVID-19. Int. Arch. Allergy Immunol. 182 (4), 324–338. doi:10.1159/000514204
Brain, K., Burrows, T. L., Bruggink, L., Malfliet, A., Hayes, C., Hodson, F. J., et al. (2021). Diet and chronic non-cancer pain: The state of the art and future directions. J. Clin. Med. 10, 5203. doi:10.3390/jcm10215203
Branco, A. F., Ferreira, A., Simões, R. F., Magalhães-Novais, S., Zehowski, C., Cope, E., et al. (2016). Ketogenic diets: From cancer to mitochondrial diseases and beyond. Eur. J. Clin. Invest 46 (3), 285–298. doi:10.1111/eci.12591
Brand, K. (1997). Aerobic glycolysis by proliferating cells: Protection against oxidative stress at the expense of energy yield. J. Bioenergetics Biomembr. 29, 355–364. doi:10.1023/a:1022498714522
Bray, F., Ferlay, J., Soerjomataram, I., Siegel, R. L., Torre, L. A., and Jemal, A. (2018). Global cancer statistics 2018: GLOBOCAN estimates of incidence and mortality worldwide for 36 cancers in 185 countries. CA Cancer J. Clin. 68 (6), 394–424. doi:10.3322/caac.21492
Bray, F., Laversanne, M., Weiderpass, E., and Soerjomataram, I. (2021). The ever-increasing importance of cancer as a leading cause of premature death worldwide. Cancer 127 (16), 3029–3030. doi:10.1002/cncr.33587
Brito, J. C. M., Lima, W. G., Cordeiro, L. P. B., and da Cruz Nizer, W. S. (2021). Effectiveness of supplementation with quercetin-type flavonols for treatment of viral lower respiratory tract infections: Systematic review and meta-analysis of preclinical studies. Phytother. Res. 35 (9), 4930–4942. doi:10.1002/ptr.7122
Brocchi, A., Rebelos, E., Dardano, A., Mantuano, M., and Daniele, G. (2022). Effects of intermittent fasting on brain metabolism. Nutrients 14 (6), 1275. doi:10.3390/nu14061275
Brooks, G. A., Curl, C. C., Leija, R. G., Osmond, A. D., Duong, J. J., and Arevalo, J. A. (2022). Tracing the lactate shuttle to the mitochondrial reticulum. Exp. Mol. Med. 54 (9), 1332–1347. doi:10.1038/s12276-022-00802-3
Brooks, G. A. (2002). Lactate shuttles in nature. Biochem. Soc. Trans. 30 (2), 258–264. doi:10.1042/bst0300258
Brooks, G. A. (2020). The precious few grams of glucose during exercise. Int. J. Mol. Sci. 21 (16), 5733. doi:10.3390/ijms21165733
Brooks, G. A. (2018). The science and translation of lactate shuttle theory. Cell Metab. 27 (4), 757–785. doi:10.1016/j.cmet.2018.03.008
Brooks, G. A. (2020). The tortuous path of lactate shuttle discovery: From cinders and boards to the lab and ICU. J. Sport Health Sci. 9 (5), 446–460. doi:10.1016/j.jshs.2020.02.006
Brown, B. I. (2017). Nutritional management of metabolic endotoxemia: A clinical review. Altern. Ther. Health Med. 23 (4), 42–54.
Brunt, V. E., and Minson, C. T. (2021). Heat therapy: Mechanistic underpinnings and applications to cardiovascular health. J. Appl. Physiol. 130 (6), 1684–1704. doi:10.1152/japplphysiol.00141.2020
Bull-Larsen, S., and Mohajeri, M. H. (2019). The potential influence of the bacterial microbiome on the development and progression of ADHD. Nutrients 11, 2805. doi:10.3390/nu11112805
Burton, J. C., Antoniades, W., Okalova, J., Roos, M. M., and Grimsey, N. J. (2021). Atypical p38 signaling, activation, and implications for disease. Int. J. Mol. Sci. 22 (8), 4183. doi:10.3390/ijms22084183
Burtscher, J., Mallet, R. T., Burtscher, M., and Millet, G. P. (2021). Hypoxia and brain aging: Neurodegeneration or neuroprotection? Ageing Res. Rev. 68, 101343. doi:10.1016/j.arr.2021.101343
Buttari, B., Arese, M., Oberley-Deegan, R. E., Saso, L., and Chatterjee, A. (2022). NRF2: A crucial regulator for mitochondrial metabolic shift and prostate cancer progression. Front. Physiol. 13, 989793. doi:10.3389/fphys.2022.989793
Calabrese, E. J., and Kozumbo, W. J. (2021). The hormetic dose-response mechanism: Nrf2 activation. Pharmacol. Res. 167, 105526. doi:10.1016/j.phrs.2021.105526
Calvo-Rodriguez, M., Hou, S. S., Snyder, A. C., Kharitonova, E. K., Russ, A. N., Das, S., et al. (2020). Increased mitochondrial calcium levels associated with neuronal death in a mouse model of Alzheimer’s disease. Nat. Commun. 11 (1), 2146. doi:10.1038/s41467-020-16074-2
Cantadori, E., Brugnoli, M., Centola, M., Uffredi, E., Colonello, A., and Gullo, M. (2022). Date fruits as raw material for vinegar and non-alcoholic fermented beverages. Foods 11, 1972. doi:10.3390/foods11131972(13)
Cardozo, L. F. M. F., Alvarenga, L. A., Ribeiro, M., Dai, L., Shiels, P. G., Stenvinkel, P., et al. (2021). Cruciferous vegetables: Rationale for exploring potential salutary effects of sulforaphane-rich foods in patients with chronic kidney disease. Nutr. Rev. 79 (11), 1204–1224. doi:10.1093/nutrit/nuaa129
Carmona, P., Mendez, N., Ili, C. G., and Brebi, P. (2020). The role of clock genes in fibrinolysis regulation: Circadian disturbance and its effect on fibrinolytic activity. Front. Physiol. 11, 129. doi:10.3389/fphys.2020.00129
Caron, J. P., Kreher, M. A., Mickle, A. M., Wu, S., Przkora, R., Estores, I. M., et al. (2022). Intermittent fasting: Potential utility in the treatment of chronic pain across the clinical spectrum. Nutrients 14 (12), 2536. doi:10.3390/nu14122536
Carrillo-vico, A., Lardone, P. J., Melatonin, Á. N., Rodríguez-Rodríguez, A., and Guerrero, J. M. (2013). Melatonin: Buffering the immune system. Int. J. Mol. Sci. 14, 8638–8683. doi:10.3390/ijms14048638
Carter, B., Justin, H. S., Gulick, D., and Gamsby, J. J. (2021). The molecular clock and neurodegenerative disease: A stressful time. Front. Mol. Biosci. 8, 644747. doi:10.3389/fmolb.2021.644747
Carucci, L., Coppola, S., Luzzetti, A., Giglio, V., Vanderhoof, J., and Berni Canani, R. (2021). The role of probiotics and postbiotics in modulating the gut microbiome-immune system axis in the pediatric age. Minerva Pediatr. 73 (2), 115–127. doi:10.23736/S2724-5276.21.06188-0
Cassim, S., Vučetić, M., Ždralević, M., and Pouyssegur, J. (2020). Warburg and beyond: The power of mitochondrial metabolism to collaborate or replace fermentative glycolysis in cancer. Cancers (Basel) 12 (5), 1119–1125. doi:10.3390/cancers12051119
Castora, F. J., Kerns, K. A., Pflanzer, H. K., Hitefield, N. L., Gershon, B., Shugoll, J., et al. (2022). Expression changes in mitochondrial genes affecting mitochondrial morphology, transmembrane potential, fragmentation, amyloidosis, and neuronal cell death found in brains of alzheimer’s disease patients. J. Alzheimers Dis. 90, 119–137. doi:10.3233/JAD-220161
Cenit, M. C., Olivares, M., Codoner-Franch, P., and Sanz, Y. (2015). Intestinal microbiota and celiac disease: Cause, consequence or Co-evolution? Nutrients 7 (8), 6900–6923. doi:10.3390/nu7085314
Chávez-Castillo, M., Ortega, Á., Cudris-Torres, L., Duran, P., Rojas, M., Manzano, A., et al. (2021). Specialized pro-resolving lipid mediators: The future of chronic pain therapy? Int. J. Mol. Sci. 22, 10370. doi:10.3390/ijms221910370
Chen, C. H., Lin, C. L., and Kao, C. H. (2016). Irritable bowel syndrome is associated with an increased risk of dementia: A nationwide population-based study. PLoS One 11 (1), e0144589. doi:10.1371/journal.pone.0144589
Chen, D., Zhang, T., and Lee, T. H. (2020). Cellular mechanisms of melatonin: Insight from neurodegenerative diseases. Biomolecules 10 (8), 1158. doi:10.3390/biom10081158
Chen, G., Kroemer, G., and Kepp, O. (2020). Mitophagy: An emerging role in aging and age-associated diseases. Front. Cell Dev. Biol. 8, 200. doi:10.3389/fcell.2020.00200
Chen, G., Zhang, Y. Q., Qadri, Y. J., Serhan, C. N., and Ji, R. R. (2018). Microglia in pain: Detrimental and protective roles in pathogenesis and resolution of pain. Neuron 100 (6), 1292–1311. doi:10.1016/j.neuron.2018.11.009
Chen, J., He, H. J., Ye, Q., Feng, F., Wang, W. W., Gu, Y., et al. (2021). Defective autophagy and mitophagy in alzheimer’s disease: Mechanisms and translational implications. Mol. Neurobiol. 58 (10), 5289–5302. doi:10.1007/s12035-021-02487-7
Chen, Q. M. (2021). Nrf2 for protection against oxidant generation and mitochondrial damage in cardiac injury. Free Radic. Biol. Med. 179, 133–143. doi:10.1016/j.freeradbiomed.2021.12.001
Chen, Y., Xu, J., and Chen, Y. (2021). Regulation of neurotransmitters by the gut microbiota and effects on cognition in neurological disorders. Nutr. MDPI 13, 2099. doi:10.3390/nu13062099
Cheng, R., Dhorajia, V. V., Kim, J., and Kim, Y. (2022). Mitochondrial iron metabolism and neurodegenerative diseases. Neurotoxicology 88, 88–101. doi:10.1016/j.neuro.2021.11.003
Chia, S. J., Tan, E. K., and Chao, Y. X. (2020). Historical perspective: Models of Parkinson’s disease. Int. J. Mol. Sci. 21, 2464. doi:10.3390/ijms21072464
Chian, S., Zhao, Y., Xu, M., Yu, X., Ke, X., Gao, R., et al. (2019). Ginsenoside Rd reverses cisplatin resistance in non-small-cell lung cancer A549 cells by downregulating the nuclear factor erythroid 2-related factor 2 pathway. Anticancer. Drugs 30, 838–845. doi:10.1097/CAD.0000000000000781
Choe, S. S., Huh, J. Y., Hwang, I. J., Kim, J. I., and Kim, J. B. (2016). Adipose tissue remodeling: Its role in energy metabolism and metabolic disorders. Front. Endocrinol. 7, 30. doi:10.3389/fendo.2016.00030
Choi, I. Y., Piccio, L., Childress, P., Bollman, B., Ghosh, A., Brandhorst, S., et al. (2016). A diet mimicking fasting promotes regeneration and reduces autoimmunity and multiple sclerosis symptoms. Cell Rep. 15 (10), 2136–2146. doi:10.1016/j.celrep.2016.05.009
Choi, S., Kim, K., Cha, M., Kim, M., and Lee, B. H. (2020). mTOR signaling intervention by Torin1 and XL388 in the insular cortex alleviates neuropathic pain. Neurosci. Lett. 718, 134742. doi:10.1016/j.neulet.2020.134742
Chopyk, D. M., and Grakoui, A. (2020). Contribution of the intestinal microbiome and gut barrier to hepatic disorders. Gastroenterology 159 (3), 849–863. doi:10.1053/j.gastro.2020.04.077
Choubey, V., Zeb, A., and Kaasik, A. (2021). Molecular mechanisms and regulation of mammalian mitophagy. Cells 11, 38. doi:10.3390/cells11010038
Choudhury, F. K., Lavender Hackman, G., Lodi, A., and Tiziani, S. (2020). Stable isotope tracing metabolomics to investigate the metabolic activity of bioactive compounds for cancer prevention and treatment. Cancers (Basel) 12 (8), 2147–2221. doi:10.3390/cancers12082147
Chun, K. S., Raut, P. K., Kim, D. H., and Surh, Y. J. (2021). Role of chemopreventive phytochemicals in NRF2-mediated redox homeostasis in humans. Free Radic. Biol. Med. 172, 699–715. doi:10.1016/j.freeradbiomed.2021.06.031
Chung, N., Park, J., and Lim, K. (2017). The effects of exercise and cold exposure on mitochondrial biogenesis in skeletal muscle and white adipose tissue. J. Exerc Nutr. Biochem. 21 (2), 39–47. doi:10.20463/jenb.2017.0020
Cianciulli, A., Calvello, R., Ruggiero, M., and Panaro, M. A. (2022). Inflammaging and brain: Curcumin and its beneficial potential as regulator of microglia activation. Molecules 27, 341. doi:10.3390/molecules27020341
Cicero, A. F. G., and Colletti, A. (2015). Nutraceuticals and blood pressure control: Results from clinical trials and meta-analyses. High. Blood Press Cardiovasc Prev. 22 (3), 203–213. doi:10.1007/s40292-015-0081-8
Clark, A., and Mach, N. (2017). The crosstalk between the gut microbiota and mitochondria during exercise. Front. Physiol. 8, 319. doi:10.3389/fphys.2017.00319
Clifford, T., Acton, J. P., Cocksedge, S. P., Davies, K. A. B., and Bailey, S. J. (2021). The effect of dietary phytochemicals on nuclear factor erythroid 2-related factor 2 (Nrf2) activation: A systematic review of human intervention trials. Mol. Biol. Rep. 48 (2), 1745–1761. doi:10.1007/s11033-020-06041-x
Coelho, M., Oliveira, T., and Fernandes, R. (2013). Biochemistry of adipose tissue: An endocrine organ. Archives Med. Sci. 9 (2), 191–200. doi:10.5114/aoms.2013.33181
Cogliati, S., Cabrera-Alarcón, J. L., and Enriquez, J. A. (2021). Regulation and functional role of the electron transport chain supercomplexes. Biochem. Soc. Trans. [Internet 49 (6), 2655–2668. doi:10.1042/BST20210460
Contreras, L., Drago, I., Zampese, E., and Pozzan, T. (2010). Mitochondria: The calcium connection. Biochimica Biophysica Acta (BBA) - Bioenergetics 1797 (6–7), 607–618. doi:10.1016/j.bbabio.2010.05.005
Coronati, M., Baratta, F., Pastori, D., Ferro, D., Angelico, F., and Del Ben, M. (2022). Added fructose in non-alcoholic fatty liver disease and in metabolic syndrome: A narrative review. Nutrients 14 (6), 1127. doi:10.3390/nu14061127
Corrêa, H., Magalhaes, K. G., and Magalhaes, (2019). The impact of the adipose organ plasticity on inflammation and cancer progression. Cells 8 (7), 662. doi:10.3390/cells8070662
Correnti, M., Binatti, E., Gammella, E., Invernizzi, P., and Recalcati, S. (2022). The emerging role of tumor microenvironmental stimuli in regulating metabolic rewiring of liver cancer stem cells. Cancers (Basel) 15 (1), 5. doi:10.3390/cancers15010005
Cox, L. M., and Weiner, H. L. (2018). Microbiota signaling pathways that influence neurologic disease. Neurotherapeutics 15 (1), 135–145. doi:10.1007/s13311-017-0598-8
Cristofori, F., Dargenio, V. N., Dargenio, C., Miniello, V. L., Barone, M., and Francavilla, R. (2021). Anti-inflammatory and immunomodulatory effects of probiotics in gut inflammation: A door to the body. Front. Immunol. 12, 578386. doi:10.3389/fimmu.2021.578386
Cuadrado, A., Rojo, A. I., Wells, G., Hayes, J. D., Cousin, S. P., Rumsey, W. L., et al. (2019). Therapeutic targeting of the NRF2 and KEAP1 partnership in chronic diseases. Nat. Rev. Drug Discov. 18 (4), 295–317. doi:10.1038/s41573-018-0008-x
Cuevas-Cervera, M., Perez-Montilla, J. J., Gonzalez-Muñoz, A., Garcia-Rios, M. C., and Navarro-Ledesma, S. (2022). The effectiveness of intermittent fasting, time restricted feeding, caloric restriction, a ketogenic diet and the mediterranean diet as part of the treatment plan to improve health and chronic musculoskeletal pain: A systematic review. Int. J. Environ. Res. Public Health 19 (11), 6698. doi:10.3390/ijerph19116698
Cuevas-Sierra, A., Ramos-Lopez, O., Riezu-Boj, J. I., Milagro, F. I., and Martinez, J. A. (2019). Diet, gut microbiota, and obesity: Links with host genetics and epigenetics and potential applications. Adv. Nutr. 10 (1), S17–S30. doi:10.1093/advances/nmy078
Cui, Z., Napolitano, G., de Araujo, M. E. G., Esposito, A., Monfregola, J., Huber, L. A., et al. (2023). Structure of the lysosomal mTORC1–TFEB–Rag–Ragulator megacomplex. Nature 614 (7948), 572–579. doi:10.1038/s41586-022-05652-7
Cunniff, B., McKenzie, A. J., Heintz, N. H., and Howe, A. K. (2016). AMPK activity regulates trafficking of mitochondria to the leading edge during cell migration and matrix invasion. Mol. Biol. Cell 27 (17), 2662–2674. doi:10.1091/mbc.E16-05-0286
Curi, R., Levada-Pires, A. C., Silva, E. B., Poma, S. D. O., Zambonatto, R. F., Domenech, P., et al. (2020). The critical role of cell metabolism for essential neutrophil functions. Cell Physiol. Biochem. 54 (4), 629–647. doi:10.33594/000000245
Cusso, L., Musteanu, M., Mulero, F., Barbacid, M., and Desco, M. (2019). Effects of a ketogenic diet on [(18)F]FDG-PET imaging in a mouse model of lung cancer. Mol. Imaging Biol. 21 (2), 279–285. doi:10.1007/s11307-018-1233-8
D'Souza, R. F., Woodhead, J. S. T., Hedges, C. P., Zeng, N., Wan, J., Kumagai, H., et al. (2020). Increased expression of the mitochondrial derived peptide, MOTS-c, in skeletal muscle of healthy aging men is associated with myofiber composition. Aging (Albany N. Y. 12, 5244–5258. doi:10.18632/aging.102944
D’Amelio, P., and Sassi, F. (2018). Gut microbiota, immune system, and bone. Calcif. Tissue Int. 102 (4), 415–425. doi:10.1007/s00223-017-0331-y
Danese, A., Leo, S., Rimessi, A., Wieckowski, M. R., Fiorica, F., Giorgi, C., et al. (2021). Cell death as a result of calcium signaling modulation: A cancer-centric prospective. Biochimica Biophysica Acta (BBA) - Mol. Cell Res. 1868 (8), 119061. doi:10.1016/j.bbamcr.2021.119061
Das, R. R., Sankar, J., and Kabra, S. K. (2022). Role of breathing exercises in asthma-yoga and pranayama. Indian J. Pediatr. 89 (2), 174–180. doi:10.1007/s12098-021-03998-w
Dasuni Wasana, P. W., Hasriadi, , Muangnoi, C., Vajragupta, O., Rojsitthisak, P., Rojsitthisak, P., et al. (2022). Curcumin and metformin synergistically modulate peripheral and central immune mechanisms of pain. Sci. Rep. 12 (1), 9713. doi:10.1038/s41598-022-13647-7
de Cabo, R., and Mattson, M. P. (2019). Effects of intermittent fasting on health, aging, and disease. N. Engl. J. Med. 381 (26), 2541–2551. doi:10.1056/NEJMra1905136
de la Cruz-López, K. G., Castro-Muñoz, L. J., Reyes-Hernández, D. O., García-Carrancá, A., and Manzo-Merino, J. (2019). Lactate in the regulation of tumor microenvironment and therapeutic approaches. Front. Oncol. 9, 1143. doi:10.3389/fonc.2019.01143
De Luca, F., and Shoenfeld, Y. (2019). The microbiome in autoimmune diseases. Clin. Exp. Immunol. 195 (1), 74–85. doi:10.1111/cei.13158
de Oliveira, G. L. V., Leite, A. Z., Higuchi, B. S., Gonzaga, M. I., and Mariano, V. S. (2017). Intestinal dysbiosis and probiotic applications in autoimmune diseases. Immunology 152 (1), 1–12. doi:10.1111/imm.12765
de Punder, K., and Pruimboom, L. (2015). Stress induces endotoxemia and low-grade inflammation by increasing barrier permeability. Front. Immunol. 6, 223. doi:10.3389/fimmu.2015.00223
de Punder, K., and Pruimboom, L. (2015). Stress induces endotoxemia and low-grade inflammation by increasing barrier permeability. Front. Immunol. 6, 223. doi:10.3389/fimmu.2015.00223
de Punder, K., and Pruimboom, L. (2013). The dietary intake of wheat and other cereal grains and their role in inflammation. Nutrients 5 (3), 771–787. doi:10.3390/nu5030771
de Vries, M. A., Klop, B., Eskes, S. A., van der Loos, T. L. J. M., Klessens-Godfroy, F. J. M., Wiebolt, J., et al. (2014). The postprandial situation as a pro-inflammatory condition. Clinica Investig. Arterioscler. 26 (4), 184–192. doi:10.1016/j.arteri.2014.02.007
Debattisti, V., Gerencser, A. A., Saotome, M., Das, S., and Hajnóczky, G. (2017). ROS control mitochondrial motility through p38 and the motor adaptor miro/trak. Cell Rep. 21 (6), 1667–1680. doi:10.1016/j.celrep.2017.10.060
Delaunay, S., Pascual, G., Feng, B., Klann, K., Behm, M., Hotz-Wagenblatt, A., et al. (2022). Mitochondrial RNA modifications shape metabolic plasticity in metastasis. Nature 607 (7919), 593–603. doi:10.1038/s41586-022-04898-5
Demeneix, B. A. (2019). Evidence for prenatal exposure to thyroid disruptors and adverse effects on brain development. Eur. Thyroid. J. 8 (6), 283–292. doi:10.1159/000504668
Deo, P., Chow, S. H., Han, M. L., Speir, M., Huang, C., Schittenhelm, R. B., et al. (2020). Mitochondrial dysfunction caused by outer membrane vesicles from Gram-negative bacteria activates intrinsic apoptosis and inflammation. Nat. Microbiol. 5 (11), 1418–1427. doi:10.1038/s41564-020-0773-2
Diaz-Vegas, A., Sanchez-Aguilera, P., Krycer, J. R., Morales, P. E., Monsalves-Alvarez, M., Cifuentes, M., et al. (2020). Is mitochondrial dysfunction a common root of noncommunicable chronic diseases? Endocr. Rev. 41 (3), bnaa005. doi:10.1210/endrev/bnaa005
Dietert, R. R. (2021). Microbiome first approaches to rescue public health and reduce human suffering. Biomedicines 9 (11), 1581. doi:10.3390/biomedicines9111581
Dietz, J. V., Fox, J. L., and Khalimonchuk, O. (2021). Down the iron path: Mitochondrial iron homeostasis and beyond. Cells 10 (9), 2198. doi:10.3390/cells10092198
Dimidi, E., Cox, S. R., Rossi, M., and Whelan, K. (2019). Fermented foods: Definitions and characteristics, impact on the gut microbiota and effects on gastrointestinal health and disease. Nutrients 11, 1806. doi:10.3390/nu11081806(8)
Dolgacheva, L. P., Berezhnov, A. v., Fedotova, E. I., Zinchenko, V. P., and Abramov, A. Y. (2019). Role of DJ-1 in the mechanism of pathogenesis of Parkinson’s disease. J. Bioenergetics Biomembr. 51, 175–188. doi:10.1007/s10863-019-09798-4
Dornas, W. C., de Lima, W. G., Pedrosa, M. L., and Silva, M. E. (2015). Health implications of high-fructose intake and current research. Adv. Nutr. 6 (6), 729–737. doi:10.3945/an.114.008144
Drozdz, D., Alvarez-Pitti, J., Wójcik, M., Borghi, C., Gabbianelli, R., Mazur, A., et al. (2021). Obesity and cardiometabolic risk factors: From childhood to adulthood. Nutrients 13 (11), 4176. doi:10.3390/nu13114176
Duraj, T., Carrión-Navarro, J., Seyfried, T. N., García-Romero, N., and Ayuso-Sacido, A. (2021). Metabolic therapy and bioenergetic analysis: The missing piece of the puzzle. Mol. Metab. 54, 101389. doi:10.1016/j.molmet.2021.101389
El-Hattab, A. W., and Scaglia, F. (2013). Mitochondrial DNA depletion syndromes: Review and updates of genetic basis, manifestations, and therapeutic options. Neurotherapeutics 10 (2), 186–198. doi:10.1007/s13311-013-0177-6
Enriquez, J. A., and Lenaz, G. (2014). Coenzyme Q and the respiratory chain: Coenzyme Q pool and mitochondrial supercomplexes. Mol. Syndromol. 5 (3–4), 119–140. doi:10.1159/000363364
Erdoğan Yüce, G., and Taşcı, S. (2020). Effect of pranayama breathing technique on asthma control, pulmonary function, and quality of life: A single-blind, randomized, controlled trial. Complement. Ther. Clin. Pract. 38, 101081. doi:10.1016/j.ctcp.2019.101081
Eysert, F., Kinoshita, P. F., Mary, A., Vaillant-Beuchot, L., Checler, F., and Chami, M. (2020). Molecular dysfunctions of mitochondria-associated membranes (MAMs) in alzheimer’s disease. Int. J. Mol. Sci. [Internet 21 (24), 9521. doi:10.3390/ijms21249521
Fahed, G., Aoun, L., Bou Zerdan, M., Allam, S., Bou Zerdan, M., Bouferraa, Y., et al. (2022). Metabolic syndrome: Updates on pathophysiology and management in 2021. Int. J. Mol. Sci. 23 (2), 786. doi:10.3390/ijms23020786
Fairbrother-Browne, A., Ali, A. T., Reynolds, R. H., Garcia-Ruiz, S., Zhang, D., Chen, Z., et al. (2021). Mitochondrial-nuclear cross-talk in the human brain is modulated by cell type and perturbed in neurodegenerative disease. Commun. Biol. 4 (1), 1262. doi:10.1038/s42003-021-02792-w
Fan, Y. J., Zhou, Y. X., Zhang, L. R., Lin, Q. F., Gao, P. Z., Cai, F., et al. (2018). C1206, a novel curcumin derivative, potently inhibits Hsp90 and human chronic myeloid leukemia cells in vitro. Acta Pharmacol. Sin. 39 (4), 649–658. doi:10.1038/aps.2017.160
Fasano, A. (2020). All disease begins in the (leaky) gut: Role of zonulin-mediated gut permeability in the pathogenesis of some chronic inflammatory diseases. F1000Res 9. doi:10.12688/f1000research.20510.1
Fassier, P., Srour, B., Raynard, B., Zelek, L., Cohen, P., Bachmann, P., et al. (2018). Fasting and weight-loss restrictive diet practices among 2,700 cancer survivors: Results from the NutriNet-santé cohort. Int. J. Cancer 143 (11), 2687–2697. doi:10.1002/ijc.31646
Favero, G., Bonomini, F., Franco, C., and Rezzani, R. (2019). Mitochondrial dysfunction in skeletal muscle of a fibromyalgia model: The potential benefits of melatonin. Int. J. Mol. Sci. 20, 765. doi:10.3390/ijms20030765(3)
Feng, J., Zhang, S., Li, W., Bai, T., Liu, Y., and Chang, X. (2022). Intermittent fasting to the eye: A new dimension involved in physiological and pathological changes. Front. Med. 9, 867624. doi:10.3389/fmed.2022.867624
Fitzgerald, K. C., Bhargava, P., Smith, M. D., Vizthum, D., Henry-Barron, B., Kornberg, M. D., et al. (2022). Intermittent calorie restriction alters T cell subsets and metabolic markers in people with multiple sclerosis. EBioMedicine 82, 104124. doi:10.1016/j.ebiom.2022.104124
Fontecha-Barriuso, M., Martin-Sanchez, D., Martinez-Moreno, J. M., Monsalve, M., Ramos, A. M., Sanchez-Niño, M. D., et al. (2020). The role of PGC-1α and mitochondrial biogenesis in kidney diseases. Biomolecules 10 (2), 347. doi:10.3390/biom10020347
Foster, C., Farland, C. V., Guidotti, F., Harbin, M., Roberts, B., Schuette, J., et al. (2015). The effects of high intensity interval training vs steady state training on aerobic and anaerobic capacity. J. Sports Sci. Med. 14 (4), 747–755.
Gabriel, B. M., Altıntaş, A., Smith, J. A. B., Sardon-Puig, L., Zhang, X., Basse, A. L., et al. (2021). Disrupted circadian oscillations in type 2 diabetes are linked to altered rhythmic mitochondrial metabolism in skeletal muscle. Sci. Adv. 7 (43), eabi9654. doi:10.1126/sciadv.abi9654
Galano, A., Tan, D. X., and Reiter, R. J. (2018). Melatonin: A versatile protector against oxidative DNA damage. Molecules 23 (3), 530. doi:10.3390/molecules23030530
Garbincius, J. F., and Elrod, J. W. (2022). Mitochondrial calcium exchange in physiology and disease. Physiol. Rev. 102 (2), 893–992. doi:10.1152/physrev.00041.2020
Gezer, C. (2018). “Stress response of dietary phytochemicals in a hormetic manner for health and longevity,” in Gene expression and regulation in mammalian cells - transcription toward the establishment of novel therapeutics (InTech).
Ghafouri-Fard, S., Shoorei, H., Khanbabapour Sasi, A., Taheri, M., and Ayatollahi, S. A. (2021). The impact of the phytotherapeutic agent quercetin on expression of genes and activity of signaling pathways. Biomed. Pharmacother. 141, 111847. doi:10.1016/j.biopha.2021.111847
Ghasemi, F., Bagheri, H., Barreto, G. E., Read, M. I., and Sahebkar, A. (2019). Effects of curcumin on microglial cells. Neurotox. Res. 36 (1), 12–26. doi:10.1007/s12640-019-00030-0
Gherardi, G., di Marco, G., Rizzuto, R., and Mammucari, C. (2019). Crosstalk between mitochondrial Ca 2+ uptake and autophagy in skeletal muscle. Oxid. Med. Cell Longev. 2019, 1845321–1845410. doi:10.1155/2019/1845321
Gilardini Montani, M. S., Tarquini, G., Santarelli, R., Gonnella, R., Romeo, M. A., Benedetti, R., et al. (2021). p62/SQSTM1 promotes mitophagy and activates the NRF2-mediated anti-oxidant and anti-inflammatory response restraining EBV-driven B lymphocyte proliferation. Carcinogenesis 43, 277–287. doi:10.1093/carcin/bgab116
Glancy, B., Kane, D. A., Kavazis, A. N., Goodwin, M. L., Willis, W. T., and Gladden, L. B. (2021). Mitochondrial lactate metabolism: History and implications for exercise and disease. J. Physiol. 599 (3), 863–888. doi:10.1113/JP278930
Glory, A., and Averill-Bates, D. A. (2016). The antioxidant transcription factor Nrf2 contributes to the protective effect of mild thermotolerance (40°C) against heat shock-induced apoptosis. Free Radic. Biol. Med. 99, 485–497. doi:10.1016/j.freeradbiomed.2016.08.032
Glory, A., Bettaieb, A., and Averill-Bates, D. A. (2014). Mild thermotolerance induced at 40 °C protects cells against hyperthermia-induced pro-apoptotic changes in Bcl-2 family proteins. Int. J. Hyperth. 30 (7), 502–512. doi:10.3109/02656736.2014.968641
Green, A., Hossain, T., and Eckmann, D. M. (2022). Mitochondrial dynamics involves molecular and mechanical events in motility, fusion and fission. Front. Cell Dev. Biol. 10, 1010232. doi:10.3389/fcell.2022.1010232
Grilc, N. K., Sova, M., and Kristl, J. (2021). Drug delivery strategies for curcumin and other natural Nrf2 modulators of oxidative stress-related diseases. Pharmaceutics 13, 2137. doi:10.3390/pharmaceutics13122137(12)
Grünewald, A., Kumar, K. R., and Sue, C. M. (2019). New insights into the complex role of mitochondria in Parkinson’s disease. Prog. Neurobiol. 177, 73–93. doi:10.1016/j.pneurobio.2018.09.003
Grytting, V. S., Chand, P., Låg, M., Øvrevik, J., and Refsnes, M. (2022). The pro-inflammatory effects of combined exposure to diesel exhaust particles and mineral particles in human bronchial epithelial cells. Part Fibre Toxicol. 19 (1), 14. doi:10.1186/s12989-022-00455-0
Guo, L., Tian, J., and Du, H. (2017). Mitochondrial dysfunction and synaptic transmission failure in alzheimer’s disease. J. Alzheimer’s Dis. 57 (4), 1071–1086. doi:10.3233/JAD-160702
Gupta, S., Swanson, A. A., Chen, Y. B., Lopez, T., Milosevic, D., Kipp, B. R., et al. (2019). Incidence of succinate dehydrogenase and fumarate hydratase–deficient renal cell carcinoma based on immunohistochemical screening with SDHA/SDHB and FH/2SC. Hum. Pathol. 91, 114–122. doi:10.1016/j.humpath.2019.07.004
Gusic, M., and Prokisch, H. (2020). ncRNAs: New players in mitochondrial health and disease? Front. Genet. 11, 95. doi:10.3389/fgene.2020.00095
Guzmán-Ruiz, R., Ortega, F., Rodríguez, A., Vázquez-Martínez, R., Díaz-Ruiz, A., Garcia-Navarro, S., et al. (2014). Alarmin high-mobility group B1 (HMGB1) is regulated in human adipocytes in insulin resistance and influences insulin secretion in β-cells. Int. J. Obes. 38 (12), 1545–1554. doi:10.1038/ijo.2014.36
Gyamfi, J., Kim, J., and Choi, J. (2022). Cancer as a metabolic disorder. Int. J. Mol. Sci. 23 (3), 1155. doi:10.3390/ijms23031155
Haas, N. B., and Nathanson, K. L. (2014). Hereditary kidney cancer syndromes. Adv. Chronic Kidney Dis. 21, 81–90. doi:10.1053/j.ackd.2013.10.001
Han, F., Wu, G., Zhang, S., Zhang, J., Zhao, Y., and Xu, J. (2021). The association of metabolic syndrome and its components with the incidence and survival of colorectal cancer: A systematic review and meta-analysis. Int. J. Biol. Sci. 17 (2), 487–497. doi:10.7150/ijbs.52452
Han, Y. M., Kang, A. E., Min Park, J., Young Oh, J., Yoon Lee, D., Hye Choi, S., et al. (2020). Dietary intake of fermented kimchi prevented colitis-associated cancer. J. Clin. Biochem. Nutr. 67 (3), 263–273. doi:10.3164/jcbn.20-77
Harapas, C. R., Idiiatullina, E., Al-Azab, M., Hrovat-Schaale, K., Reygaerts, T., Steiner, A., et al. (2022). Organellar homeostasis and innate immune sensing. Nat. Rev. Immunol. 22 (9), 535–549. doi:10.1038/s41577-022-00682-8
Hardeland, R., Cardinali, D. P., Brown, G. M., and Pandi-Perumal, S. R. (2015). Melatonin and brain inflammaging. Prog. Neurobiol. 127–128, 46–63. doi:10.1016/j.pneurobio.2015.02.001
Harvie, M. N., and Howell, T. (2016). Could intermittent energy restriction and intermittent fasting reduce rates of cancer in obese, overweight, and normal-weight subjects? A summary of evidence. Adv. Nutr. 7 (4), 690–705. doi:10.3945/an.115.011767
Hassan, A., Arnold, B. M., Caine, S., Toosi, B. M., Verge, V. M. K., and Muir, G. D. (2018). Acute intermittent hypoxia and rehabilitative training following cervical spinal injury alters neuronal hypoxia- and plasticity-associated protein expression. PLoS One 13 (5), e0197486. doi:10.1371/journal.pone.0197486
He, L., Wang, J., Yang, Y., Li, J., and Tu, H. (2022). Mitochondrial sirtuins in Parkinson’s disease. Neurochem. Res. 47 (6), 1491–1502. doi:10.1007/s11064-022-03560-w
Heindel, J. J., and Blumberg, B. (2019). Environmental obesogens: Mechanisms and controversies. Annu. Rev. Pharmacol. Toxicol. 59, 89–106. doi:10.1146/annurev-pharmtox-010818-021304
Henriksen, R. E., Nilsen, R. M., and Strandberg, R. B. (2022). Loneliness increases the risk of type 2 diabetes: A 20 year follow-up – results from the HUNT study. Diabetologia 66, 82–92. doi:10.1007/s00125-022-05791-6
Herengt, A., Thyrsted, J., and Holm, C. K. (2021). NRF2 in viral infection. Antioxidants (Basel) 10, 1491. doi:10.3390/antiox10091491
Hernansanz-Agustín, P., and Enríquez, J. A. (2021). Generation of reactive oxygen species by mitochondria. Antioxidants 10 (3), 415. doi:10.3390/antiox10030415
Herzig, S., and Shaw, R. J. (2018). Ampk: Guardian of metabolism and mitochondrial homeostasis. Nat. Rev. Mol. Cell Biol. 19, 121–135. doi:10.1038/nrm.2017.95
Ho, K. V., Lei, Z., Sumner, L. W., Coggeshall, M. V., Hsieh, H. Y., Stewart, G. C., et al. (2018). Identifying antibacterial compounds in black walnuts (juglans nigra) using a metabolomics approach. Metabolites 8 (4), 58. doi:10.3390/metabo8040058
Horta-Baas, G., Romero-Figueroa, M. D. S., Montiel-Jarquin, A. J., Pizano-Zarate, M. L., Garcia-Mena, J., and Ramirez-Duran, N. (2017). Intestinal dysbiosis and rheumatoid arthritis: A link between gut microbiota and the pathogenesis of rheumatoid arthritis. J. Immunol. Res. 2017, 4835189. doi:10.1155/2017/4835189
Hu, C., Zhao, L., Zhang, F., and Li, L. (2021). Regulation of autophagy protects against liver injury in liver surgery-induced ischaemia/reperfusion. J. Cell Mol. Med. 25 (21), 9905–9917. doi:10.1111/jcmm.16943
Huertas, J. R., Casuso, R. A., Agustín, P. H., and Cogliati, S. (2021). Corrigendum to "stay fit, stay young: Mitochondria in movement: The role of exercise in the new mitochondrial paradigm". Oxid. Med. Cell Longev. 2021, 9274841. doi:10.1155/2021/9274841
Ihsan, M., Abbiss, C. R., and Allan, R. (2021). Adaptations to post-exercise cold water immersion: Friend, foe, or futile? Front. Sports Act. Living 3, 714148. doi:10.3389/fspor.2021.714148
Inigo, J. R., and Chandra, D. (2022). The mitochondrial unfolded protein response (UPRmt): Shielding against toxicity to mitochondria in cancer. J. Hematol. Oncol. 15, 98. doi:10.1186/s13045-022-01317-0
Ippolito, L., Comito, G., Parri, M., Iozzo, M., Duatti, A., Virgilio, F., et al. (2022). Lactate rewires lipid metabolism and sustains a metabolic-epigenetic Axis in prostate cancer. Cancer Res. 82 (7), 1267–1282. doi:10.1158/0008-5472.CAN-21-0914
Jain, I. H., Zazzeron, L., Goli, R., Alexa, K., Schatzman-Bone, S., Dhillon, H., et al. (2016). Hypoxia as a therapy for mitochondrial disease. Science 352, 54–61. doi:10.1126/science.aad9642
Jayasuriya, R., Dhamodharan, U., Ali, D., Ganesan, K., Xu, B., and Ramkumar, K. M. (2021). Targeting Nrf2/Keap1 signaling pathway by bioactive natural agents: Possible therapeutic strategy to combat liver disease. Phytomedicine 92, 153755. doi:10.1016/j.phymed.2021.153755
Jayawardena, R., Ranasinghe, P., Ranawaka, H., Gamage, N., Dissanayake, D., and Misra, A. (2020). Exploring the therapeutic benefits of pranayama (yogic breathing): A systematic review. Int. J. Yoga 13 (2), 99–110. doi:10.4103/ijoy.IJOY_37_19
Jazvinšćak Jembrek, M., Oršolić, N., Mandić, L., Sadžak, A., and Šegota, S. (2021). Anti-oxidative, anti-inflammatory and anti-apoptotic effects of flavonols: Targeting Nrf2, NF-κB and p53 pathways in neurodegeneration. Antioxidants (Basel). 10 (10), 1628. doi:10.3390/antiox10101628
Jethwa, H., and Abraham, S. (2017). The evidence for microbiome manipulation in inflammatory arthritis. Rheumatol. Oxf. 56 (9), 1452–1460. doi:10.1093/rheumatology/kew374
Jung, M. E., and Mallet, R. T. (2018). Intermittent hypoxia training: Powerful, non-invasive cerebroprotection against ethanol withdrawal excitotoxicity. Respir. Physiol. Neurobiol. 256, 67–78. doi:10.1016/j.resp.2017.08.007
Kaminsky, D. A., Guntupalli, K. K., Lippmann, J., Burns, S. M., Brock, M. A., Skelly, J., et al. (2017). Effect of yoga breathing (pranayama) on exercise tolerance in patients with chronic obstructive pulmonary disease: A randomized, controlled trial. J. Altern. Complement. Med. 23 (9), 696–704. doi:10.1089/acm.2017.0102
Katsiki, N., Stoian, A. P., Steiropoulos, P., Papanas, N., Suceveanu, A. I., and Mikhailidis, D. P. (2020). Metabolic syndrome and abnormal peri-organ or intra-organ fat (APIFat) deposition in chronic obstructive pulmonary disease: An overview. Metabolites 10 (11), 465. doi:10.3390/metabo10110465
Kaur, B., Sohrabi, Y., Emilio Martinez-Outschoorn, U., and Lisanti, M. P. (2022). Editorial: Hallmark of cancer: Reprogramming of cellular metabolism. Front. Oncol. Editor. 12, 1126913. doi:10.3389/fonc.2022.1126913
Kaur, B., Sohrabi, Y., and Martinez-Outschoorn, E. (2020). Hallmark of cancer: Reprogramming of cellular metabolism. Front. Oncol. 12. doi:10.3389/fonc.2022.1126913
Keipert, S., and Ost, M. (2021). Stress-induced FGF21 and GDF15 in obesity and obesity resistance. Trends Endocrinol. Metab. 32 (11), 904–915. doi:10.1016/j.tem.2021.08.008
Kelley, N., Jeltema, D., Duan, Y., and He, Y. (2019). The NLRP3 inflammasome: An overview of mechanisms of activation and regulation. Int. J. Mol. Sci. 20 (13), 3328. doi:10.3390/ijms20133328
Kes, M. M. G., van den Bossche, J., Griffioen, A. W., and Huijbers, E. J. M. (2020). Oncometabolites lactate and succinate drive pro-angiogenic macrophage response in tumors. Biochimica Biophysica Acta (BBA) - Rev. Cancer 1874 (2), 188427. doi:10.1016/j.bbcan.2020.188427
Khan, H., Patel, S., and Majumdar, A. (2021). Role of NRF2 and sirtuin activators in COVID-19. Clin. Immunol. 233, 108879. doi:10.1016/j.clim.2021.108879
Khan, N. A., Nikkanen, J., Yatsuga, S., Jackson, C., Wang, L., Pradhan, S., et al. (2017). mTORC1 regulates mitochondrial integrated stress response and mitochondrial myopathy progression. Cell Metab. 26 (2), 419–428. doi:10.1016/j.cmet.2017.07.007
Khan, S., Luck, H., Winer, S., and Winer, D. A. (2021). Emerging concepts in intestinal immune control of obesity-related metabolic disease. Nat. Commun. 12 (1), 2598. doi:10.1038/s41467-021-22727-7
Khan, S. U., Pathania, A. S., Wani, A., Fatima, K., Mintoo, M. J., Hamza, B., et al. (2022). Activation of lysosomal mediated cell death in the course of autophagy by mTORC1 inhibitor. Sci. Rep. 12 (1), 5052. doi:10.1038/s41598-022-07955-1
Khorshidian, N., Shadnoush, M., Zabihzadeh Khajavi, M., Sohrabvandi, S., Yousefi, M., and Mortazavian, A. M. (2021). Fructose and high fructose corn syrup: Are they a two-edged sword? Int. J. Food Sci. Nutr. 72 (5), 592–614. doi:10.1080/09637486.2020.1862068
Kim, M. S., Yang, H. J., Kim, S. H., Lee, H. W., and Lee, M. S. (2018). Effects of kimchi on human health: A protocol of systematic review of controlled clinical trials. Medicine 97 (13), e0163. doi:10.1097/MD.0000000000010163
Kinashi, Y., and Hase, K. (2021). Partners in leaky gut syndrome: Intestinal dysbiosis and autoimmunity. Front. Immunol. 12, 673708. doi:10.3389/fimmu.2021.673708
Kiser, C., Gonul, C. P., Olcum, M., and Genc, S. (2021). Inhibitory effects of sulforaphane on NLRP3 inflammasome activation. Mol. Immunol. 140, 175–185. doi:10.1016/j.molimm.2021.10.014
Knezevic, J., Starchl, C., Tmava Berisha, A., and Amrein, K. (2022). Thyroid-Gut-Axis: How does the microbiota influence thyroid function? Nutrients 12, 1769. doi:10.3390/nu12061769
Kobayashi, J. (2019). D-amino acids and lactic acid bacteria. Microorganisms 7 (12), 690–714. doi:10.3390/microorganisms7120690
Kodavati, M., Wang, H., and Hegde, M. L. (2020). Altered mitochondrial dynamics in motor neuron disease: An emerging perspective. Cells 9, 1065. doi:10.3390/cells9041065
Kokura, S., Adachi, S., Manabe, E., Mizushima, K., Hattori, T., Okuda, T., et al. (2007). Whole body hyperthermia improves obesity-induced insulin resistance in diabetic mice. Int. J. Hyperth. 23, 259–265. doi:10.1080/02656730601176824
Konig, M. F. (2020). The microbiome in autoimmune rheumatic disease. Best. Pract. Res. Clin. Rheumatol. 34 (1), 101473. doi:10.1016/j.berh.2019.101473
Kornberg, M. D. (2020). The immunologic Warburg effect: Evidence and therapeutic opportunities in autoimmunity. Wiley Interdiscip. Rev. Syst. Biol. Med. 12, e1486. doi:10.1002/wsbm.1486
Kraft, L. M., and Lackner, L. L. (2018). Mitochondrial anchors: Positioning mitochondria and more. Biochem. Biophys. Res. Commun. 500 (1), 2–8. doi:10.1016/j.bbrc.2017.06.193
Kressler, J., Millard-Stafford, M., and Warren, G. L. (2011). Quercetin and endurance exercise capacity: A systematic review and meta-analysis. Med. Sci. Sports Exerc 43 (12), 2396–2404. doi:10.1249/MSS.0b013e31822495a7
Krishna, S., Arrojo e Drigo, R., Capitanio, J. S., Ramachandra, R., Ellisman, M., and Hetzer, M. W. (2020). Identification of long-lived proteins in the mitochondria reveals increased stability of the electron transport chain. Dev. Cell 56 (21), 2952–2965.e9. doi:10.1016/j.devcel.2021.10.008
Kumar, D., Basu, S., Parija, L., Rout, D., Manna, S., Dandapat, J., et al. (2016). Curcumin and Ellagic acid synergistically induce ROS generation, DNA damage, p53 accumulation and apoptosis in HeLa cervical carcinoma cells. Biomed. Pharmacother. 81, 31–37. doi:10.1016/j.biopha.2016.03.037
Kumar, V., Kundu, S., Singh, A., and Singh, S. (2022). Understanding the role of histone deacetylase and their inhibitors in neurodegenerative disorders: Current targets and future perspective. Curr. Neuropharmacol. 20 (1), 158–178. doi:10.2174/1570159X19666210609160017
Kurhaluk, N., Tkachenko, H., and Nosar, V. (2013). The effects of intermittent hypoxia training on mitochondrial oxygen consumption in rats exposed to skeletal unloading. Ann. Clin. Lab. Sci. 43 (1), 54–63.
Kwak, S. H., Cho, Y. M., Noh, G. M., and Om, A. S. (2014). Cancer preventive potential of kimchi lactic acid bacteria (weissella cibaria, lactobacillus plantarum). J. Cancer Prev. 19 (4), 253–258. doi:10.15430/JCP.2014.19.4.253
Lane, N., and Martin, W. F. (2015). Eukaryotes really are special, and mitochondria are why. Proc. Natl. Acad. Sci. 112 (35). doi:10.1073/pnas.1509237112
Laugerette, F., Vors, C., Géloën, A., Chauvin, M. A., Soulage, C., Lambert-Porcheron, S., et al. (2011). Emulsified lipids increase endotoxemia: Possible role in early postprandial low-grade inflammation. J. Nutr. Biochem. 22 (1), 53–59. doi:10.1016/j.jnutbio.2009.11.011
Lautrup, S., Sinclair, D. A., Mattson, M. P., and Fang, E. F. (2019). NAD+ in brain aging and neurodegenerative disorders. Cell Metab. 30, 630–655. doi:10.1016/j.cmet.2019.09.001
Lavallee, C. M., Bruno, A., Ma, C., and Raman, M. (2022). The role of intermittent fasting in the management of nonalcoholic fatty liver disease: A narrative review. Nutrients 14 (21), 4655. doi:10.3390/nu14214655
Le, B., Anh, P. T. N., and Yang, S. H. (2020). Prebiotic potential and anti-inflammatory activity of soluble polysaccharides obtained from soybean residue. Foods 9, 1808. doi:10.3390/foods9121808(2)
Lechado Terradas, A., Zittlau, K. I., Macek, B., Fraiberg, M., Elazar, Z., and Kahle, P. J. (2021). Regulation of mitochondrial cargo-selective autophagy by posttranslational modifications. J. Biol. Chem. 297 (5), 101339. doi:10.1016/j.jbc.2021.101339
Lee, B. J., Tseng, Y. F., Yen, C. H., and Lin, P. T. (2013). Effects of coenzyme Q10 supplementation (300 mg/day) on antioxidation and anti-inflammation in coronary artery disease patients during statins therapy: A randomized, placebo-controlled trial. Nutr. J. 12 (1), 142. doi:10.1186/1475-2891-12-142
Lee, C., Raffaghello, L., Brandhorst, S., Safdie, F. M., Bianchi, G., Martin-Montalvo, A., et al. (2012). Fasting cycles retard growth of tumors and sensitize a range of cancer cell types to chemotherapy. Sci. Transl. Med. 4 (124), 124ra27. doi:10.1126/scitranslmed.3003293
Lee, J., Jang, J., Park, S. M., and Yang, S. R. (2021). An update on the role of Nrf2 in respiratory disease: Molecular mechanisms and therapeutic approaches. Int. J. Mol. Sci. 22, 8406. doi:10.3390/ijms22168406(16)
Lee, K. W., Shim, J. M., Yao, Z., Kim, J. A., and Kim, J. H. (2018). Properties of kimchi fermented with GABA-producing lactic acid bacteria as a starter. J. Microbiol. Biotechnol. 28 (4), 534–541. doi:10.4014/jmb.1709.09011
Lee, Y. M., and Lee, D. H. (2019). Mitochondrial toxins and healthy lifestyle meet at the crossroad of hormesis. Diabetes Metab. J. 43 (5), 568–577. doi:10.4093/dmj.2019.0143
Lende, T. H., Austdal, M., Varhaugvik, A. E., Skaland, I., Gudlaugsson, E., Kvaløy, J. T., et al. (2019). Influence of pre-operative oral carbohydrate loading vs. standard fasting on tumor proliferation and clinical outcome in breast cancer patients ─ a randomized trial. BMC Cancer 19 (1), 1076. doi:10.1186/s12885-019-6275-z
Li, W., Lai, K., Chopra, N., Zheng, Z., Das, A., and Diwan, A. D. (2022). Gut-disc axis: A cause of intervertebral disc degeneration and low back pain? Eur. Spine J. 31 (4), 917–925. doi:10.1007/s00586-022-07152-8
Li, Y., Chen, X., Huang, H., Liao, L., Chong, H., Li, G., et al. (2022). A feedback loop between NONHSAT024276 and PTBP1 inhibits tumor progression and glycolysis in HCC by increasing the PKM1/PKM2 ratio. Cancer Sci. doi:10.1111/cas.15697
Li, Z., Zhang, Z., Ren, Y., Wang, Y., Fang, J., Yue, H., et al. (2021). Aging and age-related diseases: From mechanisms to therapeutic strategies. Biogerontology 22 (2), 165–187. doi:10.1007/s10522-021-09910-5
Liebman, S. E., and Le, T. H. (2021). Eat your broccoli: Oxidative stress, NRF2, and sulforaphane in chronic kidney disease. Nutrients 13, 266. doi:10.3390/nu13010266
Liesa, M., and Shirihai, O. S. (2013). Mitochondrial dynamics in the regulation of nutrient utilization and energy expenditure. Cell Metab. 17, 491–506. doi:10.1016/j.cmet.2013.03.002
Lim, R., Barker, G., Wall, C. A., and Lappas, M. (2013). Dietary phytophenols curcumin, naringenin and apigenin reduce infection-induced inflammatory and contractile pathways in human placenta, foetal membranes and myometrium. Mol. Hum. Reprod. 19 (7), 451–462. doi:10.1093/molehr/gat015
Lima, T., Li, T. Y., Mottis, A., and Auwerx, J. (2022). Pleiotropic effects of mitochondria in aging. Nat. Aging 2 (3), 199–213. doi:10.1038/s43587-022-00191-2
Lin, L., and Zhang, J. (2017). Role of intestinal microbiota and metabolites on gut homeostasis and human diseases. BMC Immunol. 18 (1), 2. doi:10.1186/s12865-016-0187-3
Lin, M. Y., Cheng, X. T., Tammineni, P., Xie, Y., Zhou, B., Cai, Q., et al. (2017). Releasing syntaphilin removes stressed mitochondria from axons independent of mitophagy under pathophysiological conditions. Neuron 94 (3), 595–610.e6. doi:10.1016/j.neuron.2017.04.004
Lin, Q. (2013). Submerged fermentation of Lactobacillus rhamnosus YS9 for γ-aminobutyric acid (GABA) production. Braz J. Microbiol. 187, 183–187. doi:10.1590/S1517-83822013000100028
Lindqvist, D., Wolkowitz, O. M., Picard, M., Ohlsson, L., Bersani, F. S., Fernström, J., et al. (2018). Circulating cell-free mitochondrial DNA, but not leukocyte mitochondrial DNA copy number, is elevated in major depressive disorder. Neuropsychopharmacology 43 (7), 1557–1564. doi:10.1038/s41386-017-0001-9
Liu, F., Gao, S., Yang, Y., Zhao, X., Fan, Y., Ma, W., et al. (2018). Antitumor activity of curcumin by modulation of apoptosis and autophagy in human lung cancer A549 cells through inhibiting PI3K/Akt/mTOR pathway. Oncol. Rep. 39 (3), 1523–1531. doi:10.3892/or.2018.6188
Liu, G. Y., and Sabatini, D. M. (2020). mTOR at the nexus of nutrition, growth, ageing and disease. Nat. Rev. Mol. Cell Biol. 21, 183–203. doi:10.1038/s41580-019-0199-y
Liu, G. Y., and Sabatini, D. M. (2020). mTOR at the nexus of nutrition, growth, ageing and disease. Nat. Rev. Mol. Cell Biol. 21 (4), 183–203. doi:10.1038/s41580-019-0199-y
Liu, M., Bai, X., Yu, S., Zhao, W., Qiao, J., Liu, Y., et al. (2019). Ginsenoside Re inhibits ROS/ASK-1 dependent mitochondrial apoptosis pathway and activation of Nrf2-antioxidant response in beta-amyloid-challenged SH-SY5Y cells. Molecules 24, 2687. doi:10.3390/molecules24152687(15)
Liu, X., and Shan, G. (2021). Mitochondria encoded non-coding RNAs in cell physiology. Front. Cell Dev. Biol. 9, 713729. doi:10.3389/fcell.2021.713729
Lizama, B. N., and Chu, C. T. (2021). Neuronal autophagy and mitophagy in Parkinson’s disease. Mol. Asp. Med. 82, 100972. doi:10.1016/j.mam.2021.100972
Lobo, F., Haase, J., and Brandhorst, S. (2022). The effects of dietary interventions on brain aging and neurological diseases. Nutrients 14 (23), 5086. doi:10.3390/nu14235086
Lobo-Jarne, T., and Ugalde, C. (2017). Respiratory chain supercomplexes: Structures, function and biogenesis. Semin. Cell Dev. Biol. 76, 179–190. doi:10.1016/j.semcdb.2017.07.021
Locati, M., Curtale, G., and Mantovani, A. (2020). Diversity, mechanisms, and significance of macrophage plasticity. Annu. Rev. Pathology Mech. Dis. 15, 123–147. doi:10.1146/annurev-pathmechdis-012418-012718
Logan, R. W., and McClung, C. A. (2019). Rhythms of life: Circadian disruption and brain disorders across the lifespan. Nat. Rev. Neurosci. 20 (1), 49–65. doi:10.1038/s41583-018-0088-y
López-Crisosto, C., Bravo-Sagua, R., Rodriguez-Peña, M., Mera, C., Castro, P. F., Quest, A. F. G., et al. (2015). ER-to-mitochondria miscommunication and metabolic diseases. Biochimica Biophysica Acta (BBA) - Mol. Basis Dis. 1852 (10), 2096–2105. doi:10.1016/j.bbadis.2015.07.011
Lv, M., Zhu, X., Wang, H., Wang, F., and Guan, W. (2014). Roles of caloric restriction, ketogenic diet and intermittent fasting during initiation, progression and metastasis of cancer in animal models: A systematic review and meta-analysis. PLoS One 9 (12), 1151477–e115217. doi:10.1371/journal.pone.0115147
Lv, Y., Cheng, L., and Peng, F. (2022). Compositions and functions of mitochondria-associated endoplasmic reticulum membranes and their contribution to cardioprotection by exercise preconditioning. Front. Physiol. 13, 910452. doi:10.3389/fphys.2022.910452
Lv, Y., Gong, L., Wang, Z., Han, F., Liu, H., Lu, X., et al. (2015). Curcumin inhibits human cytomegalovirus by downregulating heat shock protein 90. Mol. Med. Rep. 12 (3), 4789–4793. doi:10.3892/mmr.2015.3983
Ma, K., Chen, G., Li, W., Kepp, O., Zhu, Y., and Chen, Q. (2020). Mitophagy, mitochondrial homeostasis, and cell fate. Front. Cell Dev. Biol. 8, 467. doi:10.3389/fcell.2020.00467
Magalhães, J., Falcão-Pires, I., Gonçalves, I. O., Lumini-Oliveira, J., Marques-Aleixo, I., Dos Passos, E., et al. (2013). Synergistic impact of endurance training and intermittent hypobaric hypoxia on cardiac function and mitochondrial energetic and signaling. Int. J. Cardiol. 168 (6), 5363–5371. doi:10.1016/j.ijcard.2013.08.001
Magaña, J. C., Deus, C. M., Giné-Garriga, M., Montané, J., and Pereira, S. P. (2022). Exercise-boosted mitochondrial remodeling in Parkinson’s disease. Biomedicines 10 (12), 3228. doi:10.3390/biomedicines10123228
Maletic, V., and Raison, C. L. (2009). Neurobiology of depression, fibromyalgia and neuropathic pain. Front. Biosci. (Landmark Ed. 14, 5291–5338. doi:10.2741/3598
Manchester, L. C., Coto-Montes, A., Boga, J. A., Andersen, L. P. H., Zhou, Z., Galano, A., et al. (2015). Melatonin: An ancient molecule that makes oxygen metabolically tolerable. J. Pineal Res. 59 (4), 403–419. doi:10.1111/jpi.12267
Mani, S., Sevanan, M., Krishnamoorthy, A., and Sekar, S. (2021). A systematic review of molecular approaches that link mitochondrial dysfunction and neuroinflammation in Parkinson’s disease. Neurol. Sci. 42 (11), 4459–4469. doi:10.1007/s10072-021-05551-1
Manukhina, E. B., Downey, H. F., Shi, X., and Mallet, R. T. (2016). Intermittent hypoxia training protects cerebrovascular function in Alzheimer’s disease. Exp. Biol. Med. (Maywood). 241 (12), 1351–1363. doi:10.1177/1535370216649060
Manzi, L., Costantini, L., Molinari, R., and Merendino, N. (2015). Effect of dietary omega-3 polyunsaturated fatty acid DHA on glycolytic enzymes and warburg phenotypes in cancer. Biomed. Res. Int. 2015, 137097. doi:10.1155/2015/137097
Marchi, S., Guilbaud, E., Tait, S. W. G., Yamazaki, T., and Galluzzi, L. (2022). Mitochondrial control of inflammation. Nat. Rev. Immunol. 23, 159–173. doi:10.1038/s41577-022-00760-x
Marchi, S., Guilbaud, E., Tait, S. W. G., Yamazaki, T., and Galluzzi, L. (2023). Mitochondrial control of inflammation. Nat. Rev. Immunol. 23 (3), 159–173. doi:10.1038/s41577-022-00760-x
Mariani, M., Sassano, M., and Boccia, S. (2021). Metabolic syndrome and gastric cancer risk: A systematic review and meta-analysis. Eur. J. Cancer Prev. 30 (3), 239–250. doi:10.1097/CEJ.0000000000000618
Marinac, C. R., Nelson, S. H., Breen, C. I., Hartman, S. J., Natarajan, L., Pierce, J. P., et al. (2016). Prolonged nightly fasting and breast cancer prognosis. JAMA Oncol. 2 (8), 1049–1055. doi:10.1001/jamaoncol.2016.0164
Markus, R. P., Sousa, K. S., da Silveira Cruz-Machado, S., Fernandes, P. A., and Ferreira, Z. S. (2021). Possible role of pineal and extra-pineal melatonin in surveillance, immunity, and first-line defense. Int. J. Mol. Sci. 22 (22), 12143. doi:10.3390/ijms222212143
Martel, J., Chang, S. H., Ko, Y. F., Hwang, T. L., Young, J. D., and Ojcius, D. M. (2022). Gut barrier disruption and chronic disease. Trends Endocrinol. Metab. 33 (4), 247–265. doi:10.1016/j.tem.2022.01.002
Matsumaru, D., and Motohashi, H. (2021). The KEAP1-NRF2 system in healthy aging and longevity. Antioxidants (Basel) 10, 19294. doi:10.3390/antiox10121929
Mattson, M. P. (2008). Dietary factors, hormesis and health. Ageing Res Rev 7, 43. doi:10.1016/j.arr.2007.08.004
Mattson, M. P., Longo, V. D., and Harvie, M. (2017). Impact of intermittent fasting on health and disease processes. Ageing Res. Rev. 39, 46–58. doi:10.1016/j.arr.2016.10.005
Maude, H., Davidson, M., Charitakis, N., Diaz, L., Bowers, W. H. T., Gradovich, E., et al. (2019). NUMT confounding biases mitochondrial heteroplasmy calls in favor of the reference allele. Front. Cell Dev. Bio 1–7, 201. doi:10.3389/fcell.2019.00201
McCarty, M. F., Iloki Assanga, S. B., Lewis Luján, L., O’Keefe, J. H., and DiNicolantonio, J. J. (2020). Nutraceutical strategies for suppressing NLRP3 inflammasome activation: Pertinence to the management of COVID-19 and beyond. Nutrients 13, 47. doi:10.3390/nu13010047
McKenzie, C., Tan, J., Macia, L., and Mackay, C. R. (2017). The nutrition-gut microbiome-physiology axis and allergic diseases. Immunol. Rev. 278 (1), 277–295. doi:10.1111/imr.12556
Melini, F., Melini, V., Luziatelli, F., Ficca, A. G., and Ruzzi, M. (2019). Health-promoting components in fermented foods: An up-to-date systematic review. Nutrients 11, 1189. doi:10.3390/nu11051189
Meng, X., Li, Y., Li, S., Zhou, Y., Gan, R. Y., Xu, D. P., et al. (2017). Dietary sources and bioactivities of melatonin. Nutr. MDPI Ag. 9, 367. doi:10.3390/nu9040367
Meng, X., Li, Y., Li, S., Zhou, Y., Gan, R. Y., Xu, D. P., et al. (2017). Dietary sources and bioactivities of melatonin. Nutrients 9, 367. doi:10.3390/nu9040367(4)
Merry, T. L., Chan, A., Woodhead Jst, , Reynolds, J. C., Kumagai, H., Kim, S. J., et al. (2020). Mitochondrial-derived peptides in energy metabolism. Am. J. Physiology-Endocrinology Metabolism 319 (4), E659–E666. doi:10.1152/ajpendo.00249.2020
Merry, T. L., and Ristow, M. (2016). Mitohormesis in exercise training. Free Radic. Biol. Med. 98, 123–130. doi:10.1016/j.freeradbiomed.2015.11.032
Mezzelani, A., Landini, M., Facchiano, F., Raggi, M. E., Villa, L., Molteni, M., et al. (2015). Environment, dysbiosis, immunity and sex-specific susceptibility: A translational hypothesis for regressive autism pathogenesis. Nutr. Neurosci. 18 (4), 145–161. doi:10.1179/1476830513Y.0000000108
Michielan, A., and D’Incà, R. (2015). Intestinal permeability in inflammatory bowel disease: Pathogenesis, clinical evaluation, and therapy of leaky gut. Mediat. Inflamm. 2015, 628157. doi:10.1155/2015/628157
Milanova, I. v., Kalsbeek, M. J. T., Wang, X. L., Korpel, N. L., Stenvers, D. J., Wolff, S. E. C., et al. (2019). Diet-induced obesity disturbs microglial immunometabolism in a time-of-day manner. Front. Endocrinol. (Lausanne) 10, 424. doi:10.3389/fendo.2019.00424
Millichap, L. E., Damiani, E., Tiano, L., and Hargreaves, I. P. (2021). Targetable pathways for alleviating mitochondrial dysfunction in neurodegeneration of metabolic and non-metabolic diseases. Int. J. Mol. Sci. 22 (21), 11444. doi:10.3390/ijms222111444
Mima, A. (2022). Mitochondria-targeted drugs for diabetic kidney disease. Heliyon 8 (2), e08878. doi:10.1016/j.heliyon.2022.e08878
Missailidis, D., Annesley, S. J., Allan, C. Y., Sanislav, O., Lidbury, B. A., Lewis, D. P., et al. (2020). An isolated complex v inefficiency and dysregulated mitochondrial function in immortalized lymphocytes from ME/CFS patients. Int. J. Mol. Sci. 21, 1074. doi:10.3390/ijms21031074
Mohajer, N., Du, C. Y., Checkcinco, C., and Blumberg, B. (2021). Obesogens: How they are identified and molecular mechanisms underlying their action. Front. Endocrinol. (Lausanne) 12, 780888. doi:10.3389/fendo.2021.780888
Mohammad, S., and Thiemermann, C. (2021). Role of metabolic endotoxemia in systemic inflammation and potential interventions. Front. Immunol. 11, 594150. doi:10.3389/fimmu.2020.594150
Mohammadi-Bardbori, A., Najibi, A., Amirzadegan, N., Gharibi, R., Dashti, A., Omidi, M., et al. (2015). Coenzyme Q10 remarkably improves the bio-energetic function of rat liver mitochondria treated with statins. Eur. J. Pharmacol. 762, 270–274. doi:10.1016/j.ejphar.2015.05.041
Mora Barthelmess, R., Stijlemans, B., and Van Ginderachter, J. A. (2023). Hallmarks of cancer affected by the MIF cytokine family. Cancers (Basel) 15 (2), 395. doi:10.3390/cancers15020395
Moreira, O. C., Estébanez, B., Martínez-Florez, S., Paz, J. A., Cuevas, M. J., and González-Gallego, J. (2017). Mitochondrial function and mitophagy in the elderly: Effects of exercise. Oxid. Med. Cell Longev. 2017, 2012798–2012813. doi:10.1155/2017/2012798
Morris, G., Walker, A. J., Walder, K., Berk, M., Marx, W., Carvalho, A. F., et al. (2021). Increasing Nrf2 activity as a treatment approach in neuropsychiatry. Mol. Neurobiol. 58 (5), 2158–2182. doi:10.1007/s12035-020-02212-w
Mou, Y., Du, Y., Zhou, L., Yue, J., Hu, X., Liu, Y., et al. (2022). Gut microbiota interact with the brain through systemic chronic inflammation: Implications on neuroinflammation, neurodegeneration, and aging. Front. Immunol. 13, 796288. doi:10.3389/fimmu.2022.796288
Muri, J., and Kopf, M. (2020). Redox regulation of immunometabolism. Nat. Rev. Immunol. 21 (6), 363–381. doi:10.1038/s41577-020-00478-8
Muriel, P., López-Sánchez, P., and Ramos-Tovar, E. (2021). Fructose and the liver. Int. J. Mol. Sci. 22 (13), 6969. doi:10.3390/ijms22136969
Muskiet, F. A. J., Carrera-Bastos, P., Pruimboom, L., Lucia, A., and Furman, D. (2022). Obesity and leptin resistance in the regulation of the type I interferon early response and the increased risk for severe COVID-19. Nutrients 14, 1388. doi:10.3390/nu14071388
Navarro-Ledesma, S., Pruimboom, L., Lluch, E., Dueñas, L., Mena-Del Horno, S., and Gonzalez-Muñoz, A. (2022). The relationship between daily physical activity, psychological factors, and vegetative symptoms in women with fibromyalgia: A cross-sectional observational study. Int. J. Environ. Res. Public Health 19 (18), 11610. doi:10.3390/ijerph191811610
Nesci, S., Trombetti, F., Pagliarani, A., Ventrella, V., Algieri, C., Tioli, G., et al. . Molecular and supramolecular structure of the mitochondrial oxidative phosphorylation system: Implications for pathology. Life (Basel) 11, 242. doi:10.3390/life11030242
Ng, G. Y. Q., Sheng, D. P. L. K., Bae, H. G., Kang, S. W., Fann, D. Y. W., Park, J., et al. (2022). Integrative epigenomic and transcriptomic analyses reveal metabolic switching by intermittent fasting in brain. Geroscience 44 (4), 2171–2194. doi:10.1007/s11357-022-00537-z
Nicolaidis, S. (2019). Environment and obesity. Metabolism 100S, 153942. doi:10.1016/j.metabol.2019.07.006
Nikitakis, N. G., Papaioannou, W., Sakkas, L. I., and Kousvelari, E. (2017). The autoimmunity-oral microbiome connection. Oral Dis. 23 (7), 828–839. doi:10.1111/odi.12589
Nivethitha, L., Mooventhan, A., and Manjunath, N. K. (2016). Effects of various prāṇāyāma on cardiovascular and autonomic variables. Anc. Sci. Life 36 (2), 72–77. doi:10.4103/asl.ASL_178_16
Nogueira Silva Lima, M. T., Howsam, M., Anton, P. M., Delayre-Orthez, C., and Tessier, F. J. (2021). Effect of advanced glycation end-products and excessive calorie intake on diet-induced chronic low-grade inflammation biomarkers in murine models. Nutrients 13 (9), 3091. doi:10.3390/nu13093091
Novaes, M. M., Palhano-Fontes, F., Onias, H., Andrade, K. C., Lobão-Soares, B., Arruda-Sanchez, T., et al. (2020). Effects of yoga respiratory practice (bhastrika pranayama) on anxiety, affect, and brain functional connectivity and activity: A randomized controlled trial. Front. Psychiatry 11, 467. doi:10.3389/fpsyt.2020.00467
Nunn, A. V. W., Guy, G. W., Botchway, S. W., and Bell, J. D. (2020). From sunscreens to medicines: Can a dissipation hypothesis explain the beneficial aspects of many plant compounds? Phytotherapy Res. 34, 1868–1888. doi:10.1002/ptr.6654
Okamatsu-Ogura, Y., Kuroda, M., Tsutsumi, R., Tsubota, A., Saito, M., Kimura, K., et al. (2020). UCP1-dependent and UCP1-independent metabolic changes induced by acute cold exposure in Brown adipose tissue of mice. Metabolism 113, 154396. doi:10.1016/j.metabol.2020.154396
Olivas-Aguirre, M., Torres-López, L., Pottosin, I., and Dobrovinskaya, O. (2021). Overcoming glucocorticoid resistance in acute lymphoblastic leukemia: Repurposed drugs can improve the protocol. Front. Oncol. 11, 617937. doi:10.3389/fonc.2021.617937
Oliveira, L. da C., Morais, G. P., Ropelle, E. R., de Moura, L. P., Cintra, D. E., Pauli, J. R., et al. (2022). Using intermittent fasting as a non-pharmacological strategy to alleviate obesity-induced hypothalamic molecular pathway disruption. Front. Nutr. 30, 858320. doi:10.3389/fnut.2022.858320
Oliveira, S. R., Simao, A. N. C., Kallaur, A. P., de Almeida, E. R. D., Morimoto, H. K., Lopes, J., et al. (2014). Disability in patients with multiple sclerosis: Influence of insulin resistance, adiposity, and oxidative stress. Nutrition 30 (3), 268–273. doi:10.1016/j.nut.2013.08.001
Opazo, M. C., Ortega-Rocha, E. M., Coronado-Arrazola, I., Bonifaz, L. C., Boudin, H., Neunlist, M., et al. (2018). Intestinal microbiota influences non-intestinal related autoimmune diseases. Front. Microbiol. 9, 432. doi:10.3389/fmicb.2018.00432
Oppedisano, F., Maiuolo, J., Gliozzi, M., Musolino, V., Carresi, C., Nucera, S., et al. (2020). The potential for natural antioxidant supplementation in the early stages of neurodegenerative disorders. Int. J. Mol. Sci. 21, 2618. doi:10.3390/ijms21072618(7)
Osete, J. R., Akkouh, I. A., de Assis, D. R., Szabo, A., Frei, E., Hughes, T., et al. (2021). Lithium increases mitochondrial respiration in iPSC-derived neural precursor cells from lithium responders. Mol. Psychiatry 26 (11), 6789–6805. doi:10.1038/s41380-021-01164-4
Pak, M., Bozkurt, S., Pınarbaşı, A., Öz Arslan, D., and Aksungar, F. B. (2022). Effects of prolonged intermittent fasting model on energy metabolism and mitochondrial functions in neurons. Ann. Neurosci. 29 (1), 21–31. doi:10.1177/09727531211072303
Pak, V. v., Ezeriņa, D., Lyublinskaya, O. G., Pedre, B., Tyurin-Kuzmin, P. A., Mishina, N. M., et al. (2020). Ultrasensitive genetically encoded indicator for hydrogen peroxide identifies roles for the oxidant in cell migration and mitochondrial function. Cell Metab. 31 (3), 642–653.e6. doi:10.1016/j.cmet.2020.02.003
Pålsson-McDermott, E. M., and O’Neill, L. A. J. (2020). Targeting immunometabolism as an anti-inflammatory strategy. Cell Res. 30 (4), 300–314. doi:10.1038/s41422-020-0291-z
Papakonstantinou, E., Oikonomou, C., Nychas, G., and Dimitriadis, G. D. (2022). Effects of diet, lifestyle, chrononutrition and alternative dietary interventions on postprandial glycemia and insulin resistance. Nutrients 14, 823. . doi:10.3390/nu14040823
Park, B., Yang, J. S., Moon, E. W., Seo, H. Y., and Ha, J. H. (2019). Influence of capsaicinoids content on the microbial community during kimchi fermentation. J. Microbiol. Biotechnol. 29 (10), 1580–1590. doi:10.4014/jmb.1907.07023
Parsamanesh, N., Asghari, A., Sardari, S., Tasbandi, A., Jamialahmadi, T., Xu, S., et al. (2021). Resveratrol and endothelial function: A literature review. Pharmacol. Res. 170, 105725. doi:10.1016/j.phrs.2021.105725
Parveen, S., and Alhazmi, Y. A. (2022). Impact of intermittent fasting on metabolic syndrome and periodontal disease-A suggested preventive strategy to reduce the public health burden. Int. J. Environ. Res. Public Health 19 (21), 14536. doi:10.3390/ijerph192114536
Patergnani, S., Danese, A., Bouhamida, E., Aguiari, G., Previati, M., Pinton, P., et al. (2020). Various aspects of calcium signaling in the regulation of apoptosis, autophagy, cell proliferation, and cancer. Int. J. Mol. Sci. 21 (21), 8323. doi:10.3390/ijms21218323
Patra, J. K., Das, G., Paramithiotis, S., and Shin, H. S. (2016). Kimchi and other widely consumed traditional fermented foods of korea: A review. Front. Microbiol. 7, 1493. doi:10.3389/fmicb.2016.01493
Patrick, R. P., and Johnson, T. L. (2021). Sauna use as a lifestyle practice to extend healthspan. Exp. Gerontol. 154, 111509. doi:10.1016/j.exger.2021.111509
Payen, V. L., Mina, E., van Hée, V. F., Porporato, P. E., and Sonveaux, P. (2020). Monocarboxylate transporters in cancer. Mol. Metab. 33, 48–66. doi:10.1016/j.molmet.2019.07.006
Perez-Montilla, J. J., Cuevas-Cervera, M., Gonzalez-Muñoz, A., Garcia-Rios, M. C., and Navarro-Ledesma, S. (2022). Efficacy of nutritional strategies on the improvement of the performance and health of the athlete: A systematic review. Int. J. Environ. Res. Public Health 19 (7), 4240. doi:10.3390/ijerph19074240
Peruzzotti-Jametti, L., Willis, C. M., Hamel, R., Krzak, G., and Pluchino, S. (2021). Metabolic control of smoldering neuroinflammation. Front. Immunol. 12, 705920. doi:10.3389/fimmu.2021.705920
Petra, A. I., Panagiotidou, S., Hatziagelaki, E., Stewart, J. M., Conti, P., and Theoharides, T. C. (2015). Gut-microbiota-brain Axis and its effect on neuropsychiatric disorders with suspected immune dysregulation. Clin. Ther. 37 (5), 984–995. doi:10.1016/j.clinthera.2015.04.002
Petrozziello, T., Bordt, E. A., Mills, A. N., Kim, S. E., Sapp, E., Devlin, B. A., et al. (2022). Targeting tau mitigates mitochondrial fragmentation and oxidative stress in amyotrophic lateral sclerosis. Mol. Neurobiol. 59 (1), 683–702. doi:10.1007/s12035-021-02557-w
Phillips, C. M., Chen, L. W., Heude, B., Bernard, J. Y., Harvey, N. C., Duijts, L., et al. (2019). Dietary inflammatory index and non-communicable disease risk: A narrative review. Nutrients 11 (8), 1873. doi:10.3390/nu11081873
Phillips, M. C. L. (2019). Fasting as a therapy in neurological disease. Nutrients 11 (10), 2501. doi:10.3390/nu11102501
Picard, M., and Sandi, C. (2021). The social nature of mitochondria: Implications for human health. Neurosci. Biobehav. Rev. 120, 595–610. doi:10.1016/j.neubiorev.2020.04.017
Pimentel, M., and Lembo, A. (2020). Microbiome and its role in irritable bowel syndrome. Dig. Dis. Sci. 65 (3), 829–839. doi:10.1007/s10620-020-06109-5
Pinheiro, R. G. R., Pinheiro, M., and Neves, A. R. (2021). Nanotechnology innovations to enhance the therapeutic efficacy of quercetin. Nanomater. (Basel) 11, 2658. doi:10.3390/nano11102658(10)
Pinna, S., Kunz, C., Halpern, A., Harrison, S. A., Jordan, S. F., Ward, J., et al. (2022). A prebiotic basis for ATP as the universal energy currency. PLoS Biol. 20 (10), e3001437. doi:10.1371/journal.pbio.3001437
Piotrowicz, K., Gąsowski, J., Michel, J. P., and Veronese, N. (2021). Post-COVID-19 acute sarcopenia: Physiopathology and management. Aging Clin. Exp. Res. 33, 2887–2898. doi:10.1007/s40520-021-01942-8
Pluimakers, V. G., van Santen, S. S., Fiocco, M., Bakker, M. C. E., van der Lelij, A. J., van den Heuvel-Eibrink, M. M., et al. (2021). Can biomarkers be used to improve diagnosis and prediction of metabolic syndrome in childhood cancer survivors? A systematic review. Obes. Rev. 22 (11), e13312. doi:10.1111/obr.13312
Poole, J., and Ray, D. (2022). The role of circadian clock genes in critical illness: The potential role of translational clock gene therapies for targeting inflammation, mitochondrial function, and muscle mass in intensive care. J. Biol. Rhythms 37 (4), 385–402. doi:10.1177/07487304221092727
Pradhan, N., Singh, C., and Singh, A. (2021). Coenzyme Q10 a mitochondrial restorer for various brain disorders. Naunyn Schmiedeb. Arch. Pharmacol. 394 (11), 2197–2222. doi:10.1007/s00210-021-02161-8
Proshkina, E., Shaposhnikov, M., and Moskalev, A. (2020). Genome-protecting compounds as potential geroprotectors. Int. J. Mol. Sci. 21 (12), 4484–4491. doi:10.3390/ijms21124484
Pruimboom, L., and Muskiet, F. A. J. (2018). Intermittent living; the use of ancient challenges as a vaccine against the deleterious effects of modern life – a hypothesis. Med. Hypotheses 120 (5), 28–42. doi:10.1016/j.mehy.2018.08.002
Pruimboom, L., Raison, C. L., and Muskiet, F. A. J. (2015). Physical activity protects the human brain against metabolic stress induced by a postprandial and chronic inflammation. Behav. Neurol. 2015, 569869–569911. doi:10.1155/2015/569869
Pruimboom, L., Ruiz-Núñez, B., Raison, C. L., and Muskiet, F. A. J. (2016). Influence of a 10-day mimic of our ancient lifestyle on anthropometrics and parameters of metabolism and inflammation: The “study of origin”. Biomed. Res. Int. 2016, 6935123. doi:10.1155/2016/6935123
Quiles, J. M., and Gustafsson, Å. B. (2022). The role of mitochondrial fission in cardiovascular health and disease. Nat. Rev. Cardiol. 19, 723–736. doi:10.1038/s41569-022-00703-y
Radaic, A., and Kapila, Y. L. (2021). The oralome and its dysbiosis: New insights into oral microbiome-host interactions. Comput. Struct. Biotechnol. J. 19, 1335–1360. doi:10.1016/j.csbj.2021.02.010
Rai, P. K., Craven, L., Hoogewijs, K., Russell, O. M., and Lightowlers, R. N. (2018). Advances in methods for reducing mitochondrial DNA disease by replacing or manipulating the mitochondrial genome. Essays Biochem. 62 (3), 455–465. doi:10.1042/EBC20170113
Rajasekaran, S., Soundararajan, D. C. R., Tangavel, C., Muthurajan, R., Sri Vijay Anand, K. S., Matchado, M. S., et al. (2020). Human intervertebral discs harbour a unique microbiome and dysbiosis determines health and disease. Eur. Spine J. 29 (7), 1621–1640. doi:10.1007/s00586-020-06446-z
Rasa, S., Nora-Krukle, Z., Henning, N., Eliassen, E., Shikova, E., Harrer, T., et al. (2018). Chronic viral infections in myalgic encephalomyelitis/chronic fatigue syndrome (ME/CFS). J. Transl. Med. 16 (1), 268. doi:10.1186/s12967-018-1644-y
Rauchová, H. (2021). Coenzyme Q10 effects in neurological diseases. Physiol. Res. 70 (4), S683–S714. doi:10.33549/physiolres.934712
Rea, K., Dinan, T. G., and Cryan, J. F. (2016). n, 4, 23–33. doi:10.1016/j.ynstr.2016.03.001The microbiome: A key regulator of stress and neuroinflammatioNeurobiol. Stress
Reiter, R. J., Tan, D. X., Rosales-Corral, S., Galano, A., Jou, M. J., and Acuna-Castroviejo, D. (2018). Melatonin mitigates mitochondrial meltdown: Interactions with SIRT3. Int. J. Mol. Sci. 19 (8), 2439. doi:10.3390/ijms19082439
Rey, F., Ottolenghi, S., Zuccotti, G. V., Samaja, M., and Carelli, S. (2022). Mitochondrial dysfunctions in neurodegenerative diseases: Role in disease pathogenesis, strategies for analysis and therapeutic prospects. Neural Regen. Res. 17 (4), 754–758. doi:10.4103/1673-5374.322430
Rizzetto, L., Fava, F., Tuohy, K. M., and Selmi, C. (2018). Connecting the immune system, systemic chronic inflammation and the gut microbiome: The role of sex. J. Autoimmun. 92, 12–34. doi:10.1016/j.jaut.2018.05.008
Roberts, F. L., and Markby, G. R. (2021). New insights into molecular mechanisms mediating adaptation to exercise; A review focusing on mitochondrial biogenesis, mitochondrial function, mitophagy and autophagy. Cells 10 (10), 2639. doi:10.3390/cells10102639
Roca-Portoles, A., Rodriguez-Blanco, G., Sumpton, D., Cloix, C., Mullin, M., Mackay, G. M., et al. (2020). Venetoclax causes metabolic reprogramming independent of BCL-2 inhibition. Cell Death Dis. 11 (8), 616. doi:10.1038/s41419-020-02867-2
Rodríguez, L. R., Lapeña-Luzón, T., Benetó, N., Beltran-Beltran, V., Pallardó, F. V., Gonzalez-Cabo, P., et al. (2022). Therapeutic strategies targeting mitochondrial calcium signaling: A new hope for neurological diseases? Antioxidants (Basel) 11 (1), 165. doi:10.3390/antiox11010165
Rosenfeld, C. S. (2015). Microbiome disturbances and autism spectrum disorders. Drug Metab. Dispos. 43 (10), 1557–1571. doi:10.1124/dmd.115.063826
Rozova, K., and Mankovska, I. (2012). Wpływ interwałowego treningu hipoksycznego na płuca i serca zdrowych szczurów. Pneumonol. Alergol. Pol. 80 (4), 296–300. doi:10.5603/arm.27573
Ruiz-Núñez, B., Pruimboom, L., Dijck-Brouwer, D. A. J., and Muskiet, F. A. J. (2013). Lifestyle and nutritional imbalances associated with western diseases: Causes and consequences of chronic systemic low-grade inflammation in an evolutionary context. J. Nutr. Biochem. 24 (7), 1183–1201. doi:10.1016/j.jnutbio.2013.02.009
Sahebkar, A. (2017). Effects of quercetin supplementation on lipid profile: A systematic review and meta-analysis of randomized controlled trials. Crit. Rev. Food Sci. Nutr. 57 (4), 666–676. doi:10.1080/10408398.2014.948609
Sainero-Alcolado, L., Liaño-Pons, J., Ruiz-Pérez, M. V., and Arsenian-Henriksson, M. (2022). Targeting mitochondrial metabolism for precision medicine in cancer. Cell Death Differ. 29 (7), 1304–1317. doi:10.1038/s41418-022-01022-y
Saito, M., Okamatsu-Ogura, Y., Matsushita, M., Watanabe, K., Yoneshiro, T., Nio-Kobayashi, J., et al. (2009). High incidence of metabolically active Brown adipose tissue in healthy adult humans: Effects of cold exposure and adiposity. Diabetes 58 (7), 1526–1531. doi:10.2337/db09-0530
Saleh, J., Peyssonnaux, C., Singh, K. K., and Edeas, M. (2020). Mitochondria and microbiota dysfunction in COVID-19 pathogenesis. Mitochondrion 54, 1–7. doi:10.1016/j.mito.2020.06.008
Salehi, B., Sharopov, F., Fokou, P. V. T., Kobylinska, A., de Jonge, L., Tadio, K., et al. (2019). Melatonin in medicinal and food plants: Occurrence, bioavailability, and health potential for humans. MDPI 8, 681. doi:10.3390/cells8070681
Salehi, B., Sharopov, F., Fokou, P. V. T., Kobylinska, A., Jonge, L., Tadio, K., et al. (2019). Melatonin in medicinal and food plants: Occurrence, bioavailability, and health potential for humans. Cells 8 (7), 681. doi:10.3390/cells8070681
Salita, T., Rustam, Y. H., Mouradov, D., Sieber, O. M., and Reid, G. E. (2022). Reprogrammed lipid metabolism and the lipid-associated hallmarks of colorectal cancer. Cancers (Basel) 14 (15), 3714. doi:10.3390/cancers14153714
Saltykova, M. M. (2019). Cold adaptation as a means of increasing antioxidant protection. Neurosci. Behav. Physiol. 49 (3), 323–330. doi:10.1007/s11055-019-00735-x
Sampson, T. R., Debelius, J. W., Thron, T., Janssen, S., Shastri, G. G., Ilhan, Z. E., et al. (2016). Gut microbiota regulate motor deficits and neuroinflammation in a model of Parkinson’s disease. Cell 167 (6), 1469–1480. doi:10.1016/j.cell.2016.11.018
Sandhir, R., Halder, A., and Sunkaria, A. (2017). Mitochondria as a centrally positioned hub in the innate immune response. Biochim. Biophys. Acta Mol. Basis Dis. 1863 (5), 1090–1097. doi:10.1016/j.bbadis.2016.10.020
Saner, N. J., Lee, M. J. C., Kuang, J., Pitchford, N. W., Roach, G. D., Garnham, A., et al. (2021). Exercise mitigates sleep-loss-induced changes in glucose tolerance, mitochondrial function, sarcoplasmic protein synthesis, and diurnal rhythms. Mol. Metab. 43, 101110. doi:10.1016/j.molmet.2020.101110
Santín-Márquez, R., Alarcón-Aguilar, A., López-Diazguerrero, N. E., Chondrogianni, N., and Königsberg, M. (2019). Sulforaphane - role in aging and neurodegeneration. Geroscience 41 (5), 655–670. doi:10.1007/s11357-019-00061-7
Sardon Puig, L., Valera-Alberni, M., Cantó, C., and Pillon, N. J. (2018). Circadian rhythms and mitochondria: Connecting the dots. Front. Genet. 9, 452. doi:10.3389/fgene.2018.00452
Sato, T., Higuchi, Y., Shibagaki, Y., and Hattori, S. (2017). Phosphoproteomic analysis identifies signaling pathways regulated by curcumin in human colon cancer cells. Anticancer Res. 37 (9), 4789–4798. doi:10.21873/anticanres.11885
Savencu, C. E., Linţa, A., Farcaş, G., Bînă, A. M., Creţu, O. M., Maliţa, D. C., et al. (2021). Impact of dietary restriction regimens on mitochondria, heart, and endothelial function: A brief overview. Front. Media S.A. 12, 768383. doi:10.3389/fphys.2021.768383
Scalise, M., Console, L., Rovella, F., Galluccio, M., Pochini, L., and Indiveri, C. (2020). Membrane transporters for amino acids as players of cancer metabolic rewiring. Cells 9, 2028. doi:10.3390/cells9092028
Scalise, M., Pochini, L., Console, L., Losso, M. A., and Indiveri, C. (2018). The Human SLC1A5 (ASCT2) amino acid transporter: From function to structure and role in cell biology. Front. Cell Dev. Biol. 6, 96. doi:10.3389/fcell.2018.00096
Scalise, M., Pochini, L., Galluccio, M., Console, L., and Indiveri, C. (2017). Glutamine transport and mitochondrial metabolism in cancer cell growth. Front. Oncol. 7, 306. doi:10.3389/fonc.2017.00306
Scalise, M., Pochini, L., Galluccio, M., Console, L., and Indiveri, C. (2017). Glutamine transport and mitochondrial metabolism in cancer cell growth. Front. Oncol. 7, 306. doi:10.3389/fonc.2017.00306
Scalise, M., Pochini, L., Galluccio, M., Console, L., and Indiveri, C. (2017). Glutamine transport and mitochondrial metabolism in cancer cell growth. Front. Oncol. 7 (10), 306–309. doi:10.3389/fonc.2017.00306
Scheperjans, F. (2016). Gut microbiota, 1013 new pieces in the Parkinson’s disease puzzle. Curr. Opin. Neurol. 29 (6), 773–780. doi:10.1097/WCO.0000000000000389
Schmeisser, S., Schmeisser, K., Weimer, S., Groth, M., Priebe, S., Fazius, E., et al. (2013). Mitochondrial hormesis links low-dose arsenite exposure to lifespan extension. Aging Cell 12 (3), 508–517. doi:10.1111/acel.12076
Schmitt, L. O., and Gaspar, J. M. (2022). Obesity-induced brain neuroinflammatory and mitochondrial changes. Metabolites 13, 86. doi:10.3390/metabo13010086
Schwartz, L., Seyfried, T., Alfarouk, K. O., Da Veiga Moreira, J., and Fais, S. (2017). Out of Warburg effect: An effective cancer treatment targeting the tumor specific metabolism and dysregulated pH. Semin. Cancer Biol. 43, 134–138. doi:10.1016/j.semcancer.2017.01.005
Schwarz, T. L. (2013). Mitochondrial trafficking in neurons. Cold Spring Harb. Perspect. Biol. 5 (6), a011304. doi:10.1101/cshperspect.a011304
Scrima, R., Cela, O., Merla, G., Augello, B., Rubino, R., Quarato, G., et al. (2016). Clock-genes and mitochondrial respiratory activity: Evidence of a reciprocal interplay. Biochim. Biophys. Acta 1857 (8), 1344–1351. doi:10.1016/j.bbabio.2016.03.035
Segovia, S. A., Vickers, M. H., Gray, C., and Reynolds, C. M. (2014). Maternal obesity, inflammation, and developmental programming. Biomed. Res. Int. 2014, 418975. doi:10.1155/2014/418975
Serban, M. C., Sahebkar, A., Zanchetti, A., Mikhailidis, D. P., Howard, G., Antal, D., et al. (2016). Effects of quercetin on blood pressure: A systematic review and meta-analysis of randomized controlled trials. J. Am. Heart Assoc. 5, e002713. doi:10.1161/JAHA.115.002713(7)
Serebrovska, Z. O., Serebrovska, T. V., Kholin, V. A., Tumanovska, L. V., Shysh, A. M., Pashevin, D. A., et al. (2019). Intermittent hypoxia-hyperoxia training improves cognitive function and decreases circulating biomarkers of alzheimer’s disease in patients with mild cognitive impairment: A pilot study. Int. J. Mol. Sci. 20 (21), 5405. doi:10.3390/ijms20215405
Setayesh, T., Mišík, M., Langie, S. A. S., Godschalk, R., Waldherr, M., Bauer, T., et al. (2019). Impact of weight loss strategies on obesity-induced DNA damage. Mol. Nutr. Food Res. 63 (17), e1900045. doi:10.1002/mnfr.201900045
Seyfried, T. N., and Chinopoulos, C. (2021). Can the mitochondrial metabolic theory explain better the origin and management of cancer than can the somatic mutation theory? Metabolites 11 (9), 572. doi:10.3390/metabo11090572
Shanmuganathan, R., Tangavel, C., Sva, K. S., Muthurajan, R., Nayagam, S. M., Matchado, M. S., et al. (2022). Comparative metagenomic analysis of human intervertebral disc nucleus pulposus and cartilaginous end plates. Front. Cardiovasc Med. 9, 927652. doi:10.3389/fcvm.2022.927652
Sharma, N., Pasala, M. S., and Prakash, A. (2019). Mitochondrial DNA: Epigenetics and environment. Environ. Mol. Mutagen 60 (8), 668–682. doi:10.1002/em.22319
Shegay, P. v., Zabolotneva, A. A., Shatova, O. P., Shestopalov, A. v., and Kaprin, A. D. (2022). Evolutionary view on lactate-dependent mechanisms of maintaining cancer cell stemness and reprimitivization. Cancers (Basel) 14 (19), 4552. doi:10.3390/cancers14194552
Shen, C., Rolls, E., Cheng, W., Kang, J., Dong, G., Xie, C., et al. (2022). Associations of social isolation and loneliness with later dementia. Neurology 99, e164–e175. doi:10.1212/WNL.0000000000200583
Shen, K., Pender, C. L., Bar-Ziv, R., Zhang, H., Wickham, K., Willey, E., et al. (2022). Mitochondria as cellular and organismal signaling hubs. Annu. Rev. Cell Dev. Biol. 38 (1), 179–218. doi:10.1146/annurev-cellbio-120420-015303
Shen, Q., Wang, R., Liu, X., Song, P., Zheng, M., Ren, X., et al. (2022). HSF1 stimulates glutamine transport by super-enhancer-driven lncRNA LINC00857 in colorectal cancer. Cancers (Basel) 14 (16), 3855. doi:10.3390/cancers14163855
Shen, X., Wang, Y., Zhao, R., Wan, Q., Wu, Y., Zhao, L., et al. (2021). Metabolic syndrome and the risk of colorectal cancer: A systematic review and meta-analysis. Int. J. Colorectal Dis. 36 (10), 2215–2225. doi:10.1007/s00384-021-03974-y
Shetty, A. K., Kodali, M., Upadhya, R., and Madhu, L. N. (2018). Emerging anti-aging strategies - scientific basis and efficacy. Aging Dis. 9 (6), 1165–1184. doi:10.14336/AD.2018.1026
Shi, J., Ju, R., Gao, H., Huang, Y., Guo, L., and Zhang, D. (2022). Targeting glutamine utilization to block metabolic adaptation of tumor cells under the stress of carboxyamidotriazole-induced nutrients unavailability. Acta Pharm. Sin. B 12 (2), 759–773. doi:10.1016/j.apsb.2021.07.008
Shi, Y., and Williamson, G. (2016). Quercetin lowers plasma uric acid in pre-hyperuricaemic males: A randomised, double-blinded, placebo-controlled, cross-over trial. Br. J. Nutr. 115 (5), 800–806. doi:10.1017/S0007114515005310
Shu, S. A., Yuen, A. W. T., Woo, E., Chu, K. H., Kwan, H. S., Yang, G. X., et al. (2019). Microbiota and food allergy. Clin. Rev. Allergy Immunol. 57 (1), 83–97. doi:10.1007/s12016-018-8723-y
Silvestris, E., Lovero, D., and Palmirotta, R. (2019). Nutrition and female fertility: An interdependent correlation. Front. Endocrinol. (Lausanne) 10, 346. doi:10.3389/fendo.2019.00346(JUN)
Singh, M., Kapoor, A., and Bhatnagar, A. (2021). Physiological and pathological roles of aldose reductase. Metabolites 11, 655. doi:10.3390/metabo11100655
Skok, M. (2022). Mitochondrial nicotinic acetylcholine receptors: Mechanisms of functioning and biological significance. Int. J. Biochem. Cell Biol. 143, 106138. doi:10.1016/j.biocel.2021.106138
Smyl, C. (2016). Ketogenic diet and cancer-a perspective. Recent Results Cancer Res. 207, 233–240. doi:10.1007/978-3-319-42118-6_11
Soledad, R. B., Charles, S., and Samarjit, D. (2019). The secret messages between mitochondria and nucleus in muscle cell biology. Arch. Biochem. Biophys. 666, 52–62. doi:10.1016/j.abb.2019.03.019
Soofiyani, S. R., Hosseini, K., Forouhandeh, H., Ghasemnejad, T., Tarhriz, V., Asgharian, P., et al. (2021). Quercetin as a novel therapeutic approach for lymphoma. Oxid. Med. Cell Longev. 2021, 3157867. doi:10.1155/2021/3157867
Sorriento, D., di Vaia, E., and Iaccarino, G. (2021). Physical exercise: A novel tool to protect mitochondrial health. Front. Media S.A. 12, 660068. doi:10.3389/fphys.2021.660068
Spinelli, J. B., and Haigis, M. C. (2018). The multifaceted contributions of mitochondria to cellular metabolism. Nat. Cell Biol. 20, 745–754. doi:10.1038/s41556-018-0124-1
Stawski, R., Walczak, K., Kosielski, P., Meissner, P., Budlewski, T., Padula, G., et al. (2017). Repeated bouts of exhaustive exercise increase circulating cell free nuclear and mitochondrial DNA without development of tolerance in healthy men. PLoS One 12 (5), e0178216. doi:10.1371/journal.pone.0178216
Stefano, G. B., and Kream, R. M. (2022). Mitochondrial DNA heteroplasmy as an informational reservoir dynamically linked to metabolic and immunological processes associated with COVID-19 neurological disorders. Cell Mol. Neurobiol. 42 (1), 99–107. doi:10.1007/s10571-021-01117-z
Stewart, J. B., and Chinnery, P. F. (2015). The dynamics of mitochondrial DNA heteroplasmy: Implications for human health and disease. Nat. Rev. Genet. 16 (9), 530–542. doi:10.1038/nrg3966
Stiemsma, L. T., Nakamura, R. E., Nguyen, J. G., and Michels, K. B. (2020). Does consumption of fermented foods modify the human gut microbiota? J. Nutr. 150 (7), 1680–1692. doi:10.1093/jn/nxaa077
Stoker, M. L., Newport, E., Hulit, J. C., West, A. P., and Morten, K. J. (2019). Impact of pharmacological agents on mitochondrial function: A growing opportunity? Biochem. Soc. Trans. 47, 1757–1772. doi:10.1042/BST20190280
Sturm, G., Karan, K. R., Monzel, A. S., Santhanam, B., Taivassalo, T., Bris, C., et al. (2023). OxPhos defects cause hypermetabolism and reduce lifespan in cells and in patients with mitochondrial diseases. Commun. Biol. [Internet 6, 22. doi:10.1038/s42003-022-04303-x
Suliman, H. B., and Piantadosi, C. A. (2016). Mitochondrial quality control as a therapeutic target. Pharmacol. Rev. 68 (1), 20–48. doi:10.1124/pr.115.011502
Summer, R., Shaghaghi, H., Romero, F., Roque, W., Sales, D., Cuevas-Mora, K., et al. (2019). Activation of the mTORC1/PGC-1 axis promotes mitochondrial biogenesis and induces cellular senescence in the lung epithelium. Am. J. Physiol. Lung Cell Mol. Physiol. 316, L1049–L1060. doi:10.1152/ajplung.00244.2018
Sun, J., Zhang, J., Tian, J., Virzì, G. M., Digvijay, K., Cueto, L., et al. (2019). Mitochondria in sepsis-induced AKI. J. Am. Soc. Nephrol. 30 (7), 1151–1161. doi:10.1681/ASN.2018111126
Sunanda, T., Ray, B., Mahalakshmi, A. M., Bhat, A., Rashan, L., Rungratanawanich, W., et al. (2021). Mitochondria-endoplasmic reticulum crosstalk in Parkinson’s disease: The role of brain renin angiotensin system components. Biomolecules 11 (11), 1669. doi:10.3390/biom11111669
Sureshbabu, A., Patino, E., Ma, K. C., Laursen, K., Finkelsztein, E. J., Akchurin, O., et al. (2018). RIPK3 promotes sepsis-induced acute kidney injury via mitochondrial dysfunction. JCI Insight 3 (11), e98411. doi:10.1172/jci.insight.98411
Syed, A. M., Ram, C., Murty, U. S., and Sahu, B. D. (2021). A review on herbal Nrf2 activators with preclinical evidence in cardiovascular diseases. Phytother. Res. 35 (9), 5068–5102. doi:10.1002/ptr.7137
Symonds, M. E., Farhat, G., Aldiss, P., Pope, M., and Budge, H. (2019). Brown adipose tissue and glucose homeostasis–the link between climate change and the global rise in obesity and diabetes. Adipocyte 8 (1), 46–50. doi:10.1080/21623945.2018.1551689
Szewczyk-Golec, K., Wozniak, A., and Reiter, R. J. (2015). Inter-relationships of the chronobiotic, melatonin, with leptin and adiponectin: Implications for obesity. J. Pineal Res. 59 (3), 277–291. doi:10.1111/jpi.12257
Tadokoro, T., Ikeda, M., Ide, T., Deguchi, H., Ikeda, S., Okabe, K., et al. (2020). Mitochondria-dependent ferroptosis plays a pivotal role in doxorubicin cardiotoxicity. JCI Insight 5 (9). doi:10.1172/jci.insight.132747
Takahashi, S. (2021). Neuroprotective function of high glycolytic activity in astrocytes: Common roles in stroke and neurodegenerative diseases. Int. J. Mol. Sci. 22, 6568. doi:10.3390/ijms22126568
Takakura, W., and Pimentel, M. (2020). Small intestinal bacterial overgrowth and irritable bowel syndrome - an update. Front. Psychiatry 11, 664. doi:10.3389/fpsyt.2020.00664
Tamang, J. P., Shin, D., Jungjin, S., and Wan, C. S. (2016). Functional properties of microorganisms in fermented foods. Front. Microbiol. 7, 578–613. doi:10.3389/fmicb.2016.00578
Tan, D. X., Manchester, L. C., Esteban-Zubero, E., Zhou, Z., and Reiter, R. J. (2015). Melatonin as a potent and inducible endogenous antioxidant: Synthesis and metabolism. Molecules 20 (10), 18886–18906. doi:10.3390/molecules201018886
Tan, P., He, L., Cui, J., Qian, C., Cao, X., Lin, M., et al. (2017). Assembly of the WHIP-TRIM14-PPP6C mitochondrial complex promotes RIG-I-mediated antiviral signaling. Mol. Cell 68 (2), 293–307. doi:10.1016/j.molcel.2017.09.035
Tang, X., Wang, Z., Hu, S., and Zhou, B. (2022). Assessing drug-induced mitochondrial toxicity in cardiomyocytes: Implications for preclinical cardiac safety evaluation. Pharmaceutics 14, 1313. doi:10.3390/pharmaceutics14071313
Taniguchi, K., Sawamura, H., Ikeda, Y., Tsuji, A., Kitagishi, Y., and Matsuda, S. (2022). D-amino acids as a biomarker in schizophrenia. Diseases 10 (1), 9. doi:10.3390/diseases10010009
Taucher, E., Mykoliuk, I., Fediuk, M., and Smolle-Juettner, F. M. (2022). Autophagy, oxidative stress and cancer development. Cancers 14, 1637. doi:10.3390/cancers14071637
Teng, X., Cornaby, C., Li, W., and Morel, L. (2019). Metabolic regulation of pathogenic autoimmunity: Therapeutic targeting. Curr. Opin. Immunol. 61, 10–16. doi:10.1016/j.coi.2019.07.001
Terešak, P., Lapao, A., Subic, N., Boya, P., Elazar, Z., and Simonsen, A. (2022). Regulation of PRKN-independent mitophagy. Autophagy 18 (1), 24–39. doi:10.1080/15548627.2021.1888244
Teymouri, M., Pirro, M., Johnston, T. P., and Sahebkar, A. (2017). Curcumin as a multifaceted compound against human papilloma virus infection and cervical cancers: A review of chemistry, cellular, molecular, and preclinical features. Biofactors 43 (3), 331–346. doi:10.1002/biof.1344
Thakur, S., Storewala, P., Basak, U., Jalan, N., and Pethe, P. (2020). Clocking the circadian genes in human embryonic stem cells. Stem Cell Investig. 7, 9. doi:10.21037/sci-2020-014
Theunissen, F., West, P. K., Brennan, S., Petrović, B., Hooshmand, K., Akkari, P. A., et al. (2021). New perspectives on cytoskeletal dysregulation and mitochondrial mislocalization in amyotrophic lateral sclerosis. Transl. Neurodegener. 10 (1), 46. doi:10.1186/s40035-021-00272-z
Thiruvengadam, M., Venkidasamy, B., Subramanian, U., Samynathan, R., Ali Shariati, M., Rebezov, M., et al. (2021). Bioactive compounds in oxidative stress-mediated diseases: Targeting the NRF2/ARE signaling pathway and epigenetic regulation. Antioxidants (Basel). 10, 1859. doi:10.3390/antiox10121859
Tian, C., Liu, Y., Li, Z., Zhu, P., and Zhao, M. (2022). Mitochondria related cell death modalities and disease. Front. Cell Dev. Biol. 10, 832356. doi:10.3389/fcell.2022.832356
Tigchelaar, C., van Zuylen, M. L., Hulst, A. H., Preckel, B., van Beek, A. P., Kema, I. P., et al. (2022). Elevated cerebrospinal fluid glucose levels and diabetes mellitus are associated with activation of the neurotoxic polyol pathway. Diabetologia 65 (7), 1098–1107. doi:10.1007/s00125-022-05693-7
Tiwari, S., Sapkota, N., and Han, Z. (2022). Effect of fasting on cancer: A narrative review of scientific evidence. Cancer Sci. 113 (10), 3291–3302. doi:10.1111/cas.15492
Tower, J. (2015). Mitochondrial maintenance failure in aging and role of sexual dimorphism. Arch. Biochem. Biophys. 576, 17–31. doi:10.1016/j.abb.2014.10.008
Tran, D. H., and Wang, Z. V. (2019). Glucose metabolism in cardiac hypertrophy and heart failure. J. Am. Heart Assoc. 8, e012673. doi:10.1161/JAHA.119.012673
Tran, Q., Lee, H., Kim, C., Kong, G., Gong, N., Kwon, S. H., et al. (2020). Revisiting the warburg effect: Diet-based strategies for cancer prevention. Biomed. Res. Int. 2020, 8105735. doi:10.1155/2020/8105735
Traxler, L., Herdy, J. R., Stefanoni, D., Eichhorner, S., Pelucchi, S., Szücs, A., et al. (2022). Warburg-like metabolic transformation underlies neuronal degeneration in sporadic Alzheimer’s disease. Cell Metab. 34 (9), 1248–1263. doi:10.1016/j.cmet.2022.07.014
Trumpff, C., Marsland, A. L., Basualto-Alarcón, C., Martin, J. L., Carroll, J. E., Sturm, G., et al. (2019). Acute psychological stress increases serum circulating cell-free mitochondrial DNA. Psychoneuroendocrinology 106, 268–276. doi:10.1016/j.psyneuen.2019.03.026
Tsatsanis, C., Dermitzaki, E., Avgoustinaki, P., Malliaraki, N., Mytaras, V., and Margioris, A. N. (2015). The impact of adipose tissue-derived factors on the hypothalamic-pituitary-gonadal (HPG) axis. Horm. (Athens) 14 (4), 549–562. doi:10.14310/horm.2002.1649
Tsitkanou, S., della Gatta, P. A., and Russell, A. P. (2016). Skeletal muscle satellite cells, mitochondria, and MicroRNAs: Their involvement in the pathogenesis of ALS. Front. Physiol. 7, 403. doi:10.3389/fphys.2016.00403
Ulasov, A. V., Rosenkranz, A. A., Georgiev, G. P., and Sobolev, A. S. (2021). Nrf2/Keap1/ARE signaling: Towards specific regulation. Life Sci. 291, 120111. doi:10.1016/j.lfs.2021.120111
Uzunlulu, M., Telci Caklili, O., and Oguz, A. (2016). Association between metabolic syndrome and cancer. Ann. Nutr. Metab. 68 (3), 173–179. doi:10.1159/000443743
van Niekerk, G., and Engelbrecht, A. M. (2018). Role of PKM2 in directing the metabolic fate of glucose in cancer: A potential therapeutic target. Cell. Oncol. [Internet] 41 (4), 343–351. doi:10.1007/s13402-018-0383-7
Vandenbeek, R., Khan, N. P., and Estall, J. L. (2018). Linking metabolic disease with the PGC-1α Gly482Ser polymorphism. Endocrinology 159 (2), 853–865. doi:10.1210/en.2017-00872
Vashi, R., and Patel, B. M. (2021). NRF2 in cardiovascular diseases: A Ray of hope. J. Cardiovasc Transl. Res. 14 (3), 573–586. doi:10.1007/s12265-020-10083-8
Vasim, I., Majeed, C. N., and DeBoer, M. D. (2022). Intermittent fasting and metabolic health. Nutrients 14 (3), 631. doi:10.3390/nu14030631
Vaupel, P., and Multhoff, G. (2021). Revisiting the warburg effect: Historical dogma versus current understanding. J. Physiol. 599 (6), 1745–1757. doi:10.1113/JP278810
Vendramin, R., Marine, J. C., and Leucci, E. (2017). Non-coding RNAs: The dark side of nuclear-mitochondrial communication. EMBO J. 36 (9), 1123–1133. doi:10.15252/embj.201695546
Videhult, F. K., and West, C. E. (2016). Nutrition, gut microbiota and child health outcomes. Curr. Opin. Clin. Nutr. Metab. Care 19 (3), 208–213. doi:10.1097/MCO.0000000000000266
Virili, C., and Centanni, M. (2017). With a little help from my friends” - the role of microbiota in thyroid hormone metabolism and enterohepatic recycling. Mol. Cell Endocrinol. 458, 39–43. doi:10.1016/j.mce.2017.01.053
Vose, A. K., Welch, J. F., Nair, J., Dale, E. A., Fox, E. J., Muir, G. D., et al. (2022). Therapeutic acute intermittent hypoxia: A translational roadmap for spinal cord injury and neuromuscular disease. Exp. Neurol. 347, 113891. doi:10.1016/j.expneurol.2021.113891
Wacquier, B., Combettes, L., Van Nhieu, G. T., and Dupont, G. (2016). Interplay between intracellular Ca2+ oscillations and Ca2+-stimulated mitochondrial metabolism. Sci. Rep. 6 (1), 19316. doi:10.1038/srep19316
Waldron, M., Fowler, R., Heffernan, S., Tallent, J., Kilduff, L., and Jeffries, O. (2021). Effects of heat acclimation and acclimatisation on maximal aerobic capacity compared to exercise alone in both thermoneutral and hot environments: A meta-analysis and meta-regression. Sports Med. 51 (7), 1509–1525. doi:10.1007/s40279-021-01445-6
Walker, B. R., and Moraes, C. T. (2022). Nuclear-mitochondrial interactions. Biomolecules 12, 427. doi:10.3390/biom12030427
Wallace, D. C. (2005). A mitochondrial paradigm of metabolic and degenerative diseases, aging, and cancer: A dawn for evolutionary medicine. Annu. Rev. Genet. 39, 359–407. doi:10.1146/annurev.genet.39.110304.095751
Wallace, D. C. (2015). Mitochondrial DNA variation in human radiation and disease. Cell 163 (1), 33–38. doi:10.1016/j.cell.2015.08.067
Wang, H. J., Zakhari, S., and Jung, M. K. (2010). Alcohol, inflammation, and gut-liver-brain interactions in tissue damage and disease development. World J. Gastroenterol. 16 (11), 1304–1313. doi:10.3748/wjg.v16.i11.1304
Wang, H. X., and Wang, Y. P. (2016). Gut microbiota-brain Axis. Chin. Med. J. Engl. 129 (19), 2373–2380. doi:10.4103/0366-6999.190667
Wang, J., Xiao, M., Wang, J., Wang, S., Zhang, J., Guo, Y., et al. (2021). NRF2-Related epigenetic modifications in cardiac and vascular complications of diabetes mellitus. Front. Endocrinol. (Lausanne). 12, 598005. doi:10.3389/fendo.2021.598005
Wang, K., Zhang, C., Bao, J., Jia, X., Liang, Y., Wang, X., et al. (2016). Synergistic chemopreventive effects of curcumin and berberine on human breast cancer cells through induction of apoptosis and autophagic cell death. Sci. Rep. 6, 26064. doi:10.1038/srep26064
Wang, L., Yang, Z., He, X., Pu, S., Yang, C., Wu, Q., et al. (2022). Mitochondrial protein dysfunction in pathogenesis of neurological diseases. Front. Mol. Neurosci. 15, 974480. doi:10.3389/fnmol.2022.974480
Wang, N., Luo, Z., Jin, M., Sheng, W., Wang, H. T., Long, X., et al. (2019). Exploration of age-related mitochondrial dysfunction and the anti-aging effects of resveratrol in zebrafish retina. Aging 11 (10), 3117–3137. doi:10.18632/aging.101966
Wang, W., Pan, H., Ren, F., Chen, H., and Ren, P. (2022). Targeting ASCT2-mediated glutamine metabolism inhibits proliferation and promotes apoptosis of pancreatic cancer cells. Biosci. Rep. 42 (3). doi:10.1042/BSR20212171
Wang, Y., and Abolhassani, H. (2022). Updates of cancer hallmarks in patients with inborn errors of immunity. Curr. Opin. Allergy Clin. Immunol. 22 (6), 352–363. doi:10.1097/ACI.0000000000000863
Wang, Y., Zhang, X., Wen, Y., Li, S., Lu, X., Xu, R., et al. (2021). Endoplasmic reticulum-mitochondria contacts: A potential therapy target for cardiovascular remodeling-associated diseases. Front. Cell Dev. Biol. 9, 774989. doi:10.3389/fcell.2021.774989
Wang, Y. H., Gao, X., Tang, Y. R., Yu, Y., Sun, M. J., Chen, F. Q., et al. (2022). The role of NF-κB/NLRP3 inflammasome signaling pathway in attenuating pyroptosis by melatonin upon spinal nerve ligation models. Neurochem. Res. 47 (2), 335–346. doi:10.1007/s11064-021-03450-7
Ward, D. M., and Cloonan, S. M. (2019). Mitochondrial iron in human health and disease. Annu. Rev. Physiol. 81, 453–482. doi:10.1146/annurev-physiol-020518-114742
Welch, J. F., Sutor, T. W., Vose, A. K., Perim, R. R., Fox, E. J., and Mitchell, G. S. (2020). Synergy between acute intermittent hypoxia and task-specific training. Exerc Sport Sci. Rev. 48 (3), 125–132. doi:10.1249/JES.0000000000000222
Westermann, B. (2012). Bioenergetic role of mitochondrial fusion and fission. Biochimica Biophysica Acta (BBA) - Bioenergetics 1817 (10), 1833–1838. doi:10.1016/j.bbabio.2012.02.033
Wiese, M., and Bannister, A. J. (2020). Two genomes, one cell: Mitochondrial-nuclear coordination via epigenetic pathways. Mol. Metab. 38, 100942. doi:10.1016/j.molmet.2020.01.006
Williams, J. B., Pang, D., Delgado, B., Kocherginsky, M., Tretiakova, M., Krausz, T., et al. (2009). A model of gene-environment interaction reveals altered mammary gland gene expression and increased tumor growth following social isolation. Cancer Prev. Res. 2 (10), 850–861. doi:10.1158/1940-6207.CAPR-08-0238
Wong, C. O., and Venkatachalam, K. (2019). Motor neurons from ALS patients with mutations in C9ORF72 and SOD1 exhibit distinct transcriptional landscapes. Hum. Mol. Genet. 28 (16), 2799–2810. doi:10.1093/hmg/ddz104
Woo, M., Kim, M. J., and Song, Y. O. (2018). Bioactive compounds in kimchi improve the cognitive and memory functions impaired by amyloid beta. Nutrients 10, 1554. doi:10.3390/nu10101554(10)
Woodhouse, C. A., Patel, V. C., Singanayagam, A., and Shawcross, D. L. (2018). Review article: The gut microbiome as a therapeutic target in the pathogenesis and treatment of chronic liver disease. Aliment. Pharmacol. Ther. 47 (2), 192–202. doi:10.1111/apt.14397
Wu, X., Zhu, L. I., Zilbering, A., Mahadev, K., Motoshima, H., Yao, J., et al. (2005). Hyperglycemia potentiates H 2 O 2 production in adipocytes and enhances insulin signal transduction: Potential role for oxidative inhibition of thiol-sensitive protein-tyrosine phosphatases. Antioxid. Redox Signal 7, 526–537. doi:10.1089/ars.2005.7.526
Xiang, L., Shao, Y., and Chen, Y. (2021). Mitochondrial dysfunction and mitochondrion-targeted therapeutics in liver diseases. J. Drug Target 29 (10), 1080–1093. doi:10.1080/1061186X.2021.1909051
Xiong, Y., Hong, H., Liu, C., and Zhang, Y. Q. (2022). Social isolation and the brain: Effects and mechanisms. Mol. Psychiatry 28, 191–201. doi:10.1038/s41380-022-01835-w
Xu, Y., Liu, Z., Xu, S., Li, C., Li, M., Cao, S., et al. (2022). Scientific evidences of calorie restriction and intermittent fasting for neuroprotection in traumatic brain injury animal models: A review of the literature. Nutrients 14 (7), 1431. doi:10.3390/nu14071431
Yadav, E., Yadav, P., Khan, M. M. U., Singh, H. O., and Verma, A. (2022). Resveratrol: A potential therapeutic natural polyphenol for neurodegenerative diseases associated with mitochondrial dysfunction. Front. Pharmacol. 13, 922232. doi:10.3389/fphar.2022.922232
Yang, D., and Kim, J. (2019). Mitochondrial retrograde signalling and metabolic alterations in the tumour microenvironment. Cells 8 (3), 275. doi:10.3390/cells8030275
Yang, Y., Lei, W., Zhao, L., Wen, Y., and Li, Z. (2022). Insights into mitochondrial dynamics in chlamydial infection. Front. Cell Infect. Microbiol. 12, 835181. doi:10.3389/fcimb.2022.835181
Yang, Z., Zhao, T., Zou, Y., Zhang, J. H., and Feng, H. (2014). Curcumin inhibits microglia inflammation and confers neuroprotection in intracerebral hemorrhage. Immunol. Lett. 160 (1), 89–95. doi:10.1016/j.imlet.2014.03.005
Yapa, N. M. B., Lisnyak, V., Reljic, B., and Ryan, M. T. (2021). Mitochondrial dynamics in health and disease. FEBS Lett. 595 (8), 1184–1204. doi:10.1002/1873-3468.14077
Yi, H., Peng, H., Wu, X., Xu, X., Kuang, T., Zhang, J., et al. (2021). The therapeutic effects and mechanisms of quercetin on metabolic diseases: Pharmacological data and clinical evidence. Oxid. Med. Cell Longev. 2021, 6678662. doi:10.1155/2021/6678662
Yi, M., Weaver, D., and Hajnóczky, G. (2004). Control of mitochondrial motility and distribution by the calcium signal: A homeostatic circuit. J. Cell Biol. 167 (4), 661–672. doi:10.1083/jcb.200406038
Young, A., Oldford, C., and Mailloux, R. J. (2020). Lactate dehydrogenase supports lactate oxidation in mitochondria isolated from different mouse tissues. Redox Biol. 28, 101339. doi:10.1016/j.redox.2019.101339
Yu, S. B., and Pekkurnaz, G. (2018). Mechanisms orchestrating mitochondrial dynamics for energy homeostasis. J. Mol. Biol. 430, 3922–3941. doi:10.1016/j.jmb.2018.07.027
Yuan, X., Logan, T. M., and Ma, T. (2019). Metabolism in human mesenchymal stromal cells: A missing link between hmsc biomanufacturing and therapy? Front. Immunol. 10, 977. doi:10.3389/fimmu.2019.00977
Yun, J., and Finkel, T. (2014). Mitohormesis. Cell Metab. 19, 757–766. doi:10.1016/j.cmet.2014.01.011
Zampino, M., Brennan, N. A., Kuo, P. L., Spencer, R. G., Fishbein, K. W., Simonsick, E. M., et al. (2020). Poor mitochondrial health and systemic inflammation? Test of a classic hypothesis in the Baltimore longitudinal study of aging. Geroscience 42 (4), 1175–1182. doi:10.1007/s11357-020-00208-x
Zang, B. Y., He, L. X., and Xue, L. (2022). Intermittent fasting: Potential bridge of obesity and diabetes to health? Nutrients 14 (5), 981. doi:10.3390/nu14050981
Zeraattalab-Motlagh, S., Jayedi, A., and Shab-Bidar, S. (2021). The effects of resveratrol supplementation in patients with type 2 diabetes, metabolic syndrome, and nonalcoholic fatty liver disease: An umbrella review of meta-analyses of randomized controlled trials. Am. J. Clin. Nutr. 114 (5), 1675–1685. doi:10.1093/ajcn/nqab250
Zeviani, M., and Viscomi, C. (2022). Mitochondrial neurodegeneration. Cells 11 (4), 637. doi:10.3390/cells11040637
Zgorzynska, E., Dziedzic, B., and Walczewska, A. (2021). An overview of the Nrf2/ARE pathway and its role in neurodegenerative diseases. Int. J. Mol. Sci. 22, 9592. doi:10.3390/ijms22179592
Zhang, L., Jia, R., Li, H., Yu, H., Ren, K., Jia, S., et al. (2021). Insight into the double-edged role of ferroptosis in disease. Biomolecules 11, 1790. doi:10.3390/biom11121790
Zhang, S. F., Gao, J., and Liu, C. M. (2019). The role of non-coding RNAs in neurodevelopmental disorders. Front. Genet. 10, 1033. doi:10.3389/fgene.2019.01033
Zhang, T., Cui, Y., Wu, Y., Meng, J., Han, L., Zhang, J., et al. (2022). Mitochondrial GCN5L1 regulates glutaminase acetylation and hepatocellular carcinoma. Clin. Transl. Med. 12 (5), e852. doi:10.1002/ctm2.852
Zhang, X., Agborbesong, E., and Li, X. (2021). The role of mitochondria in acute kidney injury and chronic kidney disease and its therapeutic potential. Int. J. Mol. Sci. 22, 11253. doi:10.3390/ijms222011253
Zhang, X. W., Chen, J. Y., Ouyang, D., and Lu, J. H. (2020). Quercetin in animal models of alzheimer’s disease: A systematic review of preclinical studies. Int. J. Mol. Sci. 21, 493. doi:10.3390/ijms210204932
Zhang, Y., Zhang, C., Li, J., Jiang, M., Guo, S., Yang, G., et al. (2022). Inhibition of AKT induces p53/SIRT6/PARP1-dependent parthanatos to suppress tumor growth. Cell Commun. Signal. 20 (1), 93. doi:10.1186/s12964-022-00897-1
Zhao, F., Ci, X., Man, X., Li, J., Wei, Z., and Zhang, S. (2021). Food-derived pharmacological modulators of the Nrf2/ARE pathway: Their role in the treatment of diseases. Molecules 26, 1016. doi:10.3390/molecules26041016
Zhong, J., Rajaram, N., Brizel, D. M., Frees, A. E., Ramanujam, N., Batinic-Haberle, I., et al. (2013). Radiation induces aerobic glycolysis through reactive oxygen species. Radiotherapy Oncol. 106 (3), 390–396. doi:10.1016/j.radonc.2013.02.013
Zhu, J., Wang, H., and Jiang, X. (2022). mTORC1 beyond anabolic metabolism: Regulation of cell death. J. Cell Biol. 221 (12), 221. doi:10.1083/jcb.202208103
Zhu, Y., Li, X., Wang, L., Hong, X., and Yang, J. (2022). Metabolic reprogramming and crosstalk of cancer-related fibroblasts and immune cells in the tumor microenvironment. Front. Endocrinol. 13, 988295. doi:10.3389/fendo.2022.988295
Zhu, Y., Li, Y., Zhang, Q., Song, Y., Wang, L., and Zhu, Z. (2022). Interactions between intestinal microbiota and neural mitochondria: A new perspective on communicating pathway from gut to brain. Front. Microbiol. 13, 798917. doi:10.3389/fmicb.2022.798917
Zoico, E., Rubele, S., De Caro, A., Nori, N., Mazzali, G., Fantin, F., et al. (2019). Brown and beige adipose tissue and aging. Front. Endocrinol. (Lausanne) 10 (JUN), 1–10. doi:10.3389/fendo.2019.00368
Glossary
Keywords: mitochondria, mitochondrial dysfunction, mitochondrial metabolism, mitochondrial hormesis, hormesis
Citation: Casanova A, Wevers A, Navarro-Ledesma S and Pruimboom L (2023) Mitochondria: It is all about energy. Front. Physiol. 14:1114231. doi: 10.3389/fphys.2023.1114231
Received: 05 December 2022; Accepted: 29 March 2023;
Published: 25 April 2023.
Edited by:
Carlos Palmeira, University of Coimbra, PortugalReviewed by:
Jesús Rodríguez Huertas, University of Granada, SpainRicardo Marques, University of Coimbra, Portugal
Heather M. Wilkins, University of Kansas Medical Center Research Institute, United States
Copyright © 2023 Casanova, Wevers, Navarro-Ledesma and Pruimboom. This is an open-access article distributed under the terms of the Creative Commons Attribution License (CC BY). The use, distribution or reproduction in other forums is permitted, provided the original author(s) and the copyright owner(s) are credited and that the original publication in this journal is cited, in accordance with accepted academic practice. No use, distribution or reproduction is permitted which does not comply with these terms.
*Correspondence: Santiago Navarro-Ledesma, c25sQHVnci5lcw==