- College of Animal Sciences (College of Bee Science), Fujian Agriculture and Forestry University, Fuzhou, China
The detrimental impact of obesity on human health is increasingly evident with the rise in obesity-related diseases. Skeletal muscle, the crucial organ responsible for energy balance metabolism, plays a significant role as a secretory organ by releasing various myokines. Among these myokines, interleukin 6 (IL-6) is closely associated with skeletal muscle contraction. IL-6 triggers the process of lipolysis by mobilizing energy-storing adipose tissue, thereby providing energy for physical exercise. This phenomenon also elucidates the health benefits of regular exercise. However, skeletal muscle and adipose tissue maintain a constant interaction, both directly and indirectly. Direct interaction occurs through the accumulation of excess fat within skeletal muscle, known as ectopic fat deposition. Indirect interaction takes place when adipose tissue is mobilized to supply the energy for skeletal muscle during exercise. Consequently, maintaining a functional balance between skeletal muscle and adipose tissue becomes paramount in regulating energy metabolism and promoting overall health. IL-6, as a representative cytokine, participates in various inflammatory responses, including non-classical inflammatory responses such as adipogenesis. Skeletal muscle influences adipogenesis through paracrine mechanisms, primarily by secreting IL-6. In this research paper, we aim to review the role of skeletal muscle-derived IL-6 in lipid metabolism and other physiological activities, such as insulin resistance and glucose tolerance. By doing so, we provide valuable insights into the regulatory function of skeletal muscle-derived myokines in lipid metabolism.
1 Introduction
Currently, the global prevalence of obesity and its associated complications, including cardiovascular and metabolic diseases (Haslam and James, 2005), non-alcoholic fatty liver disease (NAFLD) (Pierantonelli and Svegliati-Baroni, 2019), and type 2 diabetes mellitus (T2DM) (Younossi et al., 2020), continues to rise. These complications pose significant health risks and pose a serious threat to human wellbeing (Cao et al., 2022). Alarmingly, the rates of obesity among children and adolescents have shown a substantial increase from 1975 to 2016 (Lin et al., 2022a). The root causes of obesity can be attributed to the excessive proliferation of adipocytes and the subsequent expansion of adipose tissue (Lin et al., 2022b). With the development of obesity, adipose tissue, particularly subcutaneous adipose tissue, exhibits a remarkable capacity to expand to adapt to energy storage demand requirements through a combination of adipocyte hypertrophy and hyperplasia (Pellegrinelli et al., 2016). Consequently, hyperplastic adipocytes undergo further differentiation and accumulation of lipid droplets, particularly in non-adipose tissue sites, which constitute ectopic lipid deposition (ELD) (Girousse et al., 2019). Skeletal muscle is a common tissue that is widely documented by ELD (Szendroedi and Roden, 2009; de Vries et al., 2014).
The skeletal muscle, as the most critical organ involved in regulating whole-body glucose homeostasis, responds sensitively to insulin (Samuel and Shulman, 2012; Agrawal et al., 2017). It is also an important secretory tissue, which synthesizes and secretes massive myokines involved in various physical activities. Interleukin 6 (IL-6) refers to a potent myokine (Huh, 2018; Severinsen and Pedersen, 2020). It is also a pro-inflammatory cytokine that is secreted by T cells and is considered necessary for the terminal differentiation of B cells (Martínez-Maza and Berek, 1991). With further research, more tissues or organs that can secrete IL-6, including muscles (Febbraio and Pedersen, 2005; Airi et al., 2018; Zhou et al., 2019), have been identified. Skeletal muscle contraction is the primary mode of exercise. According to reports, exercise stimulates IL-6 secretion in the central nervous system and promotes fatty acid oxidation in skeletal muscle by inducing extracellular signal-regulated kinase 1/2 (ERK1/2) phosphorylation (Steensberg et al., 2002; Katashima et al., 2022). Furthermore, the secretion levels of plasma IL-6 increase with skeletal muscle contraction (Keller et al., 2001; Steensberg et al., 2001).
Studies show that IL-6 leads to increased insulin-stimulated glucose disposal uptake, lipolysis, glucose, fatty acid oxidation, and energy expenditure when injected into healthy humans (van Hall et al., 2003; Carey et al., 2006). The physiological role of IL-6 is complex because its characteristics on metabolism require signal integration among different cell types (Scheller et al., 2011; Schmidt-Arras et al., 2016). Moreover, IL-6 has been implicated in promoting increased leptin secretion while suppressing satiety; this interaction promotes adipose tissue lipolysis (Feingold et al., 1992; Pedersen et al., 2003; Wueest and Konrad, 2018). IL-6 increases insulin secretion through an incretin-based mechanism. Indeed, IL-6 tissue-specific knockout mice, including those of the liver, skeletal muscle, and brain, further identify the important role it plays in obesity response (Ellingsgaard et al., 2011; Ferrer et al., 2014; Knudsen et al., 2016; Fernández-Gayol et al., 2019).
Skeletal muscle accounts for 40% of the total body weight; hence, it is the largest organ in the human body, and it acts on health regulation as an endocrine organ (Pedersen et al., 2003). Skeletal muscle also influences the differentiation and proliferation of adipocytes by the myokine through a paracrine mode. Therefore, this review discusses the relationship and function of muscle-derived IL-6 in lipid metabolism, focusing on IL-6 signaling and lipid metabolism in adipose tissue.
2 Signaling of IL-6
2.1 Classical signaling of IL-6
IL-6 is a phosphorylated glycoprotein consisting of a single chain composed of 184 amino acids. It features four-helix bundles (A-D), with A and B helices running in one direction while C and D helices run in the opposite direction (Kishimoto, 2010; Chen et al., 2022). It is synthesized by fibroblasts, monocytes, macrophages, T cells, endothelial cells, adipocytes, and myoblasts (Mihara et al., 2012).
IL-6 acts on target cells by binding to the interleukin 6 receptor α (IL-6Rα), which is distributed on the surface of the cell membrane. However, it does not signal competence. Initiating signaling requires the association of the IL-6/IL-6Rα complex with glycoprotein 130 (gp130), which is also known as the interleukin-6 receptor subunit (IL-6ST) that acts as the second receptor protein (Kishimoto, 2005). Specifically, IL-6 first binds to IL-6Rα on the surface of the cell membrane, thereby forming a dimer that creates a high affinity for transmembrane gp130, thus aggregating into an IL-6-IL-6Rα-gp130 trimer. Each of the two trimers further forms a homodimer. IL-6 within a single trimer binds to the D1 domain of gp130 within another trimer, thereby further forming a signal-transducing hexameric receptor complex (Boulanger et al., 2003). Notably, prior to the hexamer formation, IL-6 must first be complexed with IL-6Rα, binding to the gp130 receptor for signal transduction (Taga et al., 1989). Subsequently, the trimer activates mitogen-activated protein kinase (MAPK), phosphatidylinositide-3-kinase (PI3K), Janus kinases (JAKs), and signal transducer and activator of transcription (STATs) signaling cascades (Manore et al., 2022). Furthermore, the formation of the IL-6-IL-6Rα-gp130 hexamer recruits the JAK family of non-receptor tyrosine kinases, including JAK1/2 and tyrosine kinase 2 (TYK2), to the cell membrane, which binds to and phosphorylates gp130s cytoplasmic tail at five tyrosine residues (e.g., Y759, Y767, Y814, Y905, and Y915) (Guschin et al., 1995).
After undergoing phosphorylation, gp130 functions as a docking site for two transcription factors: signal transducer and activator of transcription 1(STAT1) and 3 (STAT3). They are phosphorylated by JAKs at Y701 and Y705 of gp130, respectively (Shuai et al., 1993; Kaptein et al., 1996). Notably, the signaling activation of IL-6/IL-6R/gp130 for STAT3 is more effective than that for STAT1 (Haan et al., 2005). Subsequently, STAT3 is phosphorylated, and its conformation undergoes a change, detaching from the receptor complex and homodimerizing, thereby allowing STAT3 translocation into the nucleus and promoting transcriptional activation for target genes (Morris et al., 2018) (Figure 1).
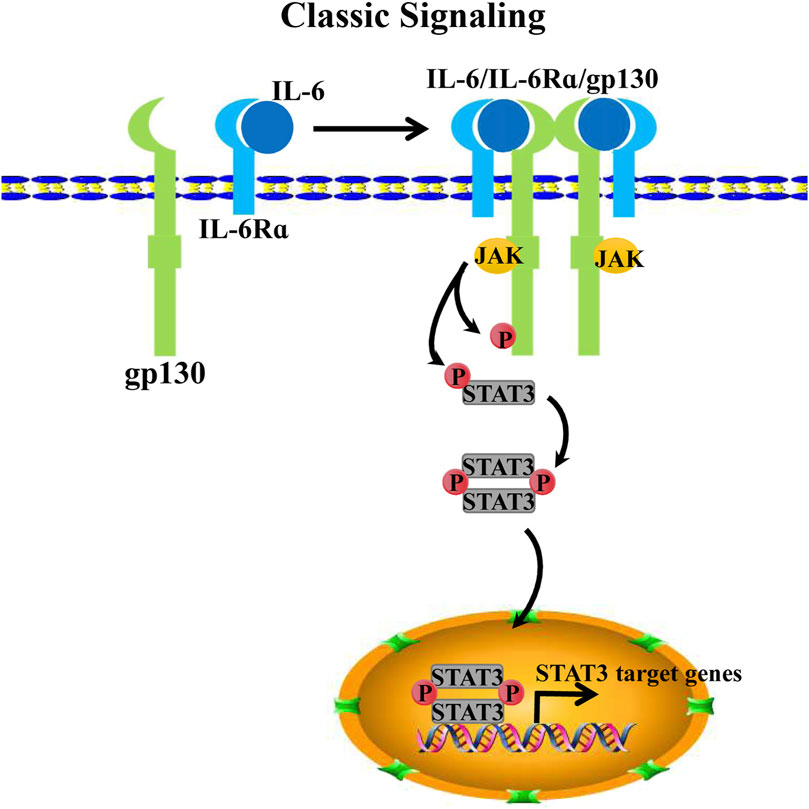
FIGURE 1. IL-6 classical signaling. IL-6 binds IL-6Rα as a dimeric complex to further form a trimeric receptor complex with pg130. Two IL-6/IL-6Ra/gp130 complexes form a hexameric receptor complex by binding via gp130s D1 domain, thereby activating the intracellular JAK/STAT3 pathway. Recruiting JAKs to the membrane and phosphorylate the cytoplasmic tail of gp130 and STAT3. Phosphorylated STAT3 homodimerize translocate into the nucleus for target transcription activation.
However, considering that IL-6 has no direct binding capacity to gp130, the expression of IL-6Rα on the cell membrane surface becomes the main limiting factor for IL-6/IL-6Rα/gp130 signaling, which acts as the activation for STAT3 phosphorylation (Taga et al., 1989). Interestingly, the expression of membrane-bound IL-6Rα is restricted to only a few cell types, including immune cells, macrophages, B cells, and subtypes of T cells (Nishimoto and Kishimoto, 2006; Rose-John et al., 2006). Meanwhile, transmembrane gp130 is almost expressed in all cell types (Oberg et al., 2006; Dmitrieva et al., 2016). Considering that IL-6Rα expression is restricted to immune cells and although IL-6 acts with pleiotropic regulatory effects, the non-classical signal outside membrane-bound receptors is subsequently identified as trans-signaling (Taga et al., 1989; Müllberg et al., 1993b).
2.2 Trans-signaling of IL-6
The key mediator of IL-6 trans-signaling is a soluble interleukin 6 receptor α (sIL-6Rα), which potentiates trans-signaling in cells that lack sufficient membrane-bound IL-6Rα expression (Taga et al., 1989; Müllberg et al., 1993b). SIL-6Rα is initially detected in the serum and urine of humans, which is considered an agonist for IL-6 signaling. However, with further research, IL-6-sIL-6Rα-gp130 has been identified as a new alternative form of IL-6 signaling (Müllberg et al., 1993b; Toniatti et al., 1996). To date, sIL-6Rα product models include either the proteolytic cleavage of membrane-bound IL-6α or the alternative splicing of IL-6α pre-mRNA (Müllberg et al., 1993a; Müller-Newen et al., 1996; Oh et al., 1996). Specifically, upon proteolysis, or ectodomain shedding, membrane-bound IL-6α produces sIL-6α by a disintegrin and metalloproteinase family proteins ADAM10 or ADAM17 (Lust et al., 1992; Garbers et al., 2011; Schumacher et al., 2015). Extracellularly secreted IL-6 dimerizes with sIL-6Rα and then binds to transmembrane gp130 as a trimer. Subsequently, two IL-6-sIL-6Rα-gp130 trimers further homodimerize to activate downstream JAK/STAT3 signaling (Manore et al., 2022) (Figure 2). Notably, gp130 is also present in soluble gp130 form (sgp130). Interestingly, sgp130 acts on IL-6-sIL6Rα dimers as an antagonist to IL-6-sIL-6Rα-gp130, thereby inhibiting IL-6 trans-signaling but not impacting IL-6 classical signaling. However, sgp130 is barely expressed compared with sIL-6Rα (Müllberg et al., 1993a; Jostock et al., 2001).
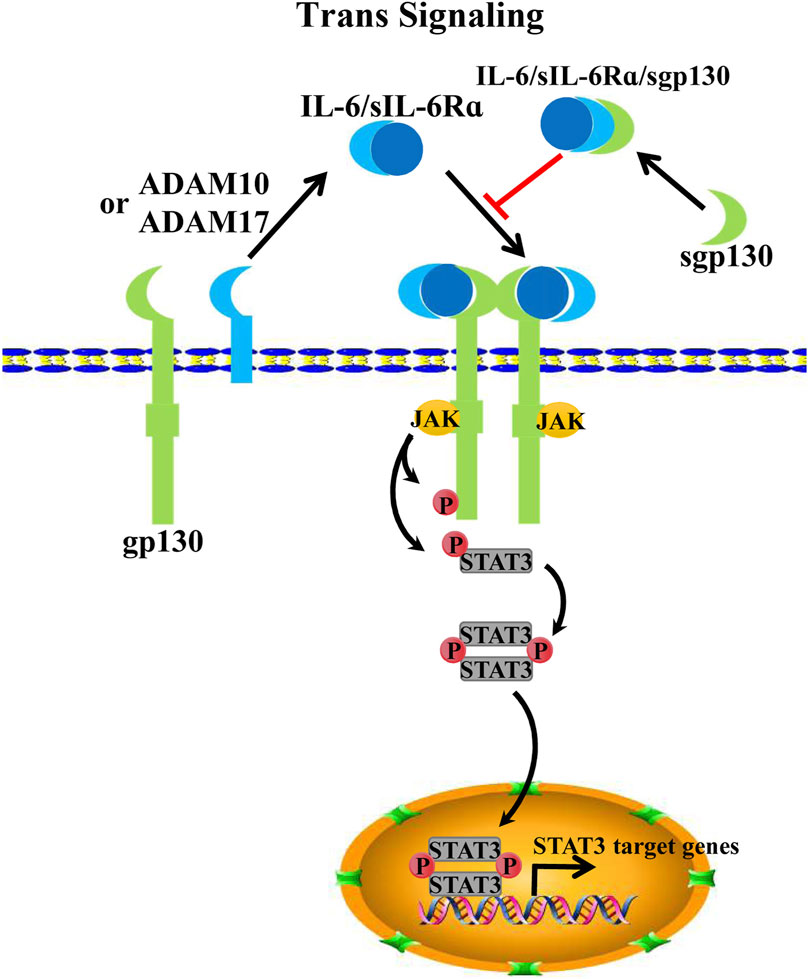
FIGURE 2. IL-6 trans-signaling. Alternative splicing or proteolysis of IL-6 mRNA by ADAM10/17 can form sIL-6Rα; IL-6 binds sIL-6Rα and forms an IL-6/sIL-6Rα/pg130 hexameric complex for signal transduction to activate JAK/STAT3 signaling. Spg130, on the other hand, usually acts as an antagonist to IL-6/sIL-6Rα, thereby inhibiting IL-6/sIL-6Rα/pg130 signaling.
Compared with the classical IL-6/IL-6Rα, which almost only exists in immune cells that mediate the immune response, the trans-signaling of IL-6 acts more widely on physiological functions. For immune response, IL-6 trans-signaling mediates pro-inflammatory responses by recruiting mononuclear cells, promoting endothelial cells and T-cell survival, and inhibiting T-cell differentiation (McLoughlin et al., 2005; Schaper and Rose-John, 2015). Moreover, IL-6 trans-signaling is involved in adipogenesis (Huang et al., 2018), especially in the development of various cancers (Schumacher and Rose-John, 2022). Given that IL-6 trans-signaling broadly mediates the pro-inflammatory response, it has been referred to as the main molecular mechanism of IL-6 that acts on tumorigenesis in multiple cancers (McLoughlin et al., 2005; Böttcher et al., 2014).
2.3 Cluster signaling of IL-6
In addition to the two aforementioned IL-6 signaling mechanisms, Heink identified a third IL-6 signaling mechanism in 2017. This IL-6 signaling model involves the interaction between two cognate cells, which is referred to as trans-presentation or “cluster signaling” (Heink et al., 2017). Specifically, IL-6 dimerizes with the membrane-bound IL-6Rα of dendritic cells (DCs) and then binds to the gp130 receptor of T helper 17 cells (TH17). Generally, dendritic cells that provide membrane-bound IL-6Rα are referred to as “donating cells” or “transmitting cells” whereas the T cell that receives the gp130 receptor is generally defined as a “receiving cell” (Chou et al., 2022; Manore et al., 2022). In co-cultured experiments with dendritic cells and T cells, STAT3 signaling activation was in T cells because soluble glycoprotein 130 (sgp130) usually acts as an antagonist to IL-6-IL-6Rα dimer in trans-signaling. To investigate whether sgp130 also inhibits “cluster signaling,” Heink et al. detected the function of sgp130 for IL-6-IL-6Rα dimer in cluster signaling. The results showed that sgp130 did not neutralize this signaling model. However, new evidence shows that sgp130 suppresses IL-6 trans-presentation signaling by neutralizing the IL-6-IL-6Rα dimer (Bergmann et al., 2017; Lamertz et al., 2018) (Figure 3). Given the contradiction between these findings, the mechanism of IL-6 trans-presentation and its biological role remain to be characterized and explored further.
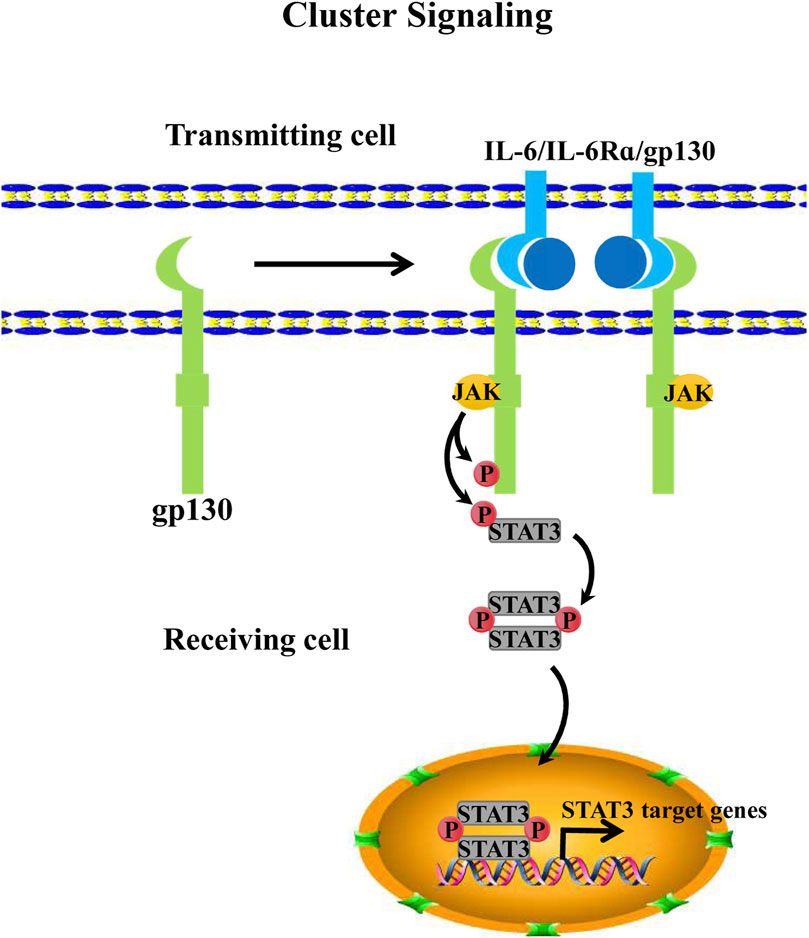
FIGURE 3. IL-6 cluster signaling. IL-6 cluster signaling consists of 2 cells. The cell that provides IL-6Rα that is binding to IL-6 is usually defined as a “transmitting cell”. Then the dimeric complex forms the trimeric one with pg130 that comes from another cell, this cell is also called the “receiving cell.” Subsequently, the IL-6/IL-6Rα/pg130 complex further activates the intracellular JAK/STAT3 pathway of the “receiving cell”.
3 IL-6 as a myokine
Skeletal muscle is a crucial organ for maintaining body movement and glucose homeostasis; moreover, it is a secretory organ that acts on multiple physiological activities by the myokines, for example, muscle hypertrophy, fat oxidation, lipolysis, glucose homeostasis, insulin secretion, anti-inflammation, angiogenesis, and bone formation (Pedersen and Febbraio, 2012; Benatti and Pedersen, 2015). Some factors that affect skeletal muscle secretion include diet, exercise, type of myofiber, and genetic factors. For example, a high-fat diet increases the level of saturated fatty acids in the blood, which harms muscle protein synthesis and muscle fiber regeneration while increasing the level of reactive oxygen species (ROS), thereby accelerating proteasome-mediated protein degradation (Woodworth-Hobbs et al., 2014; Brown et al., 2015; Ji, 2015). Exercise remains another factor that affects secreted myokines in skeletal muscles (Sharif et al., 2017; Rosanna, 2019). Among numerous myokines, IL-6 is one of the most strongly associated with exercise. During exercise, serum IL-6 concentrations increased almost 100-fold compared with baseline (Pedersen et al., 2000; Helvoort et al., 2005). Notably, plasma IL-6 levels are associated with exercise duration, training intensity, and the amount of muscle mass mobilized by exercise; specifically, exercise duration is the only determinant of IL-6 release levels. The underlying molecular mechanism of exercise-induced IL-6 secretion by skeletal muscle is the outflow of Ca2+ ions. Specifically, Ca2+ ions within the sarcoplasmic reticulum liberate the cytoplasm of a skeletal muscle cell during skeletal muscle contraction (Olson and Williams, 2000). More importantly, evidence suggests that Ca2+ ion activated nuclear factor kβ (NF-kβ), c-Jun amino-terminal kinase (JNK), and nuclear factor of activated T cells (NFAT) (Dolmetsch et al., 1998). NF-kβ and JNK are activators of the IL-6 promoter, thereby inducing its transcription (Tuyt et al., 1999). In addition, training intensity involves the depletion of intramuscular glycogen and energy storage. Specifically, the working muscle results in low glycogen (Steensberg et al., 2001; Pedersen and Fischer, 2007; Hojman et al., 2019), which induces p38/MAP kinase (p38/MAPK) to increase, thereby promoting IL-6 transcription (Boppart et al., 2000; Craig et al., 2000; Chae et al., 2001). Meanwhile, skeletal muscle contraction produces many ROS during exercise. As reported, ROS further induced muscle-derived IL-6 secretion by activating nuclear factor kβ (NF-kβ) signaling, which acts as a transcriptional role for IL-6 (Yamagishi et al., 1997; Bierhaus et al., 2001; Vo et al., 2021) (Figure 4). Exercise-mediated muscle-derived IL-6 is considered the muscle energy sensor that stimulates the release of free fatty acids from lipolysis and adipose tissue, as well as glycogenolysis in the liver that promotes the release of glucose (Pedersen and Febbraio, 2008; Hojman et al., 2019).
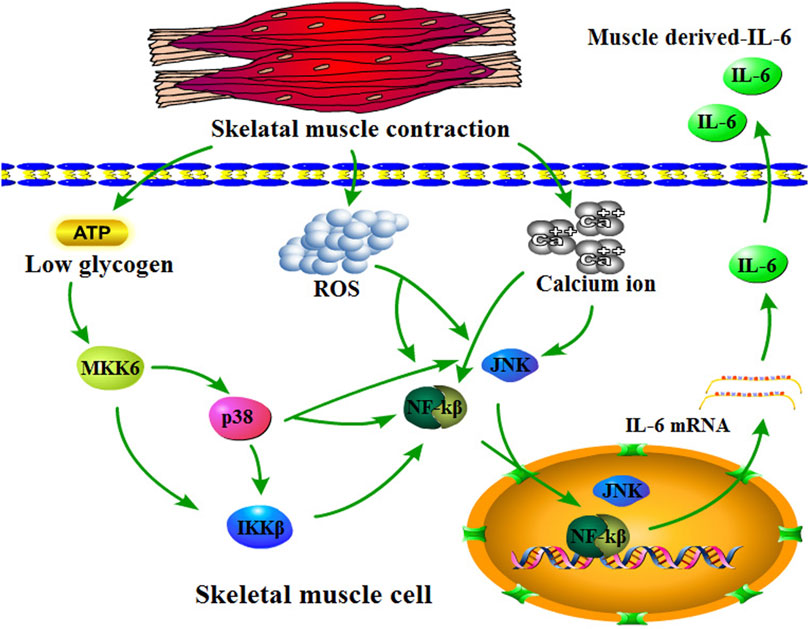
FIGURE 4. Overview of skeletal muscle-derived IL-6. Skeletal muscle contraction consumes ATP resulting in low glycogen in skeletal muscle cells, which promotes MKK6 to activate p38/IKKβ/NF-kβ or p38/JNK signaling. Moreover, skeletal muscle contraction also upregulates reactive oxygen concentration and calcium ions, which promote NF-kβ and JNK. NF-kβ and JNK translocate into the nucleus for IL-6 transcription activation.
Furthermore, muscle-derived IL-6 is released into circulation, acting on other organs or tissues in a hormonal fashion to play various regulatory roles, such as immune response (Fischer, 2007). IL-6 is classified as a pro-inflammatory cytokine. However, with the development of research, IL-6 was shown to be gradually involved in anti-inflammatory effects (Nara and Watanabe, 2021), especially muscle-derived IL-6 (Mauer et al., 2014).
Besides, muscle-derived IL-6 is involved in regulating physiological activities in other organs or tissues, such as suppressing brain-induced appetite (Schöbitz et al., 1995; Cao et al., 2015), inducing hepatic glucose production (Fritsche et al., 2010; Clementi et al., 2011), glucose oxidation and lipolysis for skeletal muscle and adipose tissue (Hardie et al., 1999; Han et al., 2020), inducing bone mass and mineral density (Barbour et al., 2014; Yokota et al., 2021), and so on (Figure 5).
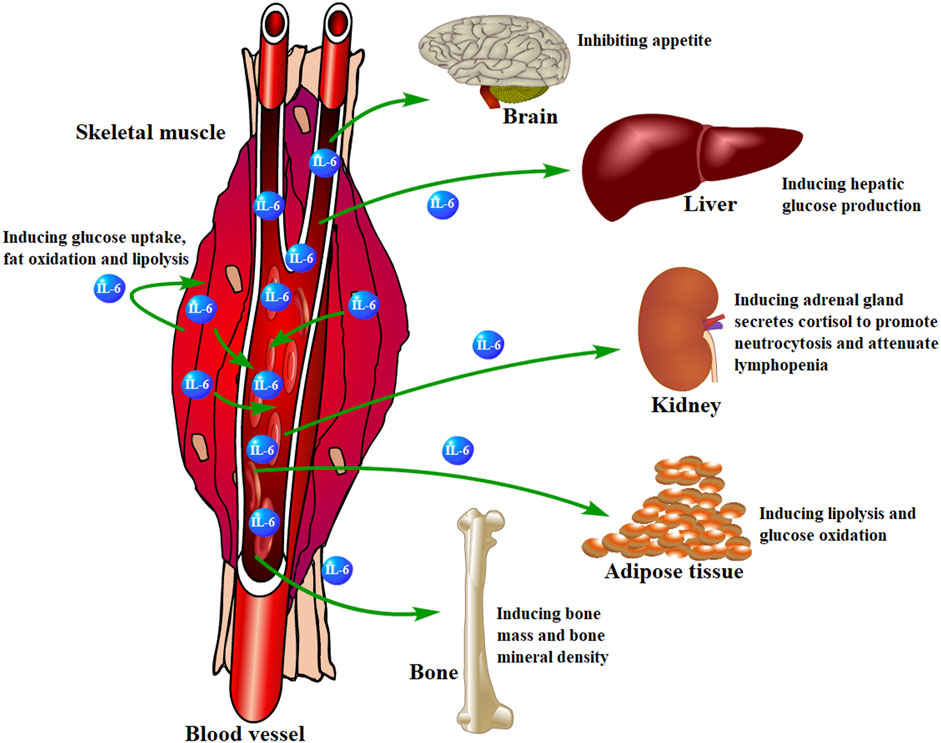
FIGURE 5. Skeletal muscle-derived IL-6 regulates organs or tissues. Skeletal muscle-derived IL-6 is released into circulation and acts on other organs or tissues in a hormonal fashion to play various regulatory roles.
4 IL-6 as an adipokine
Adipose tissue, as an important secreted organ that is involved in a series of physiological regulations, is a significant IL-6-secreted organ. With the development of obesity, cell types of innate and adaptive immunity infiltrate obese white adipose tissue (WAT). Among the infiltrating cell types, macrophages are the major subset. Their polarization defines variable functions in obese WATs (Cancello et al., 2006; Murano et al., 2008; Caspar et al., 2009; Lauterbach and Wunderlich, 2017). Of note, adipose-derived TNF-α and IL-6 are crucial adipokines that suppress adipocyte insulin sensitivity and even lead to insulin resistance by attenuating insulin receptor-substrate 1 (IRS-1), which is a necessary component of insulin signaling (Hotamisligil et al., 1996; Rui et al., 2002). For example, TNF-α/tumor necrosis factor receptor 1 (TNFR1)/IRS-1 signaling is one of the most important regulatory axes for insulin resistance. Specifically, TNF-α binds TNFR1 further to activate intracellular c-JUN N terminal kinase (JNK) and IkB kinase (IKK) signals. The phosphorylated JNK (p-JNK) and IKK1/1KK2 further attenuate IRS-1 S307 residue phosphorylation, thereby suppressing insulin signaling activation to induce insulin resistance (Hotamisligil et al., 1996; Peraldi et al., 1996; Liang et al., 2008; Tessaro et al., 2017) (Figure 6). Meanwhile, the signaling axis that IL-6 regulates insulin resistance is considered, IL-6/IL-6Rα/gp130 signaling activates JAK/STAT3 phosphorylation, and p-STAT3 further induces the inhibition of cytokine signaling 3 (SOCS3) gene transcription by binding to its promoter, whereas SOCS3 is an inhibitor of IL-6/JAK/STAT3 signaling. Moreover, SOCS3 ubiquitinates IRS-1 to induce its degradation, leading IL-6 to induce insulin resistance (Rui et al., 2002; Sachithanandan et al., 2010; Benito, 2011; Wiejak et al., 2012). (Figure 6) IL-6 secreted from adipose tissue accounts for 15%–35% of the body′s total circulating IL-6 (Mohamed-Ali et al., 1997). More interestingly, IL-6 visceral adipose tissue released three-fold outnumbered subcutaneous adipose tissue (Fried et al., 1998). However, although visceral adipose tissue is the main source of IL-6, subcutaneous adipose tissue-derived IL-6 plays a more important role in regulating glucose metabolism by mediating systemic insulin sensitivity (Bastard et al., 2002).
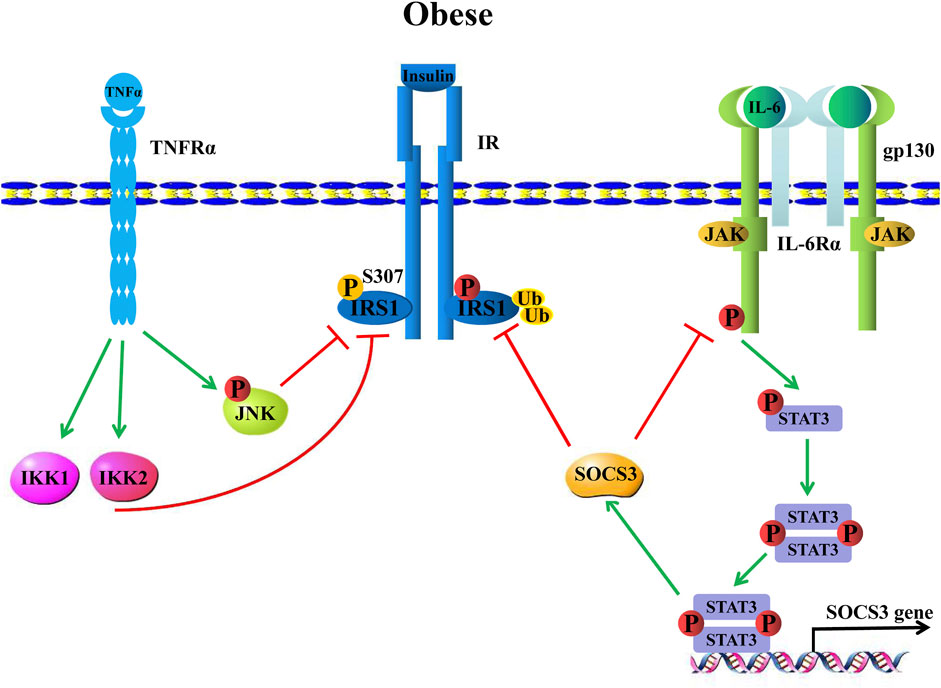
FIGURE 6. Obesity-induced inflammation causes insulin resistance. TNF-α binds the TNF-α receptor (TNF-Rα) to induce IKK and JNK signals that further attenuate insulin receptor substrate 1, IRS-1 phosphorylation, hence leading to insulin resistance. IL-6, on the other hand, upregulates SOCS3 expression through classical IL-6/JAK/STAT3 signaling, which promotes IRS-1 ubiquitination to induce IRS-1 degradation, thereby inhibiting IRS-1, resulting in insulin resistance.
Moreover, brown adipose tissue (BAT), as non-shivering thermogenesis, is an energy-consuming organ that belongs to another type of adipose tissue (Cannon and Nedergaard, 2004). Generally, BAT is an energy-mobilizing organ that promotes WAT lipolysis and oxidation (Blondin et al., 2014; Garretson et al., 2016; Blondin et al., 2017). BAT adipocytes are more closely related to myogenic cells than WAT adipocytes. BAT adipocytes and myogenic cells originate from Myf5+ positive progenitors, and PRDM16 determines myogenic or adipogenic differentiation (Seale et al., 2008; Martinez-Lopez et al., 2013). The structure of BAT adipocytes usually consists of numerous small multilocular lipid droplets and abundant mitochondria. Moreover, BAT adipocytes are smaller in size than WAT adipocytes (Frühbeck et al., 2009). Therefore, BAT acts on thermogenesis that is similar to skeletal muscle rather than WAT. However, BAT still characterizes parts of adipose properties, including inflammatory response (Gallerand et al., 2021), triglyceride storage (Scheja and Heeren, 2016), and secretion effect (Scheele and Wolfrum, 2020). Interestingly, among the types of factors that are BAT-derived or defined as batokines, those that contain IL-6, exploration identifies BAT-derived IL-6 as a necessary factor for improving glucose homeostasis (Stanford et al., 2013; Kalupahana et al., 2020).
5 Role of IL-6 in adipogenesis
Given the significant increase in global obesity rates, further exploring the molecular mechanism of adipose tissues is an urgent task for the treatment of obesity-related diseases. Numerous genes or regulators are involved in regulating adipogenesis. Among these genes and regulators, the most critical ones are CCAAT/enhancer binding protein (C/EBP) and peroxisome proliferator-activated receptor (PPAR) families (Lin et al., 2020; Lin et al., 2022a). In addition, other factors are involved in regulating adipogenesis. For example, IL-6 attenuates adipogenesis by lipolysis or fatty acid oxidation. IL-6 adipogenic classical signalings, which typically contain extracellular signal-regulated kinase 1/2 (ERK1/2) signaling, or adenosine monophosphate-activated protein kinase (AMPK) signaling and so on. For instance, IL-6 binds to IL-6R/gp130 and subsequently phosphorylates Raf-1 proto-oncogene, serine/threonine kinase (Raf1), which further induces MAP kinase/ERK kinase 1 and 2 (MEK1/2) phosphorylation, thereby activating ERK1/2 signaling (Ogasawara et al., 2010; Mihara et al., 2012; Latourte et al., 2017). Importantly, in adipocytes, ERK and JNK can phosphorylate peroxisome proliferator-activated receptor γ (PPARγ), thereby attenuating its transcriptional role and further repressing adipogenesis (Hu et al., 1996).
Furthermore, MEK/ERK signaling activation is followed by a decrease in the mRNA levels of phosphorylate peroxisome proliferator-activated receptor γ (PPARγ), glucose transporter 4 (GLUT4), fatty acid binding protein 4 (FABP4), and lipoprotein lipase (LPL), which are important adipogenic factors (Brown et al., 2004).
IL-6 also phosphorylates AMP-activated protein kinase (AMPKThr172) via IL-6/IL-6R/gp130 signaling (Kelly et al., 2004; Carey et al., 2006; Khan et al., 2012; Saini et al., 2014; Pacifici et al., 2020). AMPK is known to play a crucial role in lipolysis, which further induces the phosphorylation of acetyl-CoA carboxylase 1 (ACC1Ser79) (Luo et al., 2017; Katashima et al., 2022), and ACC1/2 are the limiting enzymes for fatty acid synthesis (Fullerton et al., 2013). AMPK induces phosphorylation to reduce the conversion of acetyl-CoA into malonyl-CoA, which is catalyzed by fatty acid synthase (FASN) to further synthesize the palmitate to form fatty acids so that the phosphorylation of AMPK-induced lipogenesis is attenuated (Saha and Ruderman, 2003; Migita et al., 2009; Day et al., 2017).
AMPK is also involved in increasing Wnt/β-catenin signaling. To put it concretely, AMPK induces the expression of β-catenin and nuclear accumulation in 3T3-L1 cells, which attenuates adipogenic gene expression, including fatty acid binding protein 4 (FABP4), CCAAT/enhancer binding protein α and β (C/EBPα and C/EBPβ), Fas cell surface death receptor (FAS), phosphorylate peroxisome proliferator-activated receptor γ (PPARγ), and sterol-regulatory element binding protein 1c (SREBP-1c) (Ducharme and Bickel, 2008; Lee et al., 2011). In addition, adipose triglyceride lipase (ATGL) is a critical triglyceride hydrolase, and AMPK has been demonstrated to mediate ATGL phosphorylation (Ser406), thereby further inducing triglycerides to hydrolyze into fatty acids (Frühbeck et al., 2014; Marzolla et al., 2020; Cho et al., 2021). Peroxisome-proliferator-activated receptor γ co-activator 1α (PGC-1α) has emerged as a master regulator of mitochondrial biogenesis, thus regulating glucose metabolism. AMPK mediates the phosphorylation of PGC-1α (Thr177/Ser538), which is required for PGC-1α-dependent induction of the PGC-1α promoter, thus mediating its transcription (Terada and Tabata, 2004; Lee et al., 2006; Jäger et al., 2007; Ramirez Reyes et al., 2021).
6 Role of IL-6 in lipid metabolic diseases
The greatest harm caused by obesity to humans is a series of metabolic diseases that extend from it. These diseases include but are not limited to cardiovascular and metabolic diseases, non-alcoholic fatty liver disease (NAFLD), and type Ⅱ diabetes mellitus (T2DM). Cardiovascular diseases, such as atherosclerotic disease, are primarily caused by lipid deposition in the blood vessel system, which is induced by high-fat food. Gradually, inflammation contributes to atherosclerosis pathogenesis (Libby and Hansson, 2019; O'Keefe, 2019; Tardif et al., 2019). Among thousands of cytokines, NOD-, LRR-, and pyrin domain-containing protein 3 (NLRP3), C-reactive protein (CRP), IL-1, and IL-6 were involved in atherosclerosis pathogenesis (Ridker, 2016; Lutgens et al., 2019; Ridker, 2019). CANTOS, a canakinumab anti-inflammatory thrombosis outcome study, demonstrated that inhibiting IL-1 and IL-6 can reduce the rates of cardiovascular events, especially IL-6, which is directly related to the risk of the occurrence of atherosclerosis (Ridker et al., 2017; Ridker et al., 2018). In particular, beyond IL-6, circulating sIL-R is associated with vascular events and cardiovascular mortality (Hedman et al., 2007; Ritschel et al., 2016).
Furthermore, non-alcoholic fatty liver disease (NAFLD) is a spectrum of liver disorders that usually consist of benign non-alcoholic fatty liver disease (NAFL) and serious non-alcoholic steatohepatitis (NASH). NAFLD is another type of ectopic fat deposition in the liver. It generally contains more than 5% steatosis hepatocytes (Sanyal et al., 2011; Cobbina and Akhlaghi, 2017). Generally, steatosis induces the activation of IKKβ/NF-kβ signaling, thereby promoting a series of pro-inflammatory mediators, including TNF-α, IL-1, and IL-6. They further induce the recruitment and activation of Kupffer cells to mediate inflammation in NASH (Ramadori and Armbrust, 2001; Joshi-Barve et al., 2007; Anderson and Borlak, 2008).
Figure 6 shows that IL-6 leads to insulin resistance, which is one of the most classic features of T2DM, caused by JAK/STAT3 signaling interfering with IRS-1 signaling. Therefore, plasma IL-6 level is one of the biomarkers of T2DM. The report shows that plasma IL-6 levels in T2DM patients are three times that of non-T2DM patients (Skuratovskaia et al., 2021). In obese mice, the plasma IL-6 levels were several fold times higher than those in lean mice (Li et al., 2010).
In summary, IL-6, as an important regulator, is involved in the regulatory process of various lipid metabolic diseases, implying that IL-6 acts as a potential therapeutic target that further plays an important medical role in the subsequent treatment of related diseases.
7 Conclusion
Obesity is associated with various metabolic diseases, such as T2DM, which is seriously harmful to human health. For T2DM, the occurrence of ectopic fat deposition in the skeletal muscle is closely related. However, the skeletal muscle serves not only as a vital organ that regulates the body′s energy metabolism but also as an important secret organ that mediates the synthesis and secretion of many myokines. Among these myokines, IL-6 is the most closely related to muscle contraction and it has been proven to mediate lipolysis. Understanding the roles of skeletal muscle-derived IL-6 in lipid mechanisms and other physiological activities is essential in comprehending the interaction between skeletal muscle and adipose tissue through muscle paracrine signaling. This understanding is especially valuable for further exploring the molecular mechanism underlying intramuscular fat deposition and for providing insights into the treatment of diseases associated with ectopic fat deposition.
Author contributions
WL and TX conceived the structure of the manuscript. WL and HS wrote the manuscript. JS, JC, and JW contributed to drawing the figures included in the manuscript. YuY, YiY, and RL implemented the complete manuscript check. RL and WL supported the project, supervised the project analysis, and contributed to manuscript preparation. All authors contributed to the article and approved the submitted version.
Funding
This project was supported by the Fujian Province Young and Middle-Aged Teacher Education Research Project (KLy22044XA), the Special Fund for Science and Technology Innovation of Fujian Agriculture and Forestry University (KFb22064XA), and the Natural Science Foundation of Fujian Province (2020J01537 and 2023J01446).
Conflict of interest
The authors declare that the research was conducted in the absence of any commercial or financial relationships that could be construed as a potential conflict of interest.
Publisher’s note
All claims expressed in this article are solely those of the authors and do not necessarily represent those of their affiliated organizations, or those of the publisher, the editors and the reviewers. Any product that may be evaluated in this article, or claim that may be made by its manufacturer, is not guaranteed or endorsed by the publisher.
References
Agrawal, M., Kern, P., and Nikolajczyk, B. (2017). The immune system in obesity: Developing paradigms amidst inconvenient truths. Curr. Diabetes Rep. 17 (10), 87. doi:10.1007/s11892-017-0917-9
Airi, O., Yuki, M., Rei, T., Tanuma, S. I., Kojima, S., and Tsukimoto, M. (2018). A novel mechanism of γ-irradiation-induced IL-6 production mediated by P2Y11 receptor in epidermal keratinocytes. Biol. Pharm. Bull. 41, 925–936. doi:10.1248/bpb.b18-00075
Anderson, N., and Borlak, J. (2008). Molecular mechanisms and therapeutic targets in steatosis and steatohepatitis. Pharmacol. Rev. 60 (3), 311–357. doi:10.1124/pr.108.00001
Barbour, K., Lui, L., Ensrud, K., Hillier, T., LeBlanc, E., Ing, S., et al. (2014). Inflammatory markers and risk of hip fracture in older white women: The study of osteoporotic fractures. J. Bone Mineral Res. 29 (9), 2057–2064. doi:10.1002/jbmr.2245
Bastard, J., Maachi, M., Van Nhieu, J., Jardel, C., Bruckert, E., Grimaldi, A., et al. (2002). Adipose tissue IL-6 content correlates with resistance to insulin activation of glucose uptake both in vivo and in vitro. J. Clin. Endocrinol. Metabolism 87 (5), 2084–2089. doi:10.1210/jcem.87.5.8450
Benatti, F. B., and Pedersen, B. K. (2015). Exercise as an anti-inflammatory therapy for rheumatic diseases—Myokine regulation. Nat. Rev. 11 (2), 86–97. doi:10.1038/nrrheum.2014.193
Benito, M. (2011). Tissue specificity on insulin action and resistance: Past to recent mechanisms. Acta Physiol. 201 (3), 297–312. doi:10.1111/j.1748-1716.2010.02201.x
Bergmann, J., Müller, M., Baumann, N., Reichert, M., Heneweer, C., Bolik, J., et al. (2017). IL-6 trans-signaling is essential for the development of hepatocellular carcinoma in mice. Hepatology 65 (1), 89–103. doi:10.1002/hep.28874
Bierhaus, A., Schiekofer, S., Schwaninger, M., Andrassy, M., Humpert, P., Chen, J., et al. (2001). Diabetes-associated sustained activation of the transcription factor nuclear factor-kappaB. Diabetes 50 (12), 2792–2808. doi:10.2337/diabetes.50.12.2792
Blondin, D., Frisch, F., Phoenix, S., Guérin, B., Turcotte, É., Haman, F., et al. (2017). Inhibition of intracellular triglyceride lipolysis suppresses cold-induced Brown adipose tissue metabolism and increases shivering in humans. Cell Metab. 25 (2), 438–447. doi:10.1016/j.cmet.2016.12.005
Blondin, D., Labbé, S., Tingelstad, H., Noll, C., Kunach, M., Phoenix, S., et al. (2014). Increased Brown adipose tissue oxidative capacity in cold-acclimated humans. J. Clin. Endocrinol. Metabolism 99 (3), 438–446. doi:10.1210/jc.2013-3901
Boppart, M., Asp, S., Wojtaszewski, J., Fielding, R., Mohr, T., and Goodyear, L. (2000). Marathon running transiently increases c-Jun NH2-terminal kinase and p38 activities in human skeletal muscle. J. Physiology 526, 663–669. doi:10.1111/j.1469-7793.2000.00663.x
Böttcher, J., Schanz, O., Garbers, C., Zaremba, A., Hegenbarth, S., Kurts, C., et al. (2014). IL-6 trans-signaling-dependent rapid development of cytotoxic CD8+ T cell function. Cell Rep. 8 (5), 1318–1327. doi:10.1016/j.celrep.2014.07.008
Boulanger, M. J., Chow, D. C., Brevnova, E. E., and Garcia, K. C. (2003). Hexameric structure and assembly of the interleukin-6/IL-6 alpha-receptor/gp130 complex. Science 300 (5628), 2101–2104. doi:10.1126/science.1083901
Brown, J., Boysen, M., Chung, S., Fabiyi, O., Morrison, R., Mandrup, S., et al. (2004). Conjugated linoleic acid induces human adipocyte delipidation: Autocrine/paracrine regulation of MEK/ERK signaling by adipocytokines. J. Biol. Chem. 279 (25), 26735–26747. doi:10.1074/jbc.M401766200
Brown, L., Lee, D., Patton, J., Perry, R., Brown, J., Baum, J., et al. (2015). Diet-induced obesity alters anabolic signalling in mice at the onset of skeletal muscle regeneration. Acta Physiol. 215 (1), 46–57. doi:10.1111/apha.12537
Cancello, R., Tordjman, J., Poitou, C., Guilhem, G., Bouillot, J., Hugol, D., et al. (2006). Increased infiltration of macrophages in omental adipose tissue is associated with marked hepatic lesions in morbid human obesity. Diabetes 55 (6), 1554–1561. doi:10.2337/db06-0133
Cannon, B., and Nedergaard, J. (2004). Brown adipose tissue: Function and physiological significance. Physiol. Rev. 84 (1), 277–359. doi:10.1152/physrev.00015.2003
Cao, H., Ye, X., Ma, J., Jiang, H., Li, S., Li, R., et al. (2015). Mimecan, a hormone abundantly expressed in adipose tissue, reduced food intake independently of leptin signaling. EBioMedicine 2 (11), 1718–1724. doi:10.1016/j.ebiom.2015.09.044
Cao, S., Pan, Y., Tang, J., Terker, A., Arroyo Ornelas, J., Jin, G., et al. (2022). EGFR-mediated activation of adipose tissue macrophages promotes obesity and insulin resistance. Nat. Commun. 13 (1), 4684. doi:10.1038/s41467-022-32348-3
Carey, A., Steinberg, G., Macaulay, S., Thomas, W., Holmes, A., Ramm, G., et al. (2006). Interleukin-6 increases insulin-stimulated glucose disposal in humans and glucose uptake and fatty acid oxidation in vitro via AMP-activated protein kinase. Diabetes 55 (10), 2688–2697. doi:10.2337/db05-1404
Caspar, B., S., Cousin, B., Bour, S., Casteilla, L., Castiella, L., Penicaud, L., et al. (2009). Adipose tissue lymphocytes: Types and roles. J. Physiology Biochem. 65 (4), 423–436. doi:10.1007/bf03185938
Chae, H. J., Kim, S. C., Chae, S. W., An, N. H., Kim, H. H., Lee, Z. H., et al. (2001). Blockade of the p38 mitogen-activated protein kinase pathway inhibits inducible nitric oxide synthase and interleukin-6 expression in MC3T3E-1 osteoblasts. Pharmacol. Res. 43 (3), 275–283. doi:10.1006/phrs.2000.0778
Chen, J., Wei, Y., Yang, W., Huang, Q., Chen, Y., Zeng, K., et al. (2022). IL-6: The link between inflammation, immunity and breast cancer. Front. Oncol. 12, 903800. doi:10.3389/fonc.2022.903800
Cho, S., Lim, S., Ahn, K., Kwak, H., Park, J., and Um, J. (2021). Farnesol induces mitochondrial/peroxisomal biogenesis and thermogenesis by enhancing the AMPK signaling pathway in vivo and in vitro. Pharmacol. Res. 163, 105312. doi:10.1016/j.phrs.2020.105312
Chou, C., Hua, K., Chen, M., Wu, C., Hsu, C., Wang, J., et al. (2022). Discovery and characterization of a monoclonal antibody targeting a conformational epitope of IL-6/IL-6Rα to inhibit IL-6/IL-6Rα/gp130 hexameric signaling complex formation. MABS 14 (1), e2029675. doi:10.1080/19420862.2022.2029675
Clementi, A., Gaudy, A., Zimmers, T., Koniaris, L., and Mooney, R. (2011). Deletion of interleukin-6 improves pyruvate tolerance without altering hepatic insulin signaling in the leptin receptor-deficient mouse. Metabolism 60 (11), 1610–1619. doi:10.1016/j.metabol.2011.04.004
Cobbina, E., and Akhlaghi, F. (2017). Non-alcoholic fatty liver disease (NAFLD)-pathogenesis, classification, and effect on drug metabolizing enzymes and transporters. Drug Metab. Rev. 49 (2), 197–211. doi:10.1080/03602532.2017.1293683
Craig, R., Larkin, A., Mingo, A., Thuerauf, D., Andrews, C., McDonough, P., et al. (2000). p38 MAPK and NF-kappa B collaborate to induce interleukin-6 gene expression and release. Evidence for a cytoprotective autocrine signaling pathway in a cardiac myocyte model system. J. Biol. Chem. 275 (31), 23814–23824. doi:10.1074/jbc.M909695199
Day, E., Ford, R., and Steinberg, G. (2017). AMPK as a therapeutic target for treating metabolic diseases. Trends Endocrinol. Metabolism 28 (8), 545–560. doi:10.1016/j.tem.2017.05.004
de Vries, A., Ruggenenti, P., Ruan, X., Praga, M., Cruzado, J., Bajema, I., et al. (2014). Fatty kidney: Emerging role of ectopic lipid in obesity-related renal disease. Lancet 2 (5), 417–426. doi:10.1016/s2213-8587(14)70065-8
Dmitrieva, O. S., Shilovskiy, I. P., Khaitov, M. R., and Grivennikov, S. I. (2016). Interleukins 1 and 6 as main mediators of inflammation and cancer. Biochemistry 81 (2), 80–90. doi:10.1134/S0006297916020024
Dolmetsch, R., Xu, K., and Lewis, R. (1998). Calcium oscillations increase the efficiency and specificity of gene expression. Nature 392 (6679), 933–936. doi:10.1038/31960
Ducharme, N. A., and Bickel, P. E. (2008). Minireview: Lipid droplets in lipogenesis and lipolysis. Endocrinology 149 (3), 942–949. doi:10.1210/en.2007-1713
Ellingsgaard, H., Hauselmann, I., Schuler, B., Habib, A., Baggio, L., Meier, D., et al. (2011). Interleukin-6 enhances insulin secretion by increasing glucagon-like peptide-1 secretion from L cells and alpha cells. Nat. Med. 17 (11), 1481–1489. doi:10.1038/nm.2513
Febbraio, M., and Pedersen, B. (2005). Contraction-induced myokine production and release: Is skeletal muscle an endocrine organ? Exerc. Sport Sci. Rev. 33 (3), 114–119. doi:10.1097/00003677-200507000-00003
Feingold, K. R., Doerrler, W., Dinarello, C. A., Fiers, W., and Grunfeld, C. (1992). Stimulation of lipolysis in cultured fat cells by tumor necrosis factor, interleukin-1, and the interferons is blocked by inhibition of prostaglandin synthesis. Endocrinology 130 (1), 10–16. doi:10.1210/endo.130.1.1370149
Fernández-Gayol, O., Sanchis, P., Aguilar, K., Navarro-Sempere, A., Comes, G., Molinero, A., et al. (2019). Different responses to a high-fat diet in IL-6 conditional knockout mice driven by constitutive GFAP-cre and synapsin 1-cre expression. Neuroendocrinology 109 (2), 113–130. doi:10.1159/000496845
Ferrer, B., Navia, B., Giralt, M., Comes, G., Carrasco, J., Molinero, A., et al. (2014). Muscle-specific interleukin-6 deletion influences body weight and body fat in a sex-dependent manner. Brain, Behav. Immun. 40, 121–130. doi:10.1016/j.bbi.2014.03.001
Fischer, P., and Fischer, C. P. (2007). Beneficial health effects of exercise - the role of IL-6 as a myokine. Trends Pharmacol. Sci. 28 (4), 152–156. doi:10.1016/j.tips.2007.02.002
Fried, S., Bunkin, D., and Greenberg, A. (1998). Omental and subcutaneous adipose tissues of obese subjects release interleukin-6: Depot difference and regulation by glucocorticoid. J. Clin. Endocrinol. Metabolism 83 (3), 847–850. doi:10.1210/jcem.83.3.4660
Fritsche, L., Hoene, M., Lehmann, R., Ellingsgaard, H., Hennige, A., Pohl, A., et al. (2010). IL-6 deficiency in mice neither impairs induction of metabolic genes in the liver nor affects blood glucose levels during fasting and moderately intense exercise. Diabetologia 53 (8), 1732–1742. doi:10.1007/s00125-010-1754-4
Frühbeck, G., Becerril, S., Sáinz, N., Garrastachu, P., and García-Velloso, M. (2009). Bat: A new target for human obesity? Trends Pharmacol. Sci. 30 (8), 387–396. doi:10.1016/j.tips.2009.05.003
Frühbeck, G., Méndez-Giménez, L., Fernández-Formoso, J., Fernández, S., and Rodríguez, A. (2014). Regulation of adipocyte lipolysis. Nutr. Res. Rev. 27 (1), 63–93. doi:10.1017/s095442241400002x
Fullerton, M., Galic, S., Marcinko, K., Sikkema, S., Pulinilkunnil, T., Chen, Z., et al. (2013). Single phosphorylation sites in Acc1 and Acc2 regulate lipid homeostasis and the insulin-sensitizing effects of metformin. Nat. Med. 19 (12), 1649–1654. doi:10.1038/nm.3372
Gallerand, A., Stunault, M., Merlin, J., Luehmann, H., Sultan, D., Firulyova, M., et al. (2021). Brown adipose tissue monocytes support tissue expansion. Nat. Commun. 12 (1), 5255. doi:10.1038/s41467-021-25616-1
Garbers, C., Jänner, N., Chalaris, A., Moss, M., Floss, D., Meyer, D., et al. (2011). Species specificity of ADAM10 and ADAM17 proteins in interleukin-6 (IL-6) trans-signaling and novel role of ADAM10 in inducible IL-6 receptor shedding. J. Biol. Chem. 286 (17), 14804–14811. doi:10.1074/jbc.M111.229393
Garretson, J., Szymanski, L., Schwartz, G., Xue, B., Ryu, V., and Bartness, T. (2016). Lipolysis sensation by white fat afferent nerves triggers Brown fat thermogenesis. Mol. Metab. 5 (8), 626–634. doi:10.1016/j.molmet.2016.06.013
Girousse, A., Gil-Ortega, M., Bourlier, V., Bergeaud, C., Sastourné-Arrey, Q., Moro, C., et al. (2019). The release of adipose stromal cells from subcutaneous adipose tissue regulates ectopic intramuscular adipocyte deposition. Cell Rep. 27 (2), 323–333.e5. doi:10.1016/j.celrep.2019.03.038
Guschin, D., Rogers, N., Briscoe, J. W., Witthuhn, B., Stark, G. R., Horn, F., et al. (1995). A major role for the protein tyrosine kinase JAK1 in the JAK/STAT signal transduction pathway in response to interleukin-6. EMBO J. 14 (7), 1421–1429. doi:10.1002/j.1460-2075.1995.tb07128.x
Haan, S., Keller, J. F., Behrmann, I., Heinrich, P. C., and Haan, C. (2005). Multiple reasons for an inefficient STAT1 response upon IL-6-type cytokine stimulation. Cell. Signal. 17 (12), 1542–1550. doi:10.1016/j.cellsig.2005.03.010
Han, M., White, A., Perry, R., Camporez, J., Hidalgo, J., Shulman, G., et al. (2020). Regulation of adipose tissue inflammation by interleukin 6. PNAS 117 (6), 2751–2760. doi:10.1073/pnas.1920004117
Hardie, D., Salt, I., Hawley, S., and Davies, S. (1999). AMP-Activated protein kinase: An ultrasensitive system for monitoring cellular energy charge. Biochem. J. 338, 717–722. doi:10.1042/bj3380717
Haslam, D. W., and James, W. P. (2005). Obesity. Lancet 366 (9492), 1197–1209. doi:10.1016/S0140-6736(05)67483-1
Hedman, A., Larsson, P., Alam, M., Wallen, N., Nordlander, R., and Samad, B. (2007). CRP, IL-6 and endothelin-1 levels in patients undergoing coronary artery bypass grafting. Do preoperative inflammatory parameters predict early graft occlusion and late cardiovascular events? Int. J. Cardiol. 120 (1), 108–114. doi:10.1016/j.ijcard.2006.09.004
Heink, S., Yogev, N., Garbers, C., Herwerth, M., Aly, L., Gasperi, C., et al. (2017). Trans-presentation of IL-6 by dendritic cells is required for the priming of pathogenic TH17 cells. Nat. Immunol. 18 (1), 74–85. doi:10.1038/ni.3632
Helvoort, H., Pol, M., Heijdra, Y. F., and Dekhuijzen, P. (2005). Systemic inflammatory response to exhaustive exercise in patients with chronic obstructive pulmonary disease. Respir. Med. 99 (12), 1555–1567. doi:10.1016/j.rmed.2005.03.028
Hojman, P., Brolin, C., Nørgaard-Christensen, N., Dethlefsen, C., Lauenborg, B., Olsen, C., et al. (2019). IL-6 release from muscles during exercise is stimulated by lactate-dependent protease activity. Am. J. Physiology-Endocrinology Metabolism 316 (5), 940–947. doi:10.1152/ajpendo.00414.2018
Hotamisligil, G., Peraldi, P., Budavari, A., Ellis, R., White, M., and Spiegelman, B. (1996). IRS-1-mediated inhibition of insulin receptor tyrosine kinase activity in TNF-alpha- and obesity-induced insulin resistance. Science 271 (5249), 665–668. doi:10.1126/science.271.5249.665
Hu, E., Kim, J., Sarraf, P., and Spiegelman, B. (1996). Inhibition of adipogenesis through MAP kinase-mediated phosphorylation of PPARgamma. Science 274 (5295), 2100–2103. doi:10.1126/science.274.5295.2100
Huang, R., Sun, Y., Ho, C., Liu, K., Tang, Q., Xie, Y., et al. (2018). IL-6 potentiates BMP-2-induced osteogenesis and adipogenesis via two different BMPR1A-mediated pathways. Cell Death Dis. 9 (2), 144. doi:10.1038/s41419-017-0126-0
Huh, J. Y. (2018). The role of exercise-induced myokines in regulating metabolism. Archives Pharmacal Res. 41 (1), 14–29. doi:10.1007/s12272-017-0994-y
Jäger, S., Handschin, C., St-Pierre, J., and Spiegelman, B. (2007). AMP-activated protein kinase (AMPK) action in skeletal muscle via direct phosphorylation of PGC-1alpha. PNAS 104 (29), 12017–12022. doi:10.1073/pnas.0705070104
Ji, L. L. (2015). Redox signaling in skeletal muscle: Role of aging and exercise. AJP Adv. Physiology Educ. 39 (4), 352–359. doi:10.1152/advan.00106.2014
Joshi-Barve, S., Barve, S., Amancherla, K., Gobejishvili, L., Hill, D., Cave, M., et al. (2007). Palmitic acid induces production of proinflammatory cytokine interleukin-8 from hepatocytes. Hepatology 46 (3), 823–830. doi:10.1002/hep.21752
Jostock, T., Müllberg, J., Ozbek, S., Atreya, R., Blinn, G., Voltz, N., et al. (2001). Soluble gp130 is the natural inhibitor of soluble interleukin-6 receptor transsignaling responses. Eur. J. Biochem. 268 (1), 160–167. doi:10.1046/j.1432-1327.2001.01867.x
Kalupahana, N., Goonapienuwala, B., and Moustaid-Moussa, N. (2020). Omega-3 fatty acids and adipose tissue: Inflammation and browning. Annu. Rev. Nutr. 40 (5), 25–49. doi:10.1146/annurev-nutr-122319-034142
Kaptein, A., Paillard, V., and Saunders, M. (1996). Dominant negative Stat3 mutant inhibits interleukin-6-induced Jak-STAT signal transduction. J. Biol. Chem. 271 (11), 5961–5964. doi:10.1074/jbc.271.11.5961
Katashima, C., de Oliveira Micheletti, T., Braga, R., Gaspar, R., Goeminne, L., Moura-Assis, A., et al. (2022). Evidence for a neuromuscular circuit involving hypothalamic interleukin-6 in the control of skeletal muscle metabolism. Sci. Adv. 8 (30), eabm7355. doi:10.1126/sciadv.abm7355
Keller, C., Steensberg, A., Pilegaard, H., Osada, T., Neufer, P. D., Pedersen, B. K., et al. (2001). Transcriptional activation of the IL-6 gene in human contracting skeletal muscle: Influence of muscle glycogen content. FASEB J. 15 (14), 2748–2750. doi:10.1096/fj.01-0507fje
Kelly, M., Keller, C., Avilucea, P., Keller, P., Luo, Z., Xiang, X., et al. (2004). AMPK activity is diminished in tissues of IL-6 knockout mice: The effect of exercise. Biochem. Biophysical Res. Commun. 320 (2), 449–454. doi:10.1016/j.bbrc.2004.05.188
Khan, K., Xu, S., Nihtyanova, S., Derrett-Smith, E., Abraham, D., Denton, C., et al. (2012). Clinical and pathological significance of interleukin 6 overexpression in systemic sclerosis. Ann. Rheumatic Dis. 71 (7), 1235–1242. doi:10.1136/annrheumdis-2011-200955
Kishimoto, T. (2010). IL-6: From its discovery to clinical applications. Int. Immunol. 22 (5), 347–352. doi:10.1093/intimm/dxq030
Kishimoto, T. (2005). Interleukin-6: From basic science to medicine--40 years in immunology. Annu. Rev. Immunol. 23, 1–21. doi:10.1146/annurev.immunol.23.021704.115806
Knudsen, J. G., Joensen, E., Bertholdt, L., Jessen, H., van Hauen, L., Hidalgo, J., et al. (2016). Skeletal muscle IL-6 and regulation of liver metabolism during high-fat diet and exercise training. Physiol. Rep. 4 (9), e12788. doi:10.14814/phy2.12788
Lamertz, L., Rummel, F., Polz, R., Baran, P., Hansen, S., Waetzig, G., et al. (2018). Soluble gp130 prevents interleukin-6 and interleukin-11 cluster signaling but not intracellular autocrine responses. Sci. Signal. 11 (550), e7388. doi:10.1126/scisignal.aar7388
Latourte, A., Cherifi, C., Maillet, J., Ea, H., Bouaziz, W., Funck-Brentano, T., et al. (2017). Systemic inhibition of IL-6/Stat3 signalling protects against experimental osteoarthritis. Ann. rheumatic Dis. 76 (4), 748–755. doi:10.1136/annrheumdis-2016-209757
Lauterbach, M., and Wunderlich, F. (2017). Macrophage function in obesity-induced inflammation and insulin resistance. Pflugers Archiv-European J. Physiology 469, 385–396. doi:10.1007/s00424-017-1955-5
Lee, H., Kang, R., Bae, S., and Yoon, Y. (2011). AICAR, an activator of AMPK, inhibits adipogenesis via the WNT/β-catenin pathway in 3T3-L1 adipocytes. Int. J. Mol. Med. 28 (1), 65–71. doi:10.3892/ijmm.2011.674
Lee, W., Kim, M., Park, H., Kim, H., Jeon, M., Oh, K., et al. (2006). AMPK activation increases fatty acid oxidation in skeletal muscle by activating PPARalpha and PGC-1. Biochem. Biophysical Res. Commun. 340 (1), 291–295. doi:10.1016/j.bbrc.2005.12.011
Li, M., Abraham, N., Vanella, L., Zhang, Y., Inaba, M., Hosaka, N., et al. (2010). Successful modulation of type 2 diabetes in db/db mice with intra-bone marrow--bone marrow transplantation plus concurrent thymic transplantation. J. Autoimmun. 35 (4), 414–423. doi:10.1016/j.jaut.2010.09.001
Liang, H., Yin, B., Zhang, H., Zhang, S., Zeng, Q., Wang, J., et al. (2008). Blockade of tumor necrosis factor (TNF) receptor type 1-mediated TNF-alpha signaling protected Wistar rats from diet-induced obesity and insulin resistance. Endocrinology 149 (6), 2943–2951. doi:10.1210/en.2007-0978
Libby, P., and Hansson, G. (2019). From focal lipid storage to systemic inflammation: JACC review topic of the week. J. Am. Coll. Cardiol. 74 (12), 1594–1607. doi:10.1016/j.jacc.2019.07.061
Lin, W., Chen, L., Meng, W., Yang, K., Wei, S., Wei, W., et al. (2022a). C/EBPα promotes porcine pre-adipocyte proliferation and differentiation via mediating MSTRG.12568.2/FOXO3 trans-activation for STYX. BBA-Molecular Cell Biol. Lipids 1867 (10), 159206. doi:10.1016/j.bbalip.2022.159206
Lin, W. M., Tang, Y. H., Zhao, Y. L., Zhao, J. D., Zhang, L. F., Wei, W., et al. (2020). MiR-144-3p targets FoxO1 to reduce its regulation of adiponectin and promote adipogenesis. Front. Genet. 11 (14), 603144. doi:10.3389/fgene.2020.603144
Lin, W., Wen, X., Li, X., Chen, L., Wei, W., Zhang, L., et al. (2022b). MiR-144 regulates adipogenesis by mediating formation of C/EBPα-FOXO1 protein complex. Biochem. Biophysical Res. Commun. 612, 126–133. doi:10.1016/j.bbrc.2022.04.093
Luo, J., Hong, Y., Lu, Y., Qiu, S., Chaganty, B., Zhang, L., et al. (2017). Acetyl-CoA carboxylase rewires cancer metabolism to allow cancer cells to survive inhibition of the Warburg effect by cetuximab. Cancer Lett. 384, 39–49. doi:10.1016/j.canlet.2016.09.020
Lust, J., Donovan, K., Kline, M., Greipp, P., Kyle, R., and Maihle, N. (1992). Isolation of an mRNA encoding a soluble form of the human interleukin-6 receptor. Cytokine 4 (2), 96–100. doi:10.1016/1043-4666(92)90043-q
Lutgens, E., Atzler, D., Döring, Y., Duchene, J., Steffens, S., and Weber, C. (2019). Immunotherapy for cardiovascular disease. Eur. Heart J. 40 (48), 3937–3946. doi:10.1093/eurheartj/ehz283
Manore, S. G., Doheny, D. L., Wong, G. L., and Lo, H. W. (2022). IL-6/JAK/STAT3 signaling in breast cancer metastasis: Biology and treatment. Front. Oncol. 12, 866014–866888. doi:10.3389/fonc.2022.866014
Martinez-Lopez, N., Athonvarangkul, D., Sahu, S., Coletto, L., Zong, H., Bastie, C., et al. (2013). Autophagy in Myf5+ progenitors regulates energy and glucose homeostasis through control of Brown fat and skeletal muscle development. EMBO Rep. 14 (9), 795–803. doi:10.1038/embor.2013.111
Martínez-Maza, O., and Berek, J. S. (1991). Interleukin 6 and cancer treatment. Vivo 5 (6), 583–588.
Marzolla, V., Feraco, A., Gorini, S., Mammi, C., Marrese, C., Mularoni, V., et al. (2020). The novel non-steroidal MR antagonist finerenone improves metabolic parameters in high-fat diet-fed mice and activates Brown adipose tissue via AMPK-ATGL pathway. FASEB J. 34 (9), 12450–12465. doi:10.1096/fj.202000164R
Mauer, J., Chaurasia, B., Goldau, J., Vogt, M., Ruud, J., Nguyen, K., et al. (2014). Signaling by IL-6 promotes alternative activation of macrophages to limit endotoxemia and obesity-associated resistance to insulin. Nat. Immunol. 15 (5), 423–430. doi:10.1038/ni.2865
McLoughlin, R., Jenkins, B., Grail, D., Williams, A., Fielding, C., Parker, C., et al. (2005). IL-6 trans-signaling via STAT3 directs T cell infiltration in acute inflammation. PNAS 102 (27), 9589–9594. doi:10.1073/pnas.0501794102
Migita, T., Ruiz, S., Fornari, A., Fiorentino, M., Priolo, C., Zadra, G., et al. (2009). Fatty acid synthase: A metabolic enzyme and candidate oncogene in prostate cancer. J. Natl. Cancer Inst. 101 (7), 519–532. doi:10.1093/jnci/djp030
Mihara, M., Hashizume, M., Yoshida, H., Suzuki, M., and Shiina, M. (2012). IL-6/IL-6 receptor system and its role in physiological and pathological conditions. Clin. Sci. 122 (4), 143–159. doi:10.1042/CS20110340
Mohamed-Ali, V., Goodrick, S., Rawesh, A., Katz, D., Miles, J., Yudkin, J., et al. (1997). Subcutaneous adipose tissue releases interleukin-6, but not tumor necrosis factor-alpha, in vivo. J. Clin. Endocrinol. Metabolism 82 (12), 4196–4200. doi:10.1210/jcem.82.12.4450
Morris, R., Kershaw, N. J., and Babon, J. J. (2018). The molecular details of cytokine signaling via the JAK/STAT pathway. Protein Sci. 27 (12), 1984–2009. doi:10.1002/pro.3519
Müllberg, J., Dittrich, E., Graeve, L., Gerhartz, C., Yasukawa, K., Taga, T., et al. (1993a). Differential shedding of the two subunits of the interleukin-6 receptor. FEBS Lett. 332 (1-2), 174–178. doi:10.1016/0014-5793(93)80507-q
Müllberg, J., Schooltink, H., Stoyan, T., Günther, M., Graeve, L., Buse, G., et al. (1993b). The soluble interleukin-6 receptor is generated by shedding. Eur. J. Immunol. 23 (2), 473–480. doi:10.1002/eji.1830230226
Müller-Newen, G., Köhne, C., Keul, R., Hemmann, U., Müller-Esterl, W., Wijdenes, J., et al. (1996). Purification and characterization of the soluble interleukin-6 receptor from human plasma and identification of an isoform generated through alternative splicing. Eur. J. Biochem. 236 (3), 837–842. doi:10.1111/j.1432-1033.1996.00837.x
Murano, I., Barbatelli, G., Parisani, V., Latini, C., Muzzonigro, G., Castellucci, M., et al. (2008). Dead adipocytes, detected as crown-like structures, are prevalent in visceral fat depots of genetically obese mice. J. Lipid Res. 49 (7), 1562–1568. doi:10.1194/jlr.M800019-JLR200
Nara, H., and Watanabe, R. (2021). Anti-inflammatory effect of muscle-derived interleukin-6 and its involvement in lipid metabolism. Int. J. Mol. Sci. 22 (18), 9889. doi:10.3390/ijms22189889
Nishimoto, N., and Kishimoto, T. (2006). Interleukin 6: From bench to bedside. Nat. Clin. Pract. Rheumatol. 2 (11), 619–626. doi:10.1038/ncprheum0338
O'Keefe, S. (2019). The association between dietary fibre deficiency and high-income lifestyle-associated diseases: Burkitt's hypothesis revisited. Lancet 4 (12), 984–996. doi:10.1016/s2468-1253(19)30257-2
Oberg, H. H., Wesch, D., Grüssel, S., Rose-John, S., Kabelitz, , and Dieter, (2006). Differential expression of CD126 and CD130 mediates different STAT-3 phosphorylation in CD4+CD25-and CD25high regulatory T cells. Int. Immunol. 18 (4), 555–563. doi:10.1093/intimm/dxh396
Ogasawara, J., Kitadate, K., Nishioka, H., Fujii, H., Sakurai, T., Kizaki, T., et al. (2010). Oligonol, an oligomerized lychee fruit-derived polyphenol, activates the Ras/Raf-1/MEK1/2 cascade independent of the IL-6 signaling pathway in rat primary adipocytes. Biochem. Biophysical Res. Commun. 402 (3), 554–559. doi:10.1016/j.bbrc.2010.10.082
Oh, J., Revel, M., and Chebath, J. (1996). A soluble interleukin 6 receptor isolated from conditioned medium of human breast cancer cells is encoded by a differentially spliced mRNA. Cytokine 8 (5), 401–409. doi:10.1006/cyto.1996.0055
Olson, E., and Williams, R. (2000). Calcineurin signaling and muscle remodeling. Cell 101 (7), 689–692. doi:10.1016/s0092-8674(00)80880-6
Pacifici, F., Farias, C., Rea, S., Capuani, B., Feraco, A., Coppola, A., et al. (2020). Tyrosol may prevent obesity by Inhibiting adipogenesis in 3T3-L1 preadipocytes. Oxidative Med. Cell. Longev. 2020, 4794780. doi:10.1155/2020/4794780
Pedersen, Bente, Klarlund, Hoffman-Goetz, and Laurie, (2000). Exercise and the immune system: Regulation, integration, and adaptation. Physiol. Rev. 80 (3), 1055–1081. doi:10.1152/physrev.2000.80.3.1055
Pedersen, B., and Febbraio, M. (2008). Muscle as an endocrine organ: Focus on muscle-derived interleukin-6. Physiol. Rev. 88 (4), 1379–1406. doi:10.1152/physrev.90100.2007
Pedersen, B., and Febbraio, M. (2012). Muscles, exercise and obesity: Skeletal muscle as a secretory organ. Nat. Rev. 8 (8), 457–465. doi:10.1038/nrendo.2012.49
Pedersen, B., and Fischer, C. (2007). Physiological roles of muscle-derived interleukin-6 in response to exercise. Curr. Opin. Clin. Nutr. Metabolic Care 10 (3), 265–271. doi:10.1097/MCO.0b013e3280ebb5b3
Pedersen, B. K., Steensberg, A., Keller, P., Keller, C., Fischer, C., Hiscock, N., et al. (2003). Muscle-derived interleukin-6: Lipolytic, anti-inflammatory and immune regulatory effects. Pflugers Arch. 446 (1), 9–16. doi:10.1007/s00424-002-0981-z
Pellegrinelli, V., Carobbio, S., and Vidal-Puig, A. (2016). Adipose tissue plasticity: How fat depots respond differently to pathophysiological cues. Diabetologia 59 (6), 1075–1088. doi:10.1007/s00125-016-3933-4
Peraldi, P., Hotamisligil, G., Buurman, W., White, M., and Spiegelman, B. (1996). Tumor necrosis factor (TNF)-alpha inhibits insulin signaling through stimulation of the p55 TNF receptor and activation of sphingomyelinase. J. Biol. Chem. 271 (22), 13018–13022. doi:10.1074/jbc.271.22.13018
Pierantonelli, I., and Svegliati-Baroni, G. (2019). Nonalcoholic fatty liver disease: Basic pathogenetic mechanisms in the progression from NAFLD to NASH. Transplantation 103 (1), e1–e13. doi:10.1097/tp.0000000000002480
Ramadori, G., and Armbrust, T. (2001). Cytokines in the liver. Eur. J. Gastroenterology Hepatology 13 (7), 777–784. doi:10.1097/00042737-200107000-00004
Ramirez Reyes, J., Cuesta, R., and Pause, A. (2021). Folliculin: A regulator of transcription through AMPK and mTOR signaling pathways. Front. Cell Dev. Biol. 9, 667311. doi:10.3389/fcell.2021.667311
Ridker, P. (2019). Anticytokine agents: Targeting interleukin signaling pathways for the treatment of atherothrombosis. Circulation Res. 124 (3), 437–450. doi:10.1161/circresaha.118.313129
Ridker, P., Everett, B., Thuren, T., MacFadyen, J., Chang, W., Ballantyne, C., et al. (2017). Antiinflammatory therapy with canakinumab for atherosclerotic disease. N. Engl. J. Med. 377 (12), 1119–1131. doi:10.1056/NEJMoa1707914
Ridker, P. (2016). From C-reactive protein to interleukin-6 to interleukin-1: Moving upstream to identify novel targets for atheroprotection. Circulation Res. 118 (1), 145–156. doi:10.1161/circresaha.115.306656
Ridker, P., Libby, P., MacFadyen, J., Thuren, T., Ballantyne, C., Fonseca, F., et al. (2018). Modulation of the interleukin-6 signalling pathway and incidence rates of atherosclerotic events and all-cause mortality: Analyses from the canakinumab anti-inflammatory thrombosis outcomes study (CANTOS). Eur. Heart J. 39 (38), 3499–3507. doi:10.1093/eurheartj/ehy310
Ritschel, V., Seljeflot, I., Arnesen, H., Halvorsen, S., Eritsland, J., Fagerland, M., et al. (2016). Circulating levels of IL-6 receptor and gp130 and long-term clinical outcomes in ST-elevation myocardial infarction. J. Am. Heart Assoc. 5 (6), e003014. doi:10.1161/jaha.115.003014
Rosanna, P. (2019). Exercise-induced myokines with therapeutic potential for muscle wasting. Front. Physiology 10, 287. doi:10.3389/fphys.2019.00287
Rose-John, S., Scheller, J., Elson, G., and Jones, S. A. (2006). Interleukin-6 biology is coordinated by membrane-bound and soluble receptors: Role in inflammation and cancer. J. Leukoc. Biol. 80 (2), 227–236. doi:10.1189/jlb.1105674
Rui, L., Yuan, M., Frantz, D., Shoelson, S., and White, M. (2002). SOCS-1 and SOCS-3 block insulin signaling by ubiquitin-mediated degradation of IRS1 and IRS2. J. Biol. Chem. 277 (44), 42394–42398. doi:10.1074/jbc.C200444200
Sachithanandan, N., Fam, B., Fynch, S., Dzamko, N., Watt, M., Wormald, S., et al. (2010). Liver-specific suppressor of cytokine signaling-3 deletion in mice enhances hepatic insulin sensitivity and lipogenesis resulting in fatty liver and obesity. Hepatology 52 (5), 1632–1642. doi:10.1002/hep.23861
Saha, A., and Ruderman, N. (2003). Malonyl-CoA and AMP-activated protein kinase: An expanding partnership. Mol. Cell. Biochem. 253, 65–70. doi:10.1023/a:1026053302036
Saini, A., Faulkner, S., Moir, H., Warwick, P., King, J., and Nimmo, M. (2014). Interleukin-6 in combination with the interleukin-6 receptor stimulates glucose uptake in resting human skeletal muscle independently of insulin action. Diabetes, Obes. Metabolism 16 (10), 931–936. doi:10.1111/dom.12299
Samuel, V., and Shulman, G. (2012). Mechanisms for insulin resistance: Common threads and missing links. Cell 148 (5), 852–871. doi:10.1016/j.cell.2012.02.017
Sanyal, A., Brunt, E., Kleiner, D., Kowdley, K., Chalasani, N., Lavine, J., et al. (2011). Endpoints and clinical trial design for nonalcoholic steatohepatitis. Hepatology 54 (1), 344–353. doi:10.1002/hep.24376
Schaper, F., and Rose-John, S. (2015). Interleukin-6: Biology, signaling and strategies of blockade. Cytokine & Growth Factor Rev. 26 (5), 475–487. doi:10.1016/j.cytogfr.2015.07.004
Scheele, C., and Wolfrum, C. (2020). Brown adipose crosstalk in tissue plasticity and human metabolism. Endocr. Rev. 41 (1), 53–65. doi:10.1210/endrev/bnz007
Scheja, L., and Heeren, J. (2016). Metabolic interplay between white, beige, Brown adipocytes and the liver. J. Hepatology 64 (5), 1176–1186. doi:10.1016/j.jhep.2016.01.025
Scheller, J., Chalaris, A., Schmidt-Arras, D., and Rose-John, S. (2011). The pro- and anti-inflammatory properties of the cytokine interleukin-6. Biochim. Biophys. Acta 1813 (5), 878–888. doi:10.1016/j.bbamcr.2011.01.034
Schmidt-Arras, Dirk, Rose-John, , and Stefan, (2016). IL-6 pathway in the liver: From physiopathology to therapy. J. Hepatology 64 (6), 1403–1415. doi:10.1016/j.jhep.2016.02.004
Schöbitz, B., Pezeshki, G., Pohl, T., Hemmann, U., Heinrich, P., Holsboer, F., et al. (1995). Soluble interleukin-6 (IL-6) receptor augments central effects of IL-6 in vivo. FASEB J. 9 (8), 659–664. doi:10.1096/fasebj.9.8.7768358
Schumacher, N., Meyer, D., Mauermann, A., von der Heyde, J., Wolf, J., Schwarz, J., et al. (2015). Shedding of endogenous interleukin-6 receptor (IL-6R) is governed by a disintegrin and metalloproteinase (ADAM) proteases while a full-length IL-6R isoform localizes to circulating microvesicles. J. Biol. Chem. 290 (43), 26059–26071. doi:10.1074/jbc.M115.649509
Schumacher, N., and Rose-John, S. (2022). ADAM17 orchestrates Interleukin-6, TNFα and EGF-R signaling in inflammation and cancer. BBA-Molecular Cell Res. 1869 (1), 119141. doi:10.1016/j.bbamcr.2021.119141
Seale, P., Bjork, B., Yang, W., Kajimura, S., Chin, S., Kuang, S., et al. (2008). PRDM16 controls a Brown fat/skeletal muscle switch. Nature 454 (7207), 961–967. doi:10.1038/nature07182
Severinsen, M., and Pedersen, B. (2020). Muscle-organ crosstalk: The emerging roles of myokines. Endocr. Rev. 41 (4), 594–609. doi:10.1210/endrev/bnaa016
Sharif, K., Watad, A., Bragazzi, N. L., Lichtbroun, M., and Shoenfeld, Y. (2017). Physical activity and autoimmune diseases: Get moving and manage the disease. Autoimmun. Rev. 17 (1), 53–72. doi:10.1016/j.autrev.2017.11.010
Shuai, K., Ziemiecki, A., Wilks, A. F., Harpur, A. G., Sadowski, H. B., Gilman, M. Z., et al. (1993). Polypeptide signalling to the nucleus through tyrosine phosphorylation of Jak and Stat proteins. Nature 366 (6455), 580–583. doi:10.1038/366580a0
Skuratovskaia, D., Komar, A., Vulf, M., Quang, H., Shunkin, E., Volkova, L., et al. (2021). IL-6 reduces mitochondrial replication, and IL-6 receptors reduce chronic inflammation in NAFLD and type 2 diabetes. Int. J. Mol. Sci. 22 (4), 1774. doi:10.3390/ijms22041774
Stanford, K., Middelbeek, R., Townsend, K., An, D., Nygaard, E., Hitchcox, K., et al. (2013). Brown adipose tissue regulates glucose homeostasis and insulin sensitivity. J. Clin. Investigation 123 (1), 215–223. doi:10.1172/jci62308
Steensberg, A., Febbraio, M., Osada, T., Schjerling, P., van Hall, G., Saltin, B., et al. (2001). Interleukin-6 production in contracting human skeletal muscle is influenced by pre-exercise muscle glycogen content. J. Physiology 537, 633–639. doi:10.1111/j.1469-7793.2001.00633.x
Steensberg, A., Keller, C., Starkie, R. L., Osada, T., Febbraio, M. A., and Pedersen, B. K. (2002). IL-6 and TNF-alpha expression in, and release from, contracting human skeletal muscle. Am. J. Physiology. Endocrinol. Metabolism 283 (6), E1272–E1278. doi:10.1152/ajpendo.00255.2002
Szendroedi, J., and Roden, M. (2009). Ectopic lipids and organ function. Curr. Opin. Lipidol. 20 (1), 50–56. doi:10.1097/mol.0b013e328321b3a8
Taga, T., Hibi, M., Hirata, Y., Yamasaki, K., Yasukawa, K., Matsuda, T., et al. (1989). Interleukin-6 triggers the association of its receptor with a possible signal transducer, gp130. Cell 58 (3), 573–581. doi:10.1016/0092-8674(89)90438-8
Tardif, J., Kouz, S., Waters, D., Bertrand, O., Diaz, R., Maggioni, A., et al. (2019). Efficacy and safety of low-dose colchicine after myocardial infarction. N. Engl. J. Med. 381 (26), 2497–2505. doi:10.1056/NEJMoa1912388
Terada, S., and Tabata, I. (2004). Effects of acute bouts of running and swimming exercise on PGC-1alpha protein expression in rat epitrochlearis and soleus muscle. Am. J. Physiology-Endocrinology Metabolism 286 (2), E208–E216. doi:10.1152/ajpendo.00051.2003
Tessaro, F., Ayala, T., Nolasco, E., Bella, L., and Martins, J. (2017). Insulin influences LPS-induced TNF-α and IL-6 release through distinct pathways in mouse macrophages from different compartments. Cell. Physiology Biochem. 42 (5), 2093–2104. doi:10.1159/000479904
Toniatti, C., Cabibbo, A., Sporena, E., Salvati, A., Cerretani, M., Serafini, S., et al. (1996). Engineering human interleukin-6 to obtain variants with strongly enhanced bioactivity. EMBO J. 15 (11), 2726–2737. doi:10.1002/j.1460-2075.1996.tb00633.x
Tuyt, L., Dokter, W., Birkenkamp, K., Koopmans, S., Lummen, C., Kruijer, W., et al. (1999). Extracellular-regulated kinase 1/2, Jun N-terminal kinase, and c-Jun are involved in NF-kappa B-dependent IL-6 expression in human monocytes. J. Immunol. 162 (8), 4893–4902. doi:10.4049/jimmunol.162.8.4893
van Hall, G., Steensberg, A., Sacchetti, M., Fischer, C., Keller, C., Schjerling, P., et al. (2003). Interleukin-6 stimulates lipolysis and fat oxidation in humans. J. Clin. Endocrinol. Metabolism 88 (7), 3005–3010. doi:10.1210/jc.2002-021687
Vo, T., Hsu, C., Wee, Y., Chen, Y., Cheng, H., Wu, C., et al. (2021). Carbon monoxide-releasing molecule-2 ameliorates particulate matter-induced aorta inflammation via toll-like receptor/NADPH oxidase/ROS/NF-B/IL-6 inhibition. Oxidative Med. Cell. Longev. 2021, 2855042. doi:10.1155/2021/2855042
Wiejak, J., Dunlop, J., Gao, S., Borland, G., and Yarwood, S. (2012). Extracellular signal-regulated kinase mitogen-activated protein kinase-dependent SOCS-3 gene induction requires c-Jun, signal transducer and activator of transcription 3, and specificity protein 3 transcription factors. Mol. Pharmacol. 81 (5), 657–668. doi:10.1124/mol.111.076976
Woodworth-Hobbs, M., Hudson, M., Rahnert, J., Zheng, B., Franch, H., and Price, S. (2014). Docosahexaenoic acid prevents palmitate-induced activation of proteolytic systems in C2C12 myotubes. J. Nutr. Biochem. 25 (8), 868–874. doi:10.1016/j.jnutbio.2014.03.017
Wueest, S., and Konrad, D. (2018). The role of adipocyte-specific IL-6-type cytokine signaling in FFA and leptin release. Adipocyte 7 (3), 226–228. doi:10.1080/21623945.2018.1493901
Yamagishi, S., Yonekura, H., Yamamoto, Y., Katsuno, K., Sato, F., Mita, I., et al. (1997). Advanced glycation end products-driven angiogenesis in vitro. Induction of the growth and tube formation of human microvascular endothelial cells through autocrine vascular endothelial growth factor. J. Biol. Chem. 272 (13), 8723–8730. doi:10.1074/jbc.272.13.8723
Yokota, K., Sato, K., Miyazaki, T., Aizaki, Y., Tanaka, S., Sekikawa, M., et al. (2021). Characterization and function of tumor necrosis factor and interleukin-6-induced osteoclasts in rheumatoid arthritis. Arthritis & Rheumatology 73 (7), 1145–1154. doi:10.1002/art.41666
Younossi, Z., Stepanova, M., Younossi, Y., Golabi, P., Mishra, A., Rafiq, N., et al. (2020). Epidemiology of chronic liver diseases in the USA in the past three decades. Gut 69 (3), 564–568. doi:10.1136/gutjnl-2019-318813
Keywords: skeletal muscle, adipose tisse, myokine, IL-6, lipids metabolism
Citation: Lin W, Song H, Shen J, Wang J, Yang Y, Yang Y, Cao J, Xue L, Zhao F, Xiao T and Lin R (2023) Functional role of skeletal muscle-derived interleukin-6 and its effects on lipid metabolism. Front. Physiol. 14:1110926. doi: 10.3389/fphys.2023.1110926
Received: 29 November 2022; Accepted: 06 July 2023;
Published: 24 July 2023.
Edited by:
Dmitri Samovski, Washington University in St. Louis, United StatesReviewed by:
Zhen Y. Jiang, Boston University, United StatesLiang Guo, Shanghai University of Sport, China
Copyright © 2023 Lin, Song, Shen, Wang, Yang, Yang, Cao, Xue, Zhao, Xiao and Lin. This is an open-access article distributed under the terms of the Creative Commons Attribution License (CC BY). The use, distribution or reproduction in other forums is permitted, provided the original author(s) and the copyright owner(s) are credited and that the original publication in this journal is cited, in accordance with accepted academic practice. No use, distribution or reproduction is permitted which does not comply with these terms.
*Correspondence: Weimin Lin, d2VpbWlubGluQGZhZnUuZWR1LmNu; Ruiyi Lin, bGlucnVpeWlAZmFmdS5lZHUuY24=
†These authors have contributed equally to this work