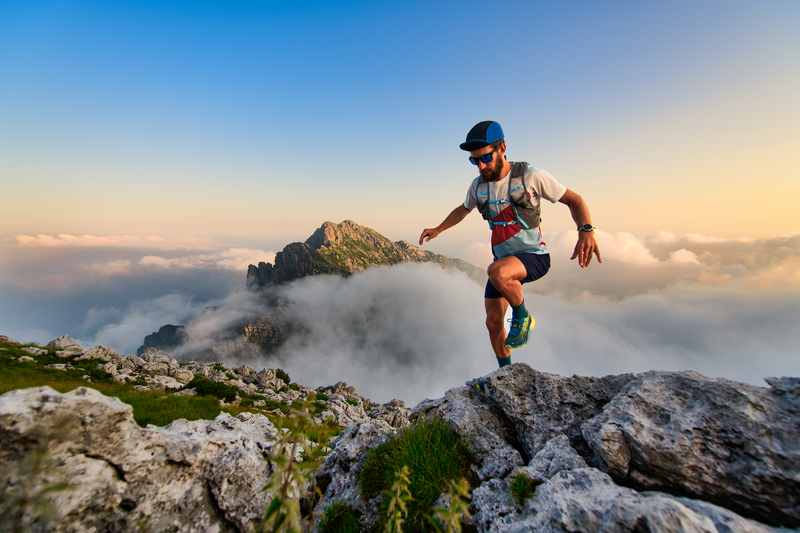
95% of researchers rate our articles as excellent or good
Learn more about the work of our research integrity team to safeguard the quality of each article we publish.
Find out more
REVIEW article
Front. Physiol. , 28 April 2023
Sec. Exercise Physiology
Volume 14 - 2023 | https://doi.org/10.3389/fphys.2023.1105772
This article is part of the Research Topic Efficacy of Small Muscle Mass Exercise Training to Promote Health View all 6 articles
Exercise with reduced muscle mass facilitates greater muscle-specific adaptations than training with larger muscle mass. The smaller active muscle mass can demand a greater portion of cardiac output which allows muscle(s) to perform greater work and subsequently elicit robust physiological adaptations that improve health and fitness. One reduced active muscle mass exercise that can promote greater positive physiological adaptations is single-leg cycling (SLC). Specifically, SLC confines the cycling exercise to a smaller muscle mass resulting in greater limb specific blood flow (i.e., blood flow is no longer “shared” by both legs) which allows the individual to exercise at a greater limb specific intensity or for a longer duration. Numerous reports describing the use of SLC have established cardiovascular and/or metabolic benefits of this exercise modality for healthy adults, athletes, and individuals living with chronic diseases. SLC has served as a valuable research tool for understanding central and peripheral factors to phenomena such as oxygen uptake and exercise tolerance (i.e., V̇O2peak and V̇O2 slow component). Together, these examples highlight the breadth of applications of SLC to promote, maintain, and study health. Accordingly, the purpose of this review was to describe: 1) acute physiological responses to SLC, 2) long-term adaptations to SLC in populations ranging from endurance athletes to middle aged adults, to individuals living with chronic disease (COPD, heart failure, organ transplant), and 3) various methods utilized to safely perform SLC. A discussion is also included on clinical application and exercise prescription of SLC for the maintenance and/or improvement of health.
Regular exercise is important for maintaining health across the lifespan, preventing and treating chronic disease, and enhancing athletic performance. The capacity to perform exercise, or exercise tolerance, can be attributed to central or peripheral factors within the integrated O2 transport system including but not limited to ventilation, alveolar-to-capillary oxygen diffusion, cardiac output, blood volume, peripheral vascular function, capillary density, oxidative enzyme concentrations, and muscle fiber type. The specific limiting factor(s) to exercise performance and peak oxygen consumption (V̇O2peak) can vary based on the health of the individual. While there is some debate regarding whether there is a central or peripheral limitation in healthy individuals (Bassett and Howley, 2000; Joyner and Dominelli, 2021), chronic obstructive pulmonary disease (COPD) and heart failure have historically been associated with central limitations and the individuals’ inability to deliver oxygenated blood to the working muscles (Martin et al., 1989; Richardson et al., 2004; Lee et al., 2016). Use of small muscle mass exercise (Richardson et al., 2004; Esposito et al., 2011; Lee et al., 2016) has facilitated separation of the influence of central cardiovascular and pulmonary dysfunction from peripheral muscle dysfunction. For example, isolated knee extension exercise completed in an aerobic fashion (e.g., 60 kicks/min for 15 min) is a small muscle mass exercise that has helped uncover the severe muscle dysfunction associated with COPD (Richardson et al., 2004) and heart failure (Esposito et al., 2011; Haykowsky et al., 2015). These findings should prompt reconsideration of rehabilitation strategies with these populations.
Another reduced muscle mass exercise is single-leg cycling (Figure 1). Specifically, single-leg cycling confines the exercise to a smaller muscle mass than traditional double-leg cycling resulting in greater limb specific blood flow (i.e., blood flow is no longer “shared” by both legs) (Burns et al., 2014b). This allows exercise at much greater limb specific intensity or for a longer duration at a similar limb specific intensity. The use of single-leg cycling stems from a report by Duner (1959) in which the author demonstrated that the working capacity of a single-leg was 73%–80% of both legs together and V̇O2peak during single-leg cycling was approximately 91% of double-leg cycling. However, the use of single-leg cycling as a research tool has been limited, likely due to the fact that single-leg cycling is less tolerable and can be more difficult to coordinate (i.e., requires greater hip flexion to lift the leg) without cycle ergometer modifications that facilitate neuromuscular patterns similar to double-leg cycling (Burns et al., 2014a; Bini et al., 2015; Elmer et al., 2016). More recently, acute single-leg cycling has been used to study the differences in muscle perfusion (Burns et al., 2014b; LaScola et al., 2020), oxygen consumption (Draper et al., 2019; 2022), substrate utilization (Draper et al., 2019; Skattebo et al., 2022), and work capacity (McPhee et al., 2009; Gordon et al., 2018) between smaller muscle mass (single-leg cycling) and larger muscle mass exercise (double-leg cycling). Single-leg cycling has also been employed as an exercise training modality to maximize peripheral adaptations in both healthy and clinical populations (Dolmage and Goldstein, 2008; Bjørgen et al., 2009; Abbiss et al., 2011; Evans et al., 2015; Munch et al., 2018). In this review, the acute physiological responses and long-term adaptations to single-leg cycling are discussed for populations ranging from trained endurance athletes to individuals living with chronic disease. Various cycle ergometer modifications that could be used to safely perform single-leg cycling as well as the clinical application and exercise prescription of single-leg cycling for the maintenance and/or improvement of health are also presented in the current review.
FIGURE 1. Overview of the physiological characteristics of single-leg cycling. The reduction in active muscle mass compared to double-leg cycling results in decreased central demands. This can in turn be utilized to facilitate comparatively greater limb specific work rates during single-leg cycling if central output is matched. Figure created in BioRender.com, with permission.
To assess the evidence supporting or refuting single-leg cycling as an efficacious exercise training and rehabilitation modality for both healthy and clinical populations, an electronic literature search was performed using the following keywords: single-leg cycling, one-leg cycling, single-limb cycling, and one-legged cycling as well as additional keywords from articles that were identified. Specifically, a search was completed for original articles published in peer-reviewed journals indexed on PubMed and Google Scholar between 1905-present. This search identified more than 100 articles. Many of these articles used single-leg cycling as a research intervention, but not directed towards understanding the acute and chronic adaptation to single-leg cycling as an exercise training modality. As a result, 16 and 11 articles were found that focused on the acute and chronic responses to single-leg cycle training, respectively. Thus, this review focuses on these 27 articles.
Several investigators have used single-leg cycling to investigate and partition the central and peripheral physiological responses and limitations to exercise in aerobically trained and untrained healthy populations (McPhee et al., 2009; Burns et al., 2014b; MacInnis et al., 2017a; Gordon et al., 2018; 2020; LaScola et al., 2020) (Figure 1). When total work rate is matched between single- and double-leg cycling (i.e., 80 W) blood flow to the active leg during single-leg cycling has been reported to be 30%–90% greater than blood flow to the same leg during double-leg cycling (Burns et al., 2014b; LaScola et al., 2020). This is the case despite similar heart rate and blood pressure responses. At higher work rates (i.e., 120 W) single-leg cycling seems to elicit greater heart rate and blood pressure responses (Burns et al., 2014b; Skattebo et al., 2022) likely due to a greater limb specific metabolic demand and afferent feedback from the active muscle resulting in an increased sympathetic response.
The additional blood flow contribution to the active limb during single-leg cycling allows the individual to perform at a greater limb specific work rate (>50% of double-leg cycling) (Abbiss et al., 2011; Gordon et al., 2018; Skattebo et al., 2022). For example, power produced during 20 × 30 s intervals at a prescribed rating of perceived exertion of 15–17 with 60 s of recovery resulted in limb specific work rates of 99 ± 34 W during double-leg cycling and 134 ± 49 W during single-leg cycling (Gordon et al., 2019). Furthermore, single-leg cycling allowed for a 21% greater limb specific work rate (176 ± 52 W) during sequential 1 min intervals compared to double-leg cycling (145 ± 38 W) (Gordon et al., 2018). This increase in limb specific work rate was accomplished with reduced cardiovascular demand (26% lower exercising V̇O2 and 5% lower heart rate) (Gordon et al., 2018). Despite lower whole body oxygen demand during single-leg cycling, limb specific oxygen consumption is greater. McPhee and others (McPhee et al., 2009) reported that 76% (range of 58%–96%) of the V̇O2peak achieved during double-leg cycling was consumed during single-leg cycling. This large range was attributed to variation in quadriceps muscle volume across individuals in that individuals with larger quadriceps volumes were able to achieve a greater percentage of the double-leg cycling V̇O2peak during single-leg cycling. The greater limb specific intensity and limb specific V̇O2 reported by McPhee (McPhee et al., 2009) agrees with other reports (MacInnis et al., 2017a; Gordon et al., 2019; Skattebo et al., 2022). However, Gordon and others (Gordon et al., 2020) reported slightly greater cardiac output and oxygen consumption relative to active muscle mass during single-leg cycling compared to double-leg cycling. In this investigation, however, limb specific work rate during single-leg cycling did not exceed that achieved during double-leg cycling. This could mean greater familiarization periods for single-leg cycling may be necessary in this population or reflect the beginning of the decrease in local oxygen delivery and distribution capacity as commonly reported in the aging population (Dinenno et al., 1999; Black et al., 2009).
Manipulating limb specific intensity during single-leg cycling can elevate either glucose or fat oxidation compared double-leg cycling (Draper et al., 2019; Skattebo et al., 2022). When total work rate is equal between single- and double-leg cycling, the work rate for the active limb during single-leg cycling is twice that during double-leg cycling. This increased work rate results in greater respiratory exchange ratio and rate of glucose oxidation (Burns et al., 2014b; MacInnis et al., 2017a; Draper et al., 2019). While cycling at an intensity of 45% V̇O2peak, the increased carbohydrate oxidation during single-leg cycling compared to double-leg cycling (1.46 ± 0.45 vs. 1.01 ± 0.49 g/min) is associated with a reduction in fat oxidation (0.22 ± 0.06 vs. 0.36 ± 0.09 g/min) (Draper et al., 2019). This increase in glucose oxidation may acutely reduce blood glucose in those with diabetes and when performed over multiple sessions is likely responsible for increased GLUT-4 expression within the muscle (Abbiss et al., 2011). In contrast, during a ramp-protocol in which limb specific work rates were matched, mass specific maximal fat oxidation was 52% greater during single-leg cycling compared to double-leg cycling due to greater blood flow for any given muscle specific work rate (Skattebo et al., 2022). Together, these reports indicate single-leg cycling can be used to maximize peripheral utilization of either glucose or fatty acids.
Single-leg cycling may also offer benefits to athletes when training at altitude (Draper et al., 2019). Typically exercise capacity is attenuated at altitude due to reduced blood oxygen content and therefore oxygen delivery to the active muscle (Klausen et al., 1966; Dill and Adams, 1971; Gavin et al., 1998; Gatterer et al., 2021). Despite elevated blood flow to the muscle, the intensity of maximal effort single-leg cycling is also reduced at altitude (Gatterer et al., 2021). However, the reduction in tissue oxygenation is greater during double-leg cycling than single-leg cycling (Draper et al., 2022). Thus, it appears that the increase in blood flow to the active muscle during single-leg cycling may partially offset the reduced blood oxygen content and allow for higher limb specific training at altitude. Consequently, single-leg cycling could be utilized for athletes at altitude to replicate limb specific work rates that would be achieved at sea level. To summarize this section, single-leg cycling has been shown to result in greater limb specific blood flow to the active limb allowing for a greater limb specific work to be completed, enhanced limb specific substrate utilization, and possibly offset oxygen related decreases in training performance at altitude.
The limited ability to ventilate the lungs and poor gas exchange within the pulmonary capillary network leads to reduced oxygen delivery to the active muscles resulting in exercise intolerance for individuals with pulmonary diseases such as COPD and pulmonary fibrosis (Dolmage and Goldstein, 2006; Dolmage et al., 2020). Greater blood flow to the reduced muscle mass during single-leg cycling should help offset the reduced blood oxygen content and improve either the duration or work rate of the exercising leg. Dolmage and Goldstein (2006) compared exercise capacity between single- and double-leg cycling in individuals with COPD (single-leg cycling work rate was set at half double-leg cycling work rate). Exercise tolerance was greater for the single-leg cycling condition as cycling with one leg allowed individuals with COPD to cycle 17 min longer and complete more total work in a single exercise session compared to double-leg cycling. In addition, during single-leg cycling participants had lower ventilation rates (-5.2 L/min), rating of dyspnea, and heart rate response (-9 bpm) at the end of exercise (Dolmage and Goldstein, 2006). A follow-up study (Dolmage et al., 2020) focused on the use of single-leg cycling in individuals with idiopathic pulmonary fibrosis during constant-load exercise and found similar improvements in endurance and work with lower V̇O2, ventilation, heart rate, and a higher tissue oxygen saturation at the end of single-leg cycling compared to double-leg cycling. These studies provide evidence that single-leg cycling allows for greater limb specific work rate and exercise tolerance in those individuals with pulmonary dysfunction.
Single-leg cycling has also been used with individuals with heart failure who have a well-established poor exercise tolerance due to reduced cardiac output, as well as poor peripheral vasculature and mitochondrial function (Bhella et al., 2011; Ives et al., 2016; Molina et al., 2016; Keir et al., 2021). LeJemtel et al. (1986) reported no difference in peak exercise leg blood flow between single and double-leg cycling in individuals with heart failure. Although this lack of increased blood flow during single-leg cycling could be due to the limited cardiac output and peripheral dilatory capacity, it is more likely due to subjects within this study achieving a greater limb specific work rate during double-leg cycling compared to single-leg cycling (LeJemtel et al., 1986). This could be due to the biomechanical constraints and hard to coordinate action of single-leg cycling on a non-modified cycle ergometer (see Considerations for single-leg cycling ergometers section for more details). Martin et al. (1989) reported no difference in maximal single- and double-leg cycling exercise responses for cardiac output, blood pressure, and a-vO2 difference in those with chronic heart failure. However, V̇O2peak during single-leg cycling (1.15 ± 0.14 L/min) was well over half of that reported for double-leg cycling (1.33 ± 0.14 L/min) indicating greater metabolic demand of the active muscle during single-leg cycling (Martin et al., 1989). These data suggest that single-leg cycling may allow individuals to tolerate greater limb specific work rates which could result in improved peripheral adaptations for individuals with heart failure. Thus, single-leg cycling has been shown to improve limb specific blood flow, improve exercise tolerance, and allow for greater limb specific work to be completed in several clinical populations.
The adaptations to continuous or interval based single-leg cycle training has been examined in healthy and aerobically trained populations (Table 1). Single-leg cycling training has resulted in 6%–13% improvements in the trained leg V̇O2peak and 8%–9% improvements in trained-leg work rate reached at the end of the incremental exercise test (Bell et al., 1988; Dela et al., 1993; Rud et al., 2012; MacInnis et al., 2017b). In addition, Rud and others (Rud et al., 2012) reported that the trained limb had a 30 ± 13% increase in citrate synthase activity, 4 ± 1% greater a-vO2 difference, and 21% ± 8% higher V̇O2 at a high-intensity work rate compared to the contralateral untrained limb. These adaptations, however, did not result in greater double-leg cycling V̇O2peak suggesting that the adaptations were limb specific and/or there are central limitations to oxygen uptake during double-leg cycling (Rud et al., 2012). Dela and co-workers (Dela et al., 1993) investigated the use of 30 min of continuous single-leg cycling exercise at 70% V̇O2peak in one leg 6 days a week for 10 weeks which resulted in a 26% increase in GLUT-4 protein concentration in the trained leg compared to the control leg.
Not only are improvements seen in the active limb following single-leg cycling, but there is some indication that these improvements are greater than what is observed following standard double-leg cycling (Abbiss et al., 2011). Abbiss et al. (2011) reported that six sessions of single-leg cycling interval training in endurance trained cyclists resulted in much greater improvements in GLUT-4 and COX II and IV protein content compared to double-leg interval training. In addition, high-intensity single-leg cycling interval training is an equally effective method for reducing cardiovascular risk in middle-aged adults compared to moderate-intensity double-leg cycling (Gordon et al., 2019). Specifically, single-leg cycling resulted in improved cardiopulmonary fitness, resting systolic blood pressure, and circulating levels of total cholesterol and low-density lipoproteins (Gordon et al., 2019). Thus, single-leg cycling training has been reported to improve single leg V̇O2peak, citrate synthase activity, a-vO2 diff, GLUT-4 concentration, COX II and IV protein content, fitness, and to decrease cardiovascular risk factors, systolic blood pressure, and blood lipids in healthy populations.
The capability to maximize oxygen delivery to the working muscle during exercise can increase the magnitude of peripheral adaptations especially in those with more severe cardio pulmonary limitations. These limitations include a reduced maximum cardiac output or ventilation, poor gas exchange at the lungs, and reduced peripheral vascular function. Single-leg cycling has been utilized as a training modality for individuals with COPD (Dolmage and Goldstein, 2008; Bjørgen et al., 2009; Evans et al., 2015), heart failure (Munch et al., 2018), and organ transplants (i.e., heart, kidney, liver) (Del Torto et al., 2021; 2022). For example, Dolmage and Goldstein (2008) investigated the effects of individuals with COPD completing 30 min of either double-leg cycling or single-leg cycling (15 min per leg) exercise 3 days per week for 7 weeks. Single-leg cycling training resulted in a 21%, 13%, and 35% improvement in V̇O2peak, ventilation, and peak work rate, respectively. These improvements were greater than those observed following double-leg cycling. In related work, Bjørgen et al. (2009) investigated single-leg cycling in those individuals with COPD and compared 8 weeks of training 3 days per week completing 4 x 4-min bouts of either double-leg cycling (4 min active: 3 min active rest) or single-leg cycling (4 min alternating active legs) (Bjørgen et al., 2009). Single-leg cycling completed by individuals with COPD resulted in greater improvements in peak work rate (23% vs. 12%) and V̇O2peak (12% vs. 6%) compared to double-leg cycling (Bjørgen et al., 2009). Finally, 6 weeks of high-intensity (8 × 4 min at 90% max work rate) single-leg cycling training completed by individuals with and without heart failure resulted in improvements in aerobic capacity and functional sympatholysis (Munch et al., 2018). An 8% improvement in 6-min walk test was also reported for individuals with heart failure that was not observed in those without heart failure (Munch et al., 2018). Collectively, these studies provide evidence that single-leg cycling has the capability to not only result in positive exercise adaptations and functional outcomes for individuals with chronic conditions but could generate greater improvements compared to traditional exercise methods such as double-leg cycling. As an additional note, a feasibility study on introducing single-leg cycling training in pulmonary rehabilitation clinics reported a high rate of training completion supporting single-leg cycling as a viable option to implement in clinical settings (Evans et al., 2015).
Most recently, single-leg cycling has been used by individuals with organ transplants. Following a transplant, severe deconditioning is observed which can largely be contributed to muscle dysfunction (Williams and McKenna, 2012) including metabolic dysfunction and muscle fiber type shifts (Kempeneers et al., 1990) as well as reductions in capillary density and endothelial dysfunction (Lampert et al., 1998; Braith et al., 2005). In addition, immunosuppressive drugs negatively affect skeletal muscle (Hokanson et al., 1995; Mercier et al., 1995). Thus, the potential for elevated limb specific work rate during single-leg cycling can potentially reverse these peripheral dysfunctions and improve overall exercise tolerance. The two reports indicated that single-leg cycling is as effective as double-leg cycling in improving V̇O2peak (Del Torto et al., 2021) and V̇O2 kinetics (Del Torto et al., 2022) following 8-week of training in individuals with heart, lung, and kidney organ transplants. However, the specific training intensities (work rate) produced by the limbs during the training sessions is unclear as double-leg cycling intensity was set by dyspnea based rating of perceived exertion while intensity for single-leg cycling was based on leg fatigue rating of perceived exertion (Del Torto et al., 2021). Thus, single-leg cycling training has been shown to improve V̇O2peak and kinetics, peripheral vascular function, functional performance such as walking, and the observed benefits may be greater than those following double-leg cycling training in various clinical populations.
Aside from utilizing single-leg cycling as a training modality to maximize peripheral adaptations, single-leg cycling has also served as an exercise option for individuals who cannot perform bilateral exercise and as a research tool to answer a variety of physiological questions. For example, individuals who have hemiplegia following a stroke or lower limb amputation may find it difficult to perform treadmill or cycling exercise, at least at the intensities required to improve cardiovascular health. The studies described above indicate that single-leg cycling can be used to achieve a cardiovascular and metabolic stress similar to double-leg cycling and therefore provides a valid exercise modality for individuals with hemiparalysis or lower limb amputation (Letombe et al., 2010; Wezenberg et al., 2012; Elmer and Martin, 2021) or during recovery from anterior cruciate ligament (ACL) injury (Olivier et al., 2008; 2010). Single-leg cycling has also been used to assess aerobic capacity between dominant and non-dominant limbs (Iannetta et al., 2019) and lower limbs with varied function due to multiple sclerosis (Larson et al., 2014) and ACL injury/surgery (Andrade et al., 2014; Hutchison et al., 2017; Bagley et al., 2020). As a research tool, single-leg cycling has been employed to partition central and peripheral contribution to exercise-induced fatigue (Elmer et al., 2013; Zhang et al., 2021), assess the impact of aerobic capacity on adaptations to resistance training (Thomas et al., 2022), evaluate recovery following eccentric muscle damage (Elmer et al., 2010; Gavin et al., 2015), investigate the influence of obesity on acute exercise response (Gries et al., 2022), and explore exercise muscle sympathetic nerve activity and the exercise pressor response (Stavres et al., 2020; Keir et al., 2021). Collectively, these examples highlight the breadth of applications of single-leg cycling to restore function, improve health, and facilitate research.
Implementation of single-leg cycling presents challenges. Most notably, the biomechanics of producing power during single-leg cycling are not the same as those during double-leg cycling. During double-leg cycling, net power is a combination of positive power produced during leg extension and negative power produced during leg flexion (Ericson, 1988; Neptune and Herzog, 1999; Elmer et al., 2011). With double-leg cycling the system is balanced in that the weight of the extending leg offsets the weight of the flexing leg requiring minimal recruitment of hip flexor muscles during the upstroke to produce a smooth cycling action. Conversely, with single-leg cycling the system is unbalanced, and the individual must actively lift the leg during the flexion phase (Elmer et al., 2016), which is unnatural and more difficult to coordinate resulting in greater perceived effort (Burns et al., 2014b) and reduced efficiency (Elmer and Martin, 2021). Thus, caution is needed when comparing physiological responses between single-and double-leg cycling with non-modified cycle ergometers (Neary and Wenger, 1986; Bell et al., 1988; Burns et al., 2014b) as the muscle recruitment is different between the two activities which could account for the differences in the reported physiological responses.
There have been several approaches to minimizing the abnormal cycling rhythm during single-leg cycling. For example, Gleser and others (Gleser, 1973) experimented with two different approaches: 1) springs attached to the active pedal to assist on the upstroke and 2) participants pedaling side-by-side with one foot of each subject secured to opposite pedals. Utilizing springs resulted in a non-linear relationship between V̇O2 and work rate while the side-by-side method maintained the linear V̇O2-workrate relationship traditionally observed with double-leg cycling and was therefore deemed to be more appropriate (Gleser, 1973). The use of a fixed gear cycle ergometer has also been utilized (Dolmage et al., 2014; Evans et al., 2015). Specifically, with this type of cycle ergometer, removal of the freewheel mechanism allows kinetic energy stored in the flywheel to help drive the single limb during hip flexion. Additionally, the use of a 10–11 kg ‘counterweight’ mounted to the unoccupied crank arm has been utilized during single-leg cycling (Burns et al., 2014b; Bini et al., 2015; Elmer et al., 2016; LaScola et al., 2020). This counterweight acts to help with the active leg during hip flexion but has no contribution to net power across an entire pedal cycle as that counterweight needs to be lifted during hip extension. Activation and work of hip and knee flexors is reduced when a counterweight is implemented, but it is still slightly greater than during double-leg cycling (Bini et al., 2015; Elmer et al., 2016). As a result, the counterweight reduces metabolic cost, cardiovascular demand, and perceived effort during single-leg cycling compared to when a counterweight is not utilized (Burns et al., 2014b; LaScola et al., 2020). Single-leg emphasis cycling facilitates cycling biomechanics of double-leg cycling without ergometer modifications. Simply encouraging participants to focus on “pushing down” with the emphasized leg during the downstroke and relax the de-emphasized leg, at least in trained speedskaters (who regularly performed cycling training), resulted in 75% of the power being produced by the emphasized leg and 25% being produced by the de-emphasized leg (Staples et al., 2020). Furthermore, the powerful extension action of the hip, knee, and ankle did not differ between single-leg emphasis cycling and double-leg cycling, but single-leg emphasis cycling required slightly more hip flexion (4%) (Staples et al., 2020). This could eliminate the need for a specialized ergometer and allow training to take place on traditional cycle ergometers and/or bicycles on the road but additional research is needed to support this. Interestingly, power-meter pedals are commercially available that can accurately quantify the work done by each leg when performing single-leg emphasis training. Finally, while assisted single-leg cycling (side-by-side, fixed gear, counterweight, emphasis) does not provide identical biomechanics to double-leg cycling (Bini et al., 2016; Elmer et al., 2016), it more closely resembles double-leg cycling than unassisted single-leg cycling.
The role of exercise intensity, duration, and volume in optimizing adaptations to rehabilitation and training has been pursued for decades. These questions also need to be answered for single-leg cycling and only a few studies have addressed these issues. As observed in Table 1 there is a range of training intensities across investigations that focus on long term adaptations to single-leg cycling in both healthy and clinical populations. The only study that directly compared how the intensity of single-leg cycling impacts the adaptations was by MacInnis and others (MacInnis et al., 2017b). In this investigation, participants performed high-intensity single-leg interval cycling in one leg with work matched moderate intensity single-leg cycling in the contralateral leg. Following only six sessions of training with each leg, citrate synthase activity and mass specific oxidative flux (phosphorylation capacities in complex I and complexes I and II) were greater in the limb that performed high-intensity interval cycling indicating a larger increase in mitochondrial volume (Larsen et al., 2012), compared to the limb that performed moderate intensity continuous cycling (MacInnis et al., 2017b). As a cautionary note, several of the authors of this review have performed extensive single-leg and single-leg-emphasis training and have, on occasion, experienced significant overtraining effects. This is likely due to the high metabolic stress that can be maintained during single-leg cycling and thus training volumes and intensities should error on the conservative side.
The impact of leg order during sequential single-leg cycling should also be considered during exercise prescription. Following single-leg cycling with the first leg, it is possible that the increased hyperemic response to single-leg cycling in the second leg could be less than the initial hyperemic response in the first leg. This seems to be the case following repeated maximal 60 s single-leg cycling efforts in which remaining vasodilation and blood pooling in the initial active limb reduces the redirection of blood flow to the subsequent active limb (Gordon et al., 2018). However, it is not clear if this would also be true following less intense bouts of single-leg cycling. Regardless, to gain maximal benefit of a single-leg cycling interval session, one should complete all intervals with the first leg before performing intervals on the second leg. Furthermore, it is recommended to alternate which leg performs the first bout of single-leg cycling between sessions.
Finally, here are some recommendations for using single-leg cycling. First, identify which single-leg cycling mode is available and if modifications to the ergometer are needed. The three most viable options are likely single-leg emphasis, fixed gear ergometer, and use of a counterweight (Figure 2). Second, familiarize the participant or patient with single-leg cycling so that the task can be performed safely and efficiently. Third, evaluate heart rate and rating of perceived exertion to ensure that the target single-leg cycling intensity is achieved. Fourth, complete all intervals with one leg first before performing intervals on the second leg. Fifth, alternate which leg performs the first bout of single-leg cycling between sessions. Finally, with any exercise intervention it is critical to monitor muscle soreness and joint pain to make sure that the exercise sessions are tolerated well over time.
FIGURE 2. The various methods for facilitating single-leg cycling. (A) Single-leg emphasis: Using a standard cycle ergometer or bicycle, the individual cycles with both legs while instructed to focus on only one leg by “pushing down” during the limb extension phase. The contralateral leg is simply “along for the ride” and its weight helps during the flexion phase of the emphasized leg. (B) Fixed gear: The cycle ergometer is adapted by removing the freewheel mechanism from the drivetrain cog and affixing it directly to the flywheel. This allows the individual to cycle with one leg while utilizing the flywheel inertia to aid during the limb flexion phase. (C) Counterweight: The cycle ergometer is adapted by attaching a specialized pedal spindle with weights (∼10 kg) mounted to the contralateral crank arm. This allows the individual to cycle with one leg while utilizing the inertia of the counterweight to aid during the limb flexion phase.
Reducing the active muscle mass during exercise by performing single-leg cycling decreases the cardiovascular demand and allows for an increased limb specific concentration of blood flow which can result in improved exercise tolerance and enhanced limb specific work rate. With chronic training, single-leg cycling can elicit cardiovascular and/or metabolic benefits in healthy adults, athletes, and individuals living with chronic diseases. Additionally, single-leg cycling serves as a viable exercise option for individuals who cannot perform bilateral exercise and as a research tool to answer a variety of physiological questions. This exercise modality is not without limitations, including the need for slight ergometer or pedaling (emphasis) modifications. Additional research is needed to better understand how to incorporate single-leg cycling into weekly exercise/training routines and determine the efficacy of single-leg cycling on performance in trained athletes and health in clinical populations.
CEH, SJE, KWW, JCM, and JM all contributed significantly to the included content and preparation of the manuscript. CEH, SJE, KWW, and JM were responsible for the first draft of the manuscript. CEH, SJE, KWW, and JM were responsible for the creation of tables and figures. CEH, SJE, KWW, JCM, and JM edited the manuscript. CEH, SJE, KWW, JCM, and JM approved the final version of the manuscript.
JCM is the inventor and patent holder on a counterweight system used with single-leg cycling.
The remaining authors declare that the research was conducted in the absence of any commercial or financial relationships that could be construed as a potential conflict of interest.
All claims expressed in this article are solely those of the authors and do not necessarily represent those of their affiliated organizations, or those of the publisher, the editors and the reviewers. Any product that may be evaluated in this article, or claim that may be made by its manufacturer, is not guaranteed or endorsed by the publisher.
Abbiss, C. R., Karagounis, L. G., Laursen, P. B., Peiffer, J. J., Martin, D. T., Hawley, J. A., et al. (2011). Single-leg cycle training is superior to double-leg cycling in improving the oxidative potential and metabolic profile of trained skeletal muscle. J. Appl. Physiol. (1985) 110, 1248–1255. doi:10.1152/japplphysiol.01247.2010
Andrade, M. S., de Lira, C. A. B., Vancini, R. L., Nakamoto, F. P., Cohen, M., and da Silva, A. C. (2014). Assessment of functional impairment after knee anterior cruciate ligament reconstruction using cardiorespiratory parameters: A cross-sectional study. BMC Musculoskelet. Disord. 15, 163. doi:10.1186/1471-2474-15-163
Bagley, M. C., Harper, S. A., McDaniel, J., and Custer, L. (2020). Single leg aerobic capacity and strength in individuals with surgically repaired anterior cruciate ligaments. Phys. Ther. Sport 46, 131–136. doi:10.1016/j.ptsp.2020.08.013
Bassett, D. R., and Howley, E. T. (2000). Limiting factors for maximum oxygen uptake and determinants of endurance performance. Med. Sci. sports Exerc. 32, 70–84. doi:10.1097/00005768-200001000-00012
Bell, G., Neary, P., and Wenger, H. A. (1988). The influence of one-legged training on cardiorespiratory fitness. J. Orthop. Sports Phys. Ther. 10, 8–11. doi:10.2519/jospt.1988.10.1.8
Bhella, P. S., Prasad, A., Heinicke, K., Hastings, J. L., Arbab-Zadeh, A., Adams-Huet, B., et al. (2011). Abnormal haemodynamic response to exercise in heart failure with preserved ejection fraction. Eur. J. heart Fail. 13, 1296–1304. doi:10.1093/eurjhf/hfr133
Bini, R. R., Jacques, T. C., Lanferdini, F. J., and Vaz, M. A. (2015). Comparison of kinetics, kinematics, and electromyography during single-leg assisted and unassisted cycling. J. Strength & Cond. Res. 29, 1534–1541. doi:10.1519/JSC.0000000000000905
Bini, R. R., Jacques, T. C., and Vaz, M. A. (2016). Joint torques and patellofemoral force during single-leg assisted and unassisted cycling. J. Sport Rehabilitation 25, 40–47. doi:10.1123/jsr.2014-0252
Bjørgen, S., Hoff, J., Husby, V. S., Høydal, M. A., Tjønna, A. E., Steinshamn, S., et al. (2009). Aerobic high intensity one and two legs interval cycling in chronic obstructive pulmonary disease: The sum of the parts is greater than the whole. Eur. J. Appl. Physiol. 106, 501–507. doi:10.1007/s00421-009-1038-1
Black, M. A., Cable, N. T., Thijssen, D. H., and Green, D. J. (2009). Impact of age, sex, and exercise on brachial artery flow-mediated dilatation. Am. J. Physiology-Heart Circulatory Physiology 297, H1109–H1116. doi:10.1152/ajpheart.00226.2009
Braith, R. W., Magyari, P. M., Pierce, G. L., Edwards, D. G., Hill, J. A., White, L. J., et al. (2005). Effect of resistance exercise on skeletal muscle myopathy in heart transplant recipients. Am. J. Cardiol. 95, 1192–1198. doi:10.1016/j.amjcard.2005.01.048
Burns, K. J., Martin, J. C., Elmer, S. J., and McDaniel, J. (2014a). Response to letter to the editor: A counterweight is not necessary to implement simple, natural and comfortable single-leg cycle training. Eur. J. Appl. physiology 114, 2457–2458. doi:10.1007/s00421-014-2965-z
Burns, K. J., Pollock, B. S., Lascola, P., and McDaniel, J. (2014b). Cardiovascular responses to counterweighted single-leg cycling: Implications for rehabilitation. Eur. J. Appl. Physiol. 114, 961–968. doi:10.1007/s00421-014-2830-0
Del Torto, A., Capelli, C., Peressutti, R., di Silvestre, A., Livi, U., Nalli, C., et al. (2021). Effect of small vs large muscle mass endurance training on maximal oxygen uptake in organ transplanted recipients. Appl. Physiol. Nutr. Metab. 46, 994–1003. doi:10.1139/apnm-2020-0987
Del Torto, A., Capelli, C., Peressutti, R., Di Silvestre, A., Livi, U., Nalli, C., et al. (2022). The effect of endurance training on pulmonary V˙O2 kinetics in solid organs transplanted recipients. Int. J. Environ. Res. Public Health 19, 9097. doi:10.3390/ijerph19159097
Dela, F., Handberg, A., Mikines, K. J., Vinten, J., and Galbo, H. (1993). GLUT 4 and insulin receptor binding and kinase activity in trained human muscle. J. Physiol. 469, 615–624. doi:10.1113/jphysiol.1993.sp019833
Dill, D., and Adams, W. C. (1971). Maximal oxygen uptake at sea level and at 3,090-m altitude in high school champion runners. J. Appl. Physiology 30, 854–859. doi:10.1152/jappl.1971.30.6.854
Dinenno, F. A., Jones, P. P., Seals, D. R., and Tanaka, H. (1999). Limb blood flow and vascular conductance are reduced with age in healthy humans: Relation to elevations in sympathetic nerve activity and declines in oxygen demand. Circulation 100, 164–170. doi:10.1161/01.cir.100.2.164
Dolmage, T. E., and Goldstein, R. S. (2006). Response to one-legged cycling in patients with COPD. Chest 129, 325–332. doi:10.1378/chest.129.2.325
Dolmage, T. E., and Goldstein, R. S. (2008). Effects of one-legged exercise training of patients with COPD. Chest 133, 370–376. doi:10.1378/chest.07-1423
Dolmage, T. E., Evans, R. A., and Goldstein, R. S. (2014). A counterweight is not necessary to implement simple, natural and comfortable single-leg cycle training. Eur. J. Appl. physiology 114, 2455–2456. doi:10.1007/s00421-014-2948-0
Dolmage, T. E., Reilly, T., Greening, N. J., Majd, S., Popat, B., Agarwal, S., et al. (2020). Cardiorespiratory responses between one-legged and two-legged cycling in patients with idiopathic pulmonary fibrosis. Ann. Am. Thorac. Soc. 17, 240–243. doi:10.1513/AnnalsATS.201907-500RL
Draper, S. N., Kearney, S. G., and McDaniel, J. (2019). Greater reliance on carbohydrates during single leg versus double leg cycling. J. Exerc. Nutr. 2, 668. doi:10.1249/01.mss.0000562499.91883.c1
Draper, S., Singer, T., Dulaney, C., and McDaniel, J. (2022). Single leg cycling offsets reduced muscle oxygenation in hypoxic environments. Int. J. Environ. Res. Public Health 19, 9139. doi:10.3390/ijerph19159139
Duner, H. (1959). Oxygen uptake and working capacity in man during work on the bicycle ergometer with one and both legs. Acta Physiol. Scand. 46, 55–61. doi:10.1111/j.1748-1716.1959.tb01736.x
Elmer, S. J., and Martin, J. C. (2021). Metabolic power and efficiency for an amputee cyclist: Implications for cycling technique. J. Appl. Physiol. (1985) 130, 479–484. doi:10.1152/japplphysiol.00661.2020
Elmer, S. J., Barratt, P. R., Korff, T., and Martin, J. C. (2011). Joint-specific power production during submaximal and maximal cycling. Med. Sci. Sports Exerc. 43 (10), 1940–1947. doi:10.1249/MSS.0b013e31821b00c5
Elmer, S. J., McDaniel, J., and Martin, J. C. (2010). Alterations in neuromuscular function and perceptual responses following acute eccentric cycling exercise. Eur. J. Appl. physiology 110, 1225–1233. doi:10.1007/s00421-010-1619-z
Elmer, S. J., Amann, M., McDaniel, J., Martin, D. T., and Martin, J. C. (2013). Fatigue is specific to working muscles: No cross-over with single-leg cycling in trained cyclists. Eur. J. Appl. Physiol. 113, 479–488. doi:10.1007/s00421-012-2455-0
Elmer, S. J., McDaniel, J., and Martin, J. C. (2016). Biomechanics of counterweighted one-legged cycling. J. Appl. biomechanics 32, 78–85. doi:10.1123/jab.2014-0209
Ericson, M. O. (1988). Mechanical muscular power output and work during ergometer cycling at different work loads and speeds. Eur. J. Appl. physiology Occup. physiology 57, 382–387. doi:10.1007/BF00417980
Esposito, F., Reese, V., Shabetai, R., Wagner, P. D., and Richardson, R. S. (2011). Isolated quadriceps training increases maximal exercise capacity in chronic heart failure: The role of skeletal muscle convective and diffusive oxygen transport. J. Am. Coll. Cardiol. 58, 1353–1362. doi:10.1016/j.jacc.2011.06.025
Evans, R. A., Dolmage, T. E., Mangovski-Alzamora, S., Romano, J., O’Brien, L., Brooks, D., et al. (2015). One-legged cycle training for chronic obstructive pulmonary disease. A pragmatic study of implementation to pulmonary rehabilitation. Ann. Am. Thorac. Soc. 12, 1490–1497. doi:10.1513/AnnalsATS.201504-231OC
Gatterer, H., Menz, V., and Burtscher, M. (2021). Acute moderate hypoxia reduces one-legged cycling performance despite compensatory increase in peak cardiac output: A pilot study. Int. J. Environ. Res. Public Health 18, 3732. doi:10.3390/ijerph18073732
Gavin, T. P., Derchak, P. A., and Stager, J. M. (1998). Ventilation’s role in the decline in VO2max and SaO2 in acute hypoxic exercise. Med. Sci. sports Exerc. 30, 195–199. doi:10.1097/00005768-199802000-00004
Gavin, J. P., Myers, S. D., and Willems, M. E. (2015). Neuromuscular responses to mild-muscle damaging eccentric exercise in a low glycogen state. J. Electromyogr. Kinesiol. 25, 53–60. doi:10.1016/j.jelekin.2014.10.005
Gleser, M. (1973). Effects of hypoxia and physical training on hemodynamic adjustments to one-legged exercise. J. Appl. Physiology 34, 655–659. doi:10.1152/jappl.1973.34.5.655
Gordon, N., Abbiss, C. R., Ihsan, M., Maiorana, A. J., and Peiffer, J. J. (2018). Active and inactive leg hemodynamics during sequential single-leg interval cycling. Med. Sci. Sports Exerc 50, 1297–1304. doi:10.1249/MSS.0000000000001553
Gordon, N., Abbiss, C. R., Maiorana, A. J., James, A. P., Clark, K., Marston, K. J., et al. (2019). High-intensity single-leg cycling improves cardiovascular disease risk factor profile. Med. Sci. Sports Exerc 51, 2234–2242. doi:10.1249/MSS.0000000000002053
Gordon, N., Abbiss, C. R., Maiorana, A. J., and Peiffer, J. J. (2020). Single-leg cycling increases limb-specific blood flow without concurrent increases in normalised power output when compared with double-leg cycling in healthy middle-aged adults. Eur. J. Sport Sci. 20, 202–210. doi:10.1080/17461391.2019.1617789
Gries, K. J., Hart, C. R., Kunz, H. E., Ryan, Z., Zhang, X., Parvizi, M., et al. (2022). Acute responsiveness to single leg cycling in adults with obesity. Physiol. Rep. 10, e15539. doi:10.14814/phy2.15539
Haykowsky, M. J., Tomczak, C. R., Scott, J. M., Paterson, D. I., and Kitzman, D. W. (2015). Determinants of exercise intolerance in patients with heart failure and reduced or preserved ejection fraction. J. Appl. physiology 119, 739–744. doi:10.1152/japplphysiol.00049.2015
Hokanson, J. F., Mercier, J. G., and Brooks, G. A. (1995). Cyclosporine A decreases rat skeletal muscle mitochondrial respiration in vitro. Am. J. Respir. Crit. care Med. 151, 1848–1851. doi:10.1164/ajrccm.151.6.7767529
Hutchison, R., Myers, J., Hayden, N., Shearer, L., Bruneau, K., and DesJardins, J. D. (2017). Evaluation of isokinetic single-leg cycling as a rehabilitation exercise following anterior cruciate ligament reconstruction surgery. J. Funct. Morphol. Kinesiol. 2, 32. doi:10.3390/jfmk2030032
Iannetta, D., Passfield, L., Qahtani, A., MacInnis, M. J., and Murias, J. M. (2019). Interlimb differences in parameters of aerobic function and local profiles of deoxygenation during double-leg and counterweighted single-leg cycling. Am. J. Physiol. Regul. Integr. Comp. Physiol. 317, R840–R851. doi:10.1152/ajpregu.00164.2019
Ives, S. J., Amann, M., Venturelli, M., Witman, M. A., Groot, H. J., Wray, D. W., et al. (2016). The mechanoreflex and hemodynamic response to passive leg movement in heart failure. Med. Sci. sports Exerc. 48, 368–376. doi:10.1249/MSS.0000000000000782
Joyner, M. J., and Dominelli, P. B. (2021). Central cardiovascular system limits to aerobic capacity. Exp. Physiol. 106, 2299–2303. doi:10.1113/EP088187
Keir, D. A., Notarius, C. F., Badrov, M. B., Millar, P. J., and Floras, J. S. (2021). Heart failure-specific inverse relationship between the muscle sympathetic response to dynamic leg exercise and V̇O2peak. Appl. Physiol. Nutr. Metab. 46, 1119–1125. doi:10.1139/apnm-2020-1074
Kempeneers, G., Noakes, T., van Zyl-Smit, R., Myburgh, K., Lambert, M., Adams, B., et al. (1990). Skeletal muscle limits the exercise tolerance of renal transplant recipients: Effects of a graded exercise training program. Am. J. Kidney Dis. 16, 57–65. doi:10.1016/s0272-6386(12)80786-4
Klausen, K., Robinson, S., Micahel, E., and Myhre, L. (1966). Effect of high altitude on maximal working capacity. J. Appl. Physiology 21, 1191–1194. doi:10.1152/jappl.1966.21.4.1191
Lampert, E., Mettauer, B., Hoppeler, H., Charloux, A., Charpentier, A., and Lonsdorfer, J. (1998). Skeletal muscle response to short endurance training in heart transplant recipients. J. Am. Coll. Cardiol. 32, 420–426. doi:10.1016/s0735-1097(98)00227-7
Larsen, S., Nielsen, J., Hansen, C. N., Nielsen, L. B., Wibrand, F., Stride, N., et al. (2012). Biomarkers of mitochondrial content in skeletal muscle of healthy young human subjects. J. physiology 590, 3349–3360. doi:10.1113/jphysiol.2012.230185
Larson, R. D., McCully, K. K., Larson, D. J., Pryor, W. M., and White, L. J. (2014). Lower-limb performance disparities: Implications for exercise prescription in multiple sclerosis. J. Rehabil. Res. Dev. 51, 1537–1544. doi:10.1682/JRRD.2013.09.0191
LaScola, P., Heidorn, C. E., Pollock, B., Burns, K., and McDaniel, J. (2020). Physiological responses to counterweighted single-leg cycling in older males. Int. J. Exerc Sci. 13, 1487–1500.
Lee, J. F., Barrett-O’Keefe, Z., Nelson, A. D., Garten, R. S., Ryan, J. J., Nativi-Nicolau, J. N., et al. (2016). Impaired skeletal muscle vasodilation during exercise in heart failure with preserved ejection fraction. Int. J. Cardiol. 211, 14–21. doi:10.1016/j.ijcard.2016.02.139
LeJemtel, T. H., Maskin, C. S., Lucido, D., and Chadwick, B. J. (1986). Failure to augment maximal limb blood flow in response to one-leg versus two-leg exercise in patients with severe heart failure. Circulation 74, 245–251. doi:10.1161/01.cir.74.2.245
Letombe, A., Cornille, C., Delahaye, H., Khaled, A., Morice, O., Tomaszewski, A., et al. (2010). Early post-stroke physical conditioning in hemiplegic patients: A preliminary study. Ann. Phys. Rehabil. Med. 53, 632–642. doi:10.1016/j.rehab.2010.09.004
MacInnis, M. J., Morris, N., Sonne, M. W., Zuniga, A. F., Keir, P. J., Potvin, J. R., et al. (2017a). Physiological responses to incremental, interval, and continuous counterweighted single-leg and double-leg cycling at the same relative intensities. Eur. J. Appl. Physiology 117, 1423–1435. doi:10.1007/s00421-017-3635-8
MacInnis, M. J., Zacharewicz, E., Martin, B. J., Haikalis, M. E., Skelly, L. E., Tarnopolsky, M. A., et al. (2017b). Superior mitochondrial adaptations in human skeletal muscle after interval compared to continuous single-leg cycling matched for total work. J. physiology 595, 2955–2968. doi:10.1113/JP272570
Martin, W. H., Berman, W. I., Buckey, J. C., Snell, P. G., and Blomqvist, C. G. (1989). Effects of active muscle mass size on cardiopulmonary responses to exercise in congestive heart failure. J. Am. Coll. Cardiol. 14, 683–694. doi:10.1016/0735-1097(89)90111-3
McPhee, J. S., Williams, A. G., Stewart, C., Baar, K., Schindler, J. P., Aldred, S., et al. (2009). The training stimulus experienced by the leg muscles during cycling in humans. Exp. Physiol. 94, 684–694. doi:10.1113/expphysiol.2008.045658
Mercier, J. G., Hokanson, J. F., and Brooks, G. A. (1995). Effects of cyclosporine A on skeletal muscle mitochondrial respiration and endurance time in rats. Am. J. Respir. Crit. care Med. 151, 1532–1536. doi:10.1164/ajrccm.151.5.7735611
Molina, A. J., Bharadwaj, M. S., Van Horn, C., Nicklas, B. J., Lyles, M. F., Eggebeen, J., et al. (2016). Skeletal muscle mitochondrial content, oxidative capacity, and Mfn2 expression are reduced in older patients with heart failure and preserved ejection fraction and are related to exercise intolerance. JACC Heart Fail. 4, 636–645. doi:10.1016/j.jchf.2016.03.011
Munch, G. W., Iepsen, U. W., Ryrsø, C. K., Rosenmeier, J. B., Pedersen, B. K., and Mortensen, S. P. (2018). Effect of 6 wk of high-intensity one-legged cycling on functional sympatholysis and ATP signaling in patients with heart failure. Am. J. Physiol. Heart Circ. Physiol. 314, H616–H626. doi:10.1152/ajpheart.00379.2017
Neary, P. J., and Wenger, H. A. (1986). The effects of one-and two-legged exercise on the lactate and ventilatory threshold. Eur. J. Appl. physiology Occup. physiology 54, 591–595. doi:10.1007/BF00943346
Neptune, R., and Herzog, W. (1999). The association between negative muscle work and pedaling rate. J. biomechanics 32, 1021–1026. doi:10.1016/s0021-9290(99)00100-1
Olivier, N., Weissland, T., Berthoin, S., Legrand, R., Prieur, F., Rogez, J., et al. (2008). [One-leg cycling aerobic training with the healthy leg in amateur soccer players after anterior cruciate ligament reconstruction]. Ann. Readapt Med. Phys. 51, 707–713. doi:10.1016/j.annrmp.2008.09.002
Olivier, N., Weissland, T., Legrand, R., Berthoin, S., Rogez, J., Thevenon, A., et al. (2010). The effect of a one-leg cycling aerobic training program during the rehabilitation period in soccer players with anterior cruciate ligament reconstruction. Clin. J. Sport Med. 20, 28–33. doi:10.1097/JSM.0b013e3181c967b8
Richardson, R. S., Leek, B. T., Gavin, T. P., Haseler, L. J., Mudaliar, S. R., Henry, R., et al. (2004). Reduced mechanical efficiency in chronic obstructive pulmonary disease but normal peak VO2 with small muscle mass exercise. Am. J. Respir. Crit. care Med. 169, 89–96. doi:10.1164/rccm.200305-627OC
Rud, B., Foss, O., Krustrup, P., Secher, N. H., and Hallén, J. (2012). One-legged endurance training: Leg blood flow and oxygen extraction during cycling exercise. Acta Physiol. (Oxf) 205, 177–185. doi:10.1111/j.1748-1716.2011.02383.x
Skattebo, Ø., Peci, D., Clauss, M., Johansen, E. I., and Jensen, J. (2022). Increased mass-specific maximal fat oxidation rate with small versus large muscle mass exercise. Med. Sci. Sports Exerc 54, 974–983. doi:10.1249/MSS.0000000000002864
Staples, T. J., Do-Duc, A., Link, J. E., and Martin, J. C. (2020). Emphasizing one leg facilitates single-leg training using standard cycling equipment. Scand. J. Med. Sci. Sports 30, 1017–1023. doi:10.1111/sms.13638
Stavres, J., Luck, J. C., Ducrocq, G. P., Cauffman, A. E., Pai, S., and Sinoway, L. I. (2020). Central and peripheral modulation of exercise pressor reflex sensitivity after nonfatiguing work. Am. J. Physiol. Regul. Integr. Comp. Physiol. 319, R575–R583. doi:10.1152/ajpregu.00127.2020
Thomas, A. C. Q., Brown, A., Hatt, A. A., Manta, K., Costa-Parke, A., Kamal, M., et al. (2022). Short-term aerobic conditioning prior to resistance training augments muscle hypertrophy and satellite cell content in healthy young men and women. FASEB J. 36, e22500. doi:10.1096/fj.202200398RR
Wezenberg, D., de Haan, A., van der Woude, L. H., and Houdijk, H. (2012). Feasibility and validity of a graded one-legged cycle exercise test to determine peak aerobic capacity in older people with a lower-limb amputation. Phys. Ther. 92, 329–338. doi:10.2522/ptj.20110125
Williams, T. J., and McKenna, M. J. (2012). Exercise limitation following transplantation. Compr. Physiol. 2, 1937–1979. doi:10.1002/cphy.c110021
Zhang, J., Iannetta, D., Alzeeby, M., MacInnis, M. J., and Aboodarda, S. J. (2021). Exercising muscle mass influences neuromuscular, cardiorespiratory, and perceptual responses during and following ramp-incremental cycling to task failure. Am. J. Physiology-Regulatory, Integr. Comp. Physiology 321, R238–R249. doi:10.1152/ajpregu.00286.2020
Keywords: modified exercise, one-leg cycling, blood flow, limb specific work, reduced muscle mass exercise
Citation: Heidorn CE, Elmer SJ, Wehmanen KW, Martin JC and McDaniel J (2023) Single-leg cycling to maintain and improve function in healthy and clinical populations. Front. Physiol. 14:1105772. doi: 10.3389/fphys.2023.1105772
Received: 23 November 2022; Accepted: 11 April 2023;
Published: 28 April 2023.
Edited by:
Stefano Longo, University of Milan, ItalyReviewed by:
Damien Vitiello, Université Paris Cité, FranceCopyright © 2023 Heidorn, Elmer, Wehmanen, Martin and McDaniel. This is an open-access article distributed under the terms of the Creative Commons Attribution License (CC BY). The use, distribution or reproduction in other forums is permitted, provided the original author(s) and the copyright owner(s) are credited and that the original publication in this journal is cited, in accordance with accepted academic practice. No use, distribution or reproduction is permitted which does not comply with these terms.
*Correspondence: C. Eric Heidorn, Y2hlaWRvcm5Aa2VudC5lZHU=
Disclaimer: All claims expressed in this article are solely those of the authors and do not necessarily represent those of their affiliated organizations, or those of the publisher, the editors and the reviewers. Any product that may be evaluated in this article or claim that may be made by its manufacturer is not guaranteed or endorsed by the publisher.
Research integrity at Frontiers
Learn more about the work of our research integrity team to safeguard the quality of each article we publish.