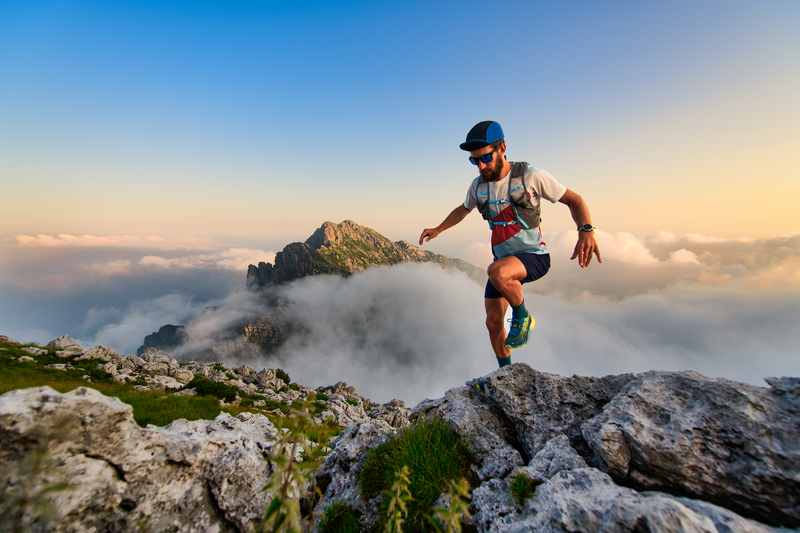
95% of researchers rate our articles as excellent or good
Learn more about the work of our research integrity team to safeguard the quality of each article we publish.
Find out more
ORIGINAL RESEARCH article
Front. Physiol. , 08 March 2023
Sec. Cell Physiology
Volume 14 - 2023 | https://doi.org/10.3389/fphys.2023.1072797
Micro- or nanoplastics, which are fragmented or otherwise tiny plastic materials, have long been a source of environmental worry. Microplastics (MPs) have been well documented to alter the physiology and behavior of marine invertebrates. The effects of some of these factors are also seen in larger marine vertebrates, such as fish. More recently, mouse models have been used to investigate the potential impacts of micro- and nanoplastics on host cellular and metabolic damages as well as mammalian gut flora. The impact on erythrocytes, which carry oxygen to all cells, has not yet been determined. Therefore, the current study aims to ascertain the impact of exposure to various MP exposure levels on hematological alterations and biochemical indicators of liver and kidney functions. In this study, a C57BL/6 murine model was concentration-dependently exposed to microplastics (6, 60, and 600 μg/day) for 15 days, followed by 15 days of recovery. The results demonstrated that exposure to 600 μg/day of MPs considerably impacted RBCs’ typical structure, resulting in numerous aberrant shapes. Furthermore, concentration-dependent reductions in hematological markers were observed. Additional biochemical testing revealed that MP exposure impacted the liver and renal functioning. Taken together, the current study reveals the severe impacts of MPs on mouse blood parameters, erythrocyte deformation, and consequently, anemic patterns of the blood.
Plastic particles with a diameter of less than 5 mm are now widely acknowledged as a threat to the environment and a health risk to human populations, ranging from oxidative stress to DNA damage (Rochman et al., 2013; Katsnelson 2015; Smith et al., 2018; Prata et al., 2020; Ibrahim et al., 2021; Blackburn and Green 2022). There are two major sources of microplastics (MPs):
1) cosmetics, detergents, sunscreens, and medicine delivery systems all containing plastic powders or particles (Galloway 2015) and
2) bigger plastic pieces breaking down in the environment due to UV radiation, mechanical abrasion, and biological deterioration (Andrady 2011).
MPs have been found in various settings and media, such as rivers, sewage, sediments, soil, and even table salt (Rillig 2012; Imhof et al., 2013; Jabeen et al., 2015; Lechner and Ramler 2015; Lusher et al., 2015).
MPs can reach human populations either directly through the environment or indirectly through food. According to numerous studies (Thompson et al., 2009; Cole et al., 2013) and food chains (Setälä et al., 2014), a variety of marine organisms (bivalves, fish, etc.) consume MPs. MPs are therefore anticipated to accumulate in the environment and increase the risk of exposure for wild creatures and human populations over time due to their extensive use and durability.
Studies demonstrating the potential health risk and tissue accumulation of MPs in mammals are scarce, although most research on the toxic effects of MPs has been on aquatic creatures. Accumulation of MPs in tissues can have various negative effects, which include physical harm. This is because the majority of these plastics have been detected in the marine animals examined, and it has been hypothesized that malnutrition contributes to their death, which is the rupture of the stomach from being trapped by debris (De Stephanis et al., 2013). Many species of birds, reptiles, and fish were found to directly ingest plastic, which may block their stomach and intestines (Hämer et al., 2014). This may cause inhibition of growth and development (Koelmans et al., 2014) and energy deficiency (Galloway 2015). Among the cellular impacts were modifications to immunological responses, the lysosomal compartment, peroxisome proliferation, antioxidant system, neurotoxic effects, and the start of genotoxicity (Avio et al., 2015; Hamed et al., 2019; Hamed et al. 2020; Hamed et al. 2021; Sayed et al., 2021; Ammar et al., 2022; Sayed et al., 2022; Sayed et al., 2022). Microplastic-induced reactive oxygen species (ROS) has been shown to be an inducer of oxidative stress in some marine organisms (Wright et al., 2013). It has been found to have severe effects on the feeding and water behavior as well as the metabolism of fish. Hence, it has been concluded that polystyrene nanoparticles have severe effects on both behavior and metabolism (Mattsson et al., 2015). Therefore, information on MP tissue accumulation in mammalian models would be crucial for determining the risk of MPs to human health (Prata et al., 2020; Ibrahim et al., 2021; Blackburn and Green 2022).
The toxic effects of MPs on erythrocytes (RBCs) are yet to be verified. Hence, the current study determined how exposure to different MP concentrations influenced haematological changes and biochemical indicators of liver and kidney function as a proxy for the effects that microplastics on human health.
This study used 60 C57BL/6 male mice purchased from the Tudor Institute in Cairo. They ranged in age from 2 months (Li et al., 2020) and were 20 g in weight. We divided the animals into four groups (15 mice in each group). At the Molecular Biology Research and Studies Institute, Assiut University, the practice was carried out in accordance with the established ethics regulations in the care and treatment of animals. The animals were housed at a temperature of 23 ± 2°C with a 12:12 light:dark cycle. Feed and drinking water were freely available to all animals.
Microplastics (MPs) were purchased from Toxemerge Pty Ltd. in Australia as powders with asymmetrical particles (>90% of the microplastics were larger than 100 nm in size). A methodology for characterizing the microplastics was performed using light and transmission electron microscopy at TEMU, Assiut University (Hamed et al., 2019).
A stock solution was prepared after the manufacturing procedure and kept at room temperature. Before each use, the stock solution (0.1 g MP/500 ml D.W.) was prepared by using a magnetic stirrer. From this stock, 30 μl representing a concentration of 6 μg, 300 µl representing 60 μg, and 3 ml representing 600 μg were taken just before the start of each experiment. MP particles were characterized using a light microscope.
Fifteen mice each were assigned to one of four groups: group 1, which is considered the control group; group 2, which got 6 μg/ml of the MPs extract each day orally; group 3, which got 60 μg/ml of MPs; and group 4, which got 600 μg/ml of MPs, for 15 days (Li et al., 2020; Ragusa et al., 2021; Huang et al., 2022; Pironti et al., 2023).
The blood was drawn, and smears were dried, fixed in 100% methanol for 10 min, and then stained with hematoxylin and eosin. Slides were chosen based on their staining quality and randomly graded to maintain anonymity. According to Al-Sabti and Metcalfe (1995), 3,000 cells (minimum of 100 cells per slide) were analyzed in each group under a ×40 objective to identify morphologically changed red blood corpuscles. The morphological changes of erythrocytes, which included acanthocytes, sickle-shaped cells, crenated cells, enlarged cells, and changes in nuclear morphology, were noted by using a VE-T2 microscope and were photographed using a 14 MP OMAX Camera (MN: A35140U3, China).
Using an automatic hematology analyzer Mindary B. 2000, hematological and biochemical parameters such as RBCs, WBCs, differential WBCs, blood platelets, hematocrit (HCT), hemoglobin (Hb), mean corpuscular volume (MCV), mean corpuscular hemoglobin (MCH), and mean corpuscular hemoglobin concentration (MCHC), as well as glucose, total protein, and lymphocyte/neutrophil rates were analyzed.
To identify MPs qualitatively and ascertain their presence in the digestive system, small, medium, and large intestinal fragments were cut and placed in a hydrogen peroxide solution (30%) before placing them in a hot water bath set to 70°C degrees for 2 hours.
The fourth group was observed to have more MPs than the second and third groups, which were also detected in these groups but in reduced amounts.
The minimum, maximum, averages, standard errors, and measured parameter ranges are considered to constitute fundamental statistics. For the raw data, the homogeneity of variance was assumed. Additionally, one-way ANOVA was used to record the pattern of differences in all treatments and the control group in the absence of interactions. The tests of Tukey and Dunnett were considered for multiple comparisons. At a significance level <0.05, the IBM-SPSS software version 21 (IBM-SPSS 2012.) and Xls sheets were considered.
The pictures taken using the light microscope at a magnification of ×40 revealed that the microplastic particles were of irregular shapes (Figure 1). When compared to the control, the treated groups had more MPs in their guts. In contrast to the exposure groups, MPs were not found following the recovery period.
FIGURE 1. Photographs of light images of microplastics (MPs) found in dissolved mouse tissues in hydrogen peroxide solution after exposure to (A) control, (B) 6 μg/ml MPs, (C) 60 μg/ml MPs, and (D) 600 μg/ml MPs.
The blood smears of mice from all groups, stained with hematoxylin and eosin, are displayed in Figure 2. The blood smear in the control group represents the erythrocytes’ typical structure. According to Figure 2A, the blood comprises rounded, biconcave, non-nucleated erythrocytes (Er) and various leucocyte types (L).
FIGURE 2. Blood smears of treatment mice groups with polyethylene microplastics: (A) control, (B) 6 μg/ml MPs, (C) 60 μg/ml MPs, and (D) 600 μg/ml MPs. Er: erythrocytes; L: leucocytes; Tr: teardrop-like cells; HE: helmet cell; Sto: stomatocytes; Sic: sickle cells; Sch: schistocytes; fol: folded cells; Bo: boat-shaped cell; Ov: ovalocytes; and Ech: echinocytes (H&E stained).
In this study, the erythrocytes from the animals treated with MPs displayed various morphological patterns of malformed cells. As shown in Figure 2, these patterns include the teardrop-like cells (Pollastro and Pillmore, 1987), helmet cells (HE), sickle cells (Sic), schistocytes (Sch), folded cells (Gregorio et al., 2009), boat-shaped cells (Bo), ovarian cells (Ov), and echinocytes (Lechner and Ramler).
Additionally, echinocytes exhibited the most specific change in red blood cell morphology that was noticed (Lechner and Ramler)—an indication of uremia—showing a significant increase to 13 in the 60 μg/ml MPs group, which spontaneously recovered after 15 days to 1.6. helmet cells (HE) that significantly increased to 7.9 in the 6 μg/ml MPs group, which also spontaneously recovered after 15 days to 0.033. Teardrop-like cells (Pollastro and Pillmore, 1987), which are considered indicators of myelofibrosis, showed a significant increase to 4.9 in the 6 μg/ml MPs group, which spontaneously recovered after 15 days to 1.5. It also showed a significant increase to 1.86 in the 60 μg/ml MPs group, which spontaneously recovered after 15 days to 0.6 (Figure 3; Table 1).
FIGURE 3. Blood smears of recovery mice groups with polyethylene microplastics: (A) control, (B) 6 μg/ml MPs, (C) 60 μg/ml MPs, and (D) 600 μg/ml MPs. ER: erythrocytes; Tr: teardrop-like cells; HE: helmet cell; Sic: sickle cells; Sch: schistocytes; fol: folded cells; Bo: boat-shaped cell; Ov: ovalocytes; Ech: echinocytes; and Tri: triangular cells (H&E stained).
TABLE 1. Effect of 15 days of exposure to microplastics (MPs) and recovery on the blood alterations of the C57BL/6 mouse model. Data are represented as means ± SE. Values with different superscript letters in the same row for each parameter are significantly different (p < 0.05). Values of recovery period with * are significantly different from the exposure period.
After 15 days of recovery under normal conditions, it was clear that with the 6 μg/ml MPs, there was a marked improvement in the teardrop and boat-shaped cells, while the schistocytes, helmet cells, ovalocytes, keratocytes, SC poikilocytes (poikilocytes are cells that have a variable appearance but are usually dense and may resemble sickle cells. They often have single or multiple angulated branches; some of which that resemble sickle cells may instead have straight edges. Classical SC poikilocytes may be quite rare, so they must be actively sought), and sickle cell types had completely disappeared. The recovery rate was 10.24%. With the 60 μg/ml MPs, there was a marked improvement in teardrop-like cells, helmet cells, ovalocytes, and echinocytes, while the boat-shaped, triangular, and folded shape cells and stomatocyte types had completely disappeared. The recovery rate was 15.63%. With the 600 μg/ml MPs, there was a marked improvement in the helmet cell types and ovalocytes, with a significant increase in the number of boat-shaped cells, teardrop-shaped cells, sickle cells, and echinocytes. The response rate of the animals to recovery appeared at the concentration of 109.6%, and this does not mean that the recovery rate here is as high as would be expected due to the continued high numbers of abnormal blood cells. This is evidence of the presence of MPs and that the animals could not eliminate the high concentration of MPs inside their bodies. Considering the preceding, we conclude that a 15-day recovery period helped mitigate the impacts of microplastics in small quantities but was insufficient to eliminate them; however, at the same time, in high concentrations, it was never sufficient (Table 2).
Moreover, a drop in the RBC diameter indicates several types of blood anemia. In this study, the diameter of the RBCs was measured in all animal groups, showing a significant decrease in the MPs-treated groups: 3.8, 3.3, and 3.4 μm for 6, 60, and 600 μg/ml MPs, respectively (Figure 4). Together, the aforementioned studies have demonstrated how altered RBC shape transitions might be impacted by lower RBC deformability.
FIGURE 4. Histogram of diameters of erythrocytes in the exposure and recovery groups of the C57BL/6 murine model.
In the treated groups, the concentration of 6 µg/ml MPs affected (p> 0.05) neutrophils and lymphocytes; the concentration of 60 µg/ml MPs significantly affected (p < 0.05) RBCs, Hbs, HTs, and monocytes; these concentrations had highly significant effects (o < 0.00001) on neutrophils, lymphocytes, and the N/L ratio. By contrast, the concentration of 600 µg/ml MPs was found to be significant on monocytes only and highly significantly on RBCs, Hbs, HTs, neutrophils, lymphocytes, and the N/L ratio (Table 3). Only the RBCs, HCT, Hbs, neutrophils, lymphocytes, monocytes, and N/L ratio remained affected by MPs as stressed by the treatment of 60 and 600 μg/ml MPs during the recovery periods. The MPs-exposed animals’ hematological parameters showed substantial fluctuations when compared to those of the control and of 6 μg/ml MPs group. Except for RBCs and HCT, which showed substantial alterations after recovery from the pollutant, there was no other significant variation in the hematological parameters. In contrast to the exposed animals, these changes may have been brought about by individual differences. In conclusion, the concentration of MPs plays a significant role in determining how harmful these are, and the 15-day recovery time improved the hematological features.
TABLE 3. Effect of 15 days of exposure to microplastics (MPs) and recovery on the hematological characteristics of the C57BL/6 mouse model. Data are represented as means ± SE. Values with different superscript letters in the same row for each parameter are significantly different (p < 0.05). Values of recovery period with * are significantly different from exposure period.
The metabolic profile of the animals exposed to MPs changed, and the liver enzyme activity, particularly elevated aspartate aminotransferase (AST) and alanine aminotransferase (ALT) (Connes, Lamarre, et al.), increased considerably with the dose. A comparable pattern of the considerable rise in serum glucose was seen. When comparing the treated animals with those of the control group, the treated animals’ creatinine levels were higher. Animals exposed to MPs displayed noticeably higher levels of total protein. Additionally, a considerable rise in the neutrophil to lymphocyte ratio (N/L), a reliable immunological indicator of inflammation, was dose-dependently seen in the MPs-treated mice (Table 4).
TABLE 4. Effect of 15 days of exposure to microplastics (MPs) and recovery on the biochemical parameters of the C57BL/6 murine model. Data are represented as means ± SE. Values with different superscript letters in the same row for each parameter are significantly different (p < 0.05). Values of recovery period with * are significantly different from exposure period.
In recent years, a significant increase in the negative impact of environmental contaminants on human health (Inhorn and Patrizio. 2015) is seen. High MP concentrations have been found in freshwater (0-1 106 items/m3) and marine (0-1 104 items/m3) waterbodies. MPs have also been seen in various animal species such as mussels and fish that humans consume (Desforges et al., 2014; Deng et al., 2017). MPs can spread through the aquatic food chain, which will likely cause a biological buildup of the substance. Microplastics (MPs), an environmental pollutant, cause toxicity in the liver, kidneys, and gastrointestinal system (Hou et al., 2021; Wang et al., 2021; Zhao et al., 2021) in animals and aquatic organisms. A recent study has shown that MPs harm the male reproductive system (Hou et al., 2021). However, little is known regarding how microplastics affect vascular biology or humans/mammals. A recent study has revealed that MPs fundamentally impact the hematological system in mice and that these alterations in gene expressions were connected (Sun et al., 2021). However, further research is needed to determine how MPs impact blood cells and other hematological variables. Red blood cell deformability has a significant impact on blood circulation at the microcirculation level. Consequently, any reduction in RBC deformability could impact flow resistance, tissue perfusion, and oxygenation (Nader et al., 2019). In this investigation, we discovered that MP particle accumulation was primarily dose dependent in the gastrointestinal system. Their particle size highly influences their distribution and the tissue accumulation kinetics, and they accumulate in the liver, kidneys, and gut (Deng et al., 2017). The cellular components in the plasma—an aqueous solution which includes organic compounds, proteins, and salts—make up the whole blood, a two-phase liquid (Baskurt and Meiselman 2003). The blood’s erythrocytes, leukocytes, and platelets make up its cellular phase. The white blood cells and platelets impact blood rheology, but under typical circumstances, erythrocytes (RBCs) have the most significant impact (Pop et al., 2002) The physical characteristics of these two phases and their proportional contributions to the total blood volume determine the rheological characteristics of the blood. Additionally, hematocrit, plasma viscosity, RBCs’ capacity to deform under flow, and RBC aggregation–disaggregation characteristics all affect blood viscosity (Baskurt and Meiselman 2003; Cokelet et al., 2007).
Teardrop-like cells (Pollastro and Pillmore, 1987), helmet cells (HE), stomatocytes (Sto), sickle cells (Sic), schistocytes (Sch), folded cells (Gregorio et al., 2009), boat-shaped cells (Bo), ovalocytes Ov), and echinocytes are only a few of the RBC alterations that have been observed in the groups exposed to MPs (Lechner and Ramler). Teardrop-like cells (Pollastro and Pillmore, 1987), which are thought to be a marker for myelofibrosis, significantly increased in the 6 μg/ml MPs and 60 μg/ml MPs groups before reducing on their own after 15 days. The echinocytes are spiculated RBCs in high numbers in the 60 μg/ml MPs blood samples and indicate widespread electrolyte depletion. The distortion of red blood cells into echinocytes is due to the bilayer membrane alterations, which is the result of a protective mechanism (Svetina 2012). On the other hand, there is another explanation mechanism as high ROS levels can easily promote lipid peroxidation because RBC membranes contain a lot of polyunsaturated fatty acids, which further damages RBCs by disrupting membrane integrity and lowering their resistance to injury (Remigante et al., 2022). It is possible to utilize the cationic surfactant benzalkonium chloride as a cell membrane surface state changer since it can integrate into the erythrocyte membrane and change the shape of the erythrocytes in saline solution (Rudenko and Saeid 2010).
A reduction in intracellular erythrocyte potassium (K+) leads to red blood cell dehydration and echinocyte formation (Glader and Sullivan 1979; Gallagher 2017). Most of these alternations in the 6 μg/ml MPs and 60 μg/ml MPs groups were recovered after 15 days, demonstrating the blood’s quick, natural mending process. The recovery time, however, was insufficient for the 600 μg/ml MPs group to return the RBCs to their characteristic morphology. Thus, a 15-day recovery period helped lessen the impacts of microplastics in lower dosages but was insufficient to eliminate them; by contrast, it was never adequate at high concentrations (Table 4). The discovered RBC alternations impacted normal blood flow because they affected their capacity for deformation (Figure 3C) and subsequently led to the formation of cellular aggregations.
Blood viscosity is linearly connected to hematocrit since it depends on the quantity (and number) of erythrocytes in the blood (Nader et al., 2019). At low shear rates (such as in the veins), hematocrit (HT) has a more significant effect on blood viscosity than it does at high shear rates (such as in the arteries) (Cokelet et al., 2007). According to estimates, a one unit increase in hematocrit at high shear rates would result in a 4% increase in blood viscosity (if RBC rheological properties remain the same). In the current investigation, the 60 and 600 μg/ml MPs groups had considerably lower HT levels. Additionally, at the higher MP concentrations (60 and 600 μg/ml MPs), hemoglobin (Hb) levels were much lower, which is thought to be an indication of sickle cell anemia (Magrì et al., 2018). Additionally, RBCs become more fragile and susceptible to hemolysis when they lose their deformability (Connes et al., 2014). Vaso-occlusion and pre-capillary obstruction can both be caused by stiff, sickle-shaped RBCs (Rees et al., 2010).
After exposure to MPs, the biochemical markers (creatinine, AST, ALT, glucose, and total protein) significantly increased. Creatinine can be used as a biomarker for renal impairment and as an indicator of glomerular filtration rate (Lien et al., 2006).
Enzymes (AST and ALT) are found in the cells of several organs throughout the body (Lenaerts et al., 2005). These enzymes’ release and increased blood levels are signs of damaged cell membranes (Lenaerts et al., 2005). According to our findings, paraquat and/or microplastic particles increased the activity of intracellular enzymes (ALT and AST), which may be a sign that cell plasma membranes have been damaged (Cheng et al., 2022). Proteins are essential for preserving physiological homeostasis and in stopping blood leak from the circulatory system (Bergmeier and Hynes 2012). Increased levels of total protein result from MP exposure, and these suggest problems in the kidney and liver functions.
With low MP dosages, when compared to the control group, microplastics buildup and hemato-biochemical changes were improved after the recovery period. However, the high dose group was affected negatively by MPs. The recovery period has an effect ranging from cells to tissues where the defense mechanisms were reported (Hamed et al., 2019; Hamed et al. 2020; Hamed et al. 2021; Sayed et al., 2021; Ammar et al., 2022; Sayed et al., 2022; Sayed et al., 2022).
In C57BL/6 mice, microplastics produced a range of toxic consequences, which included anemia and changes in hemato-biochemical parameters, which may induce severe toxic effects in all organs at higher concentrations and for extended periods. Our findings have shown that MPs had damaging effects on mice’s RBCs, reflecting the dangerous implications of these MPs on human health. The current study may initiate future comprehensive studies on the impacts of MPs on other body systems.
The raw data supporting the conclusions of this article will be made available by the authors, without undue reservation.
The animal study was reviewed and approved by the Research Ethical Committee of the Molecular Biology Research and Studies Institute (IORG0010947-MB-21-10-A), Assiut University, Assiut, Egypt.
SA-Z, MM, and AS: conceptualization; SA-Z and AS: methodology; SA-Z and AS: visualization and investigation; SA-Z, MM, and AS: data curation and writing—original draft preparation. All authors: final draft writing—reviewing and editing.
The authors declare that the research was conducted in the absence of any commercial or financial relationships that could be construed as a potential conflict of interest.
All claims expressed in this article are solely those of the authors and do not necessarily represent those of their affiliated organizations, or those of the publisher, editors, and reviewers. Any product that may be evaluated in this article, or claim that may be made by its manufacturer, is not guaranteed or endorsed by the publisher.
al-Sabti, K., and Metcalfe, C. D. (1995). Fish micronuclei for assessing genotoxicity in water. Mutat. Res. 343 (2-3), 121–135.
Ammar, E., Mohamed, M. S., and Sayed, A. E.-D. H. (2022). Polyethylene microplastics increases the tissue damage caused by 4-nonylphenol in the common carp (Cyprinus carpio) juvenile. Front. Mar. Sci. 9. doi:10.3389/fmars.2022.1041003
Andrady, A. L. (2011). Microplastics in the marine environment. Mar. Pollut. Bull. 62, 1596–1605. doi:10.1016/j.marpolbul.2011.05.030
Avio, C. G., Gorbi, S., Milan, M., Benedetti, M., Fattorini, D., d’Errico, G., et al. (2015). Pollutants bioavailability and toxicological risk from microplastics to marine mussels. Environ. Pollut. 198, 211–222. doi:10.1016/j.envpol.2014.12.021
Baskurt, O. K., and Meiselman, H. J. (2003). Blood rheology and hemodynamics. Seminars Thrombosis Hemostasis 5 (29), 435–450. doi:10.1055/s-2003-44551
Bergmeier, W., and Hynes, R. O. (2012). Extracellular matrix proteins in hemostasis and thrombosis. Cold Spring Harb. Perspect. Biol. 2 (4), a005132. doi:10.1101/cshperspect.a005132
Blackburn, K., and Green, D. (2022). The potential effects of microplastics on human health: What is known and what is unknown. Ambio 51 (3), 518–530. doi:10.1007/s13280-021-01589-9
Chandrasekharan, B. P., Foster, S. L., Saeedi, B., Weinshenker, D., Neish, A. S., Ling, C., et al. (2021) Lactobacillus rhamnosus gg (lgg)-induced enteric neuronal differentiation attenuates opioid-induced constipation sa1100 ingested microplastics pose a potentially serious risk to the gastrointestinal microenvironment sa1102 protective effect of Alismatis rhizoma on dextran sulfate sodium-induced colitis mice model.
Cheng, W., Li, X., Zhou, Y., Yu, H., Xie, Y., Guo, H., et al. (2022). Polystyrene microplastics induce hepatotoxicity and disrupt lipid metabolism in the liver organoids. Sci. Total Environ. 806, 150328. doi:10.1016/j.scitotenv.2021.150328
Cokelet, G. R., Meiselman, H. J., Baskurt, O. K., Hardeman, M. R., Rampling, M. W., and Meiselman, H. J. (2007). Macro- and micro-rheological properties of blood. Handbook of hemorheology and hemodynamics. Amsterdam: IOS Press.
Cole, M., Lindeque, P., Fileman, E., Halsband, C., Goodhead, R., Moger, J., et al. (2013). Microplastic ingestion by zooplankton. Environ. Sci. Technol. 12 (47), 6646–6655. doi:10.1021/es400663f
Connes, P., Lamarre, Y., Waltz, X., Ballas, S. K., Lemonne, N., Etienne-Julan, M., et al. (2014). Haemolysis and abnormal haemorheology in sickle cell anaemia. Br. J. Haematol. 4 (165), 564–572. doi:10.1111/bjh.12786
De Stephanis, R., Giménez, J., Carpinelli, E., Gutierrez-Exposito, C., and Cañadas, A. (2013). As main meal for sperm whales: Plastics debris. Mar. Pollut. Bull. 69 (1-2), 206–214. doi:10.1016/j.marpolbul.2013.01.033
Deng, Y., Zhang, Y., Lemos, B., and Ren, H. (2017). Tissue accumulation of microplastics in mice and biomarker responses suggest widespread health risks of exposure. Sci. Rep. 7, 46687. doi:10.1038/srep46687
Desforges, J.-P. W., Galbraith, M., Dangerfield, N., and Ross, P. S. (2014). Widespread distribution of microplastics in subsurface seawater in the NE Pacific Ocean. Mar. Pollut. Bull. 1–2 (79), 94–99. doi:10.1016/j.marpolbul.2013.12.035
Gallagher, P. G. (2017). Disorders of erythrocyte hydration. Blood 130 (25), 2699–2708. doi:10.1182/blood-2017-04-590810
Galloway, T. S. (2015). “Micro- and nano-plastics and human health,” in Marine anthropogenic litter (New York: Springer International Publishing), 343–366.
Glader, B. E., and Sullivan, D. W. (1979). Erythrocyte disorders leading to potassium loss and cellular dehydration. Prog. Clin. Biol. Res. 30, 503–513.
Gregorio, D., Teuten, E., Eriksen, M., Morishige, C., Applegate, B., Foley, D., et al. (2009). in Proceedings of the International Research Workshop on the Occurrence, Effects, and Fate of Microplastic Marine Debris, Tacoma, WA, USA, September 9-11, 2008.
Hamed, M., Osman, A. G. M., Badrey, A. E. A., Soliman, H. A. M., and Sayed, A. E. H. (2021). Microplastics-induced eryptosis and poikilocytosis in early-juvenile nile Tilapia (Oreochromis niloticus). Front. Physiol. 12, 742922. doi:10.3389/fphys.2021.742922
Hamed, M., Soliman, H. A. M., Osman, A. G. M., and Sayed, A. E. D. H. (2019). Assessment the effect of exposure to microplastics in nile Tilapia (Oreochromis niloticus) early juvenile: I. Blood biomarkers. Chemosphere 228, 345–350. doi:10.1016/j.chemosphere.2019.04.153
Hamed, M., Soliman, H. A. M., Osman, A. G. M., and Sayed, A. E. H. (2020). Antioxidants and molecular damage in Nile Tilapia (Oreochromis niloticus) after exposure to microplastics. Environ. Sci. Pollut. Res. Int. 27 (13), 14581–14588. doi:10.1007/s11356-020-07898-y
Hämer, J., Gutow, L., Köhler, A., and Saborowski, R. (2014). Fate of microplastics in the marine isopod idotea emarginata. Environ. Sci. Technol. 48 (22), 13451–13458. doi:10.1021/es501385y
Hou, B., Wang, F., Liu, T., and Wang, Z. (2021). Reproductive toxicity of polystyrene microplastics: In vivo experimental study on testicular toxicity in mice. J. Hazard. Mater. 405, 124028. doi:10.1016/j.jhazmat.2020.124028
Huang, S., Huang, X., Bi, R., Guo, Q., Yu, X., Zeng, Q., et al. (2022). Detection and analysis of microplastics in human sputum. Environ. Sci. Technol. 56 (4), 2476–2486. doi:10.1021/acs.est.1c03859
Ibrahim, Y. S., Tuan Anuar, S., Azmi, A. A., Wan Mohd Khalik, W. M. A., Lehata, S., Hamzah, S. R., et al. (2021). Detection of microplastics in human colectomy specimens. JGH open 5 (1), 116–121. doi:10.1002/jgh3.12457
Imhof, H. K., Ivleva, N. P., Schmid, J., Niessner, R., and Laforsch, C. (2013). Contamination of beach sediments of a subalpine lake with microplastic particles. Curr. Biol. 23, R867. doi:10.1016/j.cub.2013.09.001
Inhorn, M. C., and Patrizio, P. (2015). Infertility around the globe: New thinking on gender, reproductive technologies and global movements in the 21st century. Hum. Reprod. Update 214, 411–426. doi:10.1093/humupd/dmv016
Jabeen, N., Majid, I., and Nayik, G. A. (2015). Bioplastics and food packaging: A review. Cogent Food Agric. 1 (1), 1117749. doi:10.1080/23311932.2015.1117749
Katsnelson, A. (2015). News Feature: Microplastics present pollution puzzle. Proc. Natl. Acad. Sci. U. S. A. Natl. Acad. Sci. 112, 5547–5549. doi:10.1073/pnas.1504135112
Koelmans, A. A., Gouin, T., Thompson, R., Wallace, N., and Arthur, C. (2014). Plastics in the marine environment. Environ. Toxicol. Chem. 1 (33), 5–10. doi:10.1002/etc.2426
Lechner, A., and Ramler, D. (2015). The discharge of certain amounts of industrial microplastic from a production plant into the River Danube is permitted by the Austrian legislation. Environ. Pollut. 200, 159–160. doi:10.1016/j.envpol.2015.02.019
Lenaerts, A. J., Johnson, C. M., Marrieta, K. S., Gruppo, V., and Orme, I. M. (2005). Significant increases in the levels of liver enzymes in mice treated with anti-tuberculosis drugs. Int. J. Antimicrob. Agents 2 (26), 152–158. doi:10.1016/j.ijantimicag.2005.04.011
Li, B., Ding, Y., Cheng, X., Sheng, D., Xu, Z., Rong, Q., et al. (2020). Polyethylene microplastics affect the distribution of gut microbiota and inflammation development in mice. Chemosphere 244, 125492. doi:10.1016/j.chemosphere.2019.125492
Lien, Y.-H. H., Yong, K.-C., Cho, C., Igarashi, S., and Lai, L.-W. (2006). S1P1-selective agonist, SEW2871, ameliorates ischemic acute renal failure. Kidney Int. 9 (69), 1601–1608. doi:10.1038/sj.ki.5000360
Lusher, A. L., Tirelli, V., O'Connor, I., and Officer, R. (2015). Microplastics in Arctic polar waters: The first reported values of particles in surface and sub-surface samples. Sci. Rep. 5, 14947. doi:10.1038/srep14947
Magrì, D., Sánchez-Moreno, P., Caputo, G., Gatto, F., Veronesi, M., Bardi, G., et al. (2018). Laser ablation as a versatile tool to mimic polyethylene terephthalate nanoplastic pollutants: Characterization and toxicology assessment. ACS Nano 12 (8), 7690–7700. doi:10.1021/acsnano.8b01331
Mattsson, K., Ekvall, M. T., Hansson, L.-A., Linse, S., Malmendal, A., and Cedervall, T. (2015). Altered behavior, physiology, and metabolism in fish exposed to polystyrene nanoparticles. Environ. Sci. Technol. 1 (49), 553–561. doi:10.1021/es5053655
Nader, E., Skinner, S., Romana, M., Fort, R., Lemonne, N., Guillot, N., et al. (2019). Blood rheology: Key parameters, impact on blood flow, role in sickle cell disease and effects of exercise. Front. Physiology 10, 1329. doi:10.3389/fphys.2019.01329
Pironti, C., Notarstefano, V., Ricciardi, M., Motta, O., Giorgini, E., and Montano, L. (2023). First evidence of microplastics in human urine, a preliminary study of intake in the human body. Toxics 11 (1), 40. doi:10.3390/toxics11010040
Pollastro, R. M., and Pillmore, C. L. (1987). Mineralogy and petrology of the Cretaceous-Tertiary boundary clay bed and adjacent clay-rich rocks, Raton Basin, New Mexico an Colorado. J. Sediment. Petrology 57, 456–466.
Pop, G. A., Duncker, D. J., Gardien, M., Vranckx, P., Versluis, S., Hasan, D., et al. (2002). The clinical significance of whole blood viscosity in (cardio)vascular medicine. Neth. Heart J. 12 (10), 512–516.
Prata, J. C., da Costa, J. P., Lopes, I., Duarte, A. C., and Rocha-Santos, T. (2020). Environmental exposure to microplastics: An overview on possible human health effects. Sci. total Environ. 702, 134455. doi:10.1016/j.scitotenv.2019.134455
Ragusa, A., Svelato, A., Santacroce, C., Catalano, P., Notarstefano, V., Carnevali, O., et al. (2021). Plasticenta: First evidence of microplastics in human placenta. Environ. Int. 146, 106274. doi:10.1016/j.envint.2020.106274
Rees, D. C., Williams, T. N., and Gladwin, M. T. (2010). Sickle-cell disease. Lancet 9757 (376), 2018–2031. doi:10.1016/S0140-6736(10)61029-X
Remigante, A., Spinelli, S., Straface, E., Gambardella, L., Caruso, D., Falliti, G., et al. (2022). Antioxidant activity of quercetin in a H2O2-induced oxidative stress model in red blood cells: Functional role of band 3 protein. Int. J. Mol. Sci. 23, 10991. doi:10.3390/ijms231910991
Rillig, M. C. (2012). Microplastic in terrestrial ecosystems and the soil? Environ. Sci. Technol. 46, 6453–6454. doi:10.1021/es302011r
Rochman, C. M., Hoh, E., Kurobe, T., and Teh, S. J. (2013). Ingested plastic transfers hazardous chemicals to fish and induces hepatic stress. Sci. Rep. 3, 3263. doi:10.1038/srep03263
Rudenko, S. V., and Saeid, M. K. (2010). Reconstruction of erythrocyte shape during modified morphological response. Biochem. Mosc. 75 (8), 1025–1031. doi:10.1134/s0006297910080110
Sayed, A. E.-D. H., Hamed, M., and Ismail, R. F. (2022). Natural antioxidants can improve microplastics-induced male reproductive impairment in the african catfish (Clarias gariepinus). Front. Environ. Sci. 9. doi:10.3389/fenvs.2021.811466
Sayed, A. E. H., Hamed, M., Badrey, A. E. A., and Soliman, H. A. M. (2021). Bioremediation of hemotoxic and oxidative stress induced by polyethylene microplastic in Clarias gariepinus using lycopene, citric acid, and chlorella. Comp. Biochem. Physiol. C Toxicol. Pharmacol. 250, 109189. doi:10.1016/j.cbpc.2021.109189
Sayed, A. E. H., Hana, M. N., Hamed, M., Abdel-Latif, H. M. R., Lee, J. S., and Soliman, H. A. M. (2022). Protective efficacy of dietary natural antioxidants on microplastic particles-induced histopathological lesions in African catfish (Clarias gariepinus). Environ. Sci. Pollut. Res. Int. doi:10.1007/s11356-022-23789-w
Setälä, O., Fleming-Lehtinen, V., and Lehtiniemi, M. (2014). Ingestion and transfer of microplastics in the planktonic food web. Environ. Pollut. 185, 77–83. doi:10.1016/j.envpol.2013.10.013
Smith, M., Love, D. C., Rochman, C. M., and Neff, R. A. (2018). Microplastics in seafood and the implications for human health. Curr. Environ. health Rep. 5, 375–386. doi:10.1007/s40572-018-0206-z
Sun, R., Xu, K., Yu, L., Pu, Y., Xiong, F., He, Y., et al. (2021). Preliminary study on impacts of polystyrene microplastics on the hematological system and gene expression in bone marrow cells of mice. Ecotoxicol. Environ. Saf. 218, 112296. doi:10.1016/j.ecoenv.2021.112296
Svetina, S. (2012). Red blood cell shape and deformability in the context of the functional evolution of its membrane structure. Cell Mol. Biol. Lett. 17 (2), 171–181. doi:10.2478/s11658-012-0001-z
Thompson, R. C., Moore, C. J., Saal, F. S. V., and Swan, S. H. (2009). Plastics, the environment and human health: Current consensus and future trends. Philosophical Trans. R. Soc. B Biol. Sci. R. Soc. 364, 2153–2166. doi:10.1098/rstb.2009.0053
Wang, Y.-L., Lee, Y.-H., Hsu, Y.-H., Chiu, I.-J., Huang, C. C.-Y., Huang, C.-C., et al. (2021). The kidney-related effects of polystyrene microplastics on human kidney proximal tubular epithelial cells HK-2 and male C57bl/6 mice. Environ. Health Perspect. 5 (129), 57003. doi:10.1289/EHP7612
Wright, S. L., Thompson, R. C., and Galloway, T. S. (2013). The physical impacts of microplastics on marine organisms: A review. Environ. Pollut. (Barking, Essex 1987) 178, 483–492. doi:10.1016/j.envpol.2013.02.031
Zhao, L., Shi, W., Hu, F., Song, X., Cheng, Z., and Zhou, J. (2021). Prolonged oral ingestion of microplastics induced inflammation in the liver tissues of C57BL/6J mice through polarization of macrophages and increased infiltration of natural killer cells. Ecotoxicol. Environ. Saf. 227, 112882. doi:10.1016/j.ecoenv.2021.112882
Keywords: mice, PE-MPs, erythrocytes, helmet cells, liver functions
Citation: Abdel-Zaher S, Mohamed MS and Sayed AE-DH (2023) Hemotoxic effects of polyethylene microplastics on mice. Front. Physiol. 14:1072797. doi: 10.3389/fphys.2023.1072797
Received: 25 October 2022; Accepted: 14 February 2023;
Published: 08 March 2023.
Edited by:
Pengcheng Shi, Southern Medical University, ChinaReviewed by:
Zhiwu Jiang, Houston Methodist Research Institute, United StatesCopyright © 2023 Abdel-Zaher, Mohamed and Sayed. This is an open-access article distributed under the terms of the Creative Commons Attribution License (CC BY). The use, distribution or reproduction in other forums is permitted, provided the original author(s) and the copyright owner(s) are credited and that the original publication in this journal is cited, in accordance with accepted academic practice. No use, distribution or reproduction is permitted which does not comply with these terms.
*Correspondence: Alaa El-Din H. Sayed, YWxhYXNheWVkQGF1bi5lZHUuZWc=
Disclaimer: All claims expressed in this article are solely those of the authors and do not necessarily represent those of their affiliated organizations, or those of the publisher, the editors and the reviewers. Any product that may be evaluated in this article or claim that may be made by its manufacturer is not guaranteed or endorsed by the publisher.
Research integrity at Frontiers
Learn more about the work of our research integrity team to safeguard the quality of each article we publish.