- Department of Poultry Science, University of Georgia, Athens, GA, United States
Bone issues such as osteoporosis are major concerns for the laying hen industry. A study was conducted to improve bone-health in pullets. A total of 448 one-day-old Hyline W36 pullets were randomly assigned to four treatments (8 rep; 14 birds/rep) until 17 weeks (wks). Dietary treatments were: 1) vitamin D3 at (2,760 IU/kg) (D), 2) vitamin D3 (2,760 IU/kg)+62.5 mg 25-(OH)D3/ton (H25D), 3) vitamin D3 (2,760 IU/kg) + 62.5 mg 25-(OH)D3/ton + high Ca&P (H25D + Ca/P), and 4) vitamin D3 (2,760 IU/kg) + high Ca&P (D + Ca/P). The high calcium (Ca) and phosphorus (P) diet was modified by increasing both high calcium and phosphorus by 30% (2:1) for the first 12 wks and then only increasing P for 12–17 wks to reduce the Ca to P ratio. At 17 wk, growth performance was measured, whole body composition was measured by dual energy x-ray absorptiometry (DEXA), and femur bones were scanned using Micro-computed tomography (Micro-CT) for bone 3D structure analyses. The data were subjected to a one-way ANOVA using the GLM procedure, with means deemed significant at p < 0.05. There was no significant outcome for growth performance or dual energy x-ray absorptiometry parameters. Micro-computed tomography results indicated that the H25D + Ca/P treatment had lower open pore volume space, open porosity, total volume of pore space, and total porosity in the cortical bone compared to the D + Ca/P. It also showed that a higher cortical bone volume/tissue volume (BV/TV) in the H25D + Ca/P than in the D + Ca/P. Furthermore, the H25D + Ca/P treatment had the lowest trabecular pattern factor and structure model index compared to the other treatments, which indicates its beneficial effects on trabecular structural development. Moreover, the H25D + Ca/P had a higher trabecular percentage compared to the D and 25D, which suggests the additional high calcium and phosphorus supplementation on top of 25D increased trabecular content in the cavity. In conclusion, the combination of 25D with higher levels of high calcium and phosphorus could improve cortical bone quality in pullets and showed a beneficial effect on trabecular bone 3D structural development. Thus, combination of a higher bio-active form of vitamin D3 and higher levels of high calcium and phosphorus could become a potential feeding strategy to improve bone structural integrity and health in pullets.
Introduction
The ever-increasing improvement in laying hen nutrition and genetics has resulted in vastly more efficient hens capable of laying more than 340 eggs by 72 weeks of age (Lohmann, 2016; Preisinger, 2018). With advancements challenges, come with increased susceptibility to bone problems as demonstrated by osteoporosis and bone fractures in laying hens (Lohmann, 2016). Therefore, a better understanding of bone modeling; restoration before or during egg laying periods would be crucial to address bone development and health issues. One of the physiological changes in laying hens is that the structural bone development ceases at sexual maturity, meaning that the elongation, widening, and mineralization of structural bones (cortical and trabecular) discontinue when laying starts (Whitehead, 2004; Kim et al., 2012; Chen et al., 2020a). Hens lay eggs continuously without molting, suggesting no opportunity for the possibility of cortical bone regeneration once laying starts (Whitehead, 2004). Hence, rearing is a vital period for establishing optimal bone mineralization and structural development with implications on eggshell quality and bone health during laying (Regmi et al., 2015; Chen et al., 2020a; Chen et al., 2020b).
Osteoporosis is described as a progressive decrease in the amount of mineralized structural bone and leads to bone fragility and susceptibility to fracture (Kim et al., 2012; Toscano, 2018). These anomalies can also diminish skeletal calcium reserves and contribute to the seriousness of osteoporosis. However, even in the lack of caged layer paralysis, osteoporosis is prevalent in laying flocks (Whitehead and Fleming, 2000) and is a foremost contributory factor in the high incidence (approx. 30%) of hens experiencing fractures (Gregory and Wilkins, 1989; Clark et al., 2008; Kim et al., 2012). Nutritional deficiencies of calcium, phosphorus, or cholecalciferol have been shown to result in bone loss attributable to osteomalacia (Wilson and Duff, 1991) and are likely to lead to greater severity of osteoporosis (Clark et al., 2008; Kim et al., 2012).
Numerous pullet studies have addressed the importance of early bone development, and its prolonged effects on bone health during laying periods (Casey-Trott et al., 2017; Chen et al., 2020a; Chen et al., 2020b; Hester et al., 2013; Kim et al., 2012; Regmi et al., 2015). However, prior nutritional studies seldom focused on the pullet period because osteoporosis was observed during the laying period; it was not successful to reverse it by using nutritional approaches targeting the laying period (Rennie et al., 1997; Kim et al., 2012; Chen et al., 2020a). Thus, exploring early intervention for resolving late issues of osteoporosis in laying hens is recommended.
25-Hydroxyvitamin D3 (H25D) is an intermediate form of vitamin D3 and is now readily available for commercial use in the poultry industry since 2006 (Adhikari et al., 2020). H25D has been numerously studied in broiler research for enhancing bone health and Ca and P absorption (Kim et al., 2011; Wideman et al., 2015; Han et al., 2016; Świątkiewicz et al., 2017; Chen et al., 2020a; Chen et al., 2020b). However, only few studies on laying hens and especially pullets for developing the healthy bone structures for performance and maintenance during the laying period (Käppeli et al., 2011; Nascimento et al., 2014; Silva, 2017; Adhikari et al., 2020; Chen et al., 2020a; Chen et al., 2020b). The exploration of nutrients simply during a laying phase is too late for finding ways to fix or improve concerns with bone issues that should have been prevented in the rearing period to avoid the ultimate outcome of osteoporosis. Even human clinical trials demonstrated that there was little to no effect of supplementation of vitamin D or calcium on fracture occurrences in elder populations (Zhao et al., 2017).
Previous research has found that an additional 25-(OH)D3 in the pullet diet stimulated bone growth, increased bone size, and created more pores in the cortical bone during a pullet period, which allowed more mineral deposition in the bones during a laying period (Chen et al., 2020a), maintaining better bone structural integrity and health in the later laying period. Due to the larger structure size, the bone mineral density (BMD) was decreased in 25-(OH)D3 treatment during the pullet period, and this was possibly attributed to the calcium (Ca) and available phosphorous (P) level and ratio in the diets which were not ideal to provide sufficient Ca and P to induce proper mineral deposition in increased bone size by H25D (Chen et al., 2020a). Thus, increasing minerals, such as calcium and phosphorus in addition to H25D supplementation in rearing diets, can ultimately develop structurally sound bones and in the long run can possibly improve laying hen performances while maintaining bone health. Therefore, a study was conducted to explore the effect of dietary 25-Hydroxyvitamin D3combined with modified Ca and P level and ratio on bone development and mineralization in pullets. The objective was to evaluate the effect of H25D combined with modified Ca and P level and ratio in the diets on growth performance, body composition, and bone 3D structural development in pullets.
Materials and methods
Animal and housing
The study was conducted at the research facility of the Department of Poultry Science at the University of Georgia. The trial was conducted in accordance with the Institutional Animal Care and Use Committee at the University of Georgia. A total of 448 one-day-old Hyline W36 chicks were randomly assigned to 4 dietary treatments (8 replicates and14 birds/replicate) and raised until 17 wks. Chicks were obtained from at Hy-line North America hatchery (Mansfield, GA). Birds were housed in colony cages for 17 wks [(90 cm (L) × 46 cm (W) × 38 cm (H)]. Water and experimental diets were offered ad libitum from 0 to 17 wks. There were two nipples per cage. The pullets received an intermittent lighting program during the first 7 days with 4 h of light followed by 2 h of dark circles. The lighting management was customized by Hy-line North America lighting program throughout 2–17 wks (http://sales.hyline.com/NALighting/WebLighting.aspx).
Experimental diets
The diets were designed based on the Hy-Line W36 guide (2020). The formulation of corn-soybean based diet is shown in Table 1. Treatments consisted of: 1) control diet; vitamin D3 at (2,760 IU/kg)IU/kg (D), 2) vitamin D3 (2,760 IU/kg) + 62.5 mg 25-(OH)D3/ton (H25D), 3) vitamin D3 (2,760 IU/kg) + 62.5 mg 25-(OH)D3/ton + high Ca and P (H25D + Ca/P), and 4) vitamin D3 (2,760 IU/kg) + high Ca and P (D + Ca/P). The high Ca and P diet was modified by increasing 30% of both Ca and P (2:1) for the first 12 wks, and then only increasing P for 12–17 wks to reduce the Ca to P ratio (Table 2). The experimental diets were fed for 17 wks. The diets were freshly mixed every 3 weeks to minimize degradation of supplemented vitamin D3 or 25-(OH)D3 in the diets.
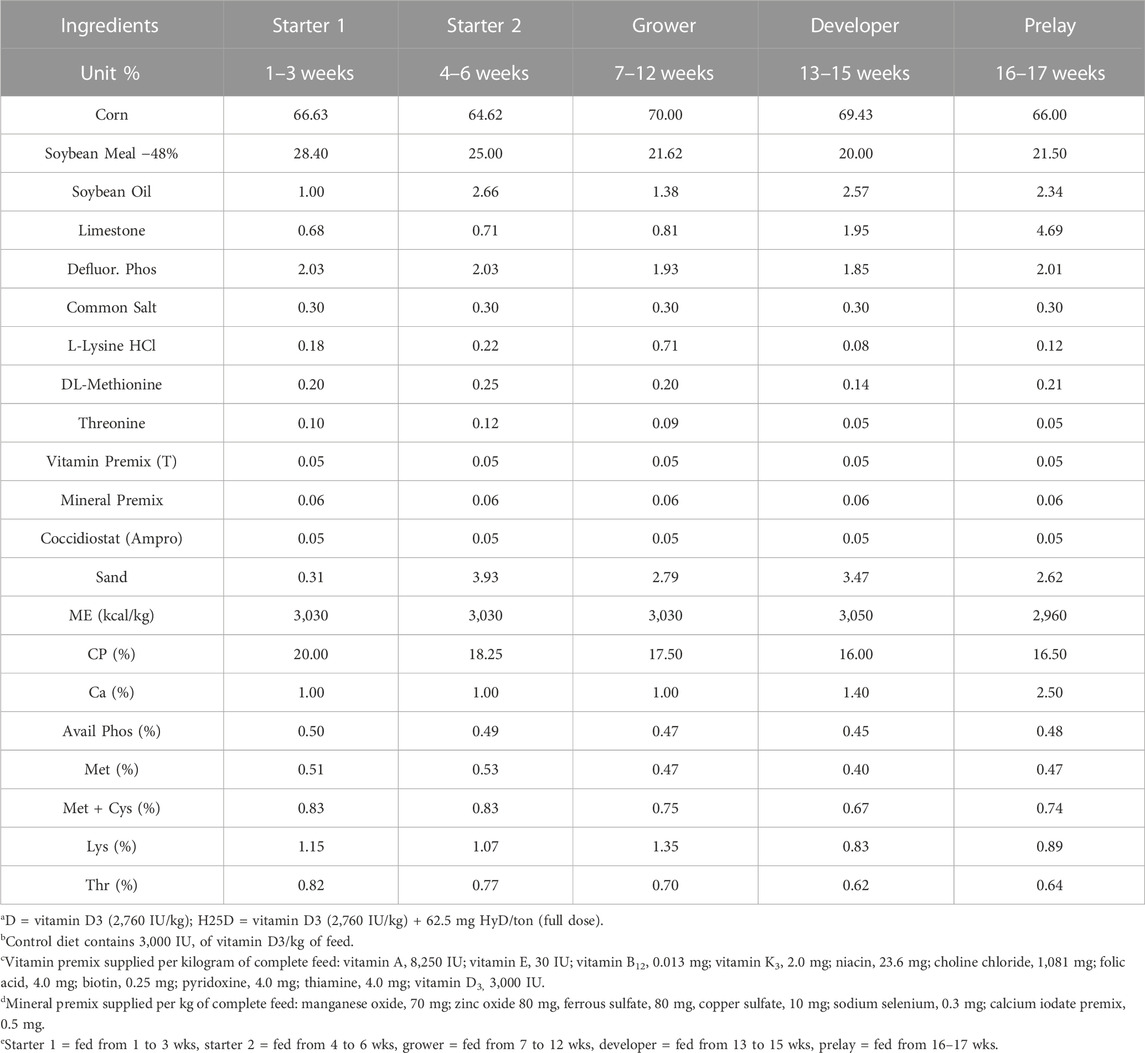
TABLE 1. Ingredients and diet composition for treatments D and H25D of the control diets fed to pullets at different stages for 17 weeks.
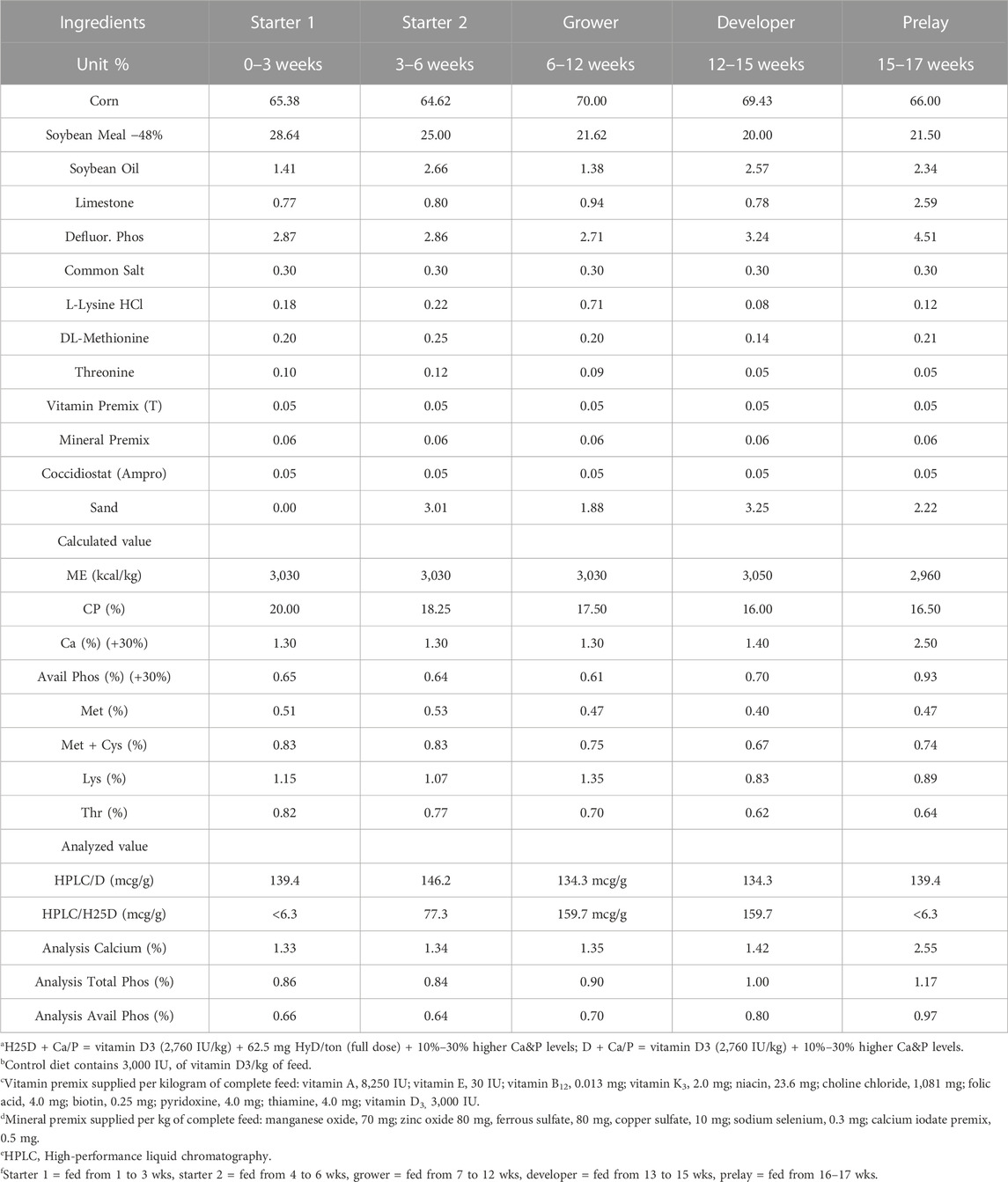
TABLE 2. Ingredients and diet composition for treatments H25D + Ca/P and D + Ca/P diets with higher levels of Ca&P fed to pullets at different stages for 17 weeks.
Serum 25-(OH)D3 content analysis
Blood samples (8 birds/treatment) were collected from the wing vein at 17 wks. After the blood was clotted, the blood samples were centrifuged at 1,500 g in a refrigerated centrifuge (Eppendorf Centrifuge 5430R; Eppendorf, Hamburg, Germany) for 12 min. The serum was collected and transferred into a clean polypropylene tube. The samples were maintained at −80°C until analysis. The serum 25-(OH)D3 level was determined using a mass spectrometry procedure (Heartland Assays, Ames, IA).
Growth performance measurement
Body weight (BW), body weight gain (BWG), and feed intake (FI) were recorded at 0, 3, 6, 12, 15, and 17 wks. The phase periods for recording are 0–3 wks (Starter 1 period), 4–6 wks (Starter 2 period), 7–12 wks (Grower period), 13–15 wks (Developer period), and 16–17 wks (Peak period). However, growth performance was reported for 0–17 wks.
Bone quality measurements
At 17 wks, two birds per cage (2 birds × 8 replicates = 16 birds per treatment) were euthanized by cervical dislocation. Dual energy x-ray absorptiometry (DEXA; pDEXA®, Bone Densitometer, General Electric Company, 41 Farnsworth Street, Boston, MA 02210, United States) was used for whole bird body composition analyses, and the whole bird was defined as a region of interest (Kim et al., 2004; Chen et al., 2020a; Adhikari et al., 2020). Whole body region scans were conducted to measure bone mineral density (g/cm2) (BMD), bone mineral content (g) (BMC), bone area (cm2), fat weight (kg), fat percent (%), muscle weight (kg), muscle percentage (%), and total body weight (kg). Each sample bird was placed chest up on the scanner at the same position and orientation during the measurement. All scans were obtained at a scan speed of 2.5 mm/s, with a voxel resolution of 0.07 mm × 0.07 mm × 0.50 mm.
The left femur bone was taken from one bird per pen (8 bones/treatment) at 17 wks of age. After the soft tissue was removed completely, the samples were wrapped with PBS-soaked cheesecloth to keep the bones moist. The femur bones were scanned with micro-computed tomography (Micro-CT; Skyscan 1275; Bruker micro-CT, Kartuizersweg 3B Kontich, Belgium) which was used for 3- dimensional image acquisition (Chen and Kim, 2020; Yamada et al., 2021). The femur bone was held in a low-density 50 ml tube; extra cheesecloth was used for holding the sample in a vertical orientation and firmly inside the container. The container was then mounted on the scanning stage. Scan settings and definitions/explanations of measurements are shown in Table 3. Before the scanning, the alignment test and flat field correction were performed for calibration according to the Bruker Micro-CT manual (Bruker micro-CT, Kartuizersweg 3B Kontich, Belgium). Random movement and 180-degree scanning were applied along with a 0.5 mm aluminum filter used to reduce beam hardening. The x-ray source was set at 80 kV and 125 µA. The pixel size was fixed at 25 μm, the rotation angle of 0.40 was applied at each step, and four images per rotation were captured. A series of 2-D images were capturedand later used to reconstruct a 3-D image using N-Recon (Bruker Micro-CT, Billerica, MA). After scanning, the pictures were carefully screened, and the appropriate alignment and mathematical method correction were used (beam-hardening correction: 35%; smoothing: NA; ring artifact reduction: NA). The 3-D model was then repositioned using Data Viewer software (Bruker Micro-CT, Billerica, MA) to ensure the consistency of selecting the region of interests for the following analysis. The volume of interest was selected using CTAn software (Bruker Micro-CT, Billerica, MA). The volume of interest was defined as the section of the bone from which morphometry and density measurements were analyzed. The bone section chosen was a distal supracondylar region from which a total of 300 slides/frames were analyzed. This 300 slide/frame section is the epiphyseal plate region of the long bone. Two phantoms (8 mm diameter) of known density (0.25 and 0.75 g/cm3) for calcium hydroxyapatite [(Ca5(PO4)3(OH)] were scanned to allow for calibration of bone mineral density (Sharma et al., 2021). The different parts of bones were separated according to the methods described by Chen and Kim (2020).
Statistical analysis
Data were subjected to statistical analyses using the general linear model procedure (GLM) of SAS (SAS Institute Inc., Cary, NC). One-way ANOVA was used with model Yij = µ +αi +εij. Significant differences among the treatments were determined using GLM procedures. Data were considered significantly different at p ≤ 0.05, and trends (0.05 ≤ p ≤ 0.1) were also presented (Choi et al., 2021).
Results
In the current study, the supplementation of different isoforms of vitamin D (D or H25D) along with higher Ca and P levels did not have an impact on growth performance parameters (p > 0.05) (Table 4) or whole bird body composition parameters (Table 5).
However, changes in mineral deposition and 3D structural changes were observed in the femurs. For cortical bone analyses by Micro-CT, the H25D + Ca/P had significantly higher cortical bone volume/tissue volume (BV/TV) compared to the D + Ca/P (Table 6). 25-(OH)D3 with higher Ca and P levels (H25D + Ca/P) had significantly lower open pore volume space, open porosity (% cortical tissue volume), total volume of pore space, and total porosity (% cortical tissue volume) in the cortical bone compared to vitamin D3 with higher Ca and P levels (D + Ca/P) (p < 0.05) (Table 7; Figure 1).
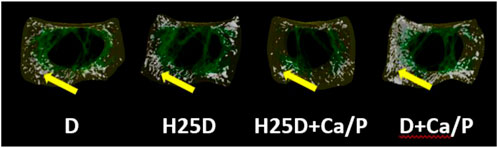
FIGURE 1. Total porosity within the cortical bone region. 1) Arrow indicating the open pores within the cortical region of the bone. 2) D = vitamin D3 (2,760 IU/kg); H25D = vitamin D3 (2,760 IU/kg) + 62.5 mg HyD/ton (full dose); H25D + Ca/P = vitamin D3 (2,760 IU/kg) + 62.5 mg HyD/ton (full dose) + 10%–30% higher Ca&P levels; D + Ca/P = vitamin D3 (2,760 IU/kg) + 10%–30% higher Ca&P levels. 3) Please refer to Table 3 for definitions of parameters.
The H25D + Ca/P treatment had the lowest trabecular pattern factor (TPF) (p = 0.009) and structure model index (SMI) (p = 0.031) among the treatments (Table 8; Figure 2). Moreover, H25D + Ca/P had a higher trabecular percentage (trabecular bone/cavity volume%) compared to D and H25D (p < 0.05).
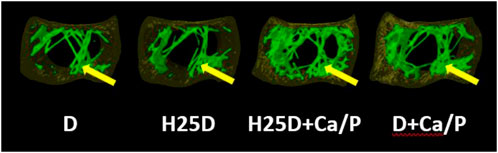
FIGURE 2. Total pattern factor, matrix, and thickness within the trabecular bone region. 1) Arrow indicating the pattern factor, matrix, and thickness within the trabecular region of the bone. 2) D = vitamin D3 (2,760 IU/kg); H25D = vitamin D3 (2,760 IU/kg) + 62.5 mg HyD/ton (full dose); H25D + Ca/P = vitamin D3 (2,760 IU/kg) + 62.5 mg HyD/ton (full dose) + 10%–30% higher Ca&P levels; D + Ca/P = vitamin D3 (2,760 IU/kg) + 10%–30% higher Ca&P levels. 3) Please refer to Table 3 for definitions of parameters.
The 25-(OH)D3 level in the serum was measured in the present study. The treatments with H25D always had the highest 25-(OH)D3 level in the serum compared to the other treatments (p < 0.0001) (Table 9).
Discussion
Bone mineral acquisition is organized by a range of factors ranging from genetic determinants and nutritional influences on the hormonal balance, including traditional regulation of mineral homeostasis by vitamin D3 (Dusso et al., 2005; Bouillon et al., 2008). Vitamin D3 is obtained from dietary sources and is synthesized in the skin by photo-conversion of 7-dehydrocholesterol to vitamin D3, which afterwards undergoes two main modifications (Chen et al., 2020a). Firstly, it is metabolized in the liver to produce the circulating form 25-hydroxyvitamin D3 (H25D, calcidiol), which is later converted in the kidney and other tissues including bone by 1α-hydroxylase to generate the active form 1,25-hydroxyvitamin D3 (1,25(OH)2D3, calcitriol) (Chen et al., 2020a). 1,25(OH)2D3 is the primary hormonal form of vitamin D3 which binds to vitamin D receptor (VDR), inducing a broad range of biological responses (Dusso et al., 2005; Bouillon et al., 2008).
The advantage of H25D during the rearing period is observed more commonly through the bone parameters of pullets such as the cortical and trabecular regions of the bone (Chen et al., 2020a). In the current study, the rearing growth parameters were not affected by H25D. This result agrees with studies reporting that different concentrations and forms of vitamin D3 did not affect the growth parameters of pullets (Wen et al., 2019; Chen et al., 2020a; Li et al., 2021). Moreover, the dual-energy X-ray absorptiometry scanning did not detect beneficial changes by vitamin D3 and Ca and P in the current study for the rearing period. Similar results were observed in the previous study (Chen et al., 2020a) where at 17 wk, there was no difference in whole-body BMD and BMC while feeding birds with 25D.
With 3D scanning and automatic bone separation process by Micro-CT, the effects of dietary H25D + Ca/P supplementation on pullet bone 3-dimensional structural changes were observed in the present study. In a previous study by Chen et al. (2020a), the H25D increased cortical bone size and porosity significantly but reduced cortical bone density compared to the D treatment alone, suggesting that mineral supplementation or utilization may not be adequate to fill in the larger cortical bone size. For the current study, the primary function of H25D in early bone development was to increase the bone structural size, and to fill the “larger” bone structure with the increased Ca and P in order to close the open pores within the cortical region. This may contribute to bone fracture resistance, as the cortical bone represents 70%–80% of the total bone mass, which is found on the outer surface of bones and its primary function is to support rigidity to the bone, with its relative ratio depending on the function and mechanical load of the respective bone (Digirolamo et al., 2013). It is distinguished by a volumetric fraction (bone mass volume/total volume ratio), which explains its common name of compact bone (Digirolamo et al., 2013). In previous research, the expansion of the cortical bone size with the same amount of BMC resulted in a low-density bone (Chen et al., 2020a). A low BMD is commonly associated with an elevated risk of bone fracture (Ammann and Rizzoli, 2003). A larger cortical region does not necessarily correlate to stronger or more structurally sound bones without having the proper mineral deposition (Estefania et al., 2019).
Inside the cortical cortex, the coupling between osteoblast and osteoclast activities is carried out in the matrix of cortical pores which provide a conduit for the movement of neurovascular structures (Rique et al., 2019). Cortical porosity is considered to be correlated with mechanical properties of cortical bone, manipulating bone strength and risk of fracture (Li et al., 2021). Because larger cavities indicate increased osteoclast recruitment and activity, there is a conjecture that higher bone resorption, either alone or in combination with inhibited osteoblast function, accounts for higher porosity. There is evidence that cortical diameter of pores increases with increased porosity, which can potentially result in decreased overall bone density (Rique et al., 2019). However, decreased porosity and cortical diameter of pores can potentially lead to better overall bone density. In addition, a recent concept of two individual osteoblast types positioned in the cortical bone (Shapiro, 2008), creates another likelihood, that is 1,25(OH)2D3 selectively suppresses the intracortical osteoblasts coating primary osteons, but without altering surface mesenchymal osteoblasts lining the periosteum. The current study observed a larger cortical bone size with increased pores, which were filled in with elevated levels of calcium (from 30% early on to 10% at later stages) and phosphorus (30% throughout) provided in the diet. This was further observed by the trending volume of closed pores and closed porosity for the H25D + Ca/P treatment, which complements the findings with the lower open pore space. Therefore, the nutritional strategy with H25D + Ca/P during rearing periods have potential to create a more compact and structurally sound bone.
In the present study, higher content of trabecular bone in the H25D treatment was found during the rearing period. The trabecular bone corresponds to 20%–30% of the bone mass, which is in the internal section of the bone (Digirolamo et al., 2013). It has a porous reticular structure with variable and rather low density that provides flexibility and strength to the whole bone structure. It is also distinguished by a relatively low volumetric fraction, offsetting with a surface area nearly twice as large as the compact bone (Digirolamo et al., 2013). Poultry bones were largely based on bone ash, breaking strength, or DEXA (Hester et al., 2004; Castro et al., 2019; Adhikari et al., 2020). These approaches are based on the results of planar morphology or bone mass. Although bone quantity and density are important factors for bone strength (Hester et al., 2004), these parameters do not consider the trabecular architectural changes that are independently related to bone strength (Siffert et al., 1996; Webber et al., 1998). An avian model study determined that over 10% loss of trabecular bone could impact bone strength (Reich and Gefen, 2006), indicating that the integrity of trabecular bone is essential for bone resistance to force. The increase of trabecular bone content within the cavity in the H25D treatment suggested the higher fracture resistance elevated by dietary supplementation. The current findings of a significant decrease in TPF and SMI by the H25D + Ca/P vs. the control D, which was complemented by higher trabecular thickness, number and bone mineral density along with lower separation for the H25D treatment is in agreement with a study (Idelevich et al., 2011). The study observed that inclusion of H25D increased trabecular number and thickness, while having a corresponding decrease in trabecular separation in rats. The study also revealed an increase in overall trabecular bone content with the inclusion of H25D. The correspondence of the SMI, which is an index evaluating whether trabecular bone is rod-like or plate-like, and a smaller value means a more plate-like structure (Hildebrand & Rüegsegger, 1997) and TPF, which is an index evaluating rod-like, plate-like, or honeycomb-like structure, and a small value means a more plate-like to honeycomb-like structure (Hahn et al., 1992), resembles a positive outcome when both parameters are lower as observed in this current study. This correlation of having both SMI and TPF lower also results in increasing overall trabecular structure by increasing connectivity (Chiba et al., 2010; Yokota et al., 2020). Furthermore, for both SMI and TPF, the smaller values indicate the positive plate-like or honeycomb-like structure also results in less osteoporosis cases vs. the rod-like structure increases osteoporosis incidences (Felder et al., 2020).
This study suggests that early supplementation of the combination of H25D with higher level of Ca and P could improve the cortical bone microstructure and have beneficial effects on trabecular bone 3D structural development in pullets. The combination of a higher bio-active forms of vitamin D3 and higher levels of Ca and P during rearing periods may become a promising feeding strategy to enhance pullet bone quality.
Data availability statement
The raw data supporting the conclusion of this article will be made available by the authors, without undue reservation.
Ethics statement
The animal study was reviewed and approved by the Institutional Animal Care and Use Committee at the University of Georgia.
Author contributions
DW, CC, and WK conceived and designed this study. DW and CC contributed to pullet husbandry and sample collection, DW and CC contributed to Micro-CT assay. DW contributed to the data analyses. The paper was written through contribution and critical review of the manuscript by all authors (DW, CC, and WK). All authors listed have made a substantial, direct and intellectual contribution to the work, and approved it for publication.
Conflict of interest
The authors declare that the research was conducted in the absence of any commercial or financial relationships that could be construed as a potential conflict of interest.
Publisher’s note
All claims expressed in this article are solely those of the authors and do not necessarily represent those of their affiliated organizations, or those of the publisher, the editors and the reviewers. Any product that may be evaluated in this article, or claim that may be made by its manufacturer, is not guaranteed or endorsed by the publisher.
References
Adhikari, R., White, D., House, J. D., and Kim, W. K. (2020). Effects of additional dosage of vitamin D3, vitamin D2, and 25-hydroxyvitamin D3 on calcium and phosphorus utilization, egg quality and bone mineralization in laying hens. Poult. Sci. 99 (1), 364–373. doi:10.3382/ps/pez502
Ammann, P., and Rizzoli, R. (2003). Bone strength and its determinants. Osteoporos. Int. 14, 13–18. doi:10.1007/s00198-002-1345-4
Bikle, D. D. (2017). Vitamin D: Production, metabolism and mechanisms of action. [Updated 2021 Dec 31]. In: K. R. Feingold, B. Anawalt, A. Boyceet al. editors. Endotext [internet]. South Dartmouth (MA): MDText.com, Inc.; Available from: https://www.ncbi.nlm.nih.gov/books/NBK278935/.
Bouillon, R., Carmeliet, G., Verlinden, L., Van Etten, E., Verstuyf, A., Luderer, H. F., et al. (2008). Vitamin D and human health: Lessons from vitamin D receptor null mice. Endocr. Rev. 29 (6), 726–776. doi:10.1210/er.2008-0004
Casey-Trott, T. M., Korver, D. R., Guerin, M. T., Sandilands, V., Torrey, S., and Widowski, T. M. (2017). Opportunities for exercise during pullet rearing, Part I: Effect on the musculoskeletal characteristics of pullets. Poult. Sci. 96, 2509–2517. doi:10.3382/ps/pex059
Castro, F. L. S., Su, S., Choi, H., Koo, E., and Kim, W. K. (2019). L-Arginine supplementation enhances growth performance, lean muscle, and bone density but not fat in broiler chickens. Poult. Sci. 98, 1716–1722. doi:10.3382/ps/pey504
Chen, C., and Kim, W. K. (2020). The application of micro-CT in egg-laying hen bone analysis: Introducing an automated bone separation algorithm. Poult. Sci. 99, 5175–5183. doi:10.1016/j.psj.2020.08.047
Chen, C., Turner, B., Applegate, T. J., Litta, G., and Kim, G. (2020a). Role of long-term supplementation of 25-hydroxyvitamin D3 on laying hen bone 3-dimensional structural development. Poult. Sci. 99, 5771–5782. doi:10.1016/j.psj.2020.06.080
Chen, C., Turner, B., Applegate, T. J., Litta, G., and Kim, W. K. (2020b). Role of long-term supplementation of 25-hydroxyvitamin D3 on egg production and egg quality of laying hen. Poult. Sci. 99, 6899–6906. doi:10.1016/j.psj.2020.09.020
Chen, C., Adhikari, R., White, D., and Kim, W. K. (2021a). Role of 1, 25-dihydroxyvitamin D3 on osteogenic differentiation and mineralization of chicken mesenchymal stem cells. Front. Physiol. 12, 479596. doi:10.3389/fphys.2021.479596
Chen, C., White, D., Marshall, B., and Kim, W. K. (2021b). Role of 25-hydroxyvitamin D3 and 1,25-dihydroxyvitamin D3 in chicken embryo osteogenesis, adipogenesis, myogenesis, and vitamin D3 metabolism. Front. Physiol. 12, 631629. doi:10.3389/fphys.2021.637629
Chiba, K., Ito, M., Osaki, M., Uetani, M., and Shindo, H. (2010). In vivo structural analysis of subchondral trabecular bone in osteoarthritis of the hip using multi-detector row CT. Osteoarthr. Cartil. 19, 180–185. doi:10.1016/j.joca.2010.11.002
Choi, J., Ko, H., Tompkins, Y. H., Teng, P. Y., Lourenco, J. M., Callaway, T. R., et al. (2021). Effects of eimeria tenella infection on key parameters for feed efficiency in broiler chickens. Animals 11, 3428. doi:10.3390/ani11123428
Clark, W. D., Cox, W. R., and Silversides, F. G. (2008). Bone fracture incidence in end-of-lay high-producing, noncommercial laying hens identified using radiographs. Poult. Sci. 87 (10), 1964–1970. doi:10.3382/ps.2008-00115
Digirolamo, D. J., Kiel, D. P., and Esser, K. A. (2013). Bone and skeletal muscle: Neighbors with close ties. J. Bone Min. Res. 28, 1509–1518. doi:10.1002/jbmr.1969
Dusso, A., González, E. A., and Martin, K. J. (2005). Vitamin D in chronic kidney disease. Best Pract. Res. Clin. Endocrinol. metabolism 25 (4), 647–655. doi:10.1016/j.beem.2011.05.005
Estefania, S. R., Reyes, C. B., Torres, C., Gasca, N. D., Ruiz, A. I. G., Lopez, S. G., et al. (2019). Changes with age (from 0 to 37 D) in tibiae bone mineralization, chemical composition and structural organization in broiler chickens. Poult. Sci. 98, 5215–5225. doi:10.3382/ps/pez363
Felder, A. A., Monzem, S., De Souza, R., Javaheri, B., Mills, D., Boyde, A., et al. (2020). The plate-to-rod transition in trabecular bone loss is elusive. R. Soc. Open Sci. 14, 081042. doi:10.1101/2020.05.14.081042
Gregory, N. G., and Wilkins, L. J. (1989). Broken bones in domestic fowl: Handling and processing damage in end-of-lay battery hens. Br. Poult. Sci. 30, 555–562. doi:10.1080/00071668908417179
Hahn, P. Y., Stroebel, J. J., and Hahn, F. J. (1992). Verification of lumbosacral segments on MR images; identification of transitional vertebrae. Radiology 182, 580–581. doi:10.1148/radiology.182.2.1732988
Han, J. C., Chen, G. H., Wang, J. G., Zhang, J. L., Qu, H. X., Zhang, C. M., et al. (2016). Evaluation of relative bioavailability of 25- hydroxycholecalciferol to cholecalciferol for broiler chickens. Asian-Australas J. Anim. Sci. 29, 1145–1151. doi:10.5713/ajas.15.0553
Hester, P., Schreiweis, M., Orban, J., Mazzuco, H., Kopka, M., Ledur, M., et al. (2004). Assessing bone mineral density in vivo: Dual energy X-ray absorptiometry. Poult. Sci. 83, 215–221. doi:10.1093/ps/83.2.215
Hester, P. Y., Enneking, S. A., Haley, B. K., Cheng, H. W., Einstein, M. E., and Rubin, D. A. (2013). The effect of perch availability during pullet rearing and egg laying on musculoskeletal health of caged White Leghorn hens. Poult. Sci. 92, 1972–1980. doi:10.3382/ps.2013-03008
Hildebrand, T., and Rüegsegger, P. (1997). Quantification of bone microarchitecture with the structure model index. Comput. Methods Biomech. Biomed. Engin 1 (1), 15–23. doi:10.1080/01495739708936692
Holick, M. F. (2007). Vitamin D deficiency. N. Engl. J. Med. 357 (3), 266–281. doi:10.1056/NEJMra070553
Hy-Line (2020). Hy-line variety W-36 commercial management guide 2020. West Des Moines (IA): Hy-Line International.
Idelevich, A., Kerschnitzki, M., Shahar, R., and Monsonego-Ornan, E. (2011). 1,25(OH)2D3 alters growth plate maturation and bone architecture in young rats with normal renal function. PLoS ONE 6(6): e20772. doi:10.1371/journal.pone.0020772
Jendral, M. J., Korver, D. R., Church, J. S., and Feddes, J. J. (2008). Bone mineral density and breaking strength of White Leghorns housed in conventional, modified, and commercially available colony battery cages. Poult. Sci. 87, 828–837. doi:10.3382/ps.2007-00192
Käppeli, S., Fröhlich, E., Gebhardt-Henrich, S. G., Pfulg, A., Schäublin, H., Zweifel, R., et al. (2011). Effects of dietary supplementation with synthetic vitamin D3 and 25-hydroxycholecalciferol on blood calcium and phosphate levels and performance in laying hens. Arch. Geflugelkd 75, 179–184.
Kim, W. K., Ford, B. C., Mitchell, A. D., Elkin, R. G., and Leach, R. M. (2004). Comparative assessment of bone among wild-type, restricted ovulator and out-of-production hens. Br. Poult. Sci. 45, 463–470. doi:10.1080/00071660412331286172
Kim, W. K., Donalson, L. M., Michell, A. D., Kubena, L. F., Nisbet, D. J., and Ricke, S. C. (2006). Effects of alfalfa and fructooligosaccharide on molting parameters and bone qualities using dual energy x-ray absorptiometry and conventional bone assays. Poult. Sci. 85, 15–20. doi:10.1093/ps/85.1.15
Kim, W. K., Donalson, L. M., Bloomfield, S. A., Hogan, H. A., Kubena, L. F., Nisbet, D. J., et al. (2007). Molt performance and bone density of cortical, medullary, and cancellous bone in laying hens during feed restriction or alfalfa-based feed molt. Poult. Sci. 86, 1821–1830. doi:10.1093/ps/86.9.1821
Kim, W. K., Bloomfield, S. A., and Ricke, S. C. (2011). Effects of age, vitamin D3, and fructooligosaccharides on bone growth and skeletal integrity of broiler chicks. Poult. Sci. 90, 2425–2432. doi:10.3382/ps.2011-01475
Kim, W. K., Bloomfield, S. A., Sugiyama, T., and Ricke, S. C. (2012). Concepts and methods for understanding bone metabolism in laying hens. World’s Poult. Sci. J. 68, 71–82. doi:10.1017/s0043933912000086
Landis, E., and Keane, D. T. (2010). X-ray microtomography. Mater. Charc 61 (12), 1305–1316. doi:10.1016/j.matchar.2010.09.012
Li, D., Zhang, K., Bai, S., Wang, J., Zeng, Q., Peng, H., et al. (2021). Effect of 25-hydroxycholecalciferol with different vitamin D3 levels in the hens diet in the rearing period on growth performance, bone quality, egg production, and eggshell quality. Bone Qual. Egg Prod. Eggshell Qual. Agri. 11, 698. doi:10.3390/agriculture11080698
Lohmann (2016). Lohmann Brown and LSL-lite commercial management guide. Available on: http://www.ltz.de/en/downloads/management-guides.php#anchor_0955c6a8_Accordion1-Cage (Accessed on October 29th, 2021).
Nakano, H., Matsunawa, M., Yasui, A., Adachi, R., Kawana, K., Shimomura, L., et al. (2005). Enhancement of ligand-dependent Vitamin D receptor transactivation by the cardiotonic steroid bufalin. Biochem. Pharm. 70, 1479–1486. doi:10.1016/j.bcp.2005.08.012
Nascimento, G. R. d., Murakami, A. E., Guerra, A., Ospinas-Rojas, I. C., Ferreira, M. F. Z., and Fanhani, J. C. (2014). Effect of different vitamin D sources and calcium levels in the diet of layers in the second laying cycle. Rev. Bras. Cienc. Avic. 16, 37–42. doi:10.1590/1516-635x160237-42
Preisinger, R. (2018). Innovative layer genetics to handle global challenges in egg production. Br. Poult. Sci. 59, 1–6. doi:10.1080/00071668.2018.1401828
Regmi, P., Deland, T. S., Steibel, J. P., Robison, C. I., Haut, R. C., Orth, M. W., et al. (2015). Effect of rearing environment on bone growth of pullets. Poult. Sci. 94, 502–511. doi:10.3382/ps/peu041
Regmi, P., Smith, N., Nelson, N., Haut, R. C., Orth, M. W., and Karcher, D. M. (2016a). Housing conditions alter properties of the tibia and humerus during the laying phase in Lohmann white Leghorn hens. Poult. Sci. 95, 198–206. doi:10.3382/ps/pev209
Regmi, P., Nelson, N., Steibel, J. P., Anderson, K. E., and Karcher, D. M. (2016b). Comparisons of bone properties and keel deformities between strains and housing systems in end-of-lay hens. Poult. Sci. 95, 2225–2234. doi:10.3382/ps/pew199
Reich, T., and Gefen, A. (2006). Effect of trabecular bone loss on cortical strain rate during impact in an in vitro model of avian femur. Biomed. Eng. Online 5, 45. doi:10.1186/1475-925X5-45
Rennie, J. S., Fleming, R. H., Mccormack, H. A., Mccorquodale, C. C., and Whitehead, C. C. (1997). Studies on effects of nutritional factors on bone structure and osteoporosis in laying hens. Br. Poult. Sci. 38, 417–424. doi:10.1080/00071669708418012
Rique, A. M., Madeira, M., Luis, L., Mlde, F., Fleius Mlde, F., Paranhos, N. F., et al. (2019). Evaluating cortical bone porosity using Hr-Pqct. Arch. Clin. Exp. Orthop. 3, 008–013. doi:10.29328/journal.aceo.1001006
Shapiro, F. (2008). Bone development and its relation to fracture repair. The role of mesenchymal osteoblasts and surface osteoblasts. Eur. Cell. Mater 15, 53–76. doi:10.22203/ecm.v015a05
Sharma, M. K., White, D., Kim, W. K., and Adhikari, P. (2021). Effects of the housing environment and laying hen strain on tibia and femur bone properties of different laying phases of Hy-Line hens. Poult. Sci. 100 (3), 100933. doi:10.1016/j.psj.2020.12.030
Siffert, R., Luo, G., Cowin, S., and Kaufman, J. (1996). Dynamic relationships of trabecular bone density, architecture, and strength in a computational model of osteopenia. Bone 18, 197–206. doi:10.1016/8756-3282(95)00446-7
Silva, F. A. (2017). Effects of dietary 25-hydroxycholecalciferol on growth, production performance, eggshell quality and bone traits of brown egg layers housed under commercial conditions. University of Alberta, Edmonton.
ŚwiĄTkiewicz, S., Arczewska-Włosek, A., Bederska-Lojewska, D., and Józefiak, D. (2017). Efficacy of dietary vitamin D and its metabolites in poultry - review and implications of the recent studies. Poult. Sci. J. 73, 57–68. doi:10.1017/s0043933916001057
Tactacan, G. B., Guenter, W., Lewis, N. J., Rodriguez-Lecompte, J. C., and House, J. D. (2009). Performance and welfare of laying hens in conventional and enriched cages. Poult. Sci. 88, 698–707. doi:10.3382/ps.2008-00369
Toscano, M. (2018). in Skeletal problems in contemporary commercial laying hens. Editor J. A. Mench 1st ed (Elsevier Ltd).
Webber, C. E., Gordon, C. L., and Nicholson, P. S. (1998). Relation between image-based assessment of distal radius trabecular structure and compressive strength. Can. Assoc. Radiol. J. 49, 390–397.
Wen, J., Livingston, K. A., and Persia, M. E. (2019). Effect of high concentrations of dietary vitamin D3 on pullet and laying hen performance, skeleton health, eggshell quality, and yolk vitamin D3 content when fed to W36 laying hens from day of hatch until 68 wk of age. Poult. Sci. 98, 6713–6720. doi:10.3382/ps/pez386
Whitehead, C. C., and Fleming, R. H. (2000). Osteoporosis in cage layers. Poult. Sci. 79 (7), 1033–1041. doi:10.1093/ps/79.7.1033
Whitehead, C. C. (2004). Overview of bone biology in the egg-laying hen. Poult. Sci. 83, 193–199. doi:10.1093/ps/83.2.193
Wideman, R., Blankenship, J., Pevzner, I., and Turner, B. (2015). Efficacy of 25-OH Vitamin D3 prophylactic administration for reducing lameness in broilers grown on wire flooring. Poult. Sci. 94, 1821–1827. doi:10.3382/ps/pev160
Wilson, S., and Duff, S. R. I. (1991). Effects of vitamin or mineral deficiency on the morphology of medullary bone in laying hens. Res. Vet. Sci. 50, 216–221. doi:10.1016/0034-5288(91)90110-a
Yamada, M., Chen, C., Sugiyama, T., and Kim, W. K. (2021). Effect of age on bone structure parameters in laying hens. Animals 11, 570. doi:10.3390/ani11020570
Yokota, K., Chiba, K., Okazaki, N., Kondo, C., Doi, M., Yamada, S., et al. (2020). Deterioration of bone microstructure by aging and menopause in Japanese healthy women: Analysis by HR-pQCT. J. Bone Min. Metab. 38, 826–838. doi:10.1007/s00774-020-01115-z
Keywords: 25-hydroxyvitamin D 3, bone 3D structure, pullets, micro-CT (μCT) scanning technology, bone health
Citation: White D, Chen C and Kim WK (2023) Effect of the combination of 25-hydroxyvitamin D3 and higher level of calcium and phosphorus in the diets on bone 3D structural development in pullets. Front. Physiol. 14:1056481. doi: 10.3389/fphys.2023.1056481
Received: 28 September 2022; Accepted: 30 March 2023;
Published: 24 April 2023.
Edited by:
Colin Guy Scanes, University of Wisconsin–Milwaukee, United StatesReviewed by:
Katarzyna B. Miska, Agricultural Research Service (USDA), United StatesKyung-Woo Lee, Konkuk University, Republic of Korea
Copyright © 2023 White, Chen and Kim. This is an open-access article distributed under the terms of the Creative Commons Attribution License (CC BY). The use, distribution or reproduction in other forums is permitted, provided the original author(s) and the copyright owner(s) are credited and that the original publication in this journal is cited, in accordance with accepted academic practice. No use, distribution or reproduction is permitted which does not comply with these terms.
*Correspondence: Woo Kyun Kim, d2traW1AdWdhLmVkdQ==