- Department of Pharmacology, University of California, Davis, Davis, CA, United States
Ion channels that influence membrane potential and intracellular calcium concentration control vascular smooth muscle excitability. Voltage-gated calcium channels (VGCC), transient receptor potential (TRP) channels, voltage (KV), and Ca2+-activated K+ (BK) channels are key regulators of vascular smooth muscle excitability and contractility. These channels are regulated by various signaling cues, including protein kinases and phosphatases. The effects of these ubiquitous signaling molecules often depend on the formation of macromolecular complexes that provide a platform for targeting and compartmentalizing signaling events to specific substrates. This manuscript summarizes our current understanding of specific molecular complexes involving VGCC, TRP, and KV and BK channels and their contribution to regulating vascular physiology.
Introduction
The diameter of small resistance arteries is a critical determinant of blood flow and tissue perfusion. The contractility of vascular smooth muscle (VSM) regulates arterial diameter. Multiple ion channels regulate VSM contraction by controlling membrane potential and the magnitude of intracellular calcium concentration [Ca2+]i (Knot and Nelson, 1995). In VSM, voltage-dependent L-type CaV1.2 (LTCCs) channels are the main Ca2+ influx pathway for contraction (Ghosh et al., 2017), and a role for T-type channels regulation of VSM excitability is also described (Cribbs, 2006; Abd El-Rahman et al., 2013). Transient receptor potential (TRP) channels contribute to vascular function by regulating membrane potential, contraction, and myogenic tone development (Brayden et al., 2008). Voltage (KV) and Ca2+-activated K+ (BK) channels provide a negative-feedback regulation of VGCC activity, hence Ca2+ influx and contraction, by modulating VSM membrane potential. Different stimuli within the body, including variations in pressure, vasoactive substances released from endothelial cells, and nerve terminals, modulate contraction by initiating cellular signaling that impinges on the function of these ion channels.
Signaling complexes permit efficient transduction of the many signals received with the specificity necessary to support function. VGCC, K+, and TRP channels form complexes with key proteins (e.g., ion channels, GPCR, signaling molecules) that modulate their function and, in doing so, regulate VSM contractility. Scaffold proteins, such as AKAP5, have been shown to facilitate molecular complex formation with signaling and effectors proteins in VSM. For more extensive reviews on the different ion channels, readers are directed to (Earley and Brayden, 2015; Ghosh et al., 2017; Tykocki et al., 2017). Here we will summarize current knowledge on specific molecular complexes involving LTTC, TTCC, TRP, KV, and BK channels and their contribution to regulating vascular physiology.
Voltage-gated calcium channels
Voltage-gated Ca2+ channels (VGCC) are found in various cell types throughout the body. Changes in membrane potential activate these channels, leading to Ca2+ influx and the regulation of many physiological processes (Catterall, 2011). VGCC comprises a family of ten members, subdivided into three major subfamilies, CaV1, CaV2, and CaV3, based on their biophysical properties (Catterall, 2000; Catterall, 2011). Members of the CaV1 and CaV2 families have been identified in VSM (Tykocki et al., 2017). The CaV1 family activates at depolarized membrane potential and is characterized by large conductance and long openings, hence their name L-type Ca2+ channels (LTCC). Meanwhile, the CaV2 or T-type (TTCC) family of VGCC has tiny currents that activate at more negative potentials than the LTCC and inactivate fast (Catterall, 2011). Of relevance to this review is the LTCC, which is necessary for the VSM myogenic contraction (Moosmang et al., 2003), and the TTCC that emerging data suggest participate in arterial tone regulation (Cribbs, 2006; Abd El-Rahman et al., 2013; Harraz et al., 2015a). In the following section, we summarize the contributions of these channels to VSM physiology, emphasizing their participation in the signaling domains that facilitate the regulation of VSM excitability.
CaV1.2 signaling complexes in VSM
L-type calcium channel CaV1.2 is the main entryway for Ca2+ in vascular smooth muscle contributing Ca2+ for contraction and gene expression. Indeed, Ca2+ influx through CaV1.2 is necessary for pressure-induced contraction (i.e., myogenic tone), and studies suggest that about 50% of phenylephrine-induced contraction is due to Ca2+ influx via these channels (Moosmang et al., 2003). Moreover, Ca2+ influx via CaV1.2 channels is linked to transcriptional regulation in VSM (Wamhoff et al., 2004; Wamhoff et al., 2006; Kudryavtseva et al., 2013). The altered function of the CaV1.2 channels is associated with contractility and gene expression changes. For instance, studies associate changes in CaV1.2 function with increased myogenic tone in conditions such as hypertension (Nieves-Cintron et al., 2008; Navedo et al., 2010a) and diabetes (Navedo et al., 2010b; Nystoriak et al., 2014; Nystoriak et al., 2017a).
LTCCs are heteromeric complexes of α1-, β, and α2δ subunits (Catterall, 2000). The α1-subunit, which forms the ion conduction pore, consists of four homologous domains (I-IV), each domain having six transmembrane segments (S1–S6) linked by three intracellular loops, and intracellular amino and carboxy-terminal. The homologous transmembrane segment S6 and the loop between S5 and S6 of each homologous domain form the ion conduction pore. Voltage sensitivity is provided by transmembrane segment S1-S4 (Catterall, 2011). β and α2δ subunits are auxiliary subunits that have been shown to contribute to membrane trafficking and regulate the channel biophysics (De Jongh et al., 1990; Singer et al., 1991; Gao et al., 1999; Dolphin, 2003; Catterall, 2011). Among the four known subtypes (β1 - β4), β3 is the principal subunit in VSM (Murakami et al., 2000; Kharade et al., 2013). The α2δ subunit arises from a single gene; subsequent posttranslational processing produces an extracellular α2 and membranal δ subunits that associate via a disulfide bridge to form a functional subunit (Gurnett et al., 1996; Arikkath and Campbell, 2003). Three different α2δ delta isoforms have been identified (α2δ1 - α2δ3) (Klugbauer et al., 1999). The α2δ1subunit was critical for membrane expression of the CaV1.2 α1c in VSM from rat cerebral artery (Bannister et al., 2009).
Alterations in the subunit expression profiles have been linked to changes in physiology. For example, studies report increased expression of CaV1.2 α1c subunit in VSM in models of high blood pressure, including genetic models of hypertension (Pratt et al., 2002; Pesic et al., 2004). Moreover, increased expression of β3 subunit contributes to the upregulation of CaV1.2 α1c subunit membrane expression in VSM from animal models of hypertension owing to its role in the channel trafficking (Kharade et al., 2013). Elevation of the α2δ1 subunit expression during hypertension was also reported and linked with higher CaV1.2 membrane surface expression and currents (Bannister et al., 2012). Changes in the expression of LTCC subunits have also been reported in a genetic model of hypertension (BPH mice) (Tajada et al., 2013). However, while several studies report increases in the expression of the CaV1.2 α1c subunit in hypertension (Pratt et al., 2002; Pesic et al., 2004; Sonkusare et al., 2006), the study by Tajada et al. shows a decrease in CaV1.2 α1c expression in mesenteric artery VSM form BPH mice relative to normotensive control mice (Tajada et al., 2013). Accordingly, mesenteric VSM from BPH mice showed a reduction in CaV1.2 α1c and a change in the expression profile of the accessory subunits. The authors proposed that in normotensive BPN mice, CaV1.2 currents were carried by α1c/β3/α2δ, whereas in BPH mice, the current was likely mediated by channels composed of α1c/β2/α2δ subunits. This discrepancy could be due to the hypertension model used (e.g., angiotensin-induced vs. genetic hypertension), the species (rat vs. mice), and vascular beds. Nevertheless, the studies highlight the relevance of the accessory subunits in regulating LTCC activity and VSM contractility.
In VSM, the CaV1.2 channel forms molecular complexes with signaling components including receptors, enzymes, and effector proteins orchestrated by scaffolds proteins, such as the A-kinase anchoring protein 5 (AKAP5) (Tykocki et al., 2017). AKAPs are structurally diverse intracellular scaffolding proteins that bind PKA, PKC, calcineurin (PP2B), and CaV1.2, thus facilitating regulation of the channel by these proteins (Colledge and Scott, 1999; Langeberg and Scott, 2005). Optical recording of Ca2+ influx revealed that protein kinases and phosphatases modulate CaV1.2 activity in an AKAP-dependent manner in VSM (M Nieves-Cintron et al., 2018) (Figure 1). Fluorescent signals elicited by LTCC openings (CaV1.2-sparklets) were visualized using TIRF microscopy. Contrary to expectation, not all CaV1.2 channels had a similar open probability. Subpopulations of channels showed stochastic transient openings (low activity sparklets). In contrast, other channels displayed events with prolonged available time produced by activating two or more channels that generated areas of almost continuous calcium influx (Navedo et al., 2005). This high-activity mode, termed persistent sparklets, required PKC activity and AKAP5 expression (Navedo et al., 2006). Moreover, the phosphatase PP2B is part of the molecular complex, and its activity limits CaV1.2-persistent sparklets (Navedo et al., 2006). Persistent CaV1.2-sparklets contributed to approximately 50% of Ca2+ influx through LTCC (Amberg et al., 2007), highlighting the relevance of these events and the macromolecular complex that regulates them to VSM physiology.
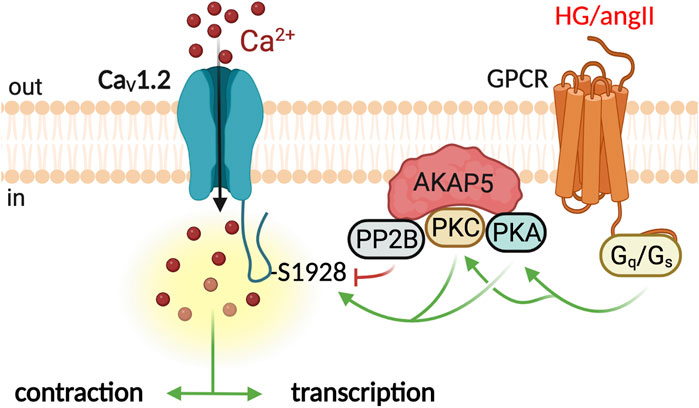
FIGURE 1. CaV1.2 molecular complex in the regulation of VSM contraction and transcription. Ca2+ influx through CaV1.2 channels is essential for the pressure-induced contraction of the VSM (Moosmang et al., 2003). Activation of Gs/PKA signaling by high glucose or Gq/PKC by angiotensin II potentiate CaV1.2 activity (Navedo et al., 2008; Navedo et al., 2010b; Prada et al., 2019). The scaffolding protein AKAP5 facilitates PKA/PKC phosphorylation of CaV1.2 channels at S1928 (Nystoriak et al., 2017a). The phosphatase PP2B opposes phosphorylation dampening CaV1.2 potentiation. Graphics created with BioRender.com.
CaV1.2-sparklets events are of higher frequency and amplitude in VSM from hypertensive mice than in corresponding control mice. In the angiotensin II-induced hypertension model, higher CaV1.2-sparklets required activation of PKC signaling (Navedo et al., 2008). Higher CaV1.2 sparklets increased arterial wall Ca2+, tone, and blood pressure. Interestingly, angiotensin II failed to elicit an increase in CaV1.2-sparklets in AKAP5 knockout mice (Navedo et al., 2008). Moreover, these mice did not develop hypertension in response to angiotensin infusion (Navedo et al., 2008). Thus, angiotensin II increases arterial tone and blood pressure by stimulating CaV1.2 sparklets locally in an AKAP5-dependent manner. VSM from murine models of diabetes also shows elevated Ca2+ sparklet activity, and AKAP5 is also central to the signaling events leading to higher CaV1.2 sparklet activity during diabetic hyperglycemia (Navedo et al., 2010b; Nieves-Cintron et al., 2021). AKAP5 orchestrates a signaling module that includes the purinergic receptor P2Y11, adenylyl cyclase 5, PKA, and the CaV1.2 subunit (Prada et al., 2019; Syed et al., 2019; Prada et al., 2020) (Figure 1). Activating this signaling module increases CaV1.2 activation and arterial contractility during diabetes. The fact that PKA was required for CaV1.2-sparklets during diabetes is surprising as this kinase is associated with vasodilation. It also highlights the exquisite level of signal specificity achieved by segregating receptors, signaling enzymes, and effector proteins (Colledge and Scott, 1999; Langeberg and Scott, 2005) into macromolecular complexes.
T-type calcium channel signaling complex in VSM
TTCC α1 subunit topology is similar to that of other VGCC. However, no auxiliary subunits have been co-purified with the TTCC, but studies suggest that TTCC activity may be modified by other proteins, including the LTCC auxiliary subunits (Perez-Reyes, 2006; Dolphin, 2016). TTCC subunits CaV3.1, CaV3.2, and CaV3.3 have been identified in VSM from several vascular beds, and emergent evidence points toward a contribution of these channels to the regulation of the VSM reactivity (Cribbs, 2006; Abd El-Rahman et al., 2013). TTCCs have been shown to contribute to vasoconstrictor responses in mesenteric arterioles (Gustafsson et al., 2001), skeletal muscle arteries (VanBavel et al., 2002), and retinal arteriolar (Fernandez et al., 2015) VSM. In cerebral VSM from rats and mice, CaV3.1 and CaV3.2 carry the nifedipine insensitive component of Ba2+ currents (Nikitina et al., 2007; Abd El-Rahman et al., 2013; Harraz et al., 2015b). Human cerebral arteries express CaV3.2 however the CaV3.1 described in rodents is replaced by the CaV3.3 isoform in these arteries (Harraz et al., 2015a). CaV3.1 and CaV3.3 activity were found to contribute to the pressure-induced constriction (Harraz et al., 2014; Harraz et al., 2015b). Interestingly, selective inhibition of CaV3.2 channels with micromolar Ni2+ concentration elicited depolarization and constriction of wild-type rat cerebral arteries (Harraz et al., 2014). Electron tomography of rat cerebral VSM showed microdomains consisting of caveolae and sarcoplasmic reticulum (SR). Immunogold labeling localized CaV3.2 and RyR to these microdomains (Harraz et al., 2014). The studies proposed that caveolae facilitate a Ca2+ signaling network that enables the coupling of CaV3.2 mediated Ca2+ influx with activation of RyR receptors leading to activation of Ca2+-activated potassium channels contributing to membrane potential hyperpolarization (Harraz et al., 2014; Harraz et al., 2015a; Harraz et al., 2015b). Thus, the segregation into a microdomain allows CaV3.2 to modulate arterial tone by regulating the RyR-BK channels axis (Harraz et al., 2014; Harraz et al., 2015a).
TRP channels signaling complexes in VSM
TRP channels are a superfamily of cation channels encoded by 28 genes. The superfamily is divided into six subfamilies based on sequence homology, which include TRPC (canonical), TRPV (vanilloid), TRPM (melastatin), TRPP (polycystin), TRPA (ankyrin), and TRPML (mucolipin) channels (Earley and Brayden, 2015). TRP channels comprise six membrane-spanning segments (S1-S6) with intracellular amino and carboxy terminals. Electron cryomicroscopy studies of the TRPV1 channel suggest a symmetrical four-fold arrangement with the S5 and S6 loops forming the ion path (Liao et al., 2013). VSM cells express several TRP channels, which contribute to regulating membrane potential, contraction, and myogenic tone development. Some TRP channels translate mechanosensitive G-coupled signaling into changes in membrane potential in VSM cells (Earley and Brayden, 2015), thus contributing to artery autoregulation. Recent studies uncovered a role for specific TRP channels in signaling networks and molecular complexes that contribute to regulating contractile state. Here we summarize these studies, emphasizing well-characterized TRP channel signaling complexes contributing to the regulation of VSM excitability.
TRPC3 & TRPC6
In VSM cells, the plasmalemmal canonical transient receptor potential 3 (TRPC3) channels decode G-protein coupled receptor (GPCR) signaling into a cation current that elicits cell depolarization (Earley and Brayden, 2015). Indeed, in VSM, TRPC3 channels are implicated in cerebral artery constriction in response to GPCR agonists such as angiotensin II and endothelin-1 (ET-1) (Reading et al., 2005; Xi et al., 2008; Earley and Brayden, 2015). Intriguingly, TRPC3 channels in VSM are activated by GPCR-phospholipase C (PLC) signaling independently of SR Ca2+ release and PKC (Xi et al., 2008). The agonist IP3 was shown to constrict rat (Sprague-Dawley (SD), male and female) cerebral arteries by facilitating coupling between type 1 inositol 1,4,5-trisphosphate receptor type 1 (IP3R1) and TRPC3 channels (Xi et al., 2008). Further studies showed that in cerebral artery (SD, male) VSM, the caveolae scaffolding protein caveolin-1 (cav-1) co-localizes the IP3R1 and TRPC3 channels close to each other (Adebiyi et al., 2011). This signaling complex allows IP3—induced coupling of IP3R1 and TRPC3, leading to TRPC3 activation and generation of cation current that depolarizes VSM, thus eliciting contraction (Adebiyi et al., 2011) (Figure 2).
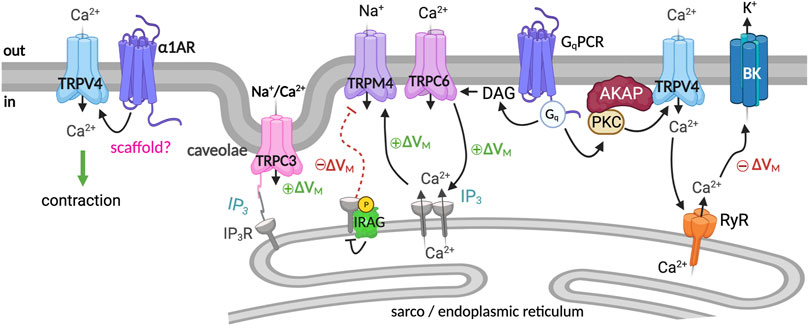
FIGURE 2. TRP channel signaling complex regulates VSM contractility. Several TRP channels are involved in regulating VSM excitability. Caveolae scaffolding protein caveolin-1 (cav-1) co-localizes the IP3R1 and TRPC3 channels close to each other. IP3—induced coupling of IP3R1 and TRPC3 leads to TRPC3 activation and VSM depolarization (Xi et al., 2008; Adebiyi et al., 2011). TRPC6/TRPM4, PLCγ1, and IP3R are part of a stretch-sensing signaling molecular complex that translates pressure-induced mechanical stimuli into VSM membrane depolarization (Reading and Brayden, 2007; Gonzales et al., 2014). PLC activation generates IP3, which sensitizes IP3Rs to TRPC6-mediated Ca2+ influx boosting SR Ca2+ release through IP3R channels to activate the TRPM4 channel causing cell depolarization and contraction (Gonzales et al., 2014). The NO/cGMP/PKG axis signals through IRAG to inhibit IP3R-mediated Ca2+ release from the SR, blunting the TRPM4 activity (Ali et al., 2021). TRPV4 is suggested to contribute to contraction and relaxation via the formation of distinct molecular complexes that are spatially segregated (Chen et al., 2022). The TRPV4/α1AR axis leads to agonist-induced contraction (Chen et al., 2022), whereas the TRPV4/BK complex hyperpolarizes the VSM membrane potential (Earley et al., 2005; Chen et al., 2022). Graphics created with BioRender.com.
TRPC6 channels also contribute to regulating pressure-induced VSM contraction (Welsh et al., 2002). Like TRPC3, TRPC6 channels are activated by GPCR-PLC agonists such as angiotensin II via mechanisms requiring diacylglycerol but independent of PKC (Helliwell and Large, 1997; Saleh et al., 2006). Studies using cerebral arteries from male SD rats suggest that TRPC6 channels are part of a stretch-sensing signaling network, including PLCγ1, TRPM4, and IP3Rs that translate pressure-induced mechanical stimuli into membrane depolarization of cerebral VSM (Gonzales et al., 2014) (Figure 2). According to the proposed model, initiation of PLCγ1 signaling generates IP3, which sensitizes IP3Rs to TRPC6-mediated Ca2+ influx. This process boosts SR Ca2+ release through IP3R, activating TRPM4 channels, cell depolarization, and artery constriction (Gonzales et al., 2014).
TRPM4
Increases in intraluminal pressure depolarize VSM and constrict cerebral arteries partly via the activation of the TRPM4 channel (Brayden et al., 2008). TRPM4 channels are selective for monovalent cations. Accordingly, TRPM4 channels are activated by Ca2+ but do not permeate this ion. Calmodulin binding sites have been identified on the C-terminus of the TRPM4 channel, which influences the sensitivity to Ca2+ (Nilius et al., 2005). Moreover, TRPM4 Ca2+ sensitivity is regulated by ATP and PKC-mediated phosphorylation of putative serine residues in the carboxy-terminal (Nilius et al., 2005). Suppressing TRPM4 expression in rat pial arteries compromises the ability of the arteries to regulate blood flow in response to changes in the mean arterial pressure (MAP) (Reading and Brayden, 2007), highlighting the physiological relevance of the channel for blood flow autoregulation. In rat’s cerebral VSM, TRPM4 channels are activated by local IP3R-mediated increase in [Ca2+]i (Gonzales et al., 2010). As mentioned above, with TRPC6, TRPM4 channels are part of a mechanosensitive signaling complex whereby IP3 is generated by activating GPCR-PLCγ1 signaling, which sensitizes IP3Rs to TRPC6-Ca2+ influx boosting IP3R-SR Ca2+ release to activate TRPM4 (Gonzales et al., 2014) (Figure 2). By regulating TRPM4 activity, this signaling complex may regulate pressure-induced constriction in cerebral arteries.
Intriguingly, a recent study suggests that the tissue soluble gaseous vasodilator nitric oxide could relax arteries by inhibiting the activity of TRPM4 channels (Ali et al., 2021) (Figure 2). NO is produced by endothelial cells and exerts its vasodilatory action by increasing soluble cyclic guanosine monophosphate (cGMP) and stimulating the activity of cGMP-activated protein kinase (PKG) (Lincoln et al., 1985; Carvajal et al., 2000). Using male and female C57BL/6J cerebral arteries, a mechanism was described involving the formation of a signaling complex on the SR of VSM comprised of PKG, IP3Rs, and IP3R-associated cGMP-kinase substrate (IRAG) (Ali et al., 2021). IRAG is a regulator of IP3-triggered Ca2+ release found to be complex with IP3Rs in the VSM (Geiselhoringer et al., 2004). Phosphorylation of IRAG by activation of the NO/cGMP/PKG axis was found to inhibit IP3R-mediated Ca2+ release from the SR (Geiselhoringer et al., 2004; Schlossmann and Desch, 2011). As a result of IP3R inhibition and concomitant SR Ca2+ release, TRPM4 channel activity is blunted. This leads to a reduction in TRPM4-mediated membrane depolarization and voltage-dependent Ca2+ channel activity leading to vasodilation (Ali et al., 2021). The formation of this NO/cGMP/PKG/IRAG signaling complex allows for distinct regulation of TRPM4 channel activity and, therefore, VSM membrane potential and contractile state.
TRPV4
VSM cells express transient receptor potential vanilloid 4 (TRPV4) channels. TRPV4 are Ca2+ permeable, nonselective cation channels, and their activation results in Ca2+ influx (Brayden et al., 2008). Interestingly, although the magnitude of the calcium influx through TRPV4 channels is significant, TRPV4 activity is linked to VSM relaxation (Earley et al., 2005). This has been attributed to relatively low basal TRPV4 activity and the formation of a signaling complex with ryanodine receptors (RyRs) and large-conductance Ca2+-activated potassium (BK) channels (Earley et al., 2005) (Figure 2). In cerebral and cerebellar arteries from male SD rats, activation of TRPV4 by endothelium-derived signals elicits Ca2+ influx that activates RyRs (Earley et al., 2005). RyR activation results in SR Ca2+ release (i.e., Ca2+-spark), coupled with the opening of BK channels at the plasma membrane of VSM cells. Activating the BK channels leads to VSM membrane hyperpolarization, decreased voltage-dependent Ca2+ channel activity, and intracellular Ca2+ concentration [Ca2+]i, thereby causing VSM relaxation (Knot et al., 1998).
Studies using cerebral arteries from rats, C57BL/6J, and AKAP150−/− mice suggest that in VSM, AKAP5 targeted PKCα facilitates regulation of TRPV4 channels by GqPCR signaling (Mercado et al., 2014; Tajada et al., 2017). Interestingly, a new study using mice mesenteric and human paraspinal muscle arteries submits that TRPV4 channels form spatially separated complexes with α1 adrenergic receptor (α1AR) and the BK channel, allowing them to respond differentially to different physiological stimuli (Chen et al., 2022). In this model, TRPV4 channels in proximity to the α1AR are activated by α1AR/GqPCR signaling and contribute to agonist-induced vasoconstriction. In contrast, pressure-induced activation of the TRPV4/BK channel axis opposes contraction (Chen et al., 2022) (Figure 2). The TRPV4/α1AR signaling axis was elevated in hypertension, whereas the TRPV4/BK complex was decreased, highlighting a potential new therapeutic target for hypertension. It will be important to determine if different scaffolds are responsible for the distinct spatial segregation of signaling components. The referenced studies suggest that forming distinct signaling complexes allows a diversity of the regulation and function of plasmalemmal TRP activity with profound consequences for VSM membrane potential and contractile state (Figure 2).
K+ channels
KV channels are a varied group of membrane proteins comprising at least 12 families, namely KV1-KV12 (Gutman et al., 2005). Structurally, KV channels are formed by tetrameric assembly of alpha subunits, which form the ion conduction pore and auxiliary beta subunits (Choe et al., 1999). The tetramer’s subunit composition and inclusion of auxiliary beta subunits provide functional and pharmacological diversity (Zhong et al., 2010a; Kilfoil et al., 2013; Tykocki et al., 2017). VSM expresses multiple KV channel subunits, including members of the KV1 (KV1.1, KV1.2, KV1.3, KV1.5, KV1.6) (Cheong et al., 2001; Albarwani et al., 2003; Amberg et al., 2003; Tykocki et al., 2017), and KV2 (KV2.1) (Amberg and Santana, 2006; Tykocki et al., 2017) families. Members of the KV7 (KV7.1–5) (Zhong et al., 2010b) and KV9 (KV9.3) (Zhong et al., 2010a; Tykocki et al., 2017) have also been identified. Homomeric and heteromeric channels consisting of an assembly of distinct alpha subunits with auxiliary KVβ have been found. For example, heteromultimeric channels consisting of KV1.2-KV1.5 subunits have been shown to contribute to vascular KV currents in VSM (Kerr et al., 2001; Albarwani et al., 2003; Plane et al., 2005). Heteromers of KV2.1 and silent KV9.3 subunits have been identified in VSM from rat cerebral arteries that contribute to regulating arterial diameter (Zhong et al., 2010a). Expression of KV6.3 silent subunit was reported in mesenteric VSM from hypertensive mice (Moreno-Dominguez et al., 2009). Studies in murine coronary microvasculature show an association between KV1.5 and auxiliary KVβ1 and KVβ2 (Nystoriak et al., 2017b). The study suggests that KVβ2 facilitates KV1.5 membrane trafficking in coronary VSM (Nystoriak et al., 2017b). KV channels control VSM contraction by regulating membrane potential and the magnitude of the VGCC-mediated Ca2+ influx (Knot and Nelson, 1995; Nystoriak and Navedo, 2017). Thus, mechanisms that regulate KV activity will impact VSM membrane potential, contractility, and ultimately arterial tone and diameter.
VSM KV channel activity is modulated by intracellular signaling (Ko et al., 2010). For example, several vasoconstrictors are shown to partially constrict arteries via PKC-dependent inhibition of KV channel activity (Aiello et al., 1996; Clement-Chomienne et al., 1996; Hayabuchi et al., 2001). In addition to PKC, the Src tyrosine kinase pathway has been shown to blunt KV activity and contract rat mesenteric arteries (Sung et al., 2013). On the other hand, protein kinase A (PKA) is associated with KV potentiation and vasodilation (Tykocki et al., 2017). For instance, β-adrenergic receptor agonists that engage adenyl cyclase (AC)/cAMP/PKA signaling promote 4-AP-sensitive KV channel activity in VSM and vasodilation (Aiello et al., 1995; Cole et al., 1996; Satake et al., 1996; Berwick et al., 2010). Despite the evidence of intracellular signaling regulation of KV activity and VSM contractility, the formation of macromolecular complexes that could facilitate this regulation is relatively unknown. Recent evidence, however, supports the role of the scaffolding protein postsynaptic density 95 (PSD95) in facilitating the regulation of KV channels by β-adrenergic signaling (Joseph et al., 2011; Moore et al., 2014; Moore et al., 2015). As its name implies, PSD95 is found at the postsynaptic density, where it facilitates the formation of macromolecular complexes between receptors, ion channels, and signaling molecules (Won et al., 2017). PSD95 was found to colocalize with the β1-adrenergic receptor in VSM and to enable phosphorylation of KV1 channels by PKA in rat cerebral VSM (Joseph et al., 2011; Moore et al., 2014). Thus, in cerebral VSM, PSD95 facilitates β-adrenergic-mediated vasodilatory regulation via the formation of a macromolecular complex with KV1 channels (Moore et al., 2015; Rhee and Rusch, 2018) (Figure 3). Whether other scaffolds are involved in KV channel regulation in VSM is an area for further research development in VSM.
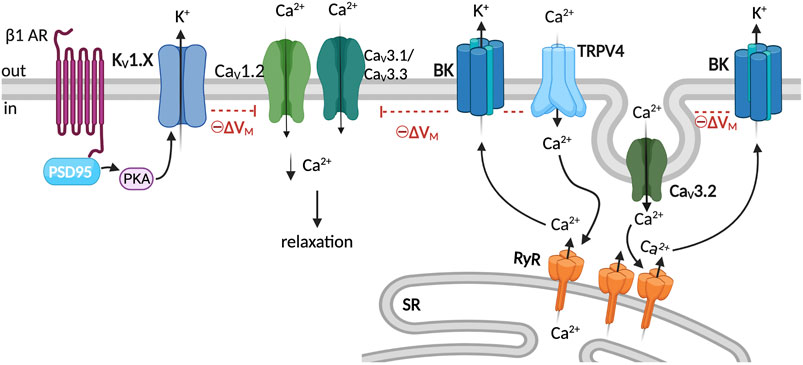
FIGURE 3. KV1 and BK complexes and membrane potential regulation. K+ channel activity provides negative-feedback regulation of membrane potential and limits CaV1.2 activity leading to VSM relaxation (Nelson, 1992). The scaffolding protein PSD95 facilitates the regulation of KV1 channels by β1AR/PKA signaling (Moore et al., 2015). BK channels are activated by the release of Ca2+ from the SR through RyR (Ca2+ sparks) (Jaggar et al., 1998). Ca2+ influx through TRPV4 channels activates the RyR/BK channel axis, which hyperpolarizes VSM membrane potential (Earley et al., 2005). Caveolae facilitate spatial proximity between CaV3.2 and RyR, CaV3.2-mediated Ca2+ influx prompts RyR, and the resultant Ca2+ sparks activate BK channels (Harraz et al., 2014; Hashad et al., 2018), which hyperpolarizes VSM membrane potential and limits VGCC activity leading to relaxation. Graphics created with BioRender.com.
BK-channels
Large-conductance Ca2+-activated potassium channels (BK) are critical regulators of VSM contractility by tonically regulating membrane potential (Jaggar et al., 1998). BK channels are activated by calcium and membrane depolarization (Latorre et al., 2017). The channel comprises tetrameric assemblies of pore-forming alpha subunits, which harbor the voltage sensor and cytosolic calcium-binding sites (Ko et al., 2008; Latorre et al., 2017). The BKα subunit assembles with auxiliary beta and gamma subunits. Four β (β1-β4) and four γ isoforms (γ1-γ4) have been identified so far (Li and Yan, 2016). The β1 subunit is the main β subunit isoform expressed in VSM cells; it increases BK calcium sensitivity and alters the biophysical properties of the channel (Brenner et al., 2000). The γ1 subunit has been reported in rat cerebral VSM (male SD) cells and suggested to increase the BK channel voltage sensitivity (Evanson et al., 2014). BK channels are activated by the simultaneous release of SR Ca2+ via RyRs in close apposition to VSM plasmalemma BK channels (Nelson et al., 1995) (Figure 3). Functional coupling between BK channels and RyR has been extensively characterized in VSM as a critical mechanism regulating arterial diameter (Nelson et al., 1995; Perez et al., 1999; Fan et al., 2019). Moreover, studies in mice mesenteric and human cerebral VSM suggest that BK channels are part of a Ca2+ signaling microdomain that includes caveolae, T-type CaV3.2, and RyRs (Harraz et al., 2014). Caveolae facilitates the coupling of CaV3.2 channel activity with RyR activation (Hashad et al., 2018), and BK channels within the signaling domain are activated by the Ca2+ sparks (Figure 3). As described above, BK channels are also suggested to be in a signaling complex with TRPV4 and RyRs, which promote BK channel activation in response to changes in the intraluminal pressure (Earley et al., 2005).
Conclusion
Small resistance arteries respond to different stimuli by adjusting their diameter to meet the tissue perfusion needs. The adjustments in the arterial diameter of small resistance arteries and arterioles are largely determined by the contractile state of VSM lining the walls of arteries (Knot and Nelson, 1995; Knot and Nelson, 1998; Cole et al., 2005). VSM contractility is dependent on the interplay of different ionic conductance that controls membrane potential and the level of intracellular [Ca2+]i. Different stimuli within the body engage signaling mechanisms that regulate these ion channels and, in turn, modulate vascular function. Here, we have provided an overview of our current knowledge of specific molecular complexes involving VGCC, TRP, and KV and BK channels emphasizing well-characterized signaling complexes contributing to the regulation of VSM excitability. The formation of macromolecular complexes could provide a way for ion channels to respond differently to specific stimuli by bringing signaling generators and effectors within proximity to discrete regions of the cell. Determining whether similar complexes exist in different vascular beds and their contribution to VSM contractility in health and disease is an area of opportunity in the vascular field. Understanding the specific mechanism that governs the formation of these molecular complexes will provide novel strategies for developing therapeutics with enhanced specificity in the fight against cardiovascular disease.
Author contributions
Each author wrote a section of the manuscript. ES gathered contributions and generated figures. MC integrated all the contributions and provided overall supervision and direction to the project. All authors revised and approved the final version.
Funding
This work was supported by American Heart Association grants 852984 (MC.), 830626 (MB), and R01HL149127 and 161872 (MN).
Conflict of interest
The authors declare that the research was conducted in the absence of any commercial or financial relationships that could be construed as a potential conflict of interest.
Publisher’s note
All claims expressed in this article are solely those of the authors and do not necessarily represent those of their affiliated organizations, or those of the publisher, the editors and the reviewers. Any product that may be evaluated in this article, or claim that may be made by its manufacturer, is not guaranteed or endorsed by the publisher.
References
Abd El-Rahman R. R., Harraz O. F., Brett S. E., Anfinogenova Y., Mufti R. E., Goldman D., et al. (2013). Identification of L- and T-type Ca2+ channels in rat cerebral arteries: Role in myogenic tone development. Am. J. Physiol. Heart Circ. Physiol. 304 (1), H58–H71. doi:10.1152/ajpheart.00476.2012
Adebiyi A., Narayanan D., Jaggar J. H. (2011). Caveolin-1 assembles type 1 inositol 1, 4, 5-trisphosphate receptors and canonical transient receptor potential 3 channels into a functional signaling complex in arterial smooth muscle cells. J. Biol. Chem. 286 (6), 4341–4348. doi:10.1074/jbc.M110.179747
Aiello E. A., Clement-Chomienne O., Sontag D. P., Walsh M. P., Cole W. C. (1996). Protein kinase C inhibits delayed rectifier K+ current in rabbit vascular smooth muscle cells. Am. J. Physiol. 271 (12), H109–H119. doi:10.1152/ajpheart.1996.271.1.H109
Aiello E. A., Walsh M. P., Cole W. C. (1995). Phosphorylation by protein kinase A enhances delayed rectifier K+ current in rabbit vascular smooth muscle cells. Am. J. Physiol. 268 (22), H926–H934. doi:10.1152/ajpheart.1995.268.2.H926
Albarwani S., Nemetz L. T., Madden J. A., Tobin A. A., England S. K., Pratt P. F., et al. (2003). Voltage-gated K+ channels in rat small cerebral arteries: Molecular identity of the functional channels. J. Physiol. 551, 751–763. doi:10.1113/jphysiol.2003.040014
Ali S., Solano A. S., Gonzales A. L., Thakore P., Krishnan V., Yamasaki E., et al. (2021). Nitric oxide signals through IRAG to inhibit TRPM4 channels and dilate cerebral arteries. Function 2 (6), zqab051. doi:10.1093/function/zqab051
Amberg G. C., Koh S. D., Imaizumi Y., Ohya S., Sanders K. M. (2003). A-type potassium currents in smooth muscle. Am. J. Physiol. Cell Physiol. 284 (3), C583–C595. doi:10.1152/ajpcell.00301.2002
Amberg G. C., Navedo M. F., Nieves-Cintrón M., Molkentin J. D., Santana L. F. (2007). Calcium sparklets regulate local and global calcium in murine arterial smooth muscle. J. Physiol. 579 (1), 187–201. doi:10.1113/jphysiol.2006.124420
Amberg G. C., Santana L. F. (2006). Kv2 channels oppose myogenic constriction of rat cerebral arteries. Am. J. Physiol. Cell Physiol. 291 (2), C348–C356. doi:10.1152/ajpcell.00086.2006
Arikkath J., Campbell K. P. (2003). Auxiliary subunits: Essential components of the voltage-gated calcium channel complex. Curr. Opin. Neurobiol. 13 (3), 298–307. doi:10.1016/s0959-4388(03)00066-7
Bannister J. P., Adebiyi A., Zhao G., Narayanan D., Thomas C. M., Feng J. Y., et al. (2009). Smooth muscle cell alpha2delta-1 subunits are essential for vasoregulation by CaV1.2 channels. Circ. Res. 105 (10), 948–955. doi:10.1161/CIRCRESAHA.109.203620
Bannister J. P., Bulley S., Narayanan D., Thomas-Gatewood C., Luzny P., Pachuau J., et al. (2012). Transcriptional upregulation of α2δ-1 elevates arterial smooth muscle cell voltage-dependent Ca2+ channel surface expression and cerebrovascular constriction in genetic hypertension. Hypertension 60 (4), 1006–1015. doi:10.1161/HYPERTENSIONAHA.112.199661
Berwick Z. C., Payne G. A., Lynch B., Dick G. M., Sturek M., Tune J. D. (2010). Contribution of adenosine A(2A) and A(2B) receptors to ischemic coronary dilation: Role of K(V) and K(ATP) channels. Microcirculation 17 (8), 600–607. doi:10.1111/j.1549-8719.2010.00054.x
Brayden J. E., Earley S., Nelson M. T., Reading S. (2008). Transient receptor potential (TRP) channels, vascular tone and autoregulation of cerebral blood flow. Clin. Exp. Pharmacol. Physiol. 35 (9), 1116–1120. doi:10.1111/j.1440-1681.2007.04855.x
Brenner R., Perez G. J., Bonev A. D., Eckman D. M., Kosek J. C., Wiler S. W., et al. (2000). Vasoregulation by the beta1 subunit of the calcium-activated potassium channel. Nature 407 (6806), 870–876. doi:10.1038/35038011
Carvajal J. A., Germain A. M., Huidobro-Toro J. P., Weiner C. P. (2000). Molecular mechanism of cGMP-mediated smooth muscle relaxation. J. Cell. Physiol. 184 (3), 409–420. doi:10.1002/1097-4652(200009)184:3<409:AID-JCP16>3.0.CO;2-K
Catterall W. A. (2000). Structure and regulation of voltage-gated Ca2+ channels. Annu. Rev. Cell Dev. Biol. 16, 521–555. doi:10.1146/annurev.cellbio.16.1.521
Catterall W. A. (2011). Voltage-gated calcium channels. Cold Spring Harb. Perspect. Biol. 3 (8), a003947. doi:10.1101/cshperspect.a003947
Chen Y. L., Daneva Z., Kuppusamy M., Ottolini M., Baker T. M., Klimentova E., et al. (2022). Novel smooth muscle Ca(2+)-signaling nanodomains in blood pressure regulation. Circulation, 101161CIRCULATIONAHA121058607. Epub ahead of print. doi:10.1161/CIRCULATIONAHA.121.05860
Cheong A., Dedman A. M., Xu S. Z., Beech D. J. (2001). K(V)alpha1 channels in murine arterioles: Differential cellular expression and regulation of diameter. Am. J. Physiol. Heart Circ. Physiol. 281 (3), H1057–H1065. doi:10.1152/ajpheart.2001.281.3.H1057
Choe S., Kreusch A., Pfaffinger P. J. (1999). Towards the three-dimensional structure of voltage-gated potassium channels. Trends biochem. Sci. 24 (9), 345–349. doi:10.1016/s0968-0004(99)01440-1
Clement-Chomienne O., Walsh M. P., Cole W. C. (1996). Angiotensin II activation of protein kinase C decreases delayed rectifier K+ current in rabbit vascular myocytes. J. Physiol. 495 (3), 689–700. doi:10.1113/jphysiol.1996.sp021626
Cole W. C., Chen T. T., Clement-Chomienne O. (2005). Myogenic regulation of arterial diameter: Role of potassium channels with a focus on delayed rectifier potassium current. Can. J. Physiol. Pharmacol. 83 (8-9), 755–765. doi:10.1139/y05-082
Cole W. C., Clement-Chomienne O., Aiello E. A. (1996). Regulation of 4-aminopyridine-sensitive, delayed rectifier K+ channels in vascular smooth muscle by phosphorylation. Biochem. Cell Biol. 74 (4), 439–447. doi:10.1139/o96-048
Colledge M., Scott J. D. (1999). AKAPs: From structure to function. Trends Cell Biol. 9 (6), 216–221. doi:10.1016/s0962-8924(99)01558-5
Cribbs L. L. (2006). T-Type Ca2+ channels in vascular smooth muscle: Multiple functions. Cell Calcium 40 (2), 221–230. doi:10.1016/j.ceca.2006.04.026
De Jongh K. S., Warner C., Catterall W. A. (1990). Subunits of purified calcium channels. Alpha 2 and delta are encoded by the same gene. J. Biol. Chem. 265 (25), 14738–14741. doi:10.1016/s0021-9258(18)77174-3
Dolphin A. C. (2003). Beta subunits of voltage-gated calcium channels. J. Bioenerg. Biomembr. 35 (6), 599–620. doi:10.1023/b:jobb.0000008026.37790.5a
Dolphin A. C. (2016). Voltage-gated calcium channels and their auxiliary subunits: Physiology and pathophysiology and pharmacology. J. Physiol. 594 (19), 5369–5390. doi:10.1113/JP272262
Earley S., Brayden J. E. (2015). Transient receptor potential channels in the vasculature. Physiol. Rev. 95 (2), 645–690. doi:10.1152/physrev.00026.2014
Earley S., Heppner T. J., Nelson M. T., Brayden J. E. (2005). TRPV4 forms a novel Ca2+ signaling complex with ryanodine receptors and BKCa channels. Circ. Res. 97 (12), 1270–1279. doi:10.1161/01.RES.0000194321.60300.d6
Evanson K., Bannister J. P., Leo M. D., Jaggar J. H. (2014). LRRC26 is a functional BK channel auxiliary gamma subunit in arterial smooth muscle cells. Circ. Res. 115, 423–431. doi:10.1161/CIRCRESAHA.115.303407
Fan G., Cui Y., Gollasch M., Kassmann M. (2019). Elementary calcium signaling in arterial smooth muscle. Channels (Austin) 13 (1), 505–519. doi:10.1080/19336950.2019.1688910
Fernandez J. A., McGahon M. K., McGeown J. G., Curtis T. M. (2015). CaV3.1 T-Type Ca2+ channels contribute to myogenic signaling in rat retinal arterioles. Invest. Ophthalmol. Vis. Sci. 56 (9), 5125–5132. doi:10.1167/iovs.15-17299
Gao T., Chien A. J., Hosey M. M. (1999). Complexes of the alpha1C and beta subunits generate the necessary signal for membrane targeting of class C L-type calcium channels. J. Biol. Chem. 274 (4), 2137–2144. doi:10.1074/jbc.274.4.2137
Geiselhoringer A., Werner M., Sigl K., Smital P., Worner R., Acheo L., et al. (2004). IRAG is essential for relaxation of receptor-triggered smooth muscle contraction by cGMP kinase. EMBO J. 23 (21), 4222–4231. doi:10.1038/sj.emboj.7600440
Ghosh D., Syed A. U., Prada M. P., Nystoriak M. A., Santana L. F., Nieves-Cintron M., et al. (2017). Calcium channels in vascular smooth muscle. Adv. Pharmacol. 78, 49–87. doi:10.1016/bs.apha.2016.08.002
Gonzales A. L., Amberg G. C., Earley S. (2010). Ca2+ release from the sarcoplasmic reticulum is required for sustained TRPM4 activity in cerebral artery smooth muscle cells. Am. J. Physiol. Cell Physiol. 299 (2), C279–C288. doi:10.1152/ajpcell.00550.2009
Gonzales A. L., Yang Y., Sullivan M. N., Sanders L., Dabertrand F., Hill-Eubanks D. C., et al. (2014). A PLCγ1-dependent, force-sensitive signaling network in the myogenic constriction of cerebral arteries. Sci. Signal. 7 (327), ra49. doi:10.1126/scisignal.2004732
Gurnett C. A., De Waard M., Campbell K. P. (1996). Dual function of the voltage-dependent Ca2+ channel alpha 2 delta subunit in current stimulation and subunit interaction. Neuron 16 (2), 431–440. doi:10.1016/s0896-6273(00)80061-6
Gustafsson F., Andreasen D., Salomonsson M., Jensen B. L., Holstein-Rathlou N. (2001). Conducted vasoconstriction in rat mesenteric arterioles: Role for dihydropyridine-insensitive Ca(2+) channels. Am. J. Physiol. Heart Circ. Physiol. 280 (2), H582–H590. doi:10.1152/ajpheart.2001.280.2.H582
Gutman G. A., Chandy K. G., Grissmer S., Lazdunski M., McKinnon D., Pardo L. A., et al. (2005). International Union of Pharmacology. LIII. Nomenclature and molecular relationships of voltage-gated potassium channels. Pharmacol. Rev. 57 (4), 473–508. doi:10.1124/pr.57.4.10
Harraz O. F., Abd El-Rahman R. R., Bigdely-Shamloo K., Wilson S. M., Brett S. E., Romero M., et al. (2014). Ca(V)3.2 channels and the induction of negative feedback in cerebral arteries. Circ. Res. 115 (7), 650–661. doi:10.1161/CIRCRESAHA.114.304056
Harraz O. F., Brett S. E., Zechariah A., Romero M., Puglisi J. L., Wilson S. M., et al. (2015). Genetic ablation of CaV3.2 channels enhances the arterial myogenic response by modulating the RyR-BKCa axis. Arterioscler. Thromb. Vasc. Biol. 35 (8), 1843–1851. doi:10.1161/ATVBAHA.115.305736
Harraz O. F., Visser F., Brett S. E., Goldman D., Zechariah A., Hashad A. M., et al. (2015). CaV1.2/CaV3.x channels mediate divergent vasomotor responses in human cerebral arteries. J. Gen. Physiol. 145 (5), 405–418. doi:10.1085/jgp.201511361
Hashad A. M., Harraz O. F., Brett S. E., Romero M., Kassmann M., Puglisi J. L., et al. (2018). Caveolae link CaV3.2 channels to BKCa-mediated feedback in vascular smooth muscle. Arterioscler. Thromb. Vasc. Biol. 38 (10), 2371–2381. doi:10.1161/ATVBAHA.118.311394
Hayabuchi Y., Standen N. B., Davies N. W. (2001). Angiotensin II inhibits and alters kinetics of voltage-gated K(+) channels of rat arterial smooth muscle. Am. J. Physiol. Heart Circ. Physiol. 281 (6), H2480–H2489. doi:10.1152/ajpheart.2001.281.6.H2480
Helliwell R. M., Large W. A. (1997). Alpha 1-adrenoceptor activation of a non-selective cation current in rabbit portal vein by 1, 2-diacyl-sn-glycerol. J. Physiol. 499 (2), 417–428. doi:10.1113/jphysiol.1997.sp021938
Jaggar J. H., Wellman G. C., Heppner T. J., Porter V. A., Perez G. J., Gollasch M., et al. (1998). Ca2+ channels, ryanodine receptors and Ca2+-activated K+ channels: A functional unit for regulating arterial tone. Acta Physiol. Scand. 164 (4), 577–587. doi:10.1046/j.1365-201X.1998.00462.x
Joseph B. K., Thakali K. M., Pathan A. R., Kang E., Rusch N. J., Rhee S. W. (2011). Postsynaptic density-95 scaffolding of Shaker-type K⁺ channels in smooth muscle cells regulates the diameter of cerebral arteries. J. Physiol. 589 (21), 5143–5152. doi:10.1113/jphysiol.2011.213843
Kerr P. M., Clement-Chomienne O., Thorneloe K. S., Chen T. T., Ishii K., Sontag D. P., et al. (2001). Heteromultimeric Kv1.2-Kv1.5 channels underlie 4-aminopyridine-sensitive delayed rectifier K+ current of rabbit vascular myocytes. Circ. Res. 89 (11), 1038–1044. doi:10.1161/hh2301.100803
Kharade S. V., Sonkusare S. K., Srivastava A. K., Thakali K. M., Fletcher T. W., Rhee S. W., et al. (2013). The β3 subunit contributes to vascular calcium channel upregulation and hypertension in angiotensin II-infused C57BL/6 mice. Hypertension 61 (1), 137–142. doi:10.1161/HYPERTENSIONAHA.112.197863
Kilfoil P. J., Tipparaju S. M., Barski O. A., Bhatnagar A. (2013). Regulation of ion channels by pyridine nucleotides. Circ. Res. 112 (4), 721–741. doi:10.1161/CIRCRESAHA.111.247940
Klugbauer N., Lacinova L., Marais E., Hobom M., Hofmann F. (1999). Molecular diversity of the calcium channel α2δ subunit. J. Neurosci. 19 (2), 684–691. doi:10.1523/jneurosci.19-02-00684.1999
Knot H. J., Nelson M. T. (1998). Regulation of arterial diameter and wall [Ca2+] in cerebral arteries of rat by membrane potential and intravascular pressure. J. Physiol. 508 (1), 199–209. doi:10.1111/j.1469-7793.1998.199br.x
Knot H. J., Nelson M. T. (1995). Regulation of membrane potential and diameter by voltage-dependent K+ channels in rabbit myogenic cerebral arteries. Am. J. Physiol. 269 (12), H348–H355. doi:10.1152/ajpheart.1995.269.1.H348
Knot H. J., Standen N. B., Nelson M. T. (1998). Ryanodine receptors regulate arterial diameter and wall [Ca2+] in cerebral arteries of rat via Ca2+-dependent K+ channels. J. Physiol. 508 (1), 211–221. doi:10.1111/j.1469-7793.1998.211br.x
Ko E. A., Han J., Jung I. D., Park W. S. (2008). Physiological roles of K+ channels in vascular smooth muscle cells. J. Smooth Muscle Res. 44 (2), 65–81. doi:10.1540/jsmr.44.65
Ko E. A., Park W. S., Firth A. L., Kim N., Yuan J. X., Han J. (2010). Pathophysiology of voltage-gated K+ channels in vascular smooth muscle cells: Modulation by protein kinases. Prog. Biophys. Mol. Biol. 103 (1), 95–101. doi:10.1016/j.pbiomolbio.2009.10.001
Kudryavtseva O., Aalkjaer C., Matchkov V. V. (2013). Vascular smooth muscle cell phenotype is defined by Ca2+-dependent transcription factors. FEBS J. 280 (21), 5488–5499. doi:10.1111/febs.12414
Langeberg L. K., Scott J. D. (2005). A-kinase-anchoring proteins. J. Cell Sci. 118 (15), 3217–3220. doi:10.1242/jcs.02416
Latorre R., Castillo K., Carrasquel-Ursulaez W., Sepulveda R. V., Gonzalez-Nilo F., Gonzalez C., et al. (2017). Molecular determinants of BK channel functional diversity and functioning. Physiol. Rev. 97 (1), 39–87. doi:10.1152/physrev.00001.2016
Li Q., Yan J. (2016). Modulation of BK channel function by auxiliary beta and gamma subunits. Int. Rev. Neurobiol. 128, 51–90. doi:10.1016/bs.irn.2016.03.015
Liao M., Cao E., Julius D., Cheng Y. (2013). Structure of the TRPV1 ion channel determined by electron cryo-microscopy. Nature 504 (7478), 107–112. doi:10.1038/nature12822
Lincoln T. M., Dey N., Sellak H. (1985). Invited review: cGMP-dependent protein kinase signaling mechanisms in smooth muscle: From the regulation of tone to gene expression. J. Appl. Physiol. 91 (3), 1421–1430. doi:10.1152/jappl.2001.91.3.1421
M Nieves-Cintron S. T., Santana L. F., Navedo M. F. (2018). Methods in signal transduction: Vascular smooth muscle. 1st Edition ed. Boca Raton, FL: CRC Press, 87–103. Total internal reflection fluorescent microscopy in vascular smooth muscle
Mercado J., Baylie R., Navedo M. F., Yuan C., Scott J. D., Nelson M. T., et al. (2014). Local control of TRPV4 channels by AKAP150-targeted PKC in arterial smooth muscle. J. Gen. Physiol. 143 (5), 559–575. doi:10.1085/jgp.201311050
Moore C. L., McClenahan S. J., Hanvey H. M., Jang D. S., Nelson P. L., Joseph B. K., et al. (2015). Beta1-adrenergic receptor-mediated dilation of rat cerebral artery requires Shaker-type KV1 channels on PSD95 scaffold. J. Cereb. Blood Flow. Metab. 35 (9), 1537–1546. doi:10.1038/jcbfm.2015.91
Moore C. L., Nelson P. L., Parelkar N. K., Rusch N. J., Rhee S. W. (2014). Protein kinase A-phosphorylated KV1 channels in PSD95 signaling complex contribute to the resting membrane potential and diameter of cerebral arteries. Circ. Res. 114 (8), 1258–1267. doi:10.1161/CIRCRESAHA.114.303167
Moosmang S., Schulla V., Welling A., Feil R., Feil S., Wegener J. W., et al. (2003). Dominant role of smooth muscle L-type calcium channel Cav1.2 for blood pressure regulation. Embo J. 22 (22), 6027–6034. doi:10.1093/emboj/cdg583
Moreno-Dominguez A., Cidad P., Miguel-Velado E., Lopez-Lopez J. R., Perez-Garcia M. T. (2009). De novo expression of Kv6.3 contributes to changes in vascular smooth muscle cell excitability in a hypertensive mice strain. J. Physiol. 587 (3), 625–640. doi:10.1113/jphysiol.2008.165217
Murakami M., Yamamura H., Murakami A., Okamura T., Nunoki K., Mitui-Saito M., et al. (2000). Conserved smooth muscle contractility and blood pressure increase in response to high-salt diet in mice lacking the beta3 subunit of the voltage-dependent calcium channel. J. Cardiovasc. Pharmacol. 36 (2), S69–S73. doi:10.1097/00005344-200000006-00015
Navedo M. F., Amberg G., Votaw S. V., Santana L. F. (2005). Constitutively active L-type Ca2+ channels. Proc. Natl. Acad. Sci. U. S. A. 102 (31), 11112–11117. doi:10.1073/pnas.0500360102
Navedo M. F., Amberg G. C., Nieves M., Molkentin J. D., Santana L. F. (2006). Mechanisms underlying heterogeneous Ca2+ sparklet activity in arterial smooth muscle. J. Gen. Physiol. 127 (6), 611–622. doi:10.1085/jgp.200609519
Navedo M. F., Cheng E. P., Yuan C., Votaw S., Molkentin J. D., Scott J. D., et al. (2010). Increased coupled gating of L-type Ca2+ channels during hypertension and Timothy syndrome. Circ. Res. 106 (4), 748–756. doi:10.1161/CIRCRESAHA.109.213363
Navedo M. F., Nieves-Cintron M., Amberg G. C., Yuan C., Votaw V. S., Lederer W. J., et al. (2008). AKAP150 is required for stuttering persistent Ca2+ sparklets and angiotensin II-induced hypertension. Circ. Res. 102 (2), e1–e11. doi:10.1161/CIRCRESAHA.107.167809
Navedo M. F., Takeda Y., Nieves-Cintron M., Molkentin J. D., Santana L. F. (2010). Elevated Ca2+ sparklet activity during acute hyperglycemia and diabetes in cerebral arterial smooth muscle cells. Am. J. Physiol. Cell Physiol. 298 (2), C211–C220. doi:10.1152/ajpcell.00267.2009
Nelson M. T., Cheng H., Rubart M., Santana L. F., Bonev A. D., Knot H. J., et al. (1995). Relaxation of arterial smooth muscle by calcium sparks. Science 270 (5236), 633–637. doi:10.1126/science.270.5236.633
Nelson M. T. (1992). Regulation of arterial tone by potassium channels. Jpn. J. Pharmacol. 58 (2), 238P–42P. doi:10.1016/s0021-5198(19)59920-6
Nieves-Cintron M., Amberg G. C., Navedo M. F., Molkentin J. D., Santana L. F. (2008). The control of Ca2+ influx and NFATc3 signaling in arterial smooth muscle during hypertension. Proc. Natl. Acad. Sci. U. S. A. 105 (40), 15623–15628. doi:10.1073/pnas.0808759105
Nieves-Cintron M., Flores-Tamez V. A., Le T., Baudel M. M., Navedo M. F. (2021). Cellular and molecular effects of hyperglycemia on ion channels in vascular smooth muscle. Cell. Mol. Life Sci. 78 (1), 31–61. doi:10.1007/s00018-020-03582-z
Nikitina E., Zhang Z. D., Kawashima A., Jahromi B. S., Bouryi V. A., Takahashi M., et al. (2007). Voltage-dependent calcium channels of dog basilar artery. J. Physiol. 580 (2), 523–541. doi:10.1113/jphysiol.2006.126128
Nilius B., Prenen J., Tang J., Wang C., Owsianik G., Janssens A., et al. (2005). Regulation of the Ca2+ sensitivity of the nonselective cation channel TRPM4. J. Biol. Chem. 280 (8), 6423–6433. doi:10.1074/jbc.M411089200
Nystoriak M. A., Navedo M. F. (2017). Regulation of microvascular function by voltage-gated potassium channels: New tricks for an "ancient" dog. Microcirculation 25, 12345. doi:10.1111/micc.12435
Nystoriak M. A., Nieves-Cintron M., Nygren P. J., Hinke S. A., Nichols C. B., Chen C. Y., et al. (2014). AKAP150 contributes to enhanced vascular tone by facilitating large-conductance Ca2+-activated K+ channel remodeling in hyperglycemia and diabetes mellitus. Circ. Res. 114 (4), 607–615. doi:10.1161/CIRCRESAHA.114.302168
Nystoriak M. A., Nieves-Cintron M., Patriarchi T., Buonarati O. R., Prada M. P., Morotti S., et al. (2017). Ser1928 phosphorylation by PKA stimulates the L-type Ca2+ channel CaV1.2 and vasoconstriction during acute hyperglycemia and diabetes. Sci. Signal. 10 (463), eaaf9647. doi:10.1126/scisignal.aaf9647
Nystoriak M. A., Zhang D., Jagatheesan G., Bhatnagar A. (2017). Heteromeric complexes of aldo-keto reductase auxiliary KVβ subunits (AKR6A) regulate sarcolemmal localization of KV1.5 in coronary arterial myocytes. Chem. Biol. Interact. 276, 210–217. doi:10.1016/j.cbi.2017.03.011
Perez G. J., Bonev A. D., Patlak J. B., Nelson M. T. (1999). Functional coupling of ryanodine receptors to KCa channels in smooth muscle cells from rat cerebral arteries. J. Gen. Physiol. 113 (2), 229–238. doi:10.1085/jgp.113.2.229
Perez-Reyes E. (2006). Molecular characterization of T-type calcium channels. Cell Calcium 40 (2), 89–96. doi:10.1016/j.ceca.2006.04.012
Pesic A., Madden J. A., Pesic M., Rusch N. J. (2004). High blood pressure upregulates arterial L-type Ca2+ channels. Is membrane depolarization the signal? Circ. Res. 94, e97–104. doi:10.1161/01.RES.0000131495.93500.3c
Plane F., Johnson R., Kerr P., Wiehler W., Thorneloe K., Ishii K., et al. (2005). Heteromultimeric Kv1 channels contribute to myogenic control of arterial diameter. Circ. Res. 96 (2), 216–224. doi:10.1161/01.RES.0000154070.06421.25
Prada M. P., Syed A. U., Buonarati O. R., Reddy G. R., Nystoriak M. A., Ghosh D., et al. (2019). A Gs-coupled purinergic receptor boosts Ca2+ influx and vascular contractility during diabetic hyperglycemia. eLife 8, e42214. doi:10.7554/eLife.42214
Prada M. P., Syed A. U., Reddy G. R., Martin-Aragon Baudel M., Flores-Tamez V. A., Sasse K. C., et al. (2020). AKAP5 complex facilitates purinergic modulation of vascular L-type Ca(2+) channel CaV1.2. Nat. Commun. 11 (1), 5303. doi:10.1038/s41467-020-18947-y
Pratt P. F., Bonnet S., Ludwig L. M., Bonnet P., Rusch N. J. (2002). Upregulation of L-type Ca2+ channels in mesenteric and skeletal arteries of SHR. Hypertension 40 (2), 214–219. doi:10.1161/01.hyp.0000025877.23309.36
Reading S. A., Brayden J. E. (2007). Central role of TRPM4 channels in cerebral blood flow regulation. Stroke 38 (8), 2322–2328. doi:10.1161/STROKEAHA.107.483404
Reading S. A., Earley S., Waldron B. J., Welsh D. G., Brayden J. E. (2005). TRPC3 mediates pyrimidine receptor-induced depolarization of cerebral arteries. Am. J. Physiol. Heart Circ. Physiol. 288 (5), H2055–H2061. doi:10.1152/ajpheart.00861.2004
Rhee S. W., Rusch N. J. (2018). Molecular determinants of beta-adrenergic signaling to voltage-gated K(+) channels in the cerebral circulation. Microcirculation 25 (1), e12425. doi:10.1111/micc.12425
Saleh S. N., Albert A. P., Peppiatt C. M., Large W. A. (2006). Angiotensin II activates two cation conductances with distinct TRPC1 and TRPC6 channel properties in rabbit mesenteric artery myocytes. J. Physiol. 577 (2), 479–495. doi:10.1113/jphysiol.2006.119305
Satake N., Shibata M., Shibata S. (1996). The inhibitory effects of iberiotoxin and 4-aminopyridine on the relaxation induced by beta 1- and beta 2-adrenoceptor activation in rat aortic rings. Br. J. Pharmacol. 119 (3), 505–510. doi:10.1111/j.1476-5381.1996.tb15700.x
Schlossmann J., Desch M. (2011). IRAG and novel PKG targeting in the cardiovascular system. Am. J. Physiol. Heart Circ. Physiol. 301 (3), H672–H682. doi:10.1152/ajpheart.00198.2011
Singer D., Biel M., Lotan I., Flockerzi V., Hofmann F., Dascal N. (1991). The roles of the subunits in the function of the calcium channel. Science 253 (5027), 1553–1557. doi:10.1126/science.1716787
Sonkusare S., Palade P. T., Marsh J. D., Telemaque S., Pesic A., Rusch N. J. (2006). Vascular calcium channels and high blood pressure: Pathophysiology and therapeutic implications. Vasc. Pharmacol. 44 (3), 131–142. doi:10.1016/j.vph.2005.10.005
Sung D. J., Noh H. J., Kim J. G., Park S. W., Kim B., Cho H., et al. (2013). Serotonin contracts the rat mesenteric artery by inhibiting 4-aminopyridine-sensitive Kv channels via the 5-HT2A receptor and Src tyrosine kinase. Exp. Mol. Med. 45, e67. doi:10.1038/emm.2013.116
Syed A. U., Reddy G. R., Ghosh D., Prada M. P., Nystoriak M. A., Morotti S., et al. (2019). Adenylyl cyclase 5–generated cAMP controls cerebral vascular reactivity during diabetic hyperglycemia. J. Clin. Invest. 129 (8), 3140–3152. doi:10.1172/JCI124705
Tajada S., Cidad P., Colinas O., Santana L. F., Lopez-Lopez J. R., Perez-Garcia M. T. (2013). Down-regulation of CaV1.2 channels during hypertension: How fewer CaV1.2 channels allow more Ca(2+) into hypertensive arterial smooth muscle. J. Physiol. 591 (24), 6175–6191. doi:10.1113/jphysiol.2013.265751
Tajada S., Moreno C. M., O'Dwyer S., Woods S., Sato D., Navedo M. F., et al. (2017). Distance constraints on activation of TRPV4 channels by AKAP150-bound PKCα in arterial myocytes. J. Gen. Physiol. 149 (6), 639–659. doi:10.1085/jgp.201611709
Tykocki N. R., Boerman E. M., Jackson W. F. (2017). Smooth muscle ion channels and regulation of vascular tone in resistance arteries and arterioles. Compr. Physiol. 7 (2), 485–581. doi:10.1002/cphy.c160011
VanBavel E., Sorop O., Andreasen D., Pfaffendorf M., Jensen B. L. (2002). Role of T-type calcium channels in myogenic tone of skeletal muscle resistance arteries. Am. J. Physiol. Heart Circ. Physiol. 283 (6), H2239–H2243. doi:10.1152/ajpheart.00531.2002
Wamhoff B. R., Bowles D. K., McDonald O. G., Sinha S., Somlyo A. P., Somlyo A. V., et al. (2004). L-type voltage-gated Ca2+ channels modulate expression of smooth muscle differentiation marker genes via a rho kinase/myocardin/SRF-dependent mechanism. Circ. Res. 95 (4), 406–414. doi:10.1161/01.RES.0000138582.36921.9e
Wamhoff B. R., Bowles D. K., Owens G. K. (2006). Excitation-transcription coupling in arterial smooth muscle. Circ. Res. 98 (7), 868–878. doi:10.1161/01.RES.0000216596.73005.3c
Welsh D. G., Morielli A. D., Nelson M. T., Brayden J. E. (2002). Transient receptor potential channels regulate myogenic tone of resistance arteries. Circ. Res. 90 (3), 248–250. doi:10.1161/hh0302.105662
Won S., Levy J. M., Nicoll R. A., Roche K. W. (2017). MAGUKs: Multifaceted synaptic organizers. Curr. Opin. Neurobiol. 43, 94–101. doi:10.1016/j.conb.2017.01.006
Xi Q., Adebiyi A., Zhao G., Chapman K. E., Waters C. M., Hassid A., et al. (2008). IP3 constricts cerebral arteries via IP3 receptor-mediated TRPC3 channel activation and independently of sarcoplasmic reticulum Ca2+ release. Circ. Res. 102 (9), 1118–1126. doi:10.1161/CIRCRESAHA.108.173948
Zhong X. Z., Abd-Elrahman K. S., Liao C. H., El-Yazbi A. F., Walsh E. J., Walsh M. P., et al. (2010). Stromatoxin-sensitive, heteromultimeric Kv2.1/Kv9.3 channels contribute to myogenic control of cerebral arterial diameter. J. Physiol. 588 (22), 4519–4537. doi:10.1113/jphysiol.2010.196618
Keywords: l-type calcium channel, TRP channels, potassium channels, blood pressure, resistance arteries
Citation: Pereira da Silva EA, Martín-Aragón Baudel M, Navedo MF and Nieves-Cintrón M (2022) Ion channel molecular complexes in vascular smooth muscle. Front. Physiol. 13:999369. doi: 10.3389/fphys.2022.999369
Received: 21 July 2022; Accepted: 02 August 2022;
Published: 26 August 2022.
Edited by:
Kevin J. Sampson, Columbia University, United StatesReviewed by:
Anna Bukiya, University of Tennessee Health Science Center (UTHSC), United StatesM. Teresa Perez-Garcia, University of Valladolid, Spain
Jonathan H. Jaggar, University of Tennessee Health Science Center (UTHSC), United States
Copyright © 2022 Pereira da Silva, Martín-Aragón Baudel, Navedo and Nieves-Cintrón. This is an open-access article distributed under the terms of the Creative Commons Attribution License (CC BY). The use, distribution or reproduction in other forums is permitted, provided the original author(s) and the copyright owner(s) are credited and that the original publication in this journal is cited, in accordance with accepted academic practice. No use, distribution or reproduction is permitted which does not comply with these terms.
*Correspondence: Madeline Nieves-Cintrón, bWNuaWV2ZXNAdWNkYXZpcy5lZHU=