- 1State Key Laboratory for Biology of Plant Diseases and Insect Pests, Institute of Plant Protection, Chinese Academy of Agricultural Sciences, Beijing, China
- 2College of Agronomy, Jiangxi Agricultural University, Nanchang, China
Acclimation to abiotic stress plays a critical role in insect adaption and evolution, particularly during extreme climate events. Heat shock proteins (HSPs) are evolutionarily conserved molecular chaperones caused by abiotic and biotic stressors. Understanding the relationship between thermal acclimation and the expression of specific HSPs is essential for addressing the functions of HSP families. This study investigated this issue using the Asian corn borer Ostrinia furnacalis, one of the most important corn pests in China. The transcription of HSP genes was induced in larvae exposed to 33°C. Thereafter, the larvae were exposed to 43°C, for 2 h, and then allowed to recover at 27 C for 0, 0.5, 1, 2, 4, 6, and 8 h. At the recovery times 0.5–4 h, most population tolerates less around 1–3 h than without recovery (at 0 h) suffering continuous heat stress (43 C). There is no difference in the heat tolerance at 6 h recovery, with similar transcriptional levels of HSPs as the control. However, a significant thermal tolerance was observed after 8 h of the recovery time, with a higher level of HSP70. In addition, the transcription of HSP60 and HSC70 (heat shock cognate protein 70) genes did not show a significant effect. HSP70 or HSP90 significantly upregulated within 1–2 h sustained heat stress (43 C) but declined at 6 h. Our findings revealed extreme thermal stress induced quick onset of HSP70 or HSP90 transcription. It could be interpreted as an adaptation to the drastic and rapid temperature variation. The thermal tolerance of larvae is significantly enhanced after 6 h of recovery and possibly regulated by HSP70.
Introduction
Ongoing global climate change has caused a substantial increase in the occurrence of extreme thermal events (Meehl and Tebaldi, 2004; Alexander et al., 2006; IPCC, 2013; Ma et al., 2021). The phenotypic plasticity of ectotherms is a nongenetic strategic response to environmental variation, including thermal extremes (Hoffmann et al., 2013; Gunderson and Stillman, 2015; van Heerwaarden et al., 2016). Insects, as ectotherms, exhibit plasticity in a suite of traits related to thermal extremes, have fast generation times, and thus are considered good models to study plasticity (Sgrò et al., 2016; Gibert et al., 2019). Understanding plasticity has taken on increased importance in the context of rapid climate change as extreme events are more frequent and show increased variability (Fox et al., 2019). For example, insects living at higher latitudes have evolved plastic responses to survive in cold winters, but extreme events are increasingly disrupting the reliability of environmental signals (Sgrò et al., 2016; Gibert et al., 2019). Thermal extremes can lead to heat injury, as well as to a series of changes at the molecular, biochemical, and physiological levels in insects (Chown and Nicolson, 2004; Bowler, 2018; Ma et al., 2021), such as water content (Prange, 1996), cell membranes (Neven, 2000; Rensing and Ruoff, 2002), and activity of enzymes (Greenspan et al., 1980). Meanwhile, thermal acclimation of insects is a form of plasticity that enables organisms to adjust their physiology, following chronic or brief exposure to stressful stimuli (Bowler, 2005; Angilletta, 2009). The challenge is understanding how thermal extremes or stresses act on and manipulate acclimation in insects.
Heat shock proteins (HSPs) are known as stress molecular chaperones, which are essential for environmental adaptation and are associated with a wide range of physiological and biochemical processes (King and MacRae, 2015). According to their molecular size, the major families of HSPs are named HSP100, HSP90, HSP70, HSP60, and HSP40, and small proteins (sHSP). HSPs play a vital role in insects’ responses to extreme temperatures (Basha et al., 2012; King and MacRae, 2015), as well as responding to cold/heat tolerances (Rutherford and Lindquist, 1998), density, starvation, poison, ultraviolet-C, and diapause (Sosalegowda et al., 2010; Tedeschiab et al., 2015; Zhang et al., 2015; Tedeschi et al., 2016). HSP70 and HSP90 are highly conserved in all eukaryotes and prokaryotes and consist of two highly conserved domains: an N-terminal ATP-binding domain and a C-terminal substrate-binding domain (Lindquist. 1986; Taipale et al., 2010). These proteins generally serve in regulating the adaptions of insects to adverse environments and serve as a predominant self-protection mechanism (Chen et al., 2015a; Guo and Feng, 2018). The heat shock protein 70 family includes stress-inducible genes (HSP70s) and constitutively expressed members or heat shock cognates (HSC70s) (Daugarrd et al., 2007). HSC70s participate in various processes in an unstressed cell, such as folding of proteins after translation or membrane translocation, and may or may not be influenced by stress (Denlinger et al., 2001; Daugarrd et al., 2007). HSP60 helps protect against protein aggregation of denaturing proteins during diapause and operates the bending and assembling of enzymes and other protein complexes related to energy metabolism (Meyer et al., 2003; Brackley and Grantham, 2009; Mayer, 2010; King and MacRae, 2015).
There is an approximate doubling in the frequency and the magnitude of regional heat wave events from 1960 to 2018 in China, with the top three regional heat wave events in the summers of 2013, 2017, and 2003 (Wang and Yan, 2021). Ostrinia furnacalis, Lepidoptera (Crambidae), is one of the most common pests of corn in China, which causes economic losses in summer (Zhou and He, 1995; He et al., 2018). The survey showed that it detected about 300 larvae per 100 corn plants in Jilin Province and even 100% damage in Qinhuangdao city (Hebei province) (Yuan et al., 2013). On a summer day (from July to August), the temperature often climbs to 38 or more and has been reported to significantly affect the O. furnacalis population (Zhou et al., 2018).
The well-known mechanism used to cope with extreme temperatures is the expression of stress-inducible HSPs (Feder and Hofmann 1999; Sørensen et al., 2003; King and MacRae, 2015; Ma et al., 2021). The transcription of genes (mRNAs) encoding inducible heat shock proteins (in the response) appears to be temperature-sensitive in insects (Lindquist, 1986; Theodorakis and Morimoto, 1987; Morimoto, 1993; Sistonen et al., 1994; Prahlad and Morimoto, 2009; Lewis et al., 2016; Jin et al., 2020; Tian et al., 2020). However, the HSP expression of thermal tolerance or acclimation of O. furnacalis and the molecular mechanisms in its physiology, are poorly understood. In our study, we focused on the high temperatures that induce HSPs and then investigated O. furnacalis thermal tolerance in progressive recovery from extremely high temperatures. We related dynamic changes of potential heat shock protein genes through reverse-transcription quantitative polymerase chain reactions (RT-qPCRs). Our study helps improve the understanding of the mechanisms of thermotolerance in O. furnacalis and at a molecular level, analyzes the acclimation characteristics.
Materials and methods
Insects
Asian corn borer, O. furnacalis, adults were collected from Luoyang city, Henan province (111.8′112.59 “E, 33.35′35.05″ N). Progenies were maintained at insectary (27 ± 1°C, 70 ± 10% RH, and L16: D8 h) with standard artificial diet and techniques (Zhou and He, 1995) in the insectary to establish a laboratory colony. The 12-day-old (4th instar) larvae, which are more resilient to stress, were used in the following experiments.
Heat shock and recovery experiments
Ten larvae were placed into a 10-ml centrifuge tube with 12 holes (0.2 cm in diameter). Three experiments for exposure to extremely high temperatures were carried out. Two independent replicates were performed in the experiments.
Exp. 1: the extreme heat wave in August has been reported at 43 C in some parts of China (https://news.bjd.com.cn/2022/08/13/10133544.shtml). Our previous findings have shown that O. furnacalis eggs could not survive at 45 C (Quan et al., 2022). Larvae (ca. 400) were exposed to extremely high temperatures of 43 C with 50% ± 10% RH (usually it is ∼40%–55% on 5–7 days at 35°C–40°C in the field) (Zhou et al., 1996; Chen et al., 2012) for 2 h in the chambers. Then, the larvae were transferred to 27°C, 70% ± 10% RH for recovery. After 0, 0.5, 1, 1.5, 2, 4, and 8 h, fifty larvae each were transferred to the chamber set up with a temperature of 43°C and 50% ± 10% RH. Survivors were checked by touching a larva’s head with a small paintbrush, and they recorded every hour until all larvae were dead. Another fifty larvae were maintained at 27°C (70% ± 10% RH); thus, unexperienced exposure to 43 C was used as control. They were also subjected to the chamber at 43°C and 50% ± 10% RH, and survivors were checked out hourly.
Exp. 2: to generate a time series of heat shocks, 30–40 larvae were exposed to treatment temperatures of 31, 33, and 35 C for 1, 2, 4, 6, and 8 h in a chamber (VM04/100, Heraeus, Germany) with 50% ± 10% RH. Treated larvae were then frozen in liquid nitrogen and stored at −80 C before being used for mRNA extraction. Larvae were maintained at 27°C, and RH 70% ± 10% were used as a control.
Exp. 3: to investigate the effect of recovery time, 270 larvae were first exposed to 43°C and 50% ± 10% RH for 2 h. They were then transferred to 27 C for recovery. Finally, 30 larvae at a time were frozen in liquid nitrogen and stored at −80 C after 0, 0.5, 1, 1.5, 2, 4, 8, 16, and 32 h of recovery.
Exp. 4: larvae were exposed to 43 C 50% ± 10% RH for 2 h. They were then transferred to 27°C and 70% ± 10% RH for recovery. After 0, 0.5, 1, 2, 4, 6, and 8 h, the larvae were re-exposed to 43°C and 50% ± 10% RH for 0, 0.5, 1, 1.5, 2, 4, 8, and 16 h. Lastly, fifty larvae at a time were immediately frozen in liquid nitrogen and stored at −80 C before being used for mRNA extraction.
RNA isolation and cDNA synthesis
TRIzol (Invitrogen, United States) reagent was used to extract total RNA from sampled insects, following the manufacturer’s instructions. In brief, frozen tissues were smashed for ∼8 min (adding 15 ml liquid nitrogen per 2–3 min) by hand. Then, ∼0.1 g of insect powder was homogenized with 1 ml of TRIzol reagent into a 1.5-ml centrifuge tube and incubated at room temperature for 5 min. Phase separation of RNA was performed using 200 µl chloroform, 500 µl isopropanol for precipitation, washing with 800 μl, and 70% ethanol. The reaction was stopped by washing, followed by centrifugation at 4°C, 7,500 rpm for 5 min, and the supernatant was removed. We repeated the washing several times, subsequently air-dried samples, and then re-dissolved them (the final sediment) in RNase-free water. The purity and concentration of RNA were measured by agarose gel electrophoresis using a Nanodrop 2000 spectrophotometer (ThermoScientific, United States). The samples with an A260/280 ratio of ≥1.8 were used in downstream applications. The aliquots were frozen and stored at −80 C.
The cDNA synthesis was performed using a commercial reverse transcription kit (AT341-02, TransGen Biotech, China). An aliquot of RNA (2000 ng) from each sample was mixed with the reaction including 10 µl of the sample (dissolved by RNase-free water), 1 µl of anchored Oligo (dT) 18 primer, 1 µl of the TransScript RT/RI enzyme mix, 1 µl of the gDNA remover, and 10 μl of the TS reaction mix. This solution was mixed; then, the reaction was incubated at 42 C for 30 min and 85 C for 5 s. When the reaction was stopped, the cDNA was stored at −20 C.
Quantification of HSP gene expression (qRT-PCR)
According to the sequences of HSP60 (accession No: XM_028317890.1), HSC70 (accession No: JF708083.1), HSP70 (accession No: XM_028322992.1), and HSP90 (accession No: GU230734.1) in Asian corn borer registered in GenBank, the specific primers were designed. Through comparison and verification, 10 primers were used to amplify selected target genes (Supplementary Table S1).
The kit used for fluorescence quantitative real-time PCR (qRT-PCR) was SYBR@ Premix Ex Taq™ (TaKaRa). Ribosomal protein L8 (18sRNA) was used as a reference gene (Wang et al., 2007). The amplification program was set as follows: 1) initial denaturation 2 min at 95°C; 2) 95°C for 5 s and 60°C for 30 s; 3) step 2) was repeated for 40 cycles. Each sample was replicated three times, and we calculated the average value (Wang et al., 2007). The amplification efficiency of each heat shock protein gene was recorded, and the different heat shock protein genes related to transcription were analyzed by the 2−△△CT method (Livak and Schmittgen, 2001).
Data analysis
Larval thermal tolerance data, i.e., the survival as influenced by the time in the various recovery time treatments after exposure to 43°C, were analyzed and calculated by the Kaplan–Meier function. HSP expression levels under different temperatures were analyzed using a one-way analysis of variance (one-way ANOVA). The means were compared with Fisher’s protected LSD test, and statistical significance was considered at p < 0.05. All data analyses were processed in SPSS 17.0 software (Chicago: SPSS Inc.).
Results
Heat shock-induced thermal tolerance
The survival curves of different recovery time were significantly different in larvae (df = 6; χ2 = 18.7; p < 0.05) (Figure 1A). The median lethal time (Lt50) (from the Kaplan–Meier function) of survival in heat-shocked larvae (43 C for 2 h) was significantly lower when they were re-exposed to 43 C within 30 min (0.5 h), but it increased as recovery time increased. This reached untreated levels after 6 h of recovery and got significantly longer hereafter (Figure 1).
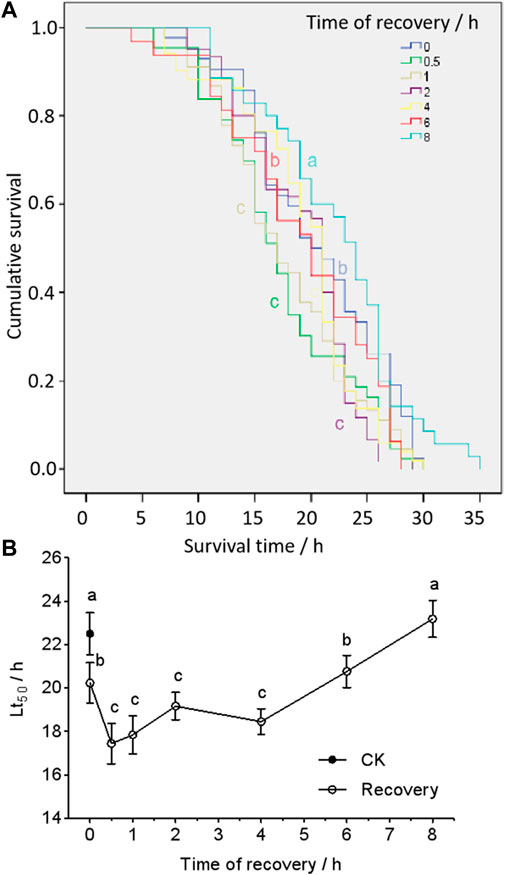
FIGURE 1. Cumulative survival curves (A) and medium lethal time (Lt50) (B) of larvae post-heat shock at 43°C, 2 h treatment. The curves (A) were analyzed by a Kaplan–Meier analysis (chi-squared test), and Lt50s (B) were from the Kaplan–Meier calculation. The different letters (the color of the letter stands for the same color curve) represent a significant difference (p < 0.05).
Transcriptional fluctuation of HSP genes in larvae exposed to different temperatures
In response to the heat shock treatment, the expression levels of the HSP genes varied significantly (F3,20 = 699, p < 0.001; F3,20 = 581, p < 0.001) (Figures 2, 3). In comparison with the control, the transcriptional levels of HSP60 and HSC70 did not change significantly among all temperature treatments (FHSP60 = 35, df = 3, 20, and p = 0.073; FHSC70 = 6, df = 3, 20, and p = 0.364) (Figures 2, 3A,B). By contrast, transcriptional levels of HSP70 and HSP90 were significantly upregulated in treatments of exposure to 33 (FHSP70 = 195, df = 8, and p < 0.05; FHSP90 = 163, df = 8, and p < 0.05) and 35 C (FHSP70 = 335, df = 8, and p < 0.001; FHSP90 = 584, df = 8, and p < 0.364) for 1 h compared to the control exposed at 27°C, but there was no significant difference observed between treatment temperature of 31°C (FHSP70 = 3.1, df = 8, p = 0.094; FHSP90 = 3.7, df = 8, p = 0.081) and the control (Figures 2C,D). However, the transcriptional level declined when exposure time increased and was at the untreated level in 4 h. Moreover, HSP70 could reach a higher peak value at the treatment of 43°C (>10-fold than the 35°C treatment) and remained high longer (16 h) (Figure 3C).
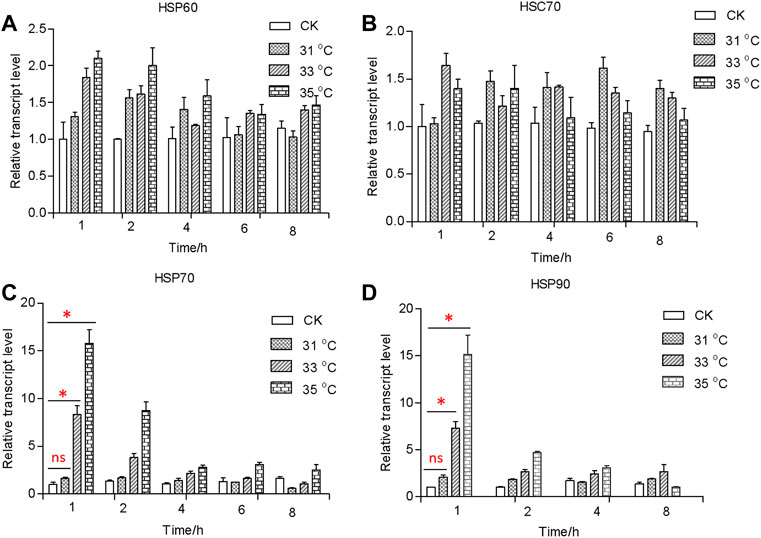
FIGURE 2. Relative transcript levels of HSP60 (A), HSC70 (B), HSP70 (C), and HSP90 (D) under different temperatures. Relative mRNA levels were analyzed using the 2−∆ ∆CT method. All values from two independent replicates are shown as the mean ± SD. The data (HSP70 and HSP90) between CK (27 C) and other treatments (31/33/35 C) at 1 h were analyzed by ANOVA. “*” stands for a significant difference (p < 0.05) between CK (27 C) and other treatments; “ns” is not significant.
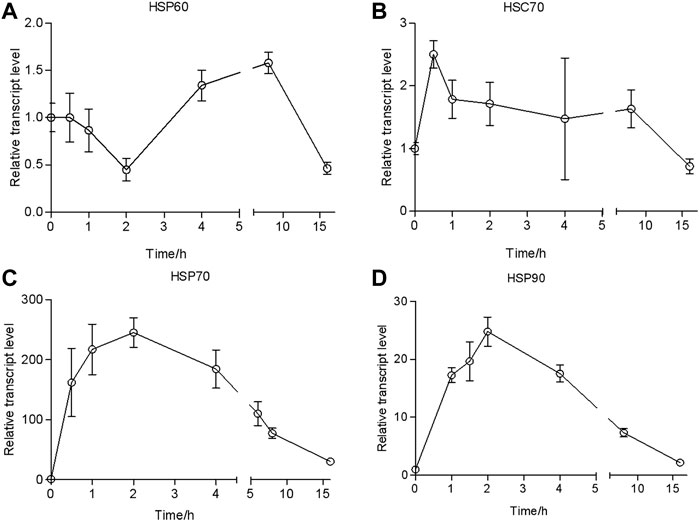
FIGURE 3. Relative transcript levels of HSP60 (A), HSC70 (B), HSP70 (C), and HSP90 (D) at different times with 43°C treatment. The values from two independent replicates are shown as the mean ± SD, as analyzed by the 2−△△CT method.
Dynamics of the transcriptional level of HSP genes in heat-shocked larvae
To investigate the responses of HSPs at different recovery times during the thermal tolerance, we quantified the dynamics of transcription of HSPs over time. The results of the qRT-PCR analysis showed that the transcriptional level of HSP60/HSC70 was upregulated only 3- and 5-fold after heat shock compared with the control (Figure 4). It would return to the untreated level after a recovery time of 0.5 h.
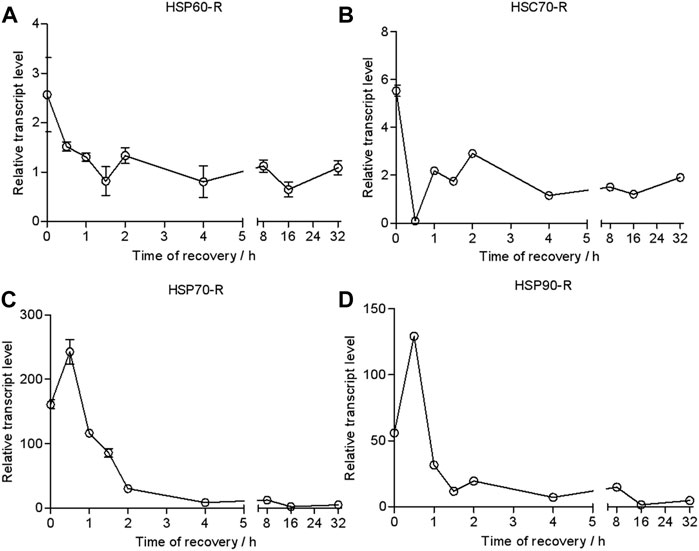
FIGURE 4. Relative transcript levels of HSP60 (A), HSC70 (B), HSP70 (C), and HSP90 (D) during recovery time (43°C, 2 h treatment). Relative mRNA levels were analyzed using the 2−∆ ∆CT method. The values from two independent replicates are shown as the mean ± SD.
By contrast, the transcriptional level of HSP70 rapidly increased 156-fold compared with the control and reached a peak of 230-fold at a recovery time of 0.5 h. It was dropped to 30-fold at a recovery time of 2 h. It returned to the control level at 16 h (Figure 4).
The transcriptional level of HSP90 was upregulated 50-fold in response to heat shock. It went up to 140-fold at a recovery time of 0.5 h but dropped down to 30-fold at a recovery time of 1 h. Then, it gradually decreased to the control level in 16 h.
Effects of recovery time on heat shock-induced transcriptional alteration of HSP genes
The recovery time of heat-shocked larvae significantly influenced the transcription of HSP70 and HSP90 genes when they were re-exposed to 43 C (Figure 5). As recovery time increased from 0.5 to 2 h, the peak of the relative transcriptional level of HSP70 increased from 177- to 209-fold and was lower than the control (0 h recovered, 245-fold) when heat-shocked larvae were resubjected to 43 C for 2 h. However, when recovery time increased to 4–6 h, the relative transcriptional level of HSP70 was ∼300- and 478-fold (at 8 h). This was similar to HSP70 whereas the transcription of HSP90 was upregulated to when 0.5 h recovered larvae were re-exposed to 43 C from 0.5 to 16 h; the peak time was 1 h. However, the transcriptional level was lower than the control (0 h recovered).
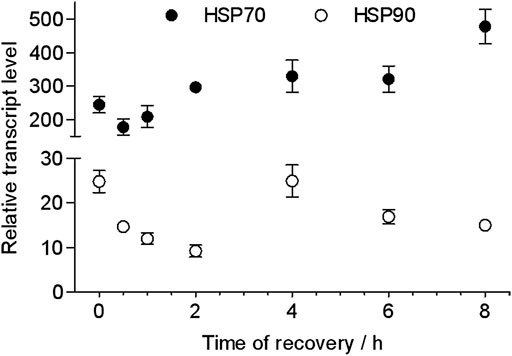
FIGURE 5. Peak relative transcript levels of HSP70 and HSP90 at different recovery times after heat shock at 43 C for 2 h. The values from two independent replicates are shown as the mean ± SD and analyzed by the 2−△△CT method.
Discussion
Thermal acclimation and/or heat shock can significantly alter thermotolerance in invertebrates, such as Tribolium castaneum (Lü and Huo, 2018), Drosophila melanogaster (Colinet et al., 2013), and Nilaparvata lugens (Piyaphongkul et al., 2014). However, thermal acclimation and/or heat shock-induced heat tolerance varies among the studies due to different exposure regimens, patterns, and hardening. (Dahlgaard et al., 1998; Ju et al., 2011; Piyaphongkul, 2013; Lu et al., 2016; Jin et al., 2019). In this study, the thermotolerance declined within 0.5–4 h after heat shock at 33°C–43°C, but it significantly increased after 6 h. This suggests that post-heat shock time is strongly related to thermotolerance.
Heat shock proteins are expressed as one of the defensive proteins in most organisms in response to various stressful conditions (Feder and Hoffmann, 1999). In most cases, heat-resistant species are characterized by a higher basal level of HSPs than more thermosensitive species. Previous studies showed that increased temperatures elicited activation of a conserved pathway involving heat shock transcription, which enhanced the heat shock response (Rinehart and Denlinger, 2000; Kayukawa et al., 2005; Lopez-Martinez and Denlinger, 2008; Gonsalves et al., 2011; Li et al., 2012; King and MacRae, 2015). In our study, the results showed that temperatures of 31 C–33 C were the threshold triggering HSP70 gene expression. We also detected increased transcription of HSP70 and HSP90 from the high-temperature (35°C–43°C) treatment. The transcription was correlated with the thermal time, but there was no significant change in the transcription of HSP60 or HSC70 after heat shock at 35°C–43°C. This suggests that HSP70 and HSP90 are responsible for heat-shocked induced defenses. This is similar to that reported for D. melanogaster, where expression of HSPs was affected by the heat shock treatment or response (Sørensen et al., 2007).
In addition, the upregulation of HSP70 or HSP90 was mostly detected after ∼1–2 h of exposure to heat, then slowed in the following period, and greatly decreased after being transferred to 27 C (for recovery). These results indicated the response to extreme heat events is related to HSP70 and HSP90 in O. furnacalis. Heat shock proteins are required for survival during heat or cold but cannot maintain a high level of expression in organisms all the time (Scott et al., 1997; Krebs and Feder, 1998; Bowler, 2005). Upregulation of the HSP70 gene in response to high temperatures was also observed in Bemisia tabaci (Hu et al., 2014), Drosophila buzzatii (Sørensen et al., 1999), Anopheles gambiae (Benoit et al., 2010), and Nilaparvata lugens (Lu et al., 2016). The temperatures >31 C upregulate the response of HSP70 and HSP90, but it was not sustainable (for more than 2 h).
The extremely high temperatures can generate damage indirectly by driving an increase in water loss, disrupting the cellular ion balance (hyperkalemia), impairing neurophysiological functions, and damaging mitochondria (O’Sullivan et al., 2017; Bowler, 2018). Insects can produce and accumulate particular molecules to prevent protein denaturation or cell inactivation when they suffer from thermal stimuli (King and MacRae, 2015; Ma et al., 2021). In this study, we observed that the thermal tolerance was significantly lower at the post-heat shock time of 0.5–4 h, with no difference at 6 h, and then enhanced at 8 h. This implies that the insects had internal injuries while suffering heat shock, but it was possibly self-healing within 6 h. Immunological/defensive memory takes over “experience” from the previous injury and rebuilds the immunological/defensive system over 6 h of recovery.
A higher transcription of HSP70 was detected in a post-heat shock time of 8 h. Correspondingly, the longest median death time was observed at this time. These findings reveal that HSP70 and HSP90 are related to the heat shock response and play an important role in heat shock-induced thermotolerance. It also indicates that the inducible HSP70 protects cells or tissue against thermal tolerance and delays thermal injury (Hoffmann et al., 2003; Ruell et al., 2009). Similar findings have been reported in other species. For instance, in D. melanogaster, the heat tolerance, metabolic rate, and gene expression significantly change after heat pretreatment (Sørensen et al., 2005; Malmendal et al., 2006). Apple maggot Rhagoletis pomonella expresses HSP increasingly from midday to a peak in the afternoon in summer (Lopez-Martinez and Denlinger, 2008). Locusts Locusta migratoria are more heat tolerant at low than at high latitudes as a result of their expression pattern of HSP70 and HSP90 (Chen et al., 2015b). HSPs (Feder et al., 1997; Lopez-Martinez and Denlinger, 2008) and cuticle proteins (Nguyen et al., 2009) are induced and/or accumulated to deal with extremely high temperatures in Aphis gossypii and sorbitol in Bemisia argentifolii. In this study, enhancing heat resistance could induce recovery in 6–8 h and is mostly regulated by HSP70.
In conclusion, our results suggested that HSP70 and HSP90 in O. furnacalis are immediately induced by heat events, but HSP60 and HSC70 are not. The highest upregulation of HSPs is achieved in 2 h and then returns to a normal level at 16 h. In addition, with a post-heat shock time of 0.5–4 h (i.e., recovery time), there is lower thermotolerance, possibly a defensive rebuilding time. Heat-induced thermotolerance achieves at a post-heat shock time of >6 h. Extreme thermal stress-induced quick dynamics of HSP70 or HSP90 could be interpreted as an 7adaptation to the drastic and rapid temperature variations. These findings are helpful to understand insect responses to stress.
Data availability statement
The datasets presented in this study can be found in online repositories. The names of the repository/repositories and accession number(s) can be found at: https://www.ncbi.nlm.nih.gov/nuccore/XM_028317890.1, XM_028317890.1; https://www.ncbi.nlm.nih.gov/nuccore/JF708083.1, JF708083.1; https://www.ncbi.nlm.nih.gov/nuccore/XM_028322992.1, XM_028322992.1; https://www.ncbi.nlm.nih.gov/nuccore/GU230734.1, GU230734.1.
Author contributions
KH and YQ conceptualized the research. YQ, KH, and ZW designed the experiments. YQ performed the experiment. YQ and KH contributed to data analyses. YQ wrote the manuscript, and KH revised the manuscript. All authors contributed to the manuscript and approved the submitted version.
Funding
This research was supported by the programs of the China Agriculture Research System of MOF and MARA (CARS-02) and the Agricultural Science and Technology Innovation Program (ASTIP) of CAAS.
Conflict of interest
The authors declare that the research was conducted in the absence of any commercial or financial relationships that could be construed as a potential conflict of interest.
Publisher’s note
All claims expressed in this article are solely those of the authors and do not necessarily represent those of their affiliated organizations, or those of the publisher, the editors, and the reviewers. Any product that may be evaluated in this article, or claim that may be made by its manufacturer, is not guaranteed or endorsed by the publisher.
Supplementary material
The Supplementary Material for this article can be found online at: https://www.frontiersin.org/articles/10.3389/fphys.2022.992293/full#supplementary-material
References
Alexander L. V., Zhang X., Peterson T. C., Caesar J., Gleason B. E., Klein Tank A. M. G., et al. (2006). Global observed changes in daily climate extremes of temperature and precipitation. J. Geophys. Res. 111, D05109. doi:10.1029/2005JD006290
Angilletta M. J. (2009). Looking for answers to questions about heat stress: Researchers are getting warmer. Funct. Ecol. 23, 231–232. doi:10.1111/j.1365-2435.2009.01548.x
Basha E., O’Neill H., Vierling E. (2012). Small heat shock proteins and α-crystallins: Dynamic proteins with flexible functions. Trends biochem. Sci. 37, 106–117. doi:10.1016/j.tibs.2011.11.005
Benoit J. B., Lopez-Martinez G., Phillips Z. P., Patrick K. R., Denlinger D. L. (2010). Heat shock proteins contribute to mosquito dehydration tolerance. J. Insect Physiol. 56, 151–156. doi:10.1016/j.jinsphys.2009.09.012
Bowler K. (2005). Acclimation, heat shock and hardening. J. Therm. Biol. 30, 125–130. doi:10.1016/j.jtherbio.2004.09.001
Bowler K. (2018). Heat death in poikilotherms: Is there a common cause? J. Therm. Biol. 76, 77–79. doi:10.1016/j.jtherbio.2018.06.007
Brackley K. I., Grantham J. (2009). Activities of the chaperonin containing TCP-1 (CCT): Implications for cell cycle progression and cytoskeletal organisation. Cell Stress Chaperones 14, 23–31. doi:10.1007/s12192-008-0057-x
Chen B., Li S., Ren Q., Tong X., Zhang X., Kang L. (2015a). Paternal epigenetic effects of population density on locust phase-related characteristics associated with heat-shock protein expression. Mol. Ecol. 24, 851–862. doi:10.1111/mec.13072
Chen W., Li D., Zhang M., Zhao Y., Wu W., Zhang G. (2015b). Cloning and differential expression of five heat shock protein genes associated with thermal stress and development in the polyphagous predatory mite Neoseiulus cucumeris (Acari: Phytoseiidae). Exp. Appl. Acarol. 67, 65–85. doi:10.1007/s10493-015-9933-0
Chen Y., Chen Y., Wen Y., Zhang X., Chen D. (2012). The effects of the relative humidity on the insecticidal expression level of Bt cotton during bolling period under high temperature. Field Crops Res. 137, 141–147. doi:10.1016/j.fcr.2012.08.015
Chown S. L., Nicolson S. W. (2004). Insect physiological ecology: Mechanisms and patterns. Oxford, UK: Oxford Univ. Press.
Colinet H., Overgaard J., Com E., Sorensen J. G. (2013). Proteomic profiling of thermal acclimation in Drosophila melanogaster. Insect biochem. Mol. Biol. 43, 352–365. doi:10.1016/j.ibmb.2013.01.006
Dahlgaard J., Loeschcke V., Michalak P., Justesen J. (1998). Induced thermotolerance and associated expression of the heat-shock protein Hsp70 in adult Drosophila melanogaster. Funct. Ecol. 12, 786–793. doi:10.1046/j.1365-2435.1998.00246.x
Daugarrd M., Rohde M., Jaattela M. (2007). The heat shock protein 70 family: Highly homologous proteins with overlapping and distinct functions. FEBS Lett. 581, 3702–3710. doi:10.1016/j.febslet.2007.05.039
Denlinger D. L., Rinehart J. P., Yocum G. D. (2001). “Stress proteins: A role in insect diapause?,” in Insect timing: Circadian rhythmicity to seasonality. Editors D. L. Denlinger, J. M. Giebultowicz, and D. S. Saunders (Amsterdam: Elsevier), 155–171.
Feder M. E., Blair N. T., Figueras H. (1997). Natural thermal stress and heat-shock protein expression in Drosophila larvae and pupae. Funct. Ecol. 11, 90–100. doi:10.1046/j.1365-2435.1997.00060.x
Feder M. E., Hofmann G. E. (1999). Heat-shock proteins, molecular chaperones, and the stress response: Evolutionary and ecological physiology. Annu. Rev. Physiol. 61, 243–282. doi:10.1146/annurev.physiol.61.1.243
Fox R. J., Donelson J. M., Schunter C., Ravasi T., Gaitán-Espitia J. D. (2019). Beyond buying time: The role of plasticity in phenotypic adaptation to rapid environmental change. Philos. Trans. R. Soc. Lond. B Biol. Sci. 374, 20180174. doi:10.1098/rstb.2018.0174
Gibert P., Debat V., Ghalambor C. K. (2019). Phenotypic plasticity, global change, and the speed of adaptive evolution. Curr. Opin. Insect Sci. 35, 34–40. doi:10.1016/j.cois.2019.06.007
Gonsalves S. E., Moses A. M., Razak Z., Robert F., Westwood J. T. (2011). Whole-genome analysis reveals that active heat shock factor binding sites are mostly associated with non-heat shock genes in Drosophila melanogaster. PLoS One 6, e15934. doi:10.1371/journal.pone.0015934
Greenspan R. J., Finn J. A., Hall J. C. (1980). Acetylcholinesterase mutants in Drosophila and their effects on the structure and function of the central nervous system. J. Comp. Neurol. 189, 741–774. doi:10.1002/cne.901890409
Gunderson A. R., Stillman J. H. (2015). Plasticity in thermal tolerance has limited potential to buffer ectotherms from global warming. Proc. Biol. Sci. 282, 20150401. doi:10.1098/rspb.2015.0401
Guo X., Feng J. (2018). Comparisons of expression levels of heat shock proteins (HSP70 and HSP90) from Anaphothrips obscurus (Thysanoptera: Thripidae) in polymorphic adults exposed to different heat shock treatments. J. Insect Sci. 18, 1–10. doi:10.1093/jisesa/iey059
He H. M., Xiao L., Chen Q. W., Xue F. S. (2018). A life-history trait of the asian corn borer, Ostrinia furnacalis (Lepidoptera: Pyralidae)—positive correlation between body weight and temperature. Acta. Entomol. Sin. 61, 340–347. doi:10.16380/j.kcxb.2018.03.009
Hoffmann A. A., Chown S. L., Clusella-Trullas S. (2013). Upper thermal limits in terrestrial ectotherms: How constrained are they? Funct. Ecol. 27, 934–949. doi:10.1111/j.1365-2435.2012.02036.x
Hoffmann A. A., Sørensen J. G., Loeschcke V. (2003). Adaptation of Drosophila to temperature extremes: Bringing together quantitative and molecular approaches. J. Therm. Biol. 28, 175–216. doi:10.1016/S0306-4565(02)00057-8
Hu J., Chen B., Li Z. (2014). Thermal plasticity is related to the hardening response of heat shock protein expression in two Bactrocera fruit flies. J. Insect Physiol. 67, 105–113. doi:10.1016/j.jinsphys.2014.06.009
Jin J., Li Y., Zhou Z., Zhang H., Guo J., Wan F. (2020). Heat shock factor is involved in regulating the transcriptional expression of two potential Hsps (AhHsp70 and AhsHsp21) and its role in heat shock response of Agasicles hygrophila. Front. Physiol. 11, 562204. doi:10.3389/fphys.2020.562204
Jin J., Zhao M., Wang Y., Zhou Z., Wan F., Guo J. (2019). Induced thermotolerance and expression of three key Hsp genes (Hsp70, Hsp21, and sHsp21) and their roles in the high temperature tolerance of Agasicles hygrophila. Front. Physiol. 10, 1593. doi:10.3389/fphys.2019.01593
Ju R. T., Chen G. B., Ju R. T., Chen G. B., Wang F., Li B., et al. (2011). Effects of heat shock, heat exposure pattern, and heat hardening on survival of the sycamore lace bug, Corythucha ciliata. Entomol. Exp. Appl. 141, 168–177. doi:10.1111/j.1570-7458.2011.01180.x
Kayukawa T., Chen B., Miyazaki S., Itoyama K., Shinoda T., Ishikawa Y. (2005). Expression of mRNA for the t-complex polypeptide–1, a subunit of chaperonin CCT, is upregulated in association with increased cold hardiness in Delia antiqua. Cell Stress Chaperones 10, 204–210. doi:10.1379/CSC-106R.1
King A. M., MacRae T. H. (2015). Insect heat shock proteins during stress and diapause. Annu. Rev. Entomol. 60, 59–75. doi:10.1146/annurev-ento-011613-162107
Krebs R., Feder E. (1998). Hsp70 and larval thermotolerance in Drosophila melanogaster: How much is enough and when is more too much? J. Insect Physiol. 44, 1091–1101. doi:10.1016/S0022-1910(98)00059-6
Lewis M., Götting M., Anttila K., Kanerva M., Prokkola J. M., Seppänen E., et al. (2016). Different relationship between hsp70 mrna and hsp70 levels in the heat shock response of two salmonids with dissimilar temperature preference. Front. Physiol. 7, 511. doi:10.3389/fphys.2016.00511
Li J., Moghaddam H. H., Du X., Zhong B., Chen Y. Y. (2012). Comparative analysis on the expression of inducible HSPs in the silkworm, Bombyx mori. Mol. Biol. Rep. 39, 3915–3923. doi:10.1007/s11033-011-1170-y
Lindquist S. (1986). The heat-shock response. Annu. Rev. Biochem. 55, 1151–1191. doi:10.1146/annurev.bi.55.070186.005443
Livak K. J., Schmittgen T. D. (2001). Analysis of relative gene expression data using real-time quantitative PCR and the 2(-Delta C(T)) Method. Methods 25, 402–408. doi:10.1006/meth.2001.1262
Lopez-Martinez G., Denlinger D. L. (2008). Regulation of heat shock proteins in the apple maggot Rhagoletis pomonella during hot summer days and overwintering diapause. Physiol. Entomol. 33, 346–352. doi:10.1111/j.1365-3032.2008.00639.x
Lü J., Huo M. (2018). Transcriptome analysis reveals heat tolerance of Tribolium castaneum (Herbst) (Coleoptera: Tenebrionidae) adults. J. Stored Prod. Res. 78, 59–66. doi:10.1016/j.jspr.2018.06.004
Lu K., Chen X., Liu W., Zhou Q. (2016). Characterization of heat shock cognate protein 70 gene and its differential expression in response to thermal stress between two wing morphs of Nilaparvata lugens (Stål). Comp. Biochem. Physiol. A Mol. Integr. Physiol. 199, 47–53. doi:10.1016/j.cbpa.2016.05.009
Ma C. S., Ma G., Pincebourde S. (2021). Survive a warming climate: Insect responses to extreme high temperatures. Annu. Rev. Entomol. 66, 163–18422. doi:10.1146/annurev-ento-041520-074454
Malmendal A., Overgaard J., Bundy J. G., Sørensen J. G., Nielsen N. C., Loeschcke V., et al. (2006). Metabolomic profiling of heat stress: Hardening and recovery of homeostasis in Drosophila. Am. J. Physiol. Regul. Integr. Comp. Physiol. 291, 205–212. doi:10.1152/ajpregu.00867.2005
Mayer M. P. (2010). Gymnastics of molecular chaperones. Mol. Cell 39, 321–331. doi:10.1016/j.molcel.2010.07.012
Meehl G. A., Tebaldi C. (2004). More intense, more frequent, and longer lasting heat waves in the 21st century. Science 305, 994–997. doi:10.1126/science.1098704
Meyer A. S., Gillespie J. R., Walther D., Millet I. S., Doniach S., Frydman J. (2003). Closing the folding chamber of the eukaryotic chaperonin requires the transition state of ATP hydrolysis. Cell 113, 369–381. doi:10.1016/S0092-8674(03)00307-6
Morimoto R. I. (1993). Cells in stress: Transcriptional activation of heat shock genes. Science 259, 1409–1410. doi:10.1126/science.8451637
Neven L. G. (2000). Physiological responses of insects to heat. Postharvest Biol. Technol. 21, 103–111. doi:10.1016/S0925-5214(00)00169-1
Nguyen T. T., Michaud D., Cloutier C. (2009). A proteomic analysis of the aphid Macrosiphum euphorbiae under heat and radiation stress. Insect biochem. Mol. Biol. 39, 20–30. doi:10.1016/j.ibmb.2008.09.014
O’Sullivan J., MacMillan H. A., Overgaard J. (2017). Heat stress is associated with disruption of ion balance in the migratory locust, Locusta migratoria. J. Therm. Biol. 68, 177–185. doi:10.1016/j.jtherbio.2016.04.001
Piyaphongkul J. (2013). Effects of thermal stress on the brown planthopper Nilaparvata lugens (Stal). Birmingham: Diss. University of Birmingham.
Piyaphongkul J., Pritchard J., Bale J. (2014). Effects of acclimation on the thermal tolerance of the Brown planthopper Nilaparvata lugens (Stal). Agric. For. Entomol. 16, 174–183. doi:10.1111/afe.12047
Prahlad V., Morimoto R. I. (2009). Integrating the stress response: Lessons for neurodegenerative diseases from C. elegans. Trends Cell Biol. 19, 52–61. doi:10.1016/j.tcb.2008.11.002
Prange H. D. (1996). Evaporative cooling in insects. J. Insect Physiol. 42 (5), 493–499. doi:10.1016/0022-1910(95)00126-3
Quan Y., Mason C. E., He K., Wang Z., Wei H. (2022). Impact of heat waves on egg survival and biological performance across life stages in the Asian corn borer. Entomol. Exp. Appl. [in press].
Rensing L., Ruoff P. (2002). Temperature effect on entrainment, phase shifting, and amplitude of circadian clocks and its molecular bases. Chronobiol. Int. 19, 807–864. doi:10.1081/CBI-120014569
Rinehart J. P., Denlinger D. L. (2000). Heat-shock protein 90 is down-regulated during pupal diapause in the flesh fly, Sarcophaga crassipalpis, but remains responsive to thermal stress. Insect Mol. Biol. 9, 641–645. doi:10.1046/j.1365-2583.2000.00230.x
Ruell P. A., Thompson M. W., Hoffman K. M. (2009). Heat shock proteins as an aid in the treatment and diagnosis of heat stroke. J. Therm. Biol. 34, 1–7. doi:10.1016/j.jtherbio.2008.09.004
Rutherford S. L., Lindquist S. (1998). Hsp90 as a capacitor for morphological evolution. Nature 396, 336–342. doi:10.1038/24550
Scott M., Berrigan D., Hoffmann A. A. (1997). Costs and benefits of acclimation to elevated temperature in Trichogramma carverae[J]. Entomol. Exp. Appl. 85, 211–219. doi:10.1046/j.1570-7458.1997.00251.x
Sgrò C. M., Terblanche J. S., Hoffmann A. A. (2016). What can plasticity contribute to insect responses to climate change? Annu. Rev. Entomol. 61, 433–451. doi:10.1146/annurev-ento-010715-023859
Sistonen L., Sarge K. D., Morimoto R. I. (1994). Human heat shock factors 1 and 2 are differentially activated and can synergistically induce hsp70 gene transcription. Mol. Cell. Biol. 14, 2087–2099. doi:10.1128/MCB.14.3.2087
Sørensen J. G., Kristensen T. N., Kristensen K. V., Loeschcke V. (2007). Sex specific effects of heat induced hormesis in Hsf-deficient Drosophila melanogaster. Exp. Gerontol. 42, 1123–1129. doi:10.1016/j.exger.2007.09.001
Sørensen J. G., Loeschcke V., Kristensen T. N. (2003). The evolutionary and ecological role of heat shock proteins. Ecol. Lett. 6, 1025–1037. doi:10.1046/j.1461-0248.2003.00528.x
Sørensen J. G., Michalak P., Justesen J., Loeschcke V. (1999). Expression of the Heat-shock protein HSP70 in Drosophila buzzatii lines selected for thermal resistance. Hereditas 131, 155–164. doi:10.1111/j.1601-5223.1999.00155.x
Sørensen J. G., Nielsen M. M., Kruhøffer M., Justesen J., Loeschcke I. (2005). Full genome expression analysis of the heat stress response in Drosophila melanogaster. Cell Stress Chaperones 10, 312–328. doi:10.1379/CSC-128R1.1
Sosalegowda A. H., Kundapur R. R., Boregowda M. H. (2010). Molecular characterization of heat shock proteins 90 (HSP83?) and 70 in tropical strains of Bombyx mori. Proteomics 10, 2734–2745. doi:10.1002/pmic.200800830
IPCC (2013). “Technical summary,” in Climate change 2013: The physical science basis. Contribution of working group I to the fifth assessment report of the intergovernmental panel on climate change. Editors T. F. Stocker, D. Qin, G-K. Plattner, M. Tignor, S. K. Allen, J. Boschunget al. (Cambridge, UK: Cambridge Univ. Press), 1–127.
Taipale M., Jarosz D. F., Lindquist S. (2010). HSP90 at the hub of protein homeostasis: Emerging mechanistic insights. Nat. Rev. Mol. Cell Biol. 11, 515–528. doi:10.1038/nrm2918
Tedeschi J. N., Kennington W. J., Tomkins J. L., Berry O., Whiting S., Meekan M. G., et al. (2016). Heritable variation in heat shock gene expression: A potential mechanism for adaptation to thermal stress in embryos of sea turtles. Proc. Biol. Sci. 283, 20152320–20152327. doi:10.1098/rspb.2015.2320
Tedeschiab J. N., Kenningtona W. J., Berryc O., Whitingd S., Meekanbe M., Mitchellab N. J. (2015). Increased expression of Hsp70 and Hsp90 mRNA as biomarkers of thermal stress in loggerhead turtle embryos (Caretta Caretta). J. Therm. Biol. 47, 42–50. doi:10.1016/j.jtherbio.2014.11.006
Theodorakis N. G., Morimoto R. I. (1987). Posttranscriptional regulation of hsp70 expression in human cells: Effects of heat shock, inhibition of protein synthesis, and adenovirus infection on translation and mRNA stability. Mol. Cell. Biol. 7, 4357–4368. doi:10.1128/MCB.7.12.4357
Tian C. B., Li Y. Y., Huang J., Chu W. Q., Wang Z. Y., Liu H. (2020). Comparative transcriptome and proteome analysis of heat acclimation in predatory mite Neoseiulus barkeri. Front. Physiol. 11, 426. doi:10.3389/fphys.2020.00426
van Heerwaarden B., Kellermann V., Sgro C. M. (2016). Limited scope for plasticity to increase upper thermal limits. Funct. Ecol. 30, 1947–1956. 56. doi:10.1111/1365-2435.12687
Wang H. S., Wang X. H., Zhou C. S., Huang L. H., Zhang W., Guo W., et al. (2007). cDNA cloning of heat shock proteins and their expression in the two phases of the migratory locust. Insect Mol. Biol. 16, 207–219. doi:10.1111/j.1365-2583.2006.00715.x
Wang J., Yan Z. (2021). Rapid rises in the magnitude and risk of extreme regional heat wave events in China. Weather Clim. Extrem. 34, 100379. doi:10.1016/j.wace.2021.100379
Yuan Z., Guo J., Wang Z., He K., Bai S. (2013). Feeding preference of the Asia corn borer larvae for different host plants. Acta phytophy. Sin. 40, 205–210.
Zhang Y., Liu Y., Zhang J., Guo Y., Ma E. (2015). Molecular cloning and mRNA expression of heat shock protein genes and their response to cadmium stress in the grasshopper Oxya chinensis. PLOS One 7, e0131244–16. doi:10.1371/journal.pone.0131244
Zhou D. R., He K. L. (1995). The integrated control technology of corn borer. Beijing, China: Golden Shield Press.
Zhou G. Y., Yang F. X., Fu X. Q., Wang X. L. (1996). The inflfluence of climatic differences in the Yangtze River Valley on cotton yield and fibre quality. China Cott. 10, 15–18.
Zhou J. C., Liu Q. Q., Han Y. X., Dong H. (2018). High temperature tolerance and thermal-adaptability plasticity of Asian corn borer (Ostrinia furnacalis guenée) after a single extreme heat wave at the egg stage - sciencedirect. J. Asia. Pac. Entomol. 21 (3), 1040–1047. doi:10.1016/j.aspen.2018.07.024
Keywords: heat shock proteins, thermal tolerance, heat shock response, Ostrinia furnacalis, extreme climate events
Citation: Quan Y, Wang Z, Wei H and He K (2022) Transcription dynamics of heat shock proteins in response to thermal acclimation in Ostrinia furnacalis. Front. Physiol. 13:992293. doi: 10.3389/fphys.2022.992293
Received: 12 July 2022; Accepted: 02 September 2022;
Published: 26 September 2022.
Edited by:
Divya Singh, Chandigarh University, IndiaCopyright © 2022 Quan, Wang, Wei and He. This is an open-access article distributed under the terms of the Creative Commons Attribution License (CC BY). The use, distribution or reproduction in other forums is permitted, provided the original author(s) and the copyright owner(s) are credited and that the original publication in this journal is cited, in accordance with accepted academic practice. No use, distribution or reproduction is permitted which does not comply with these terms.
*Correspondence: Kanglai He, aGVrYW5nbGFpQGNhYXMuY24=