- 1School of Sport Medicine and Physical Therapy, Beijing Sport University, Beijing, China
- 2Sports Coaching College, Beijing Sport University, Beijing, China
- 3California State University, Long Beach, CA, United States
- 4National University of Defense Technology, Changsha, Hunan, China
- 5China Institute of Sport and Health Science, Beijing Sport University, Beijing, China
- 6Hebrew Senior Life Hinda and Arthur Marcus Institute for Aging Research, Harvard Medical School, Boston, MA, United States
Objective: In this study, we examined the effects of pre-exercise H2 gas inhalation on physical fatigue (PF) and prefrontal cortex (PFC) activation during and after high-intensity cycling exercise.
Methods: Twenty-four young men completed four study visits. On the first two visits, the maximum workload (Wmax) of cycling exercise of each participant was determined. On each of the other two visits, participants inhaled 20 min of either H2 gas or placebo gas after a baseline test of maximal voluntary isometric contraction (MVIC) of thigh. Then participants performed cycling exercise under their maximum workload. Ratings of perceived exertion (RPE), heart rate (HR) and the PFC activation by using functional near-infrared spectroscopy (fNIRS) was measured throughout cycling exercise. The MVIC was measured again after the cycling.
Results: It was observed that compared to control, after inhaling H2 gas, participants had significantly lower RPE at each workload phase (p < 0.032) and lower HR at 50% Wmax, 75% Wmax, and 100% Wmax during cycling exercise (p < 0.037); the PFC activation was also significantly increased at 75 and 100% Wmax (p < 0.011). Moreover, the H2-induced changes in PF were significantly associated with that in PFC activation, that is, those who had higher PFC activation had lower RPE at 75% Wmax (p = 0.010) and lower HR at 100% Wmax (p = 0.016), respectively.
Conclusion: This study demonstrated that pre-exercise inhalation of H2 gas can alleviate PF, potentially by maintaining high PFC activation during high-intensity exercise in healthy young adults.
Introduction
Physical fatigue (PF) is common during exercise, especially during the vigorous exercise with prolonged periods (Cordeiro et al., 2017). The PF oftentimes results in diminished sport performance (Birfer et al., 2019) and increased risk of athletic injury (Huygaerts et al., 2020). Therefore, strategies to alleviate the burden of PF are critical to improve sport performance and reduce risk of injury.
The development of PF is not only associated with peripheral factors in human neurophysiological procedures (e.g., failure of muscle contractility and excitatory contraction (E-C) coupling (Allen et al., 2008)) (Gandevia, 2001; Rasmussen et al., 2007), but also depends upon the altered regulation of the components in central nervous system (Enoka, 1995; Siemionow et al., 2000). For example, the prefrontal cortex (PFC) of the brain is thought to be the place where the information pertaining to fatigue is processed and the command to terminate the continuation of exercise is initiated (St Clair Gibson et al., 2013; Robertson and Marino, 2016; Hyland-Monks et al., 2018). Studies have observed that the activation of PFC, which can be reliably measured by the oxygenation using functional near-infrared spectroscopy (fNIRS), is linked to the degree of fatigue, that is, greater fatigue is associated with lower PFC activation (De Wachter et al., 2021). The capacity to maintaining the activation of PFC is thus critical to alleviate fatigue and maintain the athletic performance. For example, it was observed that the elite Kenyan runners with average time of 62.2 min to complete 21-km race can maintain PFC activation during a self-paced 5-km test, which may contribute to their success of long-distance running competition (Santos-Concejero et al., 2015). Therefore, strategies that can modulate PFC activation may be of great promise to help fatigue attenuation.
Recently, hydrogen molecules (H2) has gained much attention because of its biological effects (Kawamura et al., 2020) that can benefit brain function (Mizuno et al., 2017). The brain is susceptible to damage from reactive oxygen species (ROS) (Cobley et al., 2018) which often increase dramatically along with the increase of the demand for energy and metabolism, especially during high-intensity exercise (Fisher-Wellman and Bloomer, 2009; Kawamura and Muraoka, 2018). The H2 acts as a powerful antioxidant that can cross the blood-brain barrier (Hayashida et al., 2012) and then protect neuronal cells by selectively eliminating harmful ROS (Tomofuji et al., 2014; McCarty, 2015). In a double-blinded, placebo-controlled study, 4 weeks administration of hydrogen-rich water (HRW) helped reduce and prevent accumulated oxidative stress in the brain, thereby improving mood, anxiety, and autonomic function in adult volunteers (Mizuno et al., 2017). Recently, several studies have arisen to explore the potential anti-fatigue effects of H2 (Table 1) in healthy cohorts who performed either acute or chronic exercise, and shown the promise of intaking H2 either before or after the exercise may help alleviate fatigue (Aoki et al., 2012; Da Ponte et al., 2018; Botek et al., 2019; LeBaron et al., 2019; Mikami et al., 2019; Dobashi et al., 2020; Hori et al., 2020; Shibayama et al., 2020; Timon Andrada et al., 2020; Dong et al., 2022). For example, intaking HRW before exercise has been shown to improve exercise-induced decline of muscle function (Aoki et al., 2012), and thus alleviate fatigue (Pantovic et al., 2016); and the inhalation of hydrogen-rich gas mixture after exercise can help attenuated the reduction in athletic performance (e.g., the height of countermovement jump) (Shibayama et al., 2020). However, the effects of inhalation of H2 gas before high-intensity endurance exercise/fatigue on PF have not been well characterized, and it is still not clear if such benefits of H2 are achieved by modulating the cortical characteristics of the brain.
We here thus contend that pre-exercise inhalation of H2 gas can help PF by maintaining high level of PFC activation during high-intensity cycling exercise. To examine this, we here completed a randomized and double-blinded within-subject study in a group of healthy young adults. We hypothesized that as compared to control, pre-exercise inhalation of H2 gas would induce lower level of PF and greater activation of PFC; and such H2-induced improvement in PF would be associated with that in PFC activation.
Materials and methods
Participants
Twenty-four young men were recruited from Beijing Sport University. Inclusion criteria were age 18–35 years, the ability to complete the incremental exercise test and the cycling time to exhaustion prior to the formal trial. Exclusion criteria were self-reported acute illness, injury, or unstable medical condition or hospitalization within the past 3 months; any report of diseases of the musculoskeletal system, pain, or orthopedic problems likely to affect exercise; use of antipsychotics, anti-seizure, or other neuroleptic medication. The baseline characteristics of the participants are shown in Table 2. Before the study visits, participants were instructed to refrain from exercising that might cause fatigue for 48 h and alcohol and caffeine intake for 24 h. All participants were informed of the relevant benefits and possible risks involved in participating in this study and provided signed informed consent in order to participate in this study. The consent form included information contained in the Helsinki Declaration as well as the purpose of the study and details about the study’s protocols. This study was reviewed and approved by the Institutional Review Board of Beijing Sport University (number: 2021163H).
Experimental design
In this within-subject and double-blinded study, each participant completed four study visits. During the first visit, an incremental exercise test (2 min at 50 W + 20 W increments every 2 min) was performed until exhaustion (operationally defined as a pedal frequency of less than 60 revolutions/min (RPM) for more than 5 s with strong verbal encouragement) on an electromagnetically braked cycle ergometer (Excalibur Sport, Lode, Groningen, Netherlands) to measure peak power output.
After an interval of at least 48 h, on the second visit, participants performed a time-to-exhaustion cycling test to measure the maximum riding time (MRT). The test consisted of a 3 min warm-up at 40% of peak power output followed by a rectangular workload corresponding to 80% of peak power output that was achieved in the first visit. The test was completed when the pedal frequency was less than 60 RPM for more than 5 s despite standardized verbal encouragement. On the next two visits, participants completed the exercise and functional assessments after the H2 intervention. These two visits were separated by 1 week (i.e., wash-out period) to avoid potential after effects of the interventions from the prior visit (Javorac et al., 2019). Specifically, on each of the two visits, participants inhaled H2 gas (H2 group) or placebo gas (PLAC group) for 20 min when seating quietly in a chair, respectively, in a randomized order. The duration of H2 inhalation was 20 min, as determined according to the observations from previous studies (Korovljev et al., 2018; Javorac et al., 2019). Before the inhalation, participants completed the maximal voluntary isometric contraction (MVIC) test. Immediately after gas inhalation, participants sat quietly on the cycle ergometer for 2 min to complete the baseline assessment of resting-state fNIRS. Then after warming up at a load of 40% peak power output for 3 min, participants were instructed to ride at 80% peak power output for the duration of their MRT. The Borg CR10 Scale was used to quantify the whole-body fatigue during cycling. Heart rate (HR) and oxygenation of the PFC were recorded throughout the exercise (the averaged values of the last 30 s of the 0% (baseline), 25%, 50%, 75% and 100% of the maximum workload (Wmax) phases were intercepted for statistical analysis, respectively). After the ride, participants repeated MVIC test. The overview of the assessment protocol on one visit (Visit 3 or 4) is shown in Figure 1.
H2 gas
H2 gas was supplied through a hydrogen oxygen supply unit (Zhiheng Hydrogen Health Technology Co., Ltd., Fuzhou, China) connected to a nasal inhaler. The ratio of oxygen to hydrogen in the H2 gas is 2:1, and the maximum concentrations are 21.57% and 4.08%, respectively (Javorac et al., 2019).The flow rate of H2 gas is 1800 ml/min. The placebo gas was supplied by the same hydrogen oxygen supply unit that did not initiate the hydrogen production program. Participants reported no different feelings on the gas between the two inhalations.
Ratings of perceived exertion
Ratings of Perceived Exertion (RPE) was defined as the intensity of subjective effort, exertion, discomfort, or fatigue felt during exercise (Borg, 1982), as measured by the Borg CR10. The RPE represented feedback from cardiovascular, respiratory and musculoskeletal systems and it provides a whole-body fatigue assessment, while a single physiological parameter usually provides very limited information (Crewe et al., 2008; Venhorst et al., 2018). We here recorded the RPE of participants at the end of each workload phase (i.e., at baseline, 25%Wmax, 50% Wmax, 75% Wmax and 100% Wmax) and used it as the primary outcome of PF.
Heart rate monitoring
We used the Firstbeat heart rate belt (Firstbeat Analytics, Jyvaskyla, Finland) to monitor the HR changes of the participants during the cycling exercise. The heart rate belt was worn on the participant’s chest, and the receiver was positioned to the left of the midline. The elastic band was adjusted so that the position of the receiver did not change during the ride. During exercise, especially the prolonged high-intensity exercise, cardiovascular restriction is an important factor leading to fatigue (Nybo et al., 2014). Since HR is linearly related to exercise intensity (Portier et al., 2001; James et al., 2002), lower HR at the same exercise intensity represent the ability of the cardiovascular system to adapt to fatigue (LeBlanc et al., 1977). Therefore, HR was used as one of the secondary outcomes of PF in this study.
The maximum voluntary isometric contraction test
The MVIC was measured by a LINK isometric dynamometer (KFORCE, KINVENT BIOMECANIQUE, France). The participants were seated at the edge of the treatment bed with straps securing the trunk and thighs to avoid generating compensatory force. Then participants performed MVIC tests for three times at a knee joint angle of 60° with a 5 s interval. Each test lasted 5 s, and we took the average of these 5 s as the result of each test and the average of three tests was identified as the MVIC. Muscle fatigue is an exercise-induced reduction in maximal voluntary muscle force (Gandevia, 2001). We here quantified muscle fatigue using the change in MVIC before and after exercise (ΔMVIC) and used it as one of the secondary outcomes of PF.
Measurement of fNIRS
For PFC activation, the hemodynamic response of prefrontal brain areas was recorded using a multichannel continuous-wave fNIRS device (Oxymon, Artinis, Netherlands) consisting of 10 light sources and eight detectors mounted on a head cap. The head cap placement was centered around Cz (10/20 international system for electrode placement), the mid-point between the nasion to inion and left to right preauricular distances. Light sources and detectors were placed in the F4-FP1-F3 of the PFC area, to ensure consistency between participants and sessions. Data is collected at a frequency of 10 Hz.
Prior to the ride, participants were asked to sit quietly for 2 min with eyes open, breathing normally, relaxed and not controlling their mental activity in any particular way. The data recorded in the last 30 s were used as the baseline of resting-state fNIRS. Similarly, the average values of the last 30 s of each workload phase as described above (Figure 1) were intercepted respectively. Relative concentration changes (Δμmol) of oxyhemoglobin (ΔO2Hb), deoxyhemoglobin (ΔHHb), and total hemoglobin (ΔTHb = ΔO2Hb + ΔHHb) from baseline of resting-state to each workload were obtained to infer neural activation. Among them, △O2Hb was presumed to be the metabolic adjustment in response to neural firing (Obrig and Villringer, 2003) and is the most sensitive indicator of regional brain oxygenation changes and neural activation (Hoshi et al., 2001; Pan et al., 2019), which has been widely used to assess the changes in cortical neural activation during exercise (Jung et al., 2015; Radel et al., 2017). Therefore, we here used △O2Hb as the primary outcome of the PFC activation.
Data processing of fNIRS
The raw fNIRS data were preprocessed using the NIRS_KIT toolkit, an open-source package in MATLAB. To estimate the quality of the data channel, the signal-to-noise ratio of them was measured. First, the relative coefficient of variation (CV in %) was calculated for the raw data before filtering, which is a widely-used method for measuring multichannel NIRS (Schmitz et al., 2005; Schneider et al., 2011). The CV of each channel was calculated over the entire experimental duration, and CVs above 15% were rejected (Piper et al., 2014). Then the data was pre-processed using a third order low-pass filter of 0.2 Hz to remove non-related physiological components (e.g., heartbeats: 0.5–2.0 Hz, and respiration: 0.2–0.4 Hz) (Scholkmann et al., 2014), and then removed slow drift.
Statistical analysis
Statistical analyses were performed using SPSS 26.0 (IBM, Chicago, IL, United States). The significance level was set at p ≤ 0.05. Descriptive statistics (i.e., mean, standard deviation (SD)) were used to summarize the demographic characteristics of the participants and study outcomes.
To examine the effects of H2 gas on PF and PFC activation, two-way repeated-measures analyses of variance (ANOVAs) models were used. The dependent variable of each model was the primary outcomes (i.e., RPE) and the model factors were group (i.e., PLAC and H2 group) and workload (i.e., 0%, 25%, 50%, 75% and 100%Wmax) and their interactions. Similar analyses were used to examine such effects on the secondary outcome (i.e., HR). A Fisher’s LSD Post-hoc analyses were performed if there were significant interactions. As the MVIC was measured before the inhalation and after the exercise, we used one-way ANCOVA to compare the effects of H2 gas on it. The model factor was group and the pre-exercise MVIC was included as the covariate in the model.
To examine the associations between the H2-induced significant changes in PF outcomes (e.g., RPE and HR) and that in PFC activation (e.g., △O2Hb), we first calculated the percent changes of each outcome that was significantly different between groups (see Results below) from PLAC to H2 gas group for each participant. We then used the linear regression model to examine such associations.
Results
All 24 participants completed all experiments. However, the signal quality of the fNIRS channel was poor in 3 participants at the time of the experiment, so the fNIRS data of these 3 participants were not included in the statistical analysis. In addition, a total of eight HR data sets (five in PLAC group and two in H2 group) had to be excluded from the statistical analysis due to incomplete HR recorded by the heart rate band. No significant differences in RPE, HR and fNIRS outcomes at baseline were observed between PLAC and H2 gas inhalation (p > 0.131), but at baseline the MVIC was inconsistent in H2 and PLAC group (47.61 ± 2.87 vs. 53.44 ± 3.10; p = 0.006).
The effects of H2 gas on physical fatigue
Primarily, two-way mixed design ANOVA model showed a significant interaction of group and workload (F = 3.328, p = 0.026) on RPE. The post-hoc analysis showed that: 1) compared to baseline, RPE in both groups were significantly increased when workloads increased, suggesting the success of fatigue construction (p < 0.001); 2) and compared to PLAC group, RPE was significantly smaller in H2 group at each workload phase (p < 0.032) (Table 3).
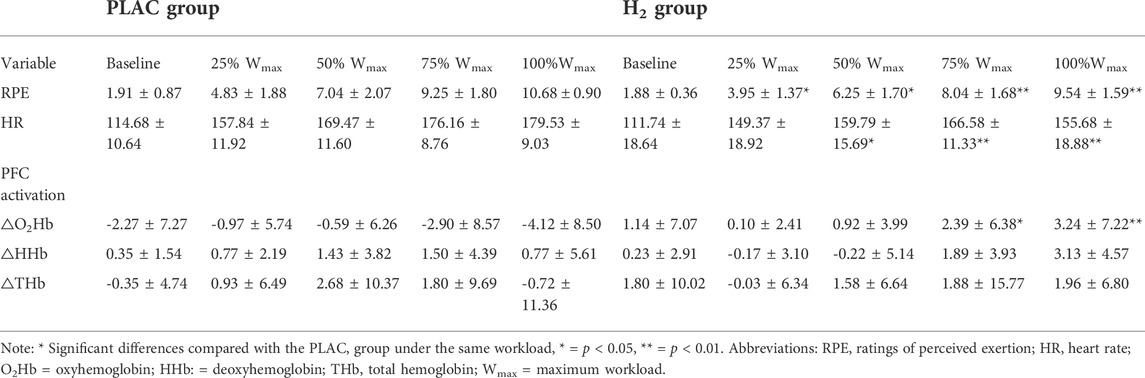
TABLE 3. RPE, HR and PFC activation during the cycling exercise in the PLAC and H2 groups (mean ± SD).
Additionally, two-way mixed design ANOVA model showed an interaction of group and workload on HR (F = 5.616, p = 0.007). The post-hoc analysis showed that the HR of the H2 group was significantly smaller than that of the PLAC group at 50%, 75% and 100% of the maximum workload phase (p < 0.037) (Table 3). The One-way ANCOVA model showed that after adjusting the pre-test MVIC, there was only a marginally significant difference between the post-test MVIC in H2 gas and PLAC group (34.38 ± 1.76 vs. 34.03 ± 1.84; F = 3.341, p = 0.074).
The effects of H2 gas on PFC activation
Two-way mixed design ANOVA model showed a significant interaction of group and workload on △O2Hb (F = 3.357, p = 0.030). The post-hoc analysis showed that the △O2Hb in H2 group was significantly higher than that of the PLAC group at 75%Wmax and 100%Wmax (p < 0.011). Similarly, a significant interaction of △HHb was also observed (F = 4.076, p = 0.016); however, only marginally significant difference of it between H2 and PLAC groups at 100%Wmax (p = 0.058) was observed and not any other significance was presented (Table 3; Figure 2).
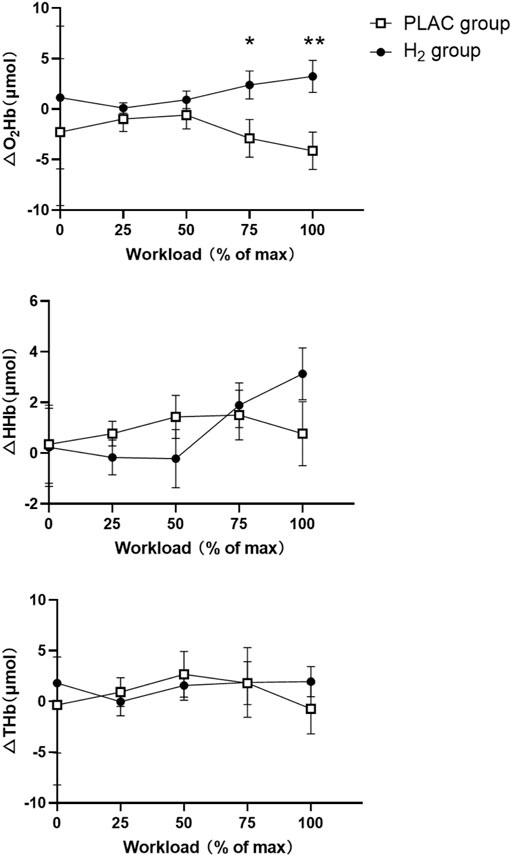
FIGURE 2. PFC activation during the cycling exercise in the PLAC and H2 groups. Note: * Significant differences compared with the PLAC group under the same workload, * = p < 0.05, ** = p < 0.01. Abbreviations: O2Hb = oxyhemoglobin; HHb = deoxyhemoglobin; THb = total hemoglobin.
The associations between physical fatigue and PFC activation
Based upon the results above, we performed linear regression analysis to examine the associations between the H2-induced changes in RPE, HR and △O2Hb at 75% Wmax and 100% Wmax. It was observed that the H2-induced change in PF was significantly associated with that in PFC activation, that is, participants with greater percent increase of △O2Hb had greater percent reduction in RPE at 75% Wmax (r = 0.577, p = 0.010). Similarly, Participants with greater increase of △O2Hb had greater percent reduction in HR at 100% Wmax (r = 0.576, p = 0.016). No other significant associations were observed (r < -0.013, p > 0.959) (Figure 3).
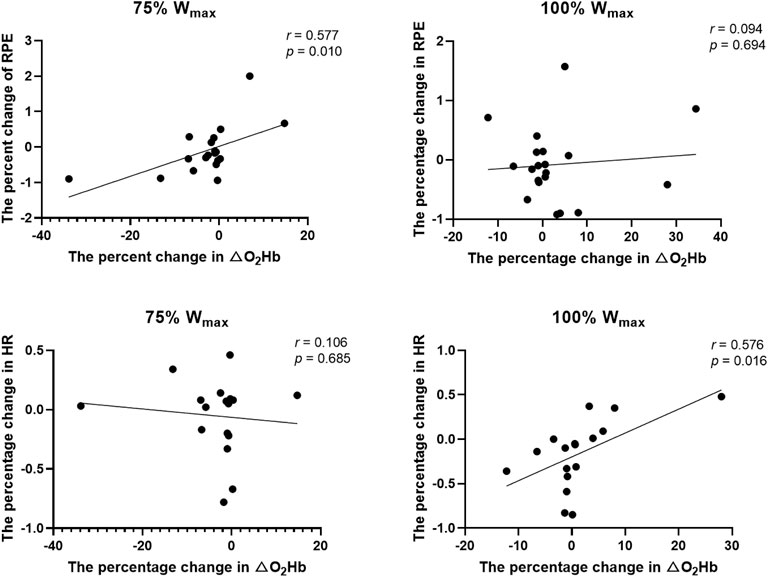
FIGURE 3. The associations between the H2-induced change in the PF and that of the PFC activation. Abbreviations: RPE = ratings of perceived exertion; HR = heart rate; Wmax = maximum workload.
Discussion
This study demonstrated that pre-exercise inhalation of H2 gas can alleviate PF and maintain the PFC activation during high-intensity exercise in healthy young adults. More importantly, this study provides first-of-its-kind evidence that the reductions in PF as induced by H2 gas was associated with H2-induced increase in PFC activation, suggesting a potential “central” pathway that H2 may alleviate the PF by altering the activation of brain cortical regions. The findings of this study provide novel insights into the mechanisms underlying the benefits of H2 for PF, helping the design of appropriate strategies for fatigue management.
The anti-fatigue effects of H2 gas and its related mechanism
We observed that H2 gas attenuated RPE during different phases of task (i.e., different workloads) and could significantly reduce HR under near-maximal workload. These are consistent with the observations in other studies using HRW (Botek et al., 2019; LeBaron et al., 2019; Mikami et al., 2019). Though the inhalation of gas form of H2 is currently not a commonly used method, studies have suggested that H2 gas can deliver large amounts of H2 to the living body quickly through a ventilator circuit or nasal cannula (Kawamura et al., 2020) without risk of explosion even the concentration in the air is below 4% (Ohta, 2011). Furthermore, studies have observed that ingesting H2 before exercise can induce anti-fatigue effects and thus benefit endurance capacity (Botek et al., 2019; Mikami et al., 2019). Taken together, we here provide confirmatory but novel evidence that pre-exercise inhalation of H2 gas can significantly alleviate PF in healthy participants. Future studies can directly compare the effects of H2 gas and HRW, and examine the most appropriate timing of the inhalation of H2 (i.e., pre, during or post-exercise) for fatigue attenuation.
It is observed that H2 inhalation increased the PFC activation during the high-workload phase, as assessed by △O2Hb of fNIRS. The brain is particularly sensitive to ROS (Magistretti and Allaman, 2015) and excessed ROS may damage neurons (Halliwell, 1996) and mitochondrial membranes of the brain, leading to increased proton leakage during mitochondrial energy production (Brookes et al., 1998; Brookes, 2005; Nanayakkara et al., 2019) and reduced efficiency of energy production. H2 gas can cross the blood-brain barrier (Hayashida et al., 2012) and selectively scavenge ROS (Ohsawa et al., 2007), helping reduce the damage to neuronal cells. In addition, antioxidant supplements such as resveratrol have been shown to balance redox status and improve brain mitochondrial function (Murphy and Hartley, 2018). Therefore, it may indicate that H2 gas could act as a powerful antioxidant to improve brain mitochondrial function and increase energy efficiency by reducing unnecessary oxygen wastage due to proton leakage, resulting in high level of cortical activation during high-intensity exercise. Future studies implementing technique (e.g., respirometry) to assess the bio-neurophysiological characteristics (e.g., mitochondrial function) related to fatigue are needed to explore the underlying pathological mechanism through which the H2 can help eliminate the alterations of fatigue on brain functions.
More importantly, we here observed direct association between H2-induced improvements in RPE and HR and that in PFC activation. The PFC is the region for the processing of directing, planning and decision making, integrating cognitive and afferent feedback from cardiovascular organs and motor muscles to regulate movement by modulating motor drive (Robertson and Marino, 2016). Specifically, the PFC may have a motivational function that allows continued movement by suppressing those peripheral fatigue signals that want to stop the movement (Miller and Cohen, 2001; Perrey et al., 2016). Therefore, PFC might help delay the onset of fatigue by inhibiting fatigue signals and decreasing subjective effort to increase tolerance to exercise load, leading to lower RPE during exercise. Meanwhile, studies have demonstrated that PFC directs a “neurovisceral integration” system that integrates external environmental stimuli for adaptive regulation of cardiac function, and that increased activation of PFC is associated with enhanced parasympathetic tone (Thayer et al., 2012). Therefore, the observation of decreased HR as induced by H2 gas may be due to the enhanced parasympathetic activity via H2-induced increase in PFC activation as well.
The demand to characterize the effects of H2 on PF induced by different types of exercise
To note, in several conditions, the inhalation of H2 gas did not induce significant improvements on PF (e.g., MVIC), and the associations between the H2-induced changes in the activation of PFC and in PF (e.g., changes in △O2Hb and RPE at 100% Wmax) were also not significant. This may indicate that the relative contribution of peripheral components to fatigue may increase in these conditions, and more focus on the development/progress of PF in different types of exercises are needed to future characterize the benefits of intaking H2 for the alleviation of PF. For example, both high-intensity interval exercise (HIIE) and endurance exercise (EE) can significantly induce PF, but studies have shown that the functional changes (e.g., changes in cardiorespiratory coupling) in response to the PF as induced by these two types of exercise differed (e.g., changes in cardiorespiratory coupling) (Andrade et al., 2020). Specifically, it was observed that the HIIE induced similar changes in muscle mitochondrial function to that induced by EE (Trewin et al., 2018), but may not induce central fatigue (e.g., the central activation ratio of the quadriceps muscle did not change before and after the HIIE) (Jurgelaitiene et al., 2021) which is oftentimes observed after EE (Azevedo et al., 2021). To this point, it is thus highly demanded in future studies to explicitly characterize the effects of different types of exercise protocol on PF and then to more comprehensively characterize the mechanisms through which H2 helps alleviate PF resulted from different types of exercise.
Limitations
Several limitations should be noted in this study. The sample size of participants here was small and only men were recruited; some data were missing or with low quality (e.g., fNIRS). Additionally, only the immediate effects of H2 on fatigue and cortical activation were examined. Future studies with a larger sample size and implementing longer term of intervention/exercise are thus highly demanded to examine and confirm the observations in this study and to explore the longer-term effects of repeated inhalation of H2 gas. The underlying pathway through which H2 gas influences PF and PFC activation needs to be explicitly examined in future studies with more sophisticated biophysiological and biochemical assessments. This will ultimately provide critical knowledge for the optimization of H2-based strategies for attenuating PF induced by exercise. Nevertheless, this study provides novel evidence suggesting that the pre-exercise inhalation of H2 may help reduce the fatigue during high-intensity exercise via a potential “central” pathway.
Data availability statement
The raw data supporting the conclusions of this article will be made available by the authors, without undue reservation.
Ethics statement
The studies involving human participants were reviewed and approved by the Institutional Review Board of Beijing Sport University. The patients/participants provided their written informed consent to participate in this study.
Author contributions
DB, YH, and DD: Design and/or conceptualization of the study. YH, DD, GJ and ML: Data collection. YH, JZ, DD and DB: Analysis and/or interpretation of the data. YH, JZ, QL, VW and DB: Drafting and/or revising the manuscript. All authors have read and agreed to the published version of the manuscript.
Funding
This study was supported by the National Key Research and Development Program of China (Grant No. 2018YFC2000602 and 2019YFF030160).
Acknowledgments
The authors thank Yubo Wang, Yiting Li, Jialin Jiahengnuer, Qiushi Wang and Jiya He for their help during the research experiments, and thank all participants for their time and effort.
Conflict of interest
The authors declare that the research was conducted in the absence of any commercial or financial relationships that could be construed as a potential conflict of interest.
Publisher’s note
All claims expressed in this article are solely those of the authors and do not necessarily represent those of their affiliated organizations, or those of the publisher, the editors and the reviewers. Any product that may be evaluated in this article, or claim that may be made by its manufacturer, is not guaranteed or endorsed by the publisher.
References
Allen D. G., Lamb G. D., Westerblad H. (2008). Skeletal muscle fatigue: Cellular mechanisms. Physiol. Rev. 88, 287–332. doi:10.1152/physrev.00015.2007
Andrade D. C., Arce‐Alvarez A., Parada F., Uribe S., Gordillo P., Dupre A., et al. (2020). Acute effects of high‐intensity interval training session and endurance exercise on pulmonary function and cardiorespiratory coupling. Physiol. Rep. 8, e14455. doi:10.14814/phy2.14455
Aoki K., Nakao A., Adachi T., Matsui Y., Miyakawa S. (2012). Pilot study: Effects of drinking hydrogen-rich water on muscle fatigue caused by acute exercise in elite athletes. Med. Gas. Res. 2, 12. doi:10.1186/2045-9912-2-12
Azevedo R. de A., Silva-Cavalcante M. D., Lima-Silva A. E., Bertuzzi R. (2021). Fatigue development and perceived response during self-paced endurance exercise: State-of-the-art review. Eur. J. Appl. Physiol. 121, 687–696. doi:10.1007/s00421-020-04549-5
Birfer R., Sonne M. W., Holmes M. W. (2019). Manifestations of muscle fatigue in baseball pitchers: A systematic review. PeerJ 7, e7390. doi:10.7717/peerj.7390
Borg G. A. (1982). Psychophysical bases of perceived exertion. Med. Sci. Sports Exerc. 14, 377–381. doi:10.1249/00005768-198205000-00012
Botek M., Krejčí J., McKune A. J., Sládečková B., Naumovski N. (2019). Hydrogen rich water improved ventilatory, perceptual and lactate responses to exercise. Int. J. Sports Med. 40, 879–885. doi:10.1055/a-0991-0268
Brookes P. S., Land J. M., Clark J. B., Heales S. J. (1998). Peroxynitrite and brain mitochondria: Evidence for increased proton leak. J. Neurochem. 70, 2195–2202. doi:10.1046/j.1471-4159.1998.70052195.x
Brookes P. S. (2005). Mitochondrial H(+) leak and ROS generation: An odd couple. Free Radic. Biol. Med. 38, 12–23. doi:10.1016/j.freeradbiomed.2004.10.016
Cobley J. N., Fiorello M. L., Bailey D. M. (2018). 13 reasons why the brain is susceptible to oxidative stress. Redox Biol. 15, 490–503. doi:10.1016/j.redox.2018.01.008
Cordeiro L. M. S., Rabelo P. C. R., Moraes M. M., Teixeira-Coelho F., Coimbra C. C., Wanner S. P., et al. (2017). Physical exercise-induced fatigue: The role of serotonergic and dopaminergic systems. Braz J. Med. Biol. Res. 50, e6432. doi:10.1590/1414-431X20176432
Crewe H., Tucker R., Noakes T. D. (2008). The rate of increase in rating of perceived exertion predicts the duration of exercise to fatigue at a fixed power output in different environmental conditions. Eur. J. Appl. Physiol. 103, 569–577. doi:10.1007/s00421-008-0741-7
Da Ponte A., Giovanelli N., Nigris D., Lazzer S. (2018). Effects of hydrogen rich water on prolonged intermittent exercise. J. Sports Med. Phys. Fit. 58, 612–621. doi:10.23736/S0022-4707.17.06883-9
De Wachter J., Proost M., Habay J., Verstraelen M., Díaz-García J., Hurst P., et al. (2021). Prefrontal cortex oxygenation during endurance performance: A systematic review of functional near-infrared spectroscopy studies. Front. Physiol. 12, 761232. doi:10.3389/fphys.2021.761232
Dobashi S., Takeuchi K., Koyama K. (2020). Hydrogen-rich water suppresses the reduction in blood total antioxidant capacity induced by 3 consecutive days of severe exercise in physically active males. Med. Gas. Res. 10, 21–26. doi:10.4103/2045-9912.279979
Dong G., Fu J., Bao D., Zhou J. (2022). Short-term consumption of hydrogen-rich water enhances power performance and heart rate recovery in dragon boat athletes: Evidence from a pilot study. Int. J. Environ. Res. Public Health 19, 5413. doi:10.3390/ijerph19095413
Enoka R. M. (1995). Mechanisms of muscle fatigue: Central factors and task dependency. J. Electromyogr. Kinesiol. 5, 141–149. doi:10.1016/1050-6411(95)00010-w
Fisher-Wellman K., Bloomer R. J. (2009). Acute exercise and oxidative stress: A 30 year history. Dyn. Med. 8, 1. doi:10.1186/1476-5918-8-1
Gandevia S. C. (2001). Spinal and supraspinal factors in human muscle fatigue. Physiol. Rev. 81, 1725–1789. doi:10.1152/physrev.2001.81.4.1725
Halliwell B. (1996). Free radicals, proteins and DNA: Oxidative damage versus redox regulation. Biochem. Soc. Trans. 24, 1023–1027. doi:10.1042/bst0241023
Hayashida K., Sano M., Kamimura N., Yokota T., Suzuki M., Maekawa Y., et al. (2012). H(2) gas improves functional outcome after cardiac arrest to an extent comparable to therapeutic hypothermia in a rat model. J. Am. Heart Assoc. 1, e003459. doi:10.1161/JAHA.112.003459
Hori A., Sobue S., Kurokawa R., Hirano S.-I., Ichihara M., Hotta N. (2020). Inhalation of molecular hydrogen increases breath acetone excretion during submaximal exercise: A randomized, single-blinded, placebo-controlled study. Med. Gas. Res. 10, 96–102. doi:10.4103/2045-9912.296038
Hoshi Y., Kobayashi N., Tamura M. (2001). Interpretation of near-infrared spectroscopy signals: A study with a newly developed perfused rat brain model. J. Appl. Physiol. 90, 1657–1662. doi:10.1152/jappl.2001.90.5.1657
Huygaerts S., Cos F., Cohen D. D., Calleja-González J., Guitart M., Blazevich A. J., et al. (2020). Mechanisms of hamstring strain injury: Interactions between fatigue, muscle activation and function. Sports (Basel) 8, E65. doi:10.3390/sports8050065
Hyland-Monks R., Cronin L., McNaughton L., Marchant D. (2018). The role of executive function in the self-regulation of endurance performance: A critical review. Prog. Brain Res. 240, 353–370. doi:10.1016/bs.pbr.2018.09.011
James D. V. B., Barnes A. J., Lopes P., Wood D. M. (2002). Heart rate variability: Response following a single bout of interval training. Int. J. Sports Med. 23, 247–251. doi:10.1055/s-2002-29077
Javorac D., Stajer V., Ratgeber L., Betlehem J., Ostojic S. (2019). Short-term H2 inhalation improves running performance and torso strength in healthy adults. Biol. Sport 36, 333–339. doi:10.5114/biolsport.2019.88756
Jung R., Moser M., Baucsek S., Dern S., Schneider S. (2015). Activation patterns of different brain areas during incremental exercise measured by near-infrared spectroscopy. Exp. Brain Res. 233, 1175–1180. doi:10.1007/s00221-015-4201-4
Jurgelaitiene G., Satas A., Cekanauskaite A., Motiejunaite K., Valanciene D., Stanislovaitiene J., et al. (2021). High-volume intermittent maximal intensity isometric exercise caused great stress, although central motor fatigue did not occur. Biol. Sport 38, 315–323. doi:10.5114/biolsport.2021.99322
Kawamura T., Higashida K., Muraoka I. (2020). Application of molecular hydrogen as a novel antioxidant in sports science. Oxid. Med. Cell. Longev. 2020, 2328768. doi:10.1155/2020/2328768
Kawamura T., Muraoka I. (2018). Exercise-induced oxidative stress and the effects of antioxidant intake from a physiological viewpoint. Antioxidants (Basel) 7, E119. doi:10.3390/antiox7090119
Korovljev D., Stajer V., Javorac D., Ostojic S. M. (2018). Hydrogen inhalation positively affects cardiometabolic risk factors in men and women aged 65 years or older: A preliminary report. Eur. Geriatr. Med. 9, 729–730. doi:10.1007/s41999-018-0087-6
LeBaron T. W., Larson A. J., Ohta S., Mikami T., Barlow J., Bulloch J., et al. (2019). Acute supplementation with molecular hydrogen benefits submaximal exercise indices. Randomized, double-blinded, placebo-controlled crossover pilot study. J. Lifestyle Med. 9, 36–43. doi:10.15280/jlm.2019.9.1.36
LeBlanc J., Boulay M., Dulac S., Jobin M., Labrie A., Rousseau-Migneron S. (1977). Metabolic and cardiovascular responses to norepinephrine in trained and nontrained human subjects. J. Appl. Physiol. Respir. Environ. Exerc. Physiol. 42, 166–173. doi:10.1152/jappl.1977.42.2.166
Magistretti P. J., Allaman I. (2015). A cellular perspective on brain energy metabolism and functional imaging. Neuron 86, 883–901. doi:10.1016/j.neuron.2015.03.035
McCarty M. F. (2015). Potential ghrelin-mediated benefits and risks of hydrogen water. Med. Hypotheses 84, 350–355. doi:10.1016/j.mehy.2015.01.018
Mikami T., Tano K., Lee H., Lee H., Park J., Ohta F., et al. (2019). Drinking hydrogen water enhances endurance and relieves psychometric fatigue: A randomized, double-blind, placebo-controlled study 1. Can. J. Physiol. Pharmacol. 97, 857–862. doi:10.1139/cjpp-2019-0059
Miller E. K., Cohen J. D. (2001). An integrative theory of prefrontal cortex function. Annu. Rev. Neurosci. 24, 167–202. doi:10.1146/annurev.neuro.24.1.167
Mizuno K., Sasaki A. T., Ebisu K., Tajima K., Kajimoto O., Nojima J., et al. (2017). Hydrogen-rich water for improvements of mood, anxiety, and autonomic nerve function in daily life. Med. Gas. Res. 7, 247–255. doi:10.4103/2045-9912.222448
Murphy M. P., Hartley R. C. (2018). Mitochondria as a therapeutic target for common pathologies. Nat. Rev. Drug Discov. 17, 865–886. doi:10.1038/nrd.2018.174
Nanayakkara G. K., Wang H., Yang X. (2019). Proton leak regulates mitochondrial reactive oxygen species generation in endothelial cell activation and inflammation - a novel concept. Arch. Biochem. Biophys. 662, 68–74. doi:10.1016/j.abb.2018.12.002
Nybo L., Rasmussen P., Sawka M. N. (2014). Performance in the heat-physiological factors of importance for hyperthermia-induced fatigue. Compr. Physiol. 4, 657–689. doi:10.1002/cphy.c130012
Obrig H., Villringer A. (2003). Beyond the visible-imaging the human brain with light. J. Cereb. Blood Flow. Metab. 23, 1–18. doi:10.1097/01.WCB.0000043472.45775.29
Ohsawa I., Ishikawa M., Takahashi K., Watanabe M., Nishimaki K., Yamagata K., et al. (2007). Hydrogen acts as a therapeutic antioxidant by selectively reducing cytotoxic oxygen radicals. Nat. Med. 13, 688–694. doi:10.1038/nm1577
Ohta S. (2011). Recent progress toward hydrogen medicine: Potential of molecular hydrogen for preventive and therapeutic applications. Curr. Pharm. Des. 17, 2241–2252. doi:10.2174/138161211797052664
Pan Y., Borragán G., Peigneux P. (2019). Applications of functional near-infrared spectroscopy in fatigue, sleep deprivation, and social cognition. Brain Topogr. 32, 998–1012. doi:10.1007/s10548-019-00740-w
Pantovic M., Jevtić N., Magoc D., Obradović J., Madić D., Howatson G. (2016). Molecular hydrogen supplementation and multiple sprint running performance; a pilot investigation.: 903 board #219 june 1, 3: 30 pm - 5: 00 pm. Med. Sci. Sports Exerc. 48, 254. doi:10.1249/01.mss.0000485766.21001.be
Perrey S., Radel R., Brisswalter J. (2016). Exercice termination is a cognitively controlled decision. J. Appl. Physiol. 120, 467–469. doi:10.1152/japplphysiol.00967.2015
Piper S. K., Krueger A., Koch S. P., Mehnert J., Habermehl C., Steinbrink J., et al. (2014). A wearable multi-channel fNIRS system for brain imaging in freely moving subjects. NeuroImage 85, 64–71. doi:10.1016/j.neuroimage.2013.06.062
Portier H., Louisy F., Laude D., Berthelot M., Guézennec C. Y. (2001). Intense endurance training on heart rate and blood pressure variability in runners. Med. Sci. Sports Exerc. 33, 1120–1125. doi:10.1097/00005768-200107000-00009
Radel R., Brisswalter J., Perrey S. (2017). Saving mental effort to maintain physical effort: A shift of activity within the prefrontal cortex in anticipation of prolonged exercise. Cogn. Affect. Behav. Neurosci. 17, 305–314. doi:10.3758/s13415-016-0480-x
Rasmussen P., Secher N. H., Petersen N. T. (2007). Understanding central fatigue: Where to go? Exp. Physiol. 92, 369–370. doi:10.1113/expphysiol.2006.036632
Robertson C. V., Marino F. E. (2016). A role for the prefrontal cortex in exercise tolerance and termination. J. Appl. Physiol. 120, 464–466. doi:10.1152/japplphysiol.00363.2015
Santos-Concejero J., Billaut F., Grobler L., Oliván J., Noakes T. D., Tucker R. (2015). Maintained cerebral oxygenation during maximal self-paced exercise in elite Kenyan runners. J. Appl. Physiol. 118, 156–162. doi:10.1152/japplphysiol.00909.2014
Schmitz C. H., Klemer D. P., Hardin R., Katz M. S., Pei Y., Graber H. L., et al. (2005). Design and implementation of dynamic near-infrared optical tomographic imaging instrumentation for simultaneous dual-breast measurements. Appl. Opt. 44, 2140–2153. doi:10.1364/ao.44.002140
Schneider P., Piper S., Schmitz C. H., Schreiter N. F., Volkwein N., Lüdemann L., et al. (2011). Fast 3D Near-infrared breast imaging using indocyanine green for detection and characterization of breast lesions. Rofo 183, 956–963. doi:10.1055/s-0031-1281726
Scholkmann F., Kleiser S., Metz A. J., Zimmermann R., Mata Pavia J., Wolf U., et al. (2014). A review on continuous wave functional near-infrared spectroscopy and imaging instrumentation and methodology. Neuroimage 85, 6–27. doi:10.1016/j.neuroimage.2013.05.004
Shibayama Y., Dobashi S., Arisawa T., Fukuoka T., Koyama K. (2020). Impact of hydrogen-rich gas mixture inhalation through nasal cannula during post-exercise recovery period on subsequent oxidative stress, muscle damage, and exercise performances in men. Med. Gas. Res. 10, 155–162. doi:10.4103/2045-9912.304222
Siemionow V., Yue G. H., Ranganathan V. K., Liu J. Z., Sahgal V. (2000). Relationship between motor activity-related cortical potential and voluntary muscle activation. Exp. Brain Res. 133, 303–311. doi:10.1007/s002210000382
St Clair Gibson A., De Koning J. J., Thompson K. G., Roberts W. O., Micklewright D., Raglin J., et al. (2013). Crawling to the finish line: Why do endurance runners collapse? Implications for understanding of mechanisms underlying pacing and fatigue. Sports Med. 43, 413–424. doi:10.1007/s40279-013-0044-y
Thayer J. F., Ahs F., Fredrikson M., Sollers J. J., Wager T. D. (2012). A meta-analysis of heart rate variability and neuroimaging studies: Implications for heart rate variability as a marker of stress and health. Neurosci. Biobehav. Rev. 36, 747–756. doi:10.1016/j.neubiorev.2011.11.009
Timon Andrada R., Olcina G., González-Custodio A., Camacho-Cardenosa M., Camacho-Cardenosa A., Martínez-Guardado I. (2020). Effects of 7-day intake of hydrogen-rich water on physical performance of trained and untrained subjects. Biol. Sport 38, 269–275. doi:10.5114/biolsport.2020.98625
Tomofuji T., Kawabata Y., Kasuyama K., Endo Y., Yoneda T., Yamane M., et al. (2014). Effects of hydrogen-rich water on aging periodontal tissues in rats. Sci. Rep. 4, 5534. doi:10.1038/srep05534
Trewin A. J., Parker L., Shaw C. S., Hiam D. S., Garnham A., Levinger I., et al. (2018). Acute HIIE elicits similar changes in human skeletal muscle mitochondrial H2O2 release, respiration, and cell signaling as endurance exercise even with less work. Am. J. Physiol. Regul. Integr. Comp. Physiol. 315, R1003–R1016. doi:10.1152/ajpregu.00096.2018
Keywords: physical fatigue, prefrontal cortex, H2 gas, fNIRS, anti-oxidation
Citation: Hong Y, Dong G, Li Q, Wang V, Liu M, Jiang G, Bao D and Zhou J (2022) Effects of pre-exercise H2 inhalation on physical fatigue and related prefrontal cortex activation during and after high-intensity exercise. Front. Physiol. 13:988028. doi: 10.3389/fphys.2022.988028
Received: 06 July 2022; Accepted: 09 August 2022;
Published: 02 September 2022.
Edited by:
Hamid Arazi, University of Guilan, IranReviewed by:
Akram Falahati, University of Oklahoma, United StatesAli Sayyah, University of Zanjan, Iran
Copyright © 2022 Hong, Dong, Li, Wang, Liu, Jiang, Bao and Zhou. This is an open-access article distributed under the terms of the Creative Commons Attribution License (CC BY). The use, distribution or reproduction in other forums is permitted, provided the original author(s) and the copyright owner(s) are credited and that the original publication in this journal is cited, in accordance with accepted academic practice. No use, distribution or reproduction is permitted which does not comply with these terms.
*Correspondence: Dapeng Bao, baodp@bsu.edu.cn