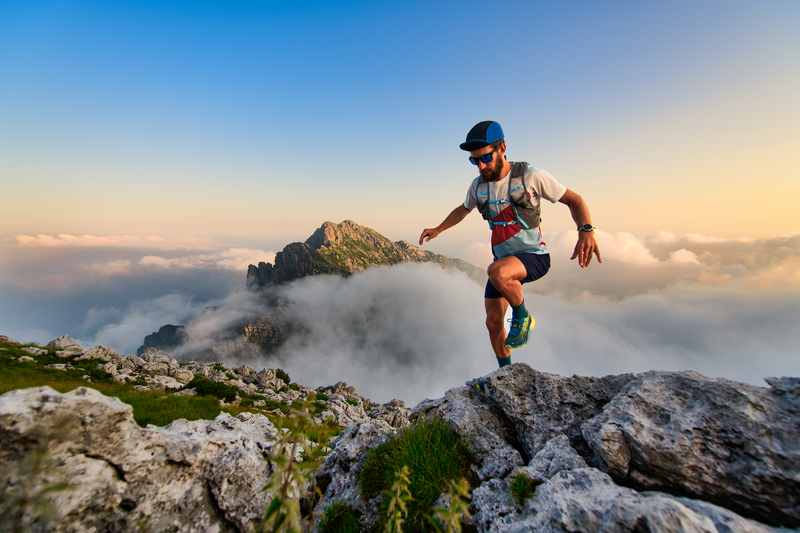
95% of researchers rate our articles as excellent or good
Learn more about the work of our research integrity team to safeguard the quality of each article we publish.
Find out more
ORIGINAL RESEARCH article
Front. Physiol. , 31 August 2022
Sec. Exercise Physiology
Volume 13 - 2022 | https://doi.org/10.3389/fphys.2022.979359
This article is part of the Research Topic Oxygen Delivery:Demand Matching in Exercising Skeletal Muscle View all 7 articles
Parts of this article's content have been modified or rectified in:
Erratum: Regulation of the microvasculature during small muscle mass exercise in chronic obstructive pulmonary disease vs. chronic heart failure
Aim: Skeletal muscle convective and diffusive oxygen (O2) transport are peripheral determinants of exercise capacity in both patients with chronic obstructive pulmonary disease (COPD) and chronic heart failure (CHF). We hypothesised that differences in these peripheral determinants of performance between COPD and CHF patients are revealed during small muscle mass exercise, where the cardiorespiratory limitations to exercise are diminished.
Methods: Eight patients with moderate to severe COPD, eight patients with CHF (NYHA II), and eight age- and sex-matched controls were studied. We measured leg blood flow (Q̇leg) by Doppler ultrasound during submaximal one-legged knee-extensor exercise (KEE), while sampling arterio-venous variables across the leg. The capillary oxyhaemoglobin dissociation curve was reconstructed from paired femoral arterial-venous oxygen tensions and saturations, which enabled the estimation of O2 parameters at the microvascular level within skeletal muscle, so that skeletal muscle oxygen conductance (DSMO2) could be calculated and adjusted for flow (DSMO2/Q̇leg) to distinguish convective from diffusive oxygen transport.
Results: During KEE, Q̇leg increased to a similar extent in CHF (2.0 (0.4) L/min) and controls (2.3 (0.3) L/min), but less in COPD patients (1.8 (0.3) L/min) (p <0.03). There was no difference in resting DSMO2 between COPD and CHF and when adjusting for flow, the DSMO2 was higher in both groups compared to controls (COPD: 0.97 (0.23) vs. controls 0.63 (0.24) mM/kPa, p= 0.02; CHF 0.98 (0.11) mM/kPa vs. controls, p= 0.001). The Q̇-adjusted DSMO2 was not different in COPD and CHF during KEE (COPD: 1.19 (0.11) vs. CHF: 1.00 (0.18) mM/kPa; p= 0.24) but higher in COPD vs. controls: 0.87 (0.28) mM/kPa (p= 0.02), and only CHF did not increase Q̇-adjusted DSMO2 from rest (p= 0.2).
Conclusion: Disease-specific factors may play a role in peripheral exercise limitation in patients with COPD compared with CHF. Thus, low convective O2 transport to contracting muscle seemed to predominate in COPD, whereas muscle diffusive O2 transport was unresponsive in CHF.
Exercise intolerance is a cardinal feature of both chronic obstructive pulmonary disease (COPD) and chronic heart failure (CHF) (Vestbo et al., 2013; Buber and Robertson, 2022). The associated decline in peak oxygen uptake (V̇O2peak) during whole-body exercise in these disease states has been attributed to ‘central’ factors, i.e., cardio-pulmonary limitations, but emerging evidence suggests that peripheral factors, such as limb muscle dysfunction, also contributes to the exercise intolerance (Maltais et al., 2014; Keller-Ross et al., 2019). The limb muscle dysfunction, characterised by a low oxidative enzyme capacity, fibre type shift (type I to II) and muscle wasting, is quite similar in COPD and CHF (Zizola and Schulze, 2013; Ausín et al., 2017). Thus, studying how the skeletal muscle maladapts to chronic pulmonary or cardiac disease may enhance our understanding of the peripheral contribution to exercise intolerance in these common disorders.
The diminished V̇O2peak in both COPD and CHF during whole-body exercise may be explained by any limiting factor of the integrated O2 transport system, including ventilation, alveolar-to-capillary diffusion, cardiac output, blood volume, muscle blood supply and muscle diffusion (Wagner, 1996; Houstis et al., 2018). Whole body V̇O2peak testing provoke cardio-respiratory symptoms in COPD and CHF due to the large muscle mass activated, thus masking any peripheral skeletal muscle limitations (Mortensen and Saltin, 2014; Poole et al., 2018; Dempsey, 2019). Small muscle mass exercise using one-legged knee-extensor exercise (KEE) is an established method to determine peripheral limitations to V̇O2peak in COPD and CHF, because both cardiac output and minute ventilation are affected to a lesser degree compared to whole body exercise (Mortensen et al., 2010). Importantly, a higher skeletal muscle peak oxygen uptake (V̇O2SMpeak) may be obtained during KEE compared to whole-body exercise in these patients (Richardson et al., 2004; Barrett-O’Keefe et al., 2014).
Oxygen transport to skeletal muscle determines peripheral exercise capacity, which depends on the convective delivery of blood flow (Q̇) to the capillary bed and diffusive transport from red blood cells to myocyte mitochondria (Poole et al., 2022). Blood flow to contracting muscle is tightly regulated to match oxygen delivery to metabolic demand in healthy humans (Saltin, 1985; Mortensen and Saltin, 2014). The pulmonary diffusion deficits in COPD or reduced cardiac output in CHF are likely to impact regulation of muscle convective and diffusive O2 transport in response to exercise, as both diseases share limb muscle maladaptions abnormalities. Notwithstanding, the neural and metabolic interactions, that may also contribute to the peripheral exercise intolerance (Gagnon et al., 2012; Maltais et al., 2014; Smith et al., 2020). Indeed, previous findings suggest that both peripheral muscle O2 convective delivery (Brønstad et al., 2012) and diffusive O2 transport are impaired during maximal KEE in COPD (Broxterman et al., 2021), while muscle convective O2 delivery has been shown to be attenuated in CHF (Barrett-O’Keefe et al., 2014, 2019).
As of now, no studies have directly compared the peripheral limitations of the oxygen transport cascade in these predominantly centrally limited disease states to determine whether disease-specific microvascular (mal)adaptations are present. In the present study, we hypothesised that such disease-specific differences between the COPD and CHF patients are present, with COPD showing both convective as well as diffusive limitation whereas CHF are mainly limited on their convective capacity compared to controls.
The studies were approved by the Ethical Committee of the Capital Region of Copenhagen (H-2–2013–150; H-3–2013–048) and performed according to the guidelines of the Declaration of Helsinki. All subjects provided oral and written informed consent prior to enrolment. Experiments were performed in the same laboratory as a part of a larger study and some results have previously been published (Iepsen et al., 2017; Munch et al., 2018). The present study involves novel analyses of arterio-venous blood gases in response to KEE from the two studies to address an independent working hypothesis.
We included eight COPD patients (Global Initiative for Chronic Obstructive Lung Disease (GOLD) II and III), eight patients with heart failure with reduced ejection fraction (HFrEF) (New York Heart Association Classification (NYHA) class II), and eight healthy age- and sex-matched controls (Table 1). In the previous study by Iepsen et al., 10 COPD patients were included, but due to technical issues with placement of the arterial catheters, two could not participate in the invasive part of the study (Iepsen et al., 2017). In the study by Munch et al. (Munch et al., 2018), eight healthy controls were included, but two of them did not want to participate in the training intervention and were only enrolled in the cross-sectional study. Due to the invasive nature of this experiment, the control group was the same for both studies. Thus, we studied 8 COPD patients, 8 CHF patients and 8 healthy controls unexposed to exercise training. In one of the COPD patients, we could only calculate arterial blood gas values at rest, and therefore, we excluded this subject from the exercise analyses.
Inclusion criteria for COPD patients were a forced expiratory volume in 1 s (FEV1)/forced vital capacity ratio <0.70, FEV1 ≤ 70% of predicted, modified Medical Research Council Dyspnoea Scale ≤2, resting arterial oxygenation >90%, and age 40–80 years. Eligibility criteria for CHF patients were left ventricle ejection fraction <40%, NYHA class < eller lig II and age 40–80 years. All patients were clinically stable and primarily recruited from outpatient clinics. Exclusion criteria were use of anticoagulant medications, diabetes, hypertension, claudication, heart failure (COPD and controls) COPD (CHF and controls), unstable ischemic heart disease or malignant diseases. Sitting spirometry (Model 2120, Vitalograph Ltd. Buckingham, United Kingdom) in COPD, standard echocardiography (Vivid 9, GE Healthcare, Pittsburgh, PA) in CHF, and a general medical examination including blood testing prior to inclusion.
Prior to the experimental day all subjects visited the laboratory to be familiarised to the KEE-model, and performed; (1) an incremental bicycle test on a bicycle ergometer (Monark 839E; Monark, Varberg, Sweden) to determine whole body V̇O2peak (l/min,) and peak workload (Wattpeak) while oxygen consumption was measured breath-by-breath using a Quark gas analyser (Cosmed, Italy) (2) an incremental KEE test, to determine peak workload, (3) 6 minutes walking distance (6MWD) and (4) whole-body dual-energy X-ray absorptiometry scanning (Lunar Prodigy Advance; GE Healthcare, Madison, WI).
On the experimental day participants refrained from caffeine, alcohol, and exercise for 24 h. The COPD patients paused their medications for 24–48 h depending on the drug profile to avoid potential vasoconstrictor effects of inhaled β2-agonists and muscarinic antagonists. The CHF patients paused their ACE-inhibitors 48 h prior to the experimental day, whereas beta-blockers, anti-platelet and diuretic drugs were withdrawn 24 h prior to the experimental day. After local anaesthesia (lidocaine 2%), catheters were placed in the femoral artery and vein of the experimental leg and one in the brachial artery.
KEE at 10 W was performed by all subjects using the same protocol. Prior to the acute exercise bout, the experimental leg was moved passively by an investigator at 60 kicks/min and subjects were instructed to keep that pace during exercise. Leg haemodynamics were evaluated, and arterio-venous blood samples were collected at baseline and during active knee-extensions at steady state (3.5 min of active contractions). Common femoral arterial blood flow was measured by the same sonographer using Doppler ultrasound (Logic E9, GE Healthcare, Milwaukee, WI, United States) equipped with a linear probe (9 MHz), as previously described (Iepsen et al., 2017; Munch et al., 2018). In brief, the site of the blood flow measurements was below the inguinal ligament but well above the bifurcation of the artery and recordings were obtained at the lowest possible insonation angle and always below 60˚. The sample volume was maximised according to the width of the vessel and kept clear of the walls. Arterial diameter was measured during systole from resting arterial B-mode images with the transducer parallel to the vessel. Doppler tracings were averaged over eight heart cycles at the same time as the blood sampling. Blood samples were drawn simultaneous from the femoral venous and arterial catheters and blood gases were immediately analysed (ABL 725, Radiometer, Glostrup, DK).
Oxygen content (CxO2) was calculated as:
Where S is the oxygen saturation of haemoglobin (fraction) and P is the partial pressure of oxygen (kPa). x denotes either arterial (a), femoral-venous (v), or capillary (cap) blood,
where avDO2 is the arterial-to-femoral venous oxygen content difference.
Skeletal muscle oxygen uptake (
Leg vascular conductance (LVC) was calculated as:
Where MAP is mean arterial blood pressure (=1/3 systolic blood pressure +2/3 diastolic blood pressure) or area under the curve (AUC) over 8 heart cycles for invasive experiments with pulse contour analysis.
Lastly, skeletal muscle oxygen conductance (DSMO2) was calculated as:
where
Given that i) the oxygen dissociation curve (ODC) is gradually modulated during the red blood cells’ passage though the microcirculation, e.g., due to changes in pH, PaCO2 and temperature, and ii) that the OCD may differ between individual capillaries throughout the skeletal muscle tissue, an ideal ‘averaged’ capillary ODC was modelled using paired arterial and femoral-venous blood gas values. For simplicity, and in accordance with our previous study (Dahl et al., 2020), ‘capillary’ here refers to the part of the microvasculature where gas exchange between blood and tissue takes place. However, it should be kept in mind, that this is not a well-defined anatomical entity, since gas exchange also takes place in other parts of the microvasculature, notably upstream and less so downstream of the anatomically defined capillary bed (Pittman, 2000; Shibata et al., 2006). Thus, it was assumed that measured blood gas values equal the oxygen tension and saturation in the arterial inlet and venous outlet of the gas exchanging microvasculature.
This averaged OCD was defined by the capillary P50 (P50cap), which reflects the dissociation constant of haemoglobin, and the capillary Hill coefficient, hcap, which is the cooperativity. A pair of P50cap- and hcap-values was calculated that fulfils the Hill equation in both arterial and femoral-venous blood (Dahl et al., 2020):
P50cap was then calculated by insertion of the Hill coefficient into the Hill equation using arterial or femoral-venous blood gas values.
The mean capillary oxygen tension (
where
Here
All statistical analyses were performed using R statistical software version 4.1.1 (R Project for Statistical Computing) within RStudio statistical software version 1.4.1717 (RStudio). Normality of data was confirmed by normality plots and homogeneity of variance was tested using the Bartlett test and data was collected in an independent manner. Changes from rest to KEE were analysed using a paired T-test. A one-way analysis of variance (ANOVA) was used to detect differences between groups and if the F test was significant (p <0.05), we used a Tukey’s post-hoc test for all group comparison combinations. Data are presented as mean (standard deviation), and differences are presented as mean. Significance was established at p <0.05. Effect sizes were calculated by Cohen’s D method.
Baseline characteristics are presented in Table 1. The V̇O2peak in the COPD group was similar to that of the CHF group, but COPD showed lower V̇O2peak compared to controls and there was no difference between CHF and controls The COPD and CHF group had similar 6MWD that were lower than in controls. The 10W workload during KEE comprised a similar percentage of maximal workload in the three groups (COPD: 33 (4) vs. CHF: 38 (23) vs. controls: 26 (7) %.
At rest, there were no differences in MAP (COPD: 110 (14) vs. CHF: 103 (8) vs. controls: 113 (11) mmHg; p=0.23), LVC (COPD: 2.3 (1.1) vs. CHF: 1.8 (0.5) vs. controls: 1.9 (0.2) ml/(min*mmHg); p=0.25) or Q̇leg (COPD: 222 (112) vs. CHF: 166 (43) vs. controls: 178 (22) ml/min; p=0.27) between the three groups.
During exercise, the Q̇leg, MAP and LVC all increased within both COPD, CHF, and controls (P<0.05). The change (Δ) from rest to exercise Q̇leg responses were similar in COPD vs. CHF, lower in COPD vs. controls, but similar in CHF vs. controls (Figure 1). There were no differences in ΔMAP between groups. Therefore, ΔLVC responses during KEE in COPD vs. CHF were not different, lower in COPD vs. controls, and similar in CHF vs. controls.
FIGURE 1. Haemodynamic responses. Panel (A) shows the change
Resting SaO2 and PaO2 were lower in the COPD group compared to the control group (Table 2). We found no differences in DelO2 between the three groups at rest (COPD: 2.1 (1.1) vs. CHF: 1.4 (0.4) vs. controls: 1.5 (0.1) mmol O2/min; p=0.2). Likewise, there was no difference in V̇O2SM between COPD and CHF at rest (COPD: 1.1 (0.2) vs. CHF: 0.9 (0.3) mmol O2/min, (p=0.57). Resting V̇O2SM was higher in COPD compared to controls (COPD: 1.1 (0.2) vs. controls: 0.7 (0.2) mmol O2/min; p=0.03), with no difference between CHF and controls groups.
FIGURE 2. Capillary oxygen tension and saturation Panel (A) shows the capillary oxygen tension (PcapO2) at rest, panel (B) the capillary oxygen tension during one leg knee-extensor exercise, panel (C) the oxygen saturation (Scap) at rest and panel (D) the oxygen saturation during one leg knee-extensor exercise. We performed a one-way ANOVA and if significant, a Tukey’s honest significant difference test was used to analyse the differences between groups. Difference between groups marked with a *= (p <0.05), **= (p <0.01), ***= (p <0.001). Dot plots with boxes showing mean and standard deviation with exact values for each participant, marked with a black dot. Chronic heart failure (CHF) participants n=8, Chronic obstructive pulmonary disease (COPD) participants n = 7, healthy control n = 8.
Exercising SaO2 and PaO2 were also lower in COPD vs. controls. We found no differences in DelO2 during KEE (COPD: 16.5 (3.3) vs. CHF: 17.0 (3.7) vs. controls: 20.6 (3.1) mmol O2/min; p=0.2). The ΔDelO2 was higher in controls compared to COPD in response to exercise (Figure 3), but similar to CHF, with no difference between COPD and CHF. The
FIGURE 3. Skeletal muscle oxygen delivery and conductance Panel (A) shows the change
At rest, there were no difference in DSMO2 between COPD and CHF (COPD: 0.21 (0.08) vs. CHF 0.16 (0.04) mmol/min/kPa; p=0.2), but DSMO2 was higher in both COPD and CHF than controls (0.11 (0.04) mmol/min/kPa, p=0.005). After Q̇-adjustment, there was still no difference in resting DSMO2 between COPD and CHF, and likewise, both groups showed higher Q̇-adjusted DSMO2 than controls (COPD: 0.97 (0.23) vs. controls 0.63 (0.24) mM/kPa, p=0.02; CHF 0.98 (0.11) mM/kPa vs. controls, p=0.001)
In response to exercise, DSMO2 increased in all groups (p <0.05) to reach similar values (COPD: 2.15 (0.3) vs. CHF: 2.1 (0.4) vs. controls: 2.0 (0.7) mmol/min/kPa. In contrast to the unadjusted DSMO2 response to exercise, the Q̇-adjusted DSMO2 was significantly increased in COPD (p=0.01) and controls (p=0.03), but not in the CHF group (p=0.2). However, we found no difference between COPD and CHF in Q̇-adjusted DSMO2 during exercise (COPD: 1.19 (0.11) vs. CHF: 1.00 (0.18) mM/kPa; p=0.24), although Q̇-adjusted DSMO2 was higher in COPD compared to controls (COPD vs. controls: 0.87 (0.28) mM/kPa, p=0.02), and similar in CHF and controls.
We studied leg muscle convective and diffusive O2 transport during small muscle mass exercise in COPD, CHF and healthy controls. There were no significant differences between COPD and CHF but we did observe disease-specific macro- and microvascular responses to exercise compared to the healthy condition. We found that while the skeletal muscle O2 delivery during exercise was lower in COPD, the microvasculature responded with an enhanced Q̇-adjusted DSMO2 or perhaps vice versa, the O2 delivery adapted to the increased muscle diffusion capacity seen at rest. This response was not observed in CHF, where Q̇-adjusted DSMO2 was unresponsive to exercise, despite high resting DSMO2 (Q-adjusted and unadjusted) values compared to controls. Likewise, CHF patients were not able to decrease CvO2 in response to exercise, as observed in COPD and controls, suggesting impaired O2 extraction during exercise. The LVC responses to exercise in CHF was similar to controls, indicating that the macrovascular response was preserved, while impaired in COPD. Nonetheless, both groups reached normal V̇O2SM values as expected during submaximal small muscle mass exercise at similar work loads. These data suggest that enhanced diffusive O2 transport capacity of the working muscles in COPD compensates for the attenuated convective O2 transport, while the convective response to exercise in CHF may downregulate muscle diffusive O2 transport capacity.
DSMO2 is an estimate of oxygen transport capacity from the capillary to the mitochondria (Østergaard, 2020). It comprises a convective component and a diffusive component, of which the latter depends on the total capillary wall area available for diffusion during red blood cell passage, reflecting the total number of perfused capillaries and their length, the capillary flow distribution, spatial distribution of capillaries and potential structural changes in the diffusion membrane (Wagner, 2000; Poole, 2019; Nyberg and Jones, 2022). The relative interdependence of convection and diffusion is difficult to establish during human muscle contractions. If, one is impaired, the other might compensate to some degree. Likewise, low convective response to small muscle mass exercise in COPD is not a universal observation in previous studies. Indeed, although some studies like us have found lower convective responses to exercise in severe COPD compared to controls (Brønstad et al., 2012; Broxterman et al., 2021), others reported either similar (Rossman et al., 2015) or even higher Q̇leg during exercise (Richardson et al., 2004) in moderate and severe COPD, respectively. These somewhat divergent findings are most likely due to the large difference between the control groups of the abovementioned studies. In our patients with moderate-to-severe COPD (FEV1 ∼50% of predicted), diffusive oxygen transport was augmented to enhance skeletal muscle O2 uptake despite an impaired blood flow response. In more severe COPD (FEV1 ∼30% of predicted), the diffusive response during KEE has previously been reported to be impaired, appeared to be specifically enhanced by 8 weeks of KEE training (Broxterman et al., 2021). Together with our findings, this indicates that enhanced diffusive oxygen transport has the potential to counter the impact of an insufficient blood flow response on V̇O2peak and that its gradual decline with disease severity is potentially reversible, i.e., by exercise training.
In our CHF patients, we found that the diffusive response to KEE seemed to be limited, which is in line with findings in some previous studies (Esposito et al., 2011). Thus, reductions in skeletal muscle diffusive oxygen transport have been reported in patients with CHF NYHA II-III, and of note, Esposito et al (2011) observed that exercise training by KEE over an eight-week period improved the diffusive component in the CHF patients to the same level as the healthy control group at baseline. Furthermore, they observed an augmented capillarization of the quadriceps muscle in parallel with improved peripheral exercise capacity after isolated leg muscle training for 8 weeks, but the cardiac output remained unchanged, suggesting that increases in V̇O2SM can be obtained without “central” improvements (Esposito et al., 2011). The same group performed another study where the control group was better matched on physical fitness and found no significant differences in haemodynamics and muscle metabolism, comparing CHF (NYHA II-III) and sedentary controls (Esposito et al., 2015). The convective response to small muscle exercise has been reported to be impaired in patients with CHF (Barrett-O’Keefe et al., 2014, 2019), while others have found that if less than 4 kg of muscle is activated the convective capacity was similar to healthy individuals (Magnusson et al., 1997). Although the numerical values in the present study showed a tendency towards lower convective responses in CHF during KEE, this was not significant when all groups were analysed in parallel and after statistical adjustment (Figure 1). A possible explanation for the divergent results across previous studies and in relation to our COPD group could be the pharmacological management of CHF patients. For example, ACE-inhibitors commonly prescribed to CHF patients affects cardiovascular regulation by relaxation of the vascular smooth muscle of resistance arterioles (Anderson et al., 2000; Radenković et al., 2019). Over the past 30 years, during which the abovementioned studies have been conducted, there has been a decrease in the use of positive inotropes such as digoxin. The interaction between these drugs and exercise must be considered because they affect the autonomic control of cardiovascular function (Watanabe, 1985; Gheorghiade et al., 1989; Heidenreich et al., 1997; van der Harst and de Boer, 2010) and vasodilatory capacity in skeletal muscle (Karsh and Bullock, 1964) which are pivotal to the peripheral adaptations to exercise.
While a reduced blood flow response to exercise in COPD was observed, and potentially also play a role in CHF, the main difference between the two disease categories was that diffusive oxygen transport was either near-maximal already at rest, or simply unresponsive to exercise in CHF, whilst augmented in skeletal muscle in COPD.
This study was performed on data from earlier studies with a new methodology. The mechanisms underlying exercise intolerance are complex and often multifactorial in both COPD and CHF patients. The pathophysiological factors that contribute are ventilatory limitations, gas exchange deficits, reduced cardiac function, and limb muscle dysfunction, and any combination might dominate the phenotype. There were no differences in ΔMAP between groups to suggest a heightened exercise pressor reflex activation, but CHF and COPD patients have been associated with sensitization of the exercise pressor reflex (Smith et al., 2005), which may contribute to their exercise intolerance (Gagnon et al.; Amann et al., 2014; Smith et al., 2020). We cannot exclude that a difference in exercise pressor reflex activation may have influenced our results, as inhibition of lower limb muscle mechano- and metabo-sensitive afferents has shown to increase exercise tolerance in CHF and COPD, perhaps through increased O2 delivery to the working muscle (Amann et al., 2014; Smith et al., 2020). Another challenge for this kind of study is sample size which is relatively small because of the invasive nature of these experiments, and results may not be extrapolated to all patients with COPD or CHF. The present findings were based on the KEE model, but it remains uncertain whether the findings also apply during more intense exercise involving a larger muscle mass or other muscle groups. It is difficult to find “matched” individuals that are healthy, but still has a very low level of physical activity, making it problematic to compare groups if there are fundamental differences in physical fitness. The effect sizes of these variables ranged from small to large, due to the small number of included patients and the large standard deviations.
Exercise training, often being conducted on a two-legged bike ergometer, is known to be one of the most effective treatments for improving quality of life and to increase exercise capacity in both CHF and COPD patients, mostly thought to be caused by “central” improvement (Hunt et al., 2005; Stav et al., 2009; Mccarthy et al., 2015; Spruit et al., 2016; Paneroni et al., 2017; Gao et al., 2021). Based on our data and the current literature it seems plausible that there is a potential for improvements of the peripheral oxygen conductance in skeletal muscle in COPD and CHF patients even if cardio-pulmonary function stays unaltered.
Disease-specific factors may play a role in peripheral exercise capacity in patients with COPD and CHF. Thus, low convective O2 transport to contracting muscle seemed to predominate the peripheral exercise limitation in COPD during small muscle mass exercise, whereas muscle diffusive O2 transport was unresponsive to exercise in CHF.
The raw data supporting the conclusions of this article will be made available by the authors, without undue reservation.
The studies involving human participants were reviewed and approved by Ethical Committee of the Capital Region of Copenhagen (H-2–2013–150; H-3–2013–048). The patients/participants provided their written informed consent to participate in this study.
JH: Performed the analysis, interpretation of the results, drafted the manuscript and revised it critically. SN drafted the manuscript, assisted with interpretation of the results, and revised the manuscript critically. UI: Conception and design of the work, acquisition, analysis, and interpretation of data, drafting of the work and revising it critically for important intellectual content; RD: analysis, and interpretation of data, drafting of the work and revising it critically for important intellectual content; GM: Acquisition and interpretation of data, revising the work critically for important intellectual content; BP, PT, and SM: design of the work, interpretation of data, revising the work critically for important intellectual content; RB: Conception of the work, analysis, and interpretation of data, drafting of the work and revising it critically for important intellectual content. All authors approved the final version of the manuscript and agree to be accountable for all aspects of the work in ensuring that questions related to the accuracy or integrity of any part of the work are appropriately investigated and resolved.
The Centre for Physical Activity Research (CFAS) is supported by TrygFonden (grants ID 101390 and ID 20045). The study was further supported by grants from Axel Muusfeldts Foundation, the Capital Region of Denmark, and the Novo Nordisk Foundation. During this work, RMGB was supported by a postdoctoral grant from Rigshospitalet.
The authors declare that the research was conducted in the absence of any commercial or financial relationships that could be construed as a potential conflict of interest.
All claims expressed in this article are solely those of the authors and do not necessarily represent those of their affiliated organizations, or those of the publisher, the editors and the reviewers. Any product that may be evaluated in this article, or claim that may be made by its manufacturer, is not guaranteed or endorsed by the publisher.
Amann M., Venturelli M., Ives S. J., Morgan D. E., Gmelch B., Witman M. A. H., et al. (2014). Group iii/iv muscle afferents impair limb blood in patients with chronic heart failure. Int. J. Cardiol. 174, 368–375. doi:10.1016/j.ijcard.2014.04.157
Anderson T. J., Elstein E., Haber H., Charbonneau F. (2000). Comparative study of ACE-inhibition, angiotensin II antagonism, and calcium channel blockade on flow-mediated vasodilation in patients with coronary disease (BANFF study). J. Am. Coll. Cardiol. 35, 60–66. doi:10.1016/S0735-1097(99)00537-9
Ausín P., Martínez-Llorens J., Sabaté-Bresco M., Casadevall C., Barreiro E., Gea J. (2017). Sex differences in function and structure of the quadriceps muscle in chronic obstructive pulmonary disease patients. Chron. Respir. Dis. 14, 127–139. doi:10.1177/1479972316674412
Barrett-O'Keefe Z., Lee J. F., Berbert A., Witman M. A. H., Nativi-Nicolau J., Stehlik J., et al. (2014). Hemodynamic responses to small muscle mass exercise in heart failure patients with reduced ejection fraction. Am. J. Physiology-Heart Circulatory Physiology 307, H1512–H1520. doi:10.1152/ajpheart.00527.2014
Barrett-O’Keefe Z., Lee J. F., Ives S. J., Trinity J. D., Witman M. A. H., Rossman M. J., et al. (2019). α-Adrenergic receptor regulation of skeletal muscle blood flow during exercise in heart failure patients with reduced ejection fraction. Am. J. Physiology-Regulatory, Integr. Comp. Physiology 316, R512–R524. doi:10.1152/ajpregu.00345.2018
Brønstad E., Rognmo O., Tjonna A. E., Dedichen H. H., Kirkeby-Garstad I., Håberg A. K., et al. (2012). High-intensity knee extensor training restores skeletal muscle function in COPD patients. Eur. Respir. J. 40, 1130–1136. doi:10.1183/09031936.00193411
Broxterman R. M., Wagner P. D., Richardson R. S. (2021). Exercise training in COPD: Muscle O2 transport plasticity. Eur. Respir. J. 58, 2004146. doi:10.1183/13993003.04146-2020
Buber J., Robertson H. T. (2022). Cardiopulmonary exercise testing for heart failure: Pathophysiology and predictive markers. Heart. 2021, 319617. doi:10.1136/heartjnl-2021-319617
Dahl R. H., Taudorf S., Bailey D. M., Møller K., Berg R. M. G. (2020). A method for modelling the oxyhaemoglobin dissociation curve at the level of the cerebral capillary in humans. Exp. Physiol. 105, 1063–1070. doi:10.1113/EP088615
Dempsey J. A. (2019). Respiratory determinants of exercise limitation. Clin. Chest Med. 40, 331–342. doi:10.1016/j.ccm.2019.02.002
Esposito F., Reese V., Shabetai R., Wagner P. D., Richardson R. S. (2011). Isolated quadriceps training increases maximal exercise capacity in chronic heart failure. J. Am. Coll. Cardiol. 58, 1353–1362. doi:10.1016/j.jacc.2011.06.025
Esposito F., Wagner P. D., Richardson R. S. (2015). Incremental large and small muscle mass exercise in patients with heart failure: Evidence of preserved peripheral haemodynamics and metabolism. Acta Physiol. 213, 688–699. doi:10.1111/apha.12423
Gagnon P., Bussières J. S., Ribeiro F., Gagnon S. L., Saey D., Gagné N., et al. (2012). Influences of spinal anesthesia on exercise tolerance in patients with chronic obstructive pulmonary disease. Am. J. Respir. Crit. Care Med. 186, 606–615. doi:10.1164/rccm.201203-0404OC
Gao M., Huang Y., Wang Q., Liu K., Sun G. (2021). Effects of high-intensity interval training on pulmonary function and exercise capacity in individuals with chronic obstructive pulmonary disease: A meta-analysis and systematic review. Adv. Ther. 39, 94–116. doi:10.1007/s12325-021-01920-6
Gheorghiade M., Hall V., Lakier J. B., Goldstein S. (1989). Comparative hemodynamic and neurohormonal effects of intravenous captopril and digoxin and their combinations in patients with severe heart failure. J. Am. Coll. Cardiol. 13, 134–142. doi:10.1016/0735-1097(89)90561-5
Heidenreich P. A., Lee T. T., Massie B. M. (1997). Effect of beta-blockade on mortality in patients with heart failure: A meta-analysis of randomized clinical trials 11All editorial decisions for this article, including selection of referees, were made by a guest editor. This policy applies to all articles with authors from the university of California san francisco. J. Am. Coll. Cardiol. 30, 27–34. doi:10.1016/S0735-1097(97)00104-6
Houstis N. E., Eisman A. S., Pappagianopoulos P. P., Wooster L., Bailey C. S., Wagner P. D., et al. (2018). Exercise intolerance in heart failure with preserved ejection fraction. Circulation 137, 148–161. doi:10.1161/CIRCULATIONAHA.117.029058
Hunt S. A., Abraham W. T., Chin M. H., Feldman A. M., Francis G. S., Ganiats T. G., et al. (2005). ACC/AHA 2005 guideline update for the diagnosis and management of chronic heart failure in the adult. J. Am. Coll. Cardiol. 46, e1–e82. doi:10.1016/j.jacc.2005.08.022
Iepsen U. W., Munch G. W., Rugbjerg M., Ryrsø C. K., Secher N. H., Hellsten Y., et al. (2017). Leg blood flow is impaired during small muscle mass exercise in patients with COPD. J. Appl. Physiology 123, 624–631. doi:10.1152/japplphysiol.00178.2017
Iepsen U. W., Munch G. W., Ryrsø C. K., Secher N. H., Lange P., Thaning P., et al. (2018). Muscle α-adrenergic responsiveness during exercise and ATP-induced vasodilation in chronic obstructive pulmonary disease patients. Am. J. Physiology-Heart Circulatory Physiology 314, H180–H187. doi:10.1152/ajpheart.00398.2017
Karsh R. B., Bullock F. A. (1964). Studies on digitalis. X. Effects of ouabain on forearm vas-cular resistance and venous tone in normal sub-jects and in patients in heart failure. J. Clin. Invest. 43, 532–543. doi:10.1172/JCI104939
Keller-Ross M. L., Larson M., Johnson B. D. (2019). Skeletal muscle fatigability in heart failure. Front. Physiol. 10, 1–8. doi:10.3389/fphys.2019.00129
Magnusson G., Kaijser L., Sylvén C., Karlberg K. E., Isberg B., Saltin B. (1997). Peak skeletal muscle perfusion is maintained in patients with chronic heart failure when only a small muscle mass is exercised. Cardiovasc. Res. 33, 297–306. doi:10.1016/S0008-6363(96)00249-0
Maltais F., Decramer M., Casaburi R., Barreiro E., Burelle Y., Debigaré R., et al. (2014). An official American thoracic society/european respiratory society statement: Update on limb muscle dysfunction in chronic obstructive pulmonary disease. Am. J. Respir. Crit. Care Med. 189, e15–e62. doi:10.1164/rccm.201402-0373ST
McCarthy B., Casey D., Devane D., Murphy K., Murphy E., Lacasse Y. (2015). Pulmonary rehabilitation for chronic obstructive pulmonary disease. Cochrane Database Syst. Rev. 23, CD003793. doi:10.1002/14651858.CD003793.pub3
Mortensen S. P., Saltin B. (2014). Regulation of the skeletal muscle blood flow in humans. Exp. Physiol. 99, 1552–1558. doi:10.1113/expphysiol.2014.081620
Mortensen S. P., Wewer U., Sp M., Damsgaard R., Saltin B. (2010). Regulation of skeletal muscle blood flow and central hemodynamics in exercising humans.
Munch G. W., Iepsen U.W., Ryrsø C. K., Rosenmeier J. B., Pedersen B. K., Mortensen S. P. (2018). Effect of 6 weeks of high-intensity one-legged cycling on functional sympatholysis and ATP signaling in patients with heart failure. Am. J. Physiology-Heart Circulatory Physiology 314, H616–H626. doi:10.1152/ajpheart.00379.2017
Nyberg M., Jones A. M. (2022). Matching of O2 utilization and O2 delivery in contracting skeletal muscle in health, aging, and heart failure. Front. Physiol. 13, 1–15. doi:10.3389/fphys.2022.898395
Østergaard L. (2020). Blood flow, capillary transit times, and tissue oxygenation: The centennial of capillary recruitment. J. Appl. Physiology 129, 1413–1421. doi:10.1152/JAPPLPHYSIOL.00537.2020
Paneroni M., Simonelli C., Vitacca M., Ambrosino N. (2017). Aerobic exercise training in very severe chronic obstructive pulmonary disease. Am. J. Phys. Med. Rehabil. 96, 541–548. doi:10.1097/PHM.0000000000000667
Pittman R. N. (2000). Oxygen supply to contracting skeletal muscle at the microcirculatory level: Diffusion vs. convection. Acta Physiol. Scand. 168, 593–602. doi:10.1046/J.1365-201X.2000.00710.X
Poole D. C. (2019). Edward F. Adolph distinguished lecture. Contemporary model of muscle microcirculation: Gateway to function and dysfunction. J. Appl. Physiology 127, 1012–1033. doi:10.1152/japplphysiol.00013.2019
Poole D. C., Musch T. I., Colburn T. D. (2022). Oxygen flux from capillary to mitochondria: Integration of contemporary discoveries. Eur. J. Appl. Physiol. 122, 7–28. doi:10.1007/s00421-021-04854-7
Poole D. C., Richardson R. S., Haykowsky M. J., Hirai D. M., Musch T. I. (2018). Exercise limitations in heart failure with reduced and preserved ejection fraction. J. Appl. Physiology 124, 208–224. doi:10.1152/japplphysiol.00747.2017
Radenković M., Stojanović M., Prostran M. (2019). Calcium channel blockers in restoration of endothelial function: Systematic review and meta-analysis of randomized controlled trials. Cmc 26, 5579–5595. doi:10.2174/0929867325666180713144806
Richardson R. S., Leek B. T., Gavin T. P., Haseler L. J., Mudaliar S. R. D., Henry R., et al. (2004). Reduced mechanical efficiency in chronic obstructive pulmonary disease but normal peak Vo2 with small muscle mass exercise. Am. J. Respir. Crit. Care Med. 169, 89–96. doi:10.1164/rccm.200305-627oc
Rossman M. J., Trinity J. D., Garten R. S., Ives S. J., Conklin J. D., Barrett-O'Keefe Z., et al. (2015). Oral antioxidants improve leg blood flow during exercise in patients with chronic obstructive pulmonary disease. Am. J. Physiology-Heart Circulatory Physiology 309, H977–H985. doi:10.1152/ajpheart.00184.2015
Saltin B. (1985). Maximal perfusion of skeletal muscle in man. J. Physiol. 366, 233–249. doi:10.1113/jphysiol.1985.sp015794
Shibata M., Ichioka S., Togawa T., Kamiya A. (2006). Arterioles' contribution to oxygen supply to the skeletal muscles at rest. Eur. J. Appl. Physiol. 97, 327–331. doi:10.1007/s00421-006-0200-2
Smith J. R., Joyner M. J., Curry T. B., Borlaug B. A., Keller-Ross M. L., Van Iterson E. H. (2020). Locomotor muscle group III/IV afferents constrain 1057 stroke volume and contribute to exercise intolerance in human heart failure. J. Physiol. 598, 5379–5390. doi:10.1113/jp280333
Smith J. R., Joyner M. J., Curry T. B., Borlaug B. A., Keller‐Ross M. L., Van Iterson E. H., et al. (2020). Locomotor muscle group III/IV afferents constrain stroke volume and contribute to exercise intolerance in human heart failure. J. Physiol. 598, 5379–5390. doi:10.1113/jp280333
Smith S. A., Mitchell J. H., Naseem H. R., Garry M. G. (2005). Mechanoreflex mediates the exaggerated exercise pressor reflex in heart failure. Circulation 112, 2293–2300. doi:10.1161/CIRCULATIONAHA.105.566745
Spruit M. A., Burtin C., De Boever P., Langer D., Vogiatzis I., Wouters E. F. M., et al. (2016). COPD and exercise: Does it make a difference? Breathe 12, e38–e49. doi:10.1183/20734735.003916
Stav D., Raz M., Shpirer I. (2009). Three years of pulmonary rehabilitation: Inhibit the decline in airflow obstruction, improves exercise endurance time, and body-mass index, in chronic obstructive pulmonary disease. BMC Pulm. Med. 9, 26. doi:10.1186/1471-2466-9-26
van der Harst P., de Boer R. A. (2010). Statins in the treatment of heart failure. Circ. Heart Fail. 3, 462–464. doi:10.1161/CIRCHEARTFAILURE.110.956342
Vestbo J., Hurd S. S., Agustí A. G., Jones P. W., Vogelmeier C., Anzueto A., et al. (2013). Global strategy for the diagnosis, management, and prevention of chronic obstructive pulmonary disease. Am. J. Respir. Crit. Care Med. 187, 347–365. doi:10.1164/rccm.201204-0596PP
Wagner P. D. (1996). Determinants of maximal oxygen transport and utilization. Annu. Rev. Physiol. 58, 21–50. doi:10.1146/annurev.ph.58.030196.000321
Wagner P. D. (2000). Diffusive resistance to O2 transport in muscle. Acta Physiol. Scand. 168, 609–614. doi:10.1046/j.1365-201X.2000.00712.x
Watanabe A. M. (1985). Digitalis and the autonomic nervous system. J. Am. Coll. Cardiol. 5, 35A–42A. doi:10.1016/S0735-1097(85)80461-7
Keywords: COPD, chronic obstructive pulmonary disease, heart failiure, knee extensor exercise, capillary recruitment, exercise capacity
Citation: Hartmann JP, Dahl RH, Nymand S, Munch GW, Ryrsø CK, Pedersen BK, Thaning P, Mortensen SP, Berg RMG and Iepsen UW (2022) Regulation of the microvasculature during small muscle mass exercise in chronic obstructive pulmonary disease vs. chronic heart failure. Front. Physiol. 13:979359. doi: 10.3389/fphys.2022.979359
Received: 27 June 2022; Accepted: 08 August 2022;
Published: 31 August 2022.
Edited by:
Michael E Tschakovsky, Queen’s University, CanadaReviewed by:
Ryan Garten, Virginia Commonwealth University, United StatesCopyright © 2022 Hartmann, Dahl, Nymand, Munch, Ryrsø, Pedersen, Thaning, Mortensen, Berg and Iepsen. This is an open-access article distributed under the terms of the Creative Commons Attribution License (CC BY). The use, distribution or reproduction in other forums is permitted, provided the original author(s) and the copyright owner(s) are credited and that the original publication in this journal is cited, in accordance with accepted academic practice. No use, distribution or reproduction is permitted which does not comply with these terms.
*Correspondence: Ronan M. G. Berg, cm9uYW5Ac3VuZC5rdS5kaw==
†ORCID: Jacob Peter Hartmann, orcid.org/0000-0003-0829-2645; Rasmus H. Dahl, orcid.org/0000-0002-2014-7400; Stine B. Nymand, orcid.org/0000-0003-0950-5032; Gregers W. Munch, orcid.org/0000-0002-2871-6794; Camilla K. Ryrsøe, orcid.org/0000-0002-8059-7331; Bente K. Pedersen, orcid.org/0000-0001-6508-6288; Pia Thaning, orcid.org/0000-0001-9374-5674; Stefan P. Mortensen, orcid.org/0000-0001-8958-1048; Ronan M.G. Berg, orcid.org/0000-0002-5757-9506; Ulrik Winning Iepsen, orcid.org/0000-0002-8140-2583
‡These authors have contributed equally to the work and share first authorship
Disclaimer: All claims expressed in this article are solely those of the authors and do not necessarily represent those of their affiliated organizations, or those of the publisher, the editors and the reviewers. Any product that may be evaluated in this article or claim that may be made by its manufacturer is not guaranteed or endorsed by the publisher.
Research integrity at Frontiers
Learn more about the work of our research integrity team to safeguard the quality of each article we publish.