- INSERM UMR1093-CAPS, Université Bourgogne Franche-Comté, UFR des Sciences du Sport, Dijon, France
Neuromuscular fatigability is a failure to produce or maintain a required torque, and commonly quantified with the decrease of maximal torque production during a few seconds-long maximal voluntary contraction (MVC). The literature shows that the MVC reduction after exercises with different torque-time integral (TTI), is often similar. However, it was shown that after a fatiguing exercise, the decline in the capacity to sustain the maximal voluntary contraction for 1 min (MVC1-MIN) differs from the decrease in the capacity to perform a brief-MVC, suggesting that this latter can only partially assess neuromuscular fatigability. This study aims to highlight the relevance of using a sustained MVC to further explore the neuromuscular alterations induced by fatiguing exercises with different TTI. We used two contraction intensities (i.e., 20% and 40% MVC) to modulate the TTI, and two exercise modalities [i.e., voluntary (VOL) and electrical induced (NMES)], since the letter are known to be more fatiguing for a given TTI. Thirteen subjects performed a plantar-flexors MVC1-MIN before and after the fatiguing exercises. A similar MVC loss was obtained for the two exercise intensities despite a greater TTI at 40% MVC, regardless of the contraction modality. On the other hand, the torque loss during MVC1-MIN was significantly greater after the 40% compared to 20% MVC exercise. These findings are crucial because they demonstrate that maximal torque production and sustainability are two complementary features of neuromuscular fatigability. Hence, MVC1-MIN assessing simultaneously both capacities is essential to provide a more detailed description of neuromuscular fatigability.
Introduction
Performance fatigability is commonly defined as an objective decline in motor performance (Kluger et al., 2013) such as a failure to produce or maintain the required torque (Edwards, 1981). This phenomenon develops progressively from the onset to the exercise end, and it involves muscular changes occurring within the muscle as well as a failure of the central nervous system to adequately drive the motoneurons (Gandevia, 2001). In accordance with the previous definition, the protocol classically used to quantify fatigability requires recording of maximal torque during a brief (i.e., a few seconds long) maximal voluntary contraction (MVC), performed before and after a fatiguing exercise. Additionally, percutaneous stimulations of the motor nerve are generally used to estimate the relative contributions of neural and muscular processes to the decline in MVC (Millet et al., 2011).
The decline in maximal torque production (i.e., MVC loss) has been widely used in the literature to assess the neuromuscular fatigability induced by ecological exercises, like running or cycling, but also by isometric exercises involving a specific muscle group or task. For instance, some studies investigated the impact of isometric exercises carried out with different muscle activation modalities on maximal torque production. Muthalib et al. (2010) observed that the same number of elbow flexors contractions, evoked by neuromuscular electrical stimulations (NMES) or voluntarily performed (VOL), induced the same MVC loss, but for a muscle torque-time integral (i.e., the total amount of torque produced during the exercise, TTI) that was twice lower in NMES. Similarly, Hachard et al. (2019) compared the MVC loss induced by VOL and NMES isometric contractions of knee extensors at 20% MVC, performed until task failure. The authors observed that the MVC loss of the knee extensors was identical for these two exercise modalities although the time to task failure, and thus the TTI, were greater in VOL. Taken together, these results suggest that VOL and NMES exercises are equally altering the maximal torque production capacity, despite a different TTI. It, therefore, appears that the MVC loss caused by these exercises is independent from TTI. This consideration led us to look for an index sensitive to the exercise TTI.
To explore neuromuscular fatigability, Byrne and Eston (2002) used, in addition to the brief MVC, a MVC sustained for 1 min (MVC1-MIN) before and after an eccentric exercise. These authors showed that 10 × 10 eccentric contractions of the quadriceps muscle, performed at 80% of 1-repetition maximum, differently affects maximal torque production capacity (i.e., torque loss during a brief MVC), and maximal torque sustainability (i.e., torque loss during a MVC1-MIN); the former was reduced while the latter was not. This result suggests that loss of maximal torque production and maximal torque sustainability represent distinct aspects of neuromuscular fatigability. The MVC loss provides an insight into nervous and/or muscular alterations induced by muscle exercise (Boyas and Guével, 2011; Kent-Braun et al., 2012). Whereas, torque loss during a sustained MVC reflects the adaptive response of the neuromuscular system to maintain a high level of torque despite the nervous and/or muscular alterations induced by exercise.
We thought to consider the torque decline observed during a prolonged MVC, because a protracted contraction could be more affected by the energy expenditure generated by a fatiguing exercise (assessed by the TTI) and other homeostatic parameters (Boska et al., 1990; Davis and Bailey, 1997; Gandevia, 1998; Kent-Braun, 1999; Amann et al., 2013), than a short effort (brief-MVC). Because a MVC1-MIN can assess both maximal torque production and sustainability, this study aims to highlight the relevance of using a sustained MVC to further explore the neuromuscular alterations induced by fatiguing exercises with different TTI. The relevance of this study lies in the fact that it could emphasize the necessity of assessing both capacities when aiming to characterize neuromuscular fatigability. To this end, we proposed to use a sustained MVC for 1 min (MVC1-MIN) before and after submaximal fatiguing exercises with different contraction modalities (VOL and NMES) and muscle TTIs (contractions at 20% and 40% MVC). NMES contractions are known to be more fatiguing than VOL ones, when muscle TTIs are matched, as indicated by the greater MVC loss induced by muscle exogenous activation (Theurel et al., 2007; Jubeau et al., 2008). Thus, the comparison between these two contraction modalities, at two levels of muscle TTI, seems to be the adequate experimental paradigm to evaluate the interest of associating indexes of maximal torque production and sustainability since the former will be more impacted by the contraction modality (NMES vs. VOL) and the latter by the muscle contraction level (20% vs. 40% MVC). Our hypothesis is that the fatiguing exercise with the greatest TTI induces the greatest loss of torque during the sustained MVC (∆MVC1-MIN). Finally, because reductions in maximal torque production and sustainability are transient phenomena, our second aim was to investigate the recovery of MVC loss and ∆MVC1-MIN after a 10-min rest period.
Materials and methods
Subjects
Thirteen healthy volunteers (three females; age: 23.5 ± 2.0 years, body mass: 72.2 ± 15.7 kg, height: 177.6 ± 9.2 cm, body mass index: 22.7 ± 3.2 kg m−2) without neurological or physical disorders gave written informed consent to participate in this study. All subjects were right-footed and physically active [Physical Activity Scale (Robert et al., 2004): 21.5 ± 3.9]. The protocol was approved by the CPP SOOM III ethics committee (number 2017-A00064-49; ClinicalTrials.gov Identifier: NCT03334526) and complied with the Declaration of Helsinki.
Protocol
Experimental design
All subjects took part in four experimental sessions randomly administered. The sessions lasted 1.5 h each and were interspaced by an interval of 7 days. For each subject, sessions were planned at the same time of the day. Each session included a neuromuscular assessment of plantar flexors (PF) (torque and electromyographic (EMG) recordings) before (Pre) and after a submaximal fatiguing exercise (Post), and after a recovery period of 10 min (Post-10). The submaximal fatiguing exercise started 3 min after the completion of the pre-exercise neuromuscular assessments. This fatiguing exercise changed among sessions. Briefly, it consisted of neuromuscular electrical stimulation (NMES) or voluntary contraction (VOL) performed each at two submaximal intensities (i.e., 20% and 40% MVC). Six out of thirteen subjects also participated in a control session during which only pre and post-tests were performed without fatiguing exercise.
Neuromuscular assessment
The neuromuscular assessment was preceded by a standardized warm-up protocol for PF. Subjects were asked to perform five isometric contractions at 25% of their perceived maximal effort, 3% at 50%, and 2% at 80%, each lasting 5 s. Two MVC lasting 5 s with 30-s rest in-between were performed to ensure that subjects were ready to start the neuromuscular assessment. Then, a 1 min-rest period was given to the subjects before starting the neuromuscular assessment. The neuromuscular assessment consisted in a MVC1-MIN of PF. A single percutaneous stimulation of the tibial nerve at rest gave subjects the signal to start the sustained contraction. Electrical doublets superimposed to the contraction were delivered at the same time during the MVC1-MIN (i.e., 5 and 55 s after the single stimulation). To ensure maximal performance, loud verbal encouragements were given to the subject during the effort. In addition, participants were informed of the MVC1-MIN duration to lessen the adoption of a pacing strategy (Halperin et al., 2014) during the sustained contraction. The choice to set to 1 min the duration of the sustained MVC was made because it has been shown that the greatest torque loss occurs during the first minute of contraction (Gandevia et al., 1996; Kent-Braun, 1999; Post et al., 2009).
Fatiguing exercise
The fatiguing exercise was composed of intermittent isometric contractions of PF (10 s on/5 s off, duty cycle = 67%). In VOL session, subjects had to adjust their torque to a visual target-torque feedback displayed on a computer screen placed in front of them (according sound signals to respect the duty cycle), while in NMES session, the stimulation intensity was set to reach the target torque and kept constant. The TTI produced during each fatiguing exercise was controlled with a custom-made system made of a voltmeter (5XP-A, Amprobe) and synchronized with the dynamometer. In the first session of each exercise intensity (20% and 40% MVC), the number of fatiguing contractions was set to 40, regardless of the exercise modality (NMES or VOL). In the second session at the same intensity, but performed in the remaining modality, the number of contractions was adjusted to obtain a similar TTI in both modalities.
To ensure that pre-exercise MVC1-MIN did not affect post-test torque production, six out of 13 subjects had an extra session without the fatiguing exercise, but with a 10-min rest period (corresponding to the time of exercise) between the neuromuscular assessments (i.e., control session).
The experimental protocol is depicted in Figure 1.
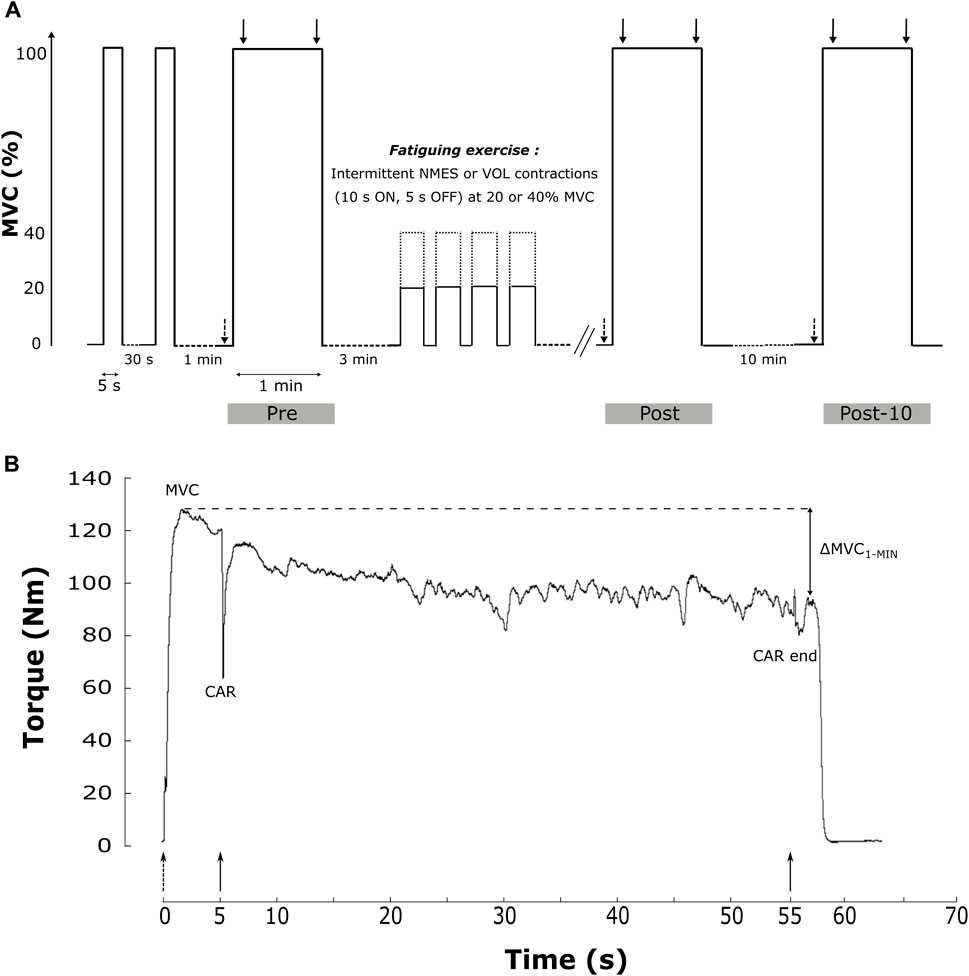
FIGURE 1. Sum up of the experimental design of the study. (A). The protocol included two brief-MVC and a 1 min sustained MVC (MVC1-MIN) before (Pre), after (Post) the fatiguing exercise and after a 10 min rest period (Post-10). The fatiguing exercise was an intermittent task with 10 s of contraction and 5 s of rest, in VOL or NMES modalities and at 20% (solid line) or 40% MVC (dashed line). Single (dashed arrow) and 100 Hz doublet (solid arrow) electrical stimulations are delivered during the protocol. (B). Data of torque production during the MVC1-MIN from one representative subject before the fatiguing exercise. Main studied parameters (MVC, CAR and ∆MVC1-MIN) are reported. Dotted up arrows represent single electrical stimulations of PF at rest before the MVC1-MIN, while solid down arrows represent double electrical stimulations (100 Hz) superimposed at the start and the end of the MVC1-MIN. CAR = central activation ratio; MVC = maximal voluntary contraction; MVC1-MIN = 1-min sustained MVC; ∆MVC1-MIN = torque loss during the sustained MVC; VOL = voluntary fatiguing exercise; NMES = neuromuscular electrical stimulation fatiguing exercise; 20% and 40% MVC represent the intensity of the fatiguing exercise.
Data acquisition
Torque recordings
Voluntary and electrically-evoked PF isometric torque was recorded using an isokinetic dynamometer (Biodex Medical System Inc. Shirley, NY, United States). Subjects were comfortably seated on the chair of the dynamometer with the right foot securely strapped to the footplate at the ankle level. The centre of rotation of the dynamometer shaft was aligned with the lateral malleolus. The ankle angle was set at 90°, the knee joint was at 110° and the hip angle was at 130° (180° full extension). To limit trunk movements that could affect torque development, the hip was firmly strapped to the seat with a belt. During fatiguing exercises, subjects had to adjust their torque to a visual feedback of the target torque displayed on a computer screen placed at 2 m in front of them for VOL sessions while the stimulation intensity is initially set to reach the target torque during NMES and kept constant. The setting configuration was kept constant throughout the entire experimental session and among sessions.
EMG recordings
Electromyographic activity was recorded from four muscles of the right leg: the soleus (SOL), the gastrocnemii medialis (GM) and lateralis (GL), and the tibialis anterior (TA). EMG recording of the antagonist muscle allowed checking that no inadvertent activation of this muscle occurs during tibial nerve stimulation. EMG was recorded bipolarly using silver chloride circular surface electrodes (7 mm recording diameter) (Contrôle Graphique Medical, Brie-Compte-Robert, France) with an inter-electrode distance (centre-to-centre) of 20 mm. To minimize skin impedance (<5 kΩ), the skin was shaved, abraded and cleaned with alcohol, before electrode positioning. For SOL, electrodes were positioned 3 cm below the insertion of the two gastrocnemii over the Achilles’ tendon. For GM and GL, electrodes were placed over the muscle bellies. For TA, they were positioned on the upper third of the distance between the fibula head and the tip of the lateral malleolus. The reference electrode was placed between the two gastrocnemii bellies of the same leg. EMG signals were amplified (gain = 1,000) and filtered (10 Hz–500 Hz). Torque and EMG data were recorded at a sampling frequency of 2 kHz with the Biopac acquisition system (Biopac System Inc., United States) and stored with commercially available software (Acqknowledge MP150) for off-line analysis.
Stimulation
Nerve stimulation test
Percutaneous electrical stimulation of the posterior tibial nerve in the popliteal fossa was carried out to evoke electrophysiological and mechanical responses of the triceps surae muscles. Monophasic rectangular pulses (1 ms) were delivered using a Digitimer stimulator (DS7AH, Digitimer, Hertfordshire, United Kingdom), triggered by a commercially available software (Tida, Heka Elektronik, Lambrecht/Pfalz, Germany). The anode (5 cm × 10 cm, Compex SA, Ecublens, Switzerland) was placed beneath the patella. The optimal stimulation site, namely the site where the greatest M-wave amplitude in SOL was evoked, was located with a hand-held cathode ball (0.5 cm diameter). Once the stimulation site was determined, the cathode (a self-adhesive electrode with 7 mm diameter, Contrôle Graphique Medical, Brie-Compte-Robert, France) was firmly fixed to this site with tape. The intensity of stimulation was progressively increased by 5 mA from the M-wave threshold to the maximal M-wave (MMAX). The intensity at which MMAX amplitude plateaued (i.e., no further increase in the M-wave amplitude was evident in SOL) was further increased by 20% to ensure a supramaximal stimulation. This stimulation intensity was kept constant throughout the experimental session (mean supramaximal intensity was 65.3 ± 30.3 mA). Electrical doublets superimposed to the contraction during the MVC1-MIN were delivered at supramaximal stimulation intensity with an inter-pulse duration of 10 ms (100 Hz).
Electrically evoked fatiguing exercise
PF NMES contractions were evoked using two rectangular electrodes (5 cm × 10 cm, Compex SA, Ecublens, Switzerland) placed over the triceps surae muscle, respectively 5 cm beneath the popliteal fossa and on the insertion point of the two gastrocnemii muscles to the Achilles tendon, around 2 cm above SOL EMG electrodes. The NMES fatiguing intermittent exercise consisted of trains of monophasic rectangular pulses (50 Hz, 500 µs) lasting 10 s interspaced by 5 s and delivered to PF via the Digitimer stimulator. The stimulation intensity was progressively increased in order to achieve 20% or 40% MVC, according to the experimental session, and kept constant during the NMES protocol.
Data analysis
Torque analysis
MVC was determined as the highest torque achieved during the first 5 s of the MVC1-MIN. To ensure that participants did not adopt a pacing strategy that could influence the MVC measure (Šambaher et al., 2016), we also measured the maximal torque reached during brief-MVC to compare it with pre-exercise MVC. The pre-exercise MVC was used to set the target torque of the fatiguing exercise. The MVC loss corresponds to the relative difference between the MVC values recorded before and after the fatiguing exercise. The torque loss during the MVC1-MIN (∆MVC1-MIN) was calculated as the relative change between the MVC value and the mean torque of the last 5 s of contraction as follows:
Change in ∆MVC1-MIN between pre and post-exercise was quantified as the difference between both measures:
The central activation ratio (CAR) was calculated at the beginning and the end of the MVC1-MIN (after 5 and 55 s of contraction, respectively), according to the formula of Gandevia et al. (1996):
A value of 100 indicate a full central activation. The ratio between the CAR at the end and at the beginning of the MVC1-MIN, expressed in percentage of change, is called ∆CAR1-MIN and represents the evolution of central activation during the MVC1-MIN. The ∆CAR1-MIN was calculated with the following equation:
The TTI of the fatiguing exercise represents the total amount of torque produced by the PF and corresponds to the integral of the entire exercise period. This time window started at the onset of the raising phase of the first fatiguing contraction and ended after the decline phase of the last one.
EMG analysis
Considering that SOL, MG and LG concomitantly contribute to the plantar flexion mechanical response, we chose to express the EMG data (EMG activity and MMAX) as the sum of these three triceps surae muscles. The sum of the peak-to-peak amplitude of MMAX of the three triceps surae muscles, evoked at rest, before and after each MVC1-MIN, was calculated (ΣMMAX). The EMG activity of each muscle during the MVC1-MIN was quantified by the root mean square (RMS) value of the raw EMG signal. The RMS associated with the peak force was calculated from 250 ms prior the MVC to 250 ms after for each muscle and summed (ΣRMSMVC). This sum was then normalized with respect to the ΣMMAX recorded before the MVC1-MIN (ΣRMSMVC/ΣMMAX). The ΣRMS value was also calculated 500 ms prior to the second superimposed doublet (i.e., 55 s after the MVC1-MIN start) and normalized by the ΣMMAX recorded, at rest, after the MVC1-MIN. Then, this latter ratio was expressed as the relative change with respect to ΣRMSMVC/ΣMMAX (∆RMS/ΣMMAX) to evaluate muscle activity changes during the MVC1-MIN.
Statistical analysis
Normality criteria were tested using the Shapiro-Wilk test. When the normality test failed, non-parametric analysis was performed. The factors used for ANOVA analysis were condition (NMES and VOL), intensity (20 and 40% MVC), time (pre, post and post-10) and measure (brief and sustained contraction). Paired t-tests were performed on MVC and ∆MVC1-MIN obtained during the control session (pre vs. post). Two-way factorial ANOVA (condition and intensity) on fatiguing exercise TTI was conducted. Three-way ANOVAs with repeated measures (condition, intensity, and time) were performed on MVC, MVC loss, and ∆MVC1-MIN. A Three-way ANOVA with repeated measures (condition, intensity and measure) was performed on MVC before the fatiguing exercise. When a significant main effect or interaction was found, a post-hoc analysis was made using the HSD Tukey’s test. The Non-parametric related samples Friedman’s two-way ANOVA by rank with condition and intensity factors was used for contraction number during the fatiguing exercise. Friedman’s three-way ANOVAs with condition, intensity, and time factors were conducted on CAR and ∆CAR1-MIN, ΣRMSMVC/ΣMMAX and ∆RMS/ΣMMAX, and on ΣMMAX. When the Friedman’s ANOVA revealed an effect, the Wilcoxon’s signed-rank test for paired multiple comparisons (with a Bonferroni correction) was performed. Correlations between exercise TTI and torque loss variables (MVC loss and ∆MVC1-MIN change) were analysed using repeated measures correlations (rmcorr R package, Bakdash and Marusich, 2017). We calculated the intraclass correlation coefficient (ICC) for the maximal torque from brief MVC and MVC1-MIN before the fatiguing exercise. The ICC calculation was based on a mean-rating (k = 2), absolute-agreement, 2-way mixed-effects model estimates and their 95% confident intervals (CI95%). Values less than 0.5 are indicative of poor reliability, values between 0.5 and 0.75 indicate moderate reliability, values between 0.75 and 0.9 indicate good reliability, and values greater than 0.90 indicate excellent reliability (Koo and Li, 2016).
It was estimated that thirteen participants were needed to detect differences with moderate effect size (ηp2 = 0.08) using standard parameters of 1−β = 0.95 and α = 0.05. The critical level for statistical significance was set at 5%. Effect sizes of parametric ANOVA are reported as partial eta squared (ηp2) with small (≥0.01), moderate (≥0.07) and large effects (≥0.14). Effect sizes (ES) were also calculated for parametric (Cohen’s d) and non-parametric comparisons (Cohen’s r) (Fritz et al., 2012). Small, moderate and large effects are considered for Cohen’s d ≥ 0.2, ≥0.5, ≥0.8, and Cohen’s r ≥ 0.1, ≥0.3, ≥0.5 respectively. Results are reported as mean ± standard deviation. Repeated measures correlations were performed and plotted with R (version 4.1.3, R Core Team, 2017). ICC analysis was performed with SPSS Statistical package (SPSS Inc., version 22, Chicago, IL, United States). Other frequentist statistical tests were performed using Statistica software (Statsoft, version 12, Tulsa, OK, United States) except for power analysis and the Cohen’s effect size that were calculated with G*Power software (version 3.1.9.2, Universität Düsseldorf, Germany).
Results
Control session
In subjects who performed the extra session without fatiguing exercise, no significant difference was observed between pre and post-test for MVC (120.5 ± 15.7 Nm vs. 121.7 ± 11.9 Nm respectively, t (5) = −0.54, p = 0.613, CI95% = [−6.736: 4.403], ES = 0.086) and ∆MVC1-MIN (−31.1% ± 5.2% vs. −29.8% ± 4.6% respectively, t (5) = −1.60, p = 0.171, CI95% = [-3.346: 0.779], ES = 0.265). Thus, it may be concluded that the pre-test MVC1-MIN did not affect the post-test MVC and ∆MVC1-MIN, analysed after a 10 min rest period (corresponding to the time of exercise).
Fatiguing exercise
As the TTI of the fatiguing exercises was matched for the same intensity between modalities, the statistical analysis only revealed a significant intensity effect (F1,12 = 94.802, p < 0.001, ηp2 = 0.888). As expected, the TTI was higher in 40% than in 20% MVC intensity (20,438 ± 2,939 Nms vs. 12,009 ± 2,340 Nms, p < 0.001, ES = 3.173). Because torque production during NMES contractions was not as steady as in the VOL condition, we let participants to perform more contraction in NMES condition than in VOL condition, for both contraction intensities (20% MVC: NMES = 51 ± 16 vs. VOL = 35 ± 6, Z = 3.06, p = 0.002, ES = 0.849; 40% MVC: NMES = 50 ± 11 vs. VOL = 37 ± 4, Z = 3.18, p = 0.002, ES = 0.882).
Assessments related to maximal torque production capacity
Torque
To ensure that MVC1-MIN provide a reliable index of maximal torque production, the maximal torque recorded during the brief and the MVC1-MIN were compared before the fatiguing exercise. No significant difference between these values was detected (F1,12 = 0.818, p = 0.384, ηp2 = 0.064). In addition, the ICC of MVC between both measures was 0.951 (CI95% = 0.915–0.972), indicating an excellent reliability.
By comparing MVC among protocol time points, we observed a significant time effect (F2,24 = 35.503, p < 0.001, ηp2 = 0.747) whereas no condition, intensity or interaction effect was found. As depicted in Figure 2, pooled pre-exercise value (110.6 ± 23.5 Nm) was significantly higher compared to post-exercise (98.2 ± 21.3 Nm, p < 0.001) and post-10 min (99.3 ± 21.7 Nm, p < 0.001). No significant difference in MVC between post and post-10 was observed (p = 0.769). Moreover, no significant correlation was detected between post-exercise MVC loss and exercise TTI [rrm (38) = −0.294, p = 0.066, CI95% = (−0.561 : 0.029)] (Figure 3A).
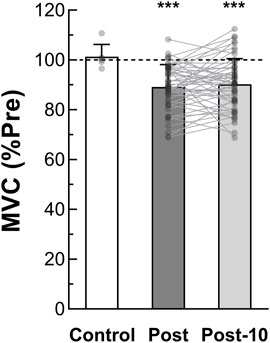
FIGURE 2. Maximal voluntary torque production (n = 13). MVC is determined as the maximal voluntary torque generated during the MVC1-MIN. Pooled Post-exercise and Post 10-min recovery period MVC are expressed as a percentage of pre-exercise value. MVC after the control session (without fatiguing exercise, n = 6) is also reported. *** Significant difference from pre-exercise (p < 0.001). MVC = maximal voluntary contraction; MVC1-MIN = 1-min sustained MVC; Pre = before the fatiguing exercise; Post = immediately after the fatiguing exercise; Post-10 = after the 10-min rest period following the fatiguing exercise.
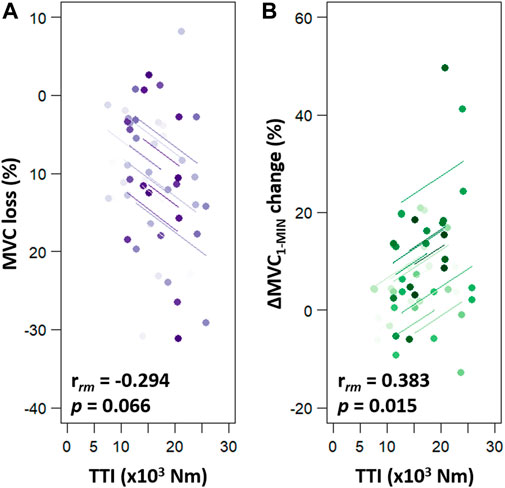
FIGURE 3. Repeated measures correlations (rmcorr) between exercise TTI and torque production changes (MVC loss, panel (A); ∆MVC1-MIN change, panel (B). MVC loss corresponds to the percent of MVC loss from before to after the fatiguing exercise. ∆MVC1-MIN change corresponds to the difference in ∆MVC1-MIN between before and after the fatiguing exercise. In each panel, four similar colour dots represent each participant’s data from each session sessions and coloured lines show rmcorr fits for each participant. MVC = maximal voluntary contraction; ∆MVC1-MIN = torque loss during a 1-min sustained MVC; TTI = exercise torque-time integral.
CAR and RMS
Before the fatiguing exercise, the CAR was similar among sessions (Table 1; Z-range: 0.314–0.734; all p = 1.000; ES-range: 0.087–0.204). The only exercise that had an impact on the CAR was the VOL 40% MVC (−3.5% ± 4.0%, Z = 3.18, p = 0.005, ES = 0.882). After the recovery period, the CAR was slightly lower, compared to the CAR calculated before the fatiguing exercise, for all sessions (EMS 20% MVC: −1.0% ± 1.1%, Z = 2.970, p = 0.009, ES = 0.824; VOL 20% MVC: −1.2% ± 1.2%, Z = 2.481, p = 0.039, ES = 0.688; EMS 40% MVC: −1.2% ± 0.9%, Z = 3.180, p = 0.004, ES = 0.882), except for the VOL 40% MVC (Z = 2.062, p = 0.118, ES = 0.572). For this latter session, the CAR seems to have completely recovered following the recovery period, even though the post-10 value was not different from the post exercise (Z = 1.49, p = 0.408, ES = 0.413) (Table 1).
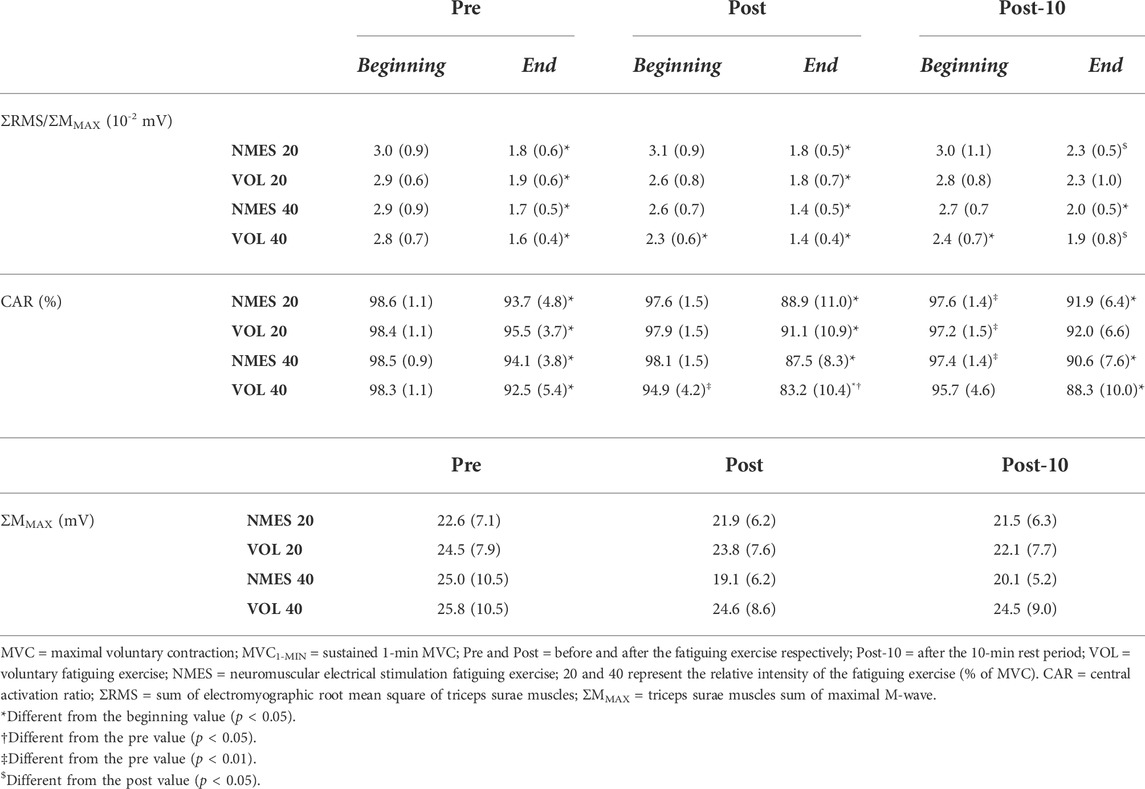
TABLE 1. Electrophysiological variables before (ΣMMAX), as well as at the beginning and the end (ΣRMS/ΣMMAX, CAR) of each MVC1-MIN for 13 subjects (mean (standard deviation)).
Before the exercise, no difference in ΣRMSMVC/ΣMMAX was detected among sessions (Table 1; Z-range: 0.078–1.020; all p = 1.000; ES-range: 0.022–0.283). The ratio was reduced only by the VOL 40% MVC exercise (−17.2% ± 15.2%, Z = 2.830, p = 0.014, ES = 0.785), and was still depressed after the 10-min recovery period (−14.5% ± 15.0%, Z = 2.481, p = 0.039, ES = 0.688) (Table 1).
MMAX
The ΣMMAX recorded at rest, before the fatiguing exercise, was similar among sessions, and was not modified neither by time and condition nor by the intensity (χ2 = 16.46, p = 0.125) (Table 1).
Assessments related to maximal torque sustainability
Torque
A significant interaction effect (intensity × time) was detected on the ∆MVC1-MIN (F2,24 = 8.988, p = 0.001, ηp2 = 0.428) (Figure 4). Before the fatiguing exercises, no difference in ∆MVC1-MIN was detected between the 20% and the 40% MVC sessions (p = 1.000, ES = 0.021). First, no change was observed following the 20% MVC exercises (pre = −36.72% ± 11.85% MVC vs. post = −40.86% ± 12.13% MVC, p = 0.196, ES = 0.345). Second, pooled data show that the decrease in torque during the 60-s MVC performed after the fatiguing exercise at 40% MVC (−49.10% ± 13.10%) was significantly greater than that observed before (−36.98% ± 12.36%, p < 0.001, ES = 0.952). In addition, the post 40% MVC exercise value was greater than the post 20% exercise one (p = 0.001, ES = 0.653). The 10 min of rest, after exercise at 40% MVC, allowed a complete recovery of the ∆MVC1-MIN (Post-10 = −38.67% ± 10.90% MVC vs. pre, p = 0.920, ES = 0.198) (Figure 4). Since there was no change in ∆MVC1-MIN after the 20% MVC exercises, no change was observed after the recovery period (−40.11% ± 13.11% MVC vs. pre, p = 0.389, ES = 0.271). Moreover, a positive correlation was detected (rrm (38) = 0.383, p = 0.015, CI95% = [0.072: 0.626]) between ∆MVC1-MIN change and exercise TTI (Figure 3B).
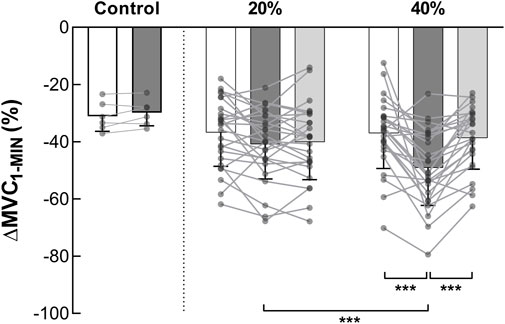
FIGURE 4. Torque loss during MVC1-MIN (n = 13). ∆MVC1-MIN represents the torque loss during the MVC1-MIN. Pooled ∆MVC1-MIN data (NMES and VOL) at pre (white columns), post fatiguing exercise (dark grey columns) and post 10-min recovery period (light grey columns) are expressed as percentage of the pre-exercise MVC. Control represents the session without fatiguing exercise. *** Significant difference (p < 0.001). MVC = maximal voluntary contraction; MVC1-MIN = 1-min sustained MVC.
CAR and RMS
The ∆CAR1-MIN was similar, before the fatiguing exercise, for all sessions (range: −2.98%—−6.01%; Z-range: 0.035–1.922; p-range: 0.328–1.000; ES-range: 0.010–0.533) (Figure 5A). Considering the fatiguing task, only the 40% MVC exercises had an impact on the ∆CAR1-MIN (VOL: pre = −6.01% ± 4.96% vs. post = −12.46% ± 9.46%, Z = 2.551, p = 0.032, ES = 0.708; NMES: pre = −4.55% ± 3.29% vs. post = −10.86% ± 7.84%, Z = 2.760 p = 0.017, ES = 0.765). The 10-min recovery period was sufficient to restore the ∆CAR1-MIN at its initial level after the 40% MVC exercises (Figure 5A). No difference in ∆CAR1-MIN between pre and post-10 was observed for the exercises at 20% MVC.
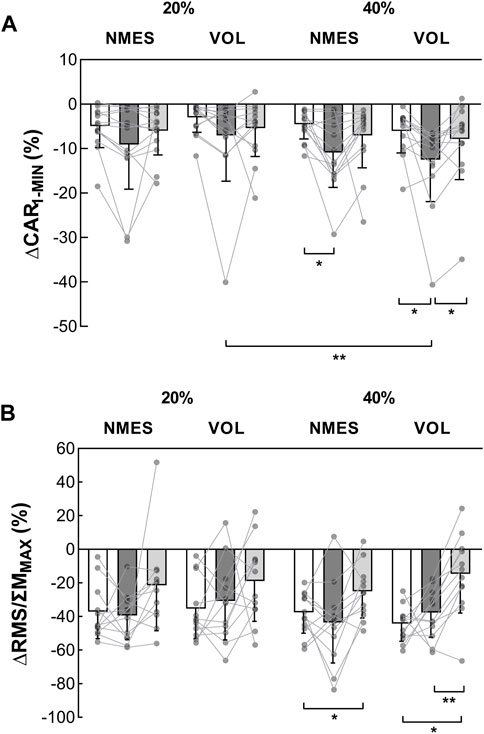
FIGURE 5. Evolution of voluntary activation indexes during the MVC1-MIN (n = 13). (A) ∆CAR1-MIN represents the reduction in CAR during the MVC1-MIN (%). (B) ∆RMS/ΣMMAX represents the evolution of the ΣRMS/ΣMMAX during the MVC1-MIN (%). These indexes were obtained before (white columns), after the fatiguing exercise (dark grey columns) and after the 10-min recovery period (light grey columns). *Significant difference (p < 0.05). **Significant difference (p < 0.01). MVC = maximal voluntary contraction; MVC1-MIN = 1-min sustained MVC; CAR = central activation ratio; ΣRMS = sum of EMG root mean square of triceps surae muscles; ΣMMAX = sum of triceps surae muscles maximal M-wave amplitude. VOL = voluntary fatiguing exercise; NMES = neuromuscular electrical stimulation fatiguing exercise; 20% and 40% MVC represent the intensity of the fatiguing exercise.
Before the fatiguing exercise, no difference in ∆RMS/ΣMMAX was detected among sessions (Figure 5B). Regardless of the session, the ∆RMS/ΣMMAX was not altered by the fatiguing exercise. After the recovery, following the exercise at 40% MVC, the ∆RMS/ΣMMAX was lower than before for both contraction modalities (NMES: pre = −37.6% ± 12.4% vs. post-10 = −25.0% ± 15.8%, Z = 2.510, p = 0.036, ES = 0.696; VOL: pre = −44.2% ± 10.4% vs. post-10 = −14.6% ± 23.3%, Z = 2.589, p = 0.029, ES = 0.718). No change was observed during the 20% MVC sessions.
Discussion
The main finding of this study is that maximal torque production and sustainability (respectively quantified by MVC and ∆MVC1-MIN) are differently altered by fatiguing exercises consisting of intermittent voluntary and electrically induced contractions, performed at low and moderate intensities. Our results show that, unlike the MVC loss, ∆MVC1-MIN is an index sensitive to the exercise TTI. Thus the association of these two indexes provide a more detailed description of the neuromuscular changes induced by a fatiguing exercise. In addition, the MVC1-MIN has the further advantage of allowing the simultaneous assessment of both maximal torque production and sustainability. Additionally, it has been observed that the recovery of the maximal torque sustainability is faster than the recovery of the maximal torque production capacity.
Maximal torque production
Since no difference in maximal torque production was observed between the brief MVC and the MVC1-MIN, performed before the fatiguing exercise, as well as an excellent reliability of this measure, it could be stated that the MVC1-MIN provides a reliable index of the maximal torque production capacity, at least in an unfatigued muscle.
Our findings showed that, regardless of the contraction modality (i.e., NMES or VOL), MVC was reduced by the same amount after 20% and 40% MVC exercises and this despite the greater TTI for the higher contraction intensity. This finding is in line with the extensive literature comparing reductions in maximal torque production induced by exercises with different muscle TTIs, performed until exhaustion (Hunter et al., 2008; Rudroff et al., 2010; Baudry et al., 2011) or not (Vitry et al., 2019). Taken together, the present analysis and the foregoing studies seem to suggest that the exercise with the greatest muscle TTI is the exercise that surprisingly generates the smallest decrease in maximal torque production, as highlighted when the MVC loss is normalized by the TTI. A rational explanation for this is that MVC loss is not sensitive to exercise TTI, as evidenced by the absence of a significant correlation between these two parameters.
For the same contraction intensity, namely for a similar TTI, our findings show that VOL and NMES protocols induced an equivalent MVC loss. This result is in accordance with those of Doix et al. (2014) which show that NMES and VOL contractions of PF, at moderate intensity, brought about a similar MVC loss, when their TTIs were matched. However, it is noteworthy that most of the studies observed a larger MVC loss after NMES compared to VOL exercise, with an equivalent TTI (Theurel et al., 2007; Jubeau et al., 2008). This disagreement could be attributed to differences in the experimental paradigms of these earlier investigations compared to ours (e.g., frequency and intensity of electrical stimulation, duty cycle, target torque and the investigated muscles). Additionally, NMES stimulations used by Jubeau et al. (2008) and Theurel et al. (2007), delivered at the maximally tolerated intensity, induced a greater soreness compared with the submaximal VOL exercises performed in these same studies. Discomfort due to NMES may be responsible for the greater MVC loss despite the TTI being equivalent in both contraction modalities.
The contribution of nervous and muscular factors to the MVC loss could be investigated with several methods and techniques. One of these methods consists of using the central activation ratio as an index of muscle activation since it reflects the capacity to activate all the available motor units. In the current investigation, no effect of the contraction modality was observed on the investigated neural indexes (i.e., CAR and ΣRMSMVC/ΣMMAX ratio) during the 20% MVC sessions, corroborating what was already observed by Doix et al. (2014). This indirectly suggests that the MVC loss could be mainly ascribed to muscular mechanisms. The absence of alteration in neuromuscular propagation (ΣMMAX), observed in the present study, suggests that changes in intracellular processes are implied. Such modifications that could have been assess by analysing the evoked twitches (Millet et al., 2012; Rozand et al., 2015) have not been done in the present study limiting in that way the direct assessment of alterations in muscle contractility.
On the other hand, at 40% MVC, a reduction in the CAR was observed, but only after the VOL exercise. Consequently, it seems likely that neural mechanisms contribute to the loss of MVC only after the VOL exercise at the higher intensity of contraction. This may, to some extent, be due to the fact that neural changes resulting from VOL exercise can be induced by both spinal and supraspinal mechanisms (Gandevia, 1998, 2001), while NMES, delivered at moderate intensity, seems to involve only supraspinal factors (Papaiordanidou et al., 2010; Alexandre et al., 2015).
As for the recovery, we observed that, whatever the fatiguing exercise, the maximal torque production capacity did not recover within 10-min rest. This observation is in accordance with data from several previous investigations showing that MVC was only partially restored after a rest period, even longer than 10 min (Jubeau et al., 2008; Chaubet et al., 2013). In our study, after the rest period, CAR values were still lower than pre-exercise, except for the VOL exercise at 40% MVC. However, in this session the CAR value at post-10 was not different from that post-exercise, and the latter was lower than that before the fatiguing exercise. Thus, it seems reasonable to presume that, overall, CAR did not recover after 10-min rest, this means that neural factors are responsible for the incomplete recovery of MVC.
Maximal torque sustainability
The choice to set the duration of the sustained MVC to 1 min was made because the greatest torque loss generally occurs during the first minute of contraction (Gandevia et al., 1996; Kent-Braun, 1999; Post et al., 2009) Thus, this time frame makes the sustained MVC a useful index of maximal torque sustainability. The ∆MVC1-MIN that we observed before the fatiguing exercise was about −37%, in accordance with the results of Moritani et al. (1985) which found a torque decline of −30%, in the same muscle group, after 60-s sustained contraction.
In order to investigate the nervous contribution to the torque loss during the MVC1-MIN, we analysed the CAR modification during this sustained contraction (∆CAR1-MIN). The ∆CAR1-MIN was about −4% between the beginning and the end of the pre-exercise MVC1-MIN. Some studies showed that nervous alterations appear approximately 45 s after the onset of the MVC1-MIN (Bigland-Ritchie et al., 1978; Schillings et al., 2003), explaining the small contribution of neural adjustments to the torque reduction throughout a maximal contraction of 1-min duration. The major part of the torque loss during the 1-min sustained contraction could, therefore, be accounted for by muscular changes. This result is in line with previous investigations which found that the greatest part of torque loss during a 1- to 3-min MVC is due to muscular alterations (Gandevia et al., 1996; Kent-Braun, 1999; Schillings et al., 2003; Place et al., 2007; Post et al., 2009; Vera-Ibáñez et al., 2018). In the present study, the maximal M-wave was not altered by the pre-exercise MVC1-MIN, as observed in previous studies for 45–120 s sustained MVC (Bigland-Ritchie et al., 1978; Place et al., 2007; Vera-Ibáñez et al., 2018). This finding indicates that the MVC loss should not depend on a change in neuromuscular transmission and propagation.
The current results showed that maximal torque sustainability was affected by the fatiguing exercise at 40% MVC, for both contraction modalities. Repeated measures correlation analysis confirms that the exercise TTI affects the neuromuscular sustainability, validating the hypothesis that ∆MVC1-MIN is sensitive to muscle TTI, at least for submaximal exercises. This means, as expected, that the higher the TTI generated by the fatiguing exercise, the greater the torque loss during the sustained MVC. This also implies that the physiological mechanisms involved in protracting the MVC were more impaired after the exercise with the greatest TTI (i.e., 40% MVC). To better understand the underlying mechanisms, we examined changes in muscle activation during the sustained MVC. Our results showed that the greater ∆MVC1-MIN, observed after the exercise at 40% MVC was associated with a greater ∆CAR1-MIN. Thus, the reduction in maximal torque sustainability may, at least in part, be attributed to a reduction in muscle activation capacity. This could be explained by the fact that the higher exercise intensity may cause a greater occlusion of blood vessels and thus reduce the muscle metabolite wash-out, compared with the lower intensity (Sejersted et al., 1984). The by-products accumulation during the fatiguing exercises could increase the stimulation of muscle afferents (in particular group III/IV muscle afferents) by receptors sensitives to mechanic and metabolic changes (Garland and Kaufman, 1995). These muscle afferents are known to have an inhibitory effect on the motor cortex, limiting the efferent motor command (Gandevia et al., 1996; Taylor et al., 2016). The lower intramuscular pressure induced by the 20% MVC contractions, compared with the 40% MVC contractions (Osada et al., 2015), leads to a lower accumulation of muscle metabolites, this could explain why the maximal torque sustainability was not altered by the fatiguing exercises performed at 20% MVC.
In addition, the greater muscle oxygen consumption, observed by Pethick et al. (2019) during an intermittent exercise at 40% MVC compared to 20% MVC, could be a further explanation for the different behaviour of the ∆MVC1-MIN between these two intensities of exercise. Furthermore, the contribution of glycogen, as energy supply to produce adenosine triphosphate, increases with the intensity of the exercise (Holloszy et al., 1998). The glycogen is the main energy substrate between the sixth and the 50th second of a MVC1-MIN (Lanza et al., 2005) and its depletion could partly account for the greater ∆MVC1-MIN following the 40% MVC fatiguing exercises.
Concerning the contraction modalities, we observed a similar ∆MVC1-MIN following the NMES and VOL exercises, whether the contraction intensity was 20% or 40% MVC. The reason could be that the muscle TTI was similar for the NMES and VOL exercises, at each contraction intensity. Hence, maximal torque sustainability seems to be mainly sensitive to muscle TTI than to contraction modality, at least when the fatiguing exercise is performed at low and moderate intensities.
We also investigated the recovery of maximal torque sustainability and we observed that the increased ∆MVC1-MIN, found after the exercise performed at 40% MVC (in both modalities), returned to baseline values after the rest period of 10 min. This suggests that this time frame is enough for a full recovery of maximal torque sustainability. The latter result allows us to refute the hypothesis according to which the exercises at 40% MVC induce a greater glycogen depletion. Indeed, the rapid and complete recovery of ∆MVC1-MIN after the short rest period is inconsistent with the slow process of glycogen restoration. As observed by Robergs et al. (1991) it occurs hours for a complete glycogen renewal after an intermittent exercise at 35% MVC of the quadriceps muscle.
As previously mentioned, the partial occlusion of muscle blood flow, during the exercises at 40% MVC, may lead to the accumulation of by-products from metabolic processes reducing the central activation, with repercussions on the ∆MVC1-MIN. However, in a fatigued muscle, restoration of blood flow after circulatory occlusion attenuates the central inhibitory effect of group III/IV muscle afferent firing, which allows the recovery of voluntary activation capacity in ∼30 s (Kennedy et al., 2015). In our study, the ∆CAR1-MIN, after the 10-min rest period, returned to pre-exercise value for both VOL and NMES session at 40% MVC. This result supports the statement that the voluntary activation has a critical role in the maximal torque sustainability.
Perspectives
The current study showed that MVC loss and ∆MVC1-MIN have different evolution during various submaximal intermittent fatiguing exercises, as well as during a 10-min recovery period. Their divergent behaviour reveals that maximal torque sustainability, unlike maximal torque production, is sensitive to the exercise TTI. This highlights the importance to assess maximal torque sustainability, in addition to maximal torque production, in order to determine the neuromuscular impact of different fatiguing exercises. The MVC1-MIN, contrary to the brief MVC, has the advantage to provide simultaneously a reliable index of maximal torque production capacity and an assessment of maximal torque sustainability before and after the investigated muscle exercise. This protocol, using only a few peripheral nerve stimulations superimposed to the MVC1-MIN, is easy to set up in both healthy and pathological populations to monitor muscle training and deconditioning, and to discriminate neural and muscular contributions to torque production (Schillings et al., 2003). However, further investigations should be carried out to elucidate how these two physiological features (i.e., maximal torque production and sustainability) are modified as a result of different fatiguing protocols and recovery periods in main muscle groups. Finally, some computational models have been developed e.g., (Callahan et al., 2013, 2016; Eriksson et al., 2016; Herold et al., 2018; Rockenfeller et al., 2020) to predict the neuromuscular fatigability induced by different contraction conditions. Thus, it would be interesting to evaluate whether these predictive simulations fit with our experimental data. Also, it would be an interesting project in the future to see if such a model could predict the relation between exercise TTI and maximal torque sustainability we identified in the present study.
Data availability statement
The raw data supporting the conclusion of this article will be made available by the authors, without undue reservation.
Ethics statement
The studies involving human participants were reviewed and approved by CPP SOOM III. The patients/participants provided their written informed consent to participate in this study.
Author contributions
All authors: Study conception and design. LL: Data acquisition and analysis. All authors: Data interpretations. LL: Drafting of manuscript. All authors: Critical revision of the manuscript for important intellectual content.
Funding
This work was supported by the Regional Council of Bourgogne Franche-Comté.
Acknowledgments
The authors would like to thank all volunteers that participated in the study.
Conflict of interest
The authors declare that the research was conducted in the absence of any commercial or financial relationships that could be construed as a potential conflict of interest.
Publisher’s note
All claims expressed in this article are solely those of the authors and do not necessarily represent those of their affiliated organizations, or those of the publisher, the editors and the reviewers. Any product that may be evaluated in this article, or claim that may be made by its manufacturer, is not guaranteed or endorsed by the publisher.
Abbreviations
∆CAR1-MIN, Change in CAR between during the MVC1-MIN; ∆MVC1-MIN, Torque change during the MVC1-MIN; ∆RMS/ΣMMAX, Change in muscle activity during the MVC1-MIN; ANOVA, Analysis of variance; CAR, Central activation ratio; EMG, Electromyographic activity; ES, Effect size; GL, Gastrocnemius lateralis; GM, Gastrocnemius medialis; ICC, Intraclass correlation coefficient; MMAX, Maximal M-wave; MVC, Maximal voluntary contraction; MVC1-MIN, 1-min maximal voluntary contraction; NMES, Neuromuscular electrical stimulation; PF, Plantar flexors; Rmcorr, repeated measures correlation; RMS, Root mean square; SOL, Soleus; TA, Tibialis anterior; TTI, Torque-time integral; VOL, Voluntary contraction; ΣMMAX, Sum of maximal M-wave of soleus and gastrocnemii; ΣRMS, Sum of EMG root mean square of soleus and gastrocnemii.
References
Alexandre F., Derosiere G., Papaiordanidou M., Billot M., Varray A. (2015). Cortical motor output decreases after neuromuscular fatigue induced by electrical stimulation of the plantar flexor muscles. Acta Physiol. 214, 124–134. doi:10.1111/apha.12478
Amann M., Venturelli M., Ives S. J., McDaniel J., Layec G., Rossman M. J., et al. (2013). Peripheral fatigue limits endurance exercise via a sensory feedback-mediated reduction in spinal motoneuronal output. J. Appl. Physiol. 115, 355–364. doi:10.1152/japplphysiol.00049.2013
Bakdash J. Z., Marusich L. R. (2017). Repeated measures correlation. Front. Psychol. 8, 456. doi:10.3389/fpsyg.2017.00456
Baudry S., Maerz A. H., Gould J. R., Enoka R. M. (2011). Task- and time-dependent modulation of Ia presynaptic inhibition during fatiguing contractions performed by humans. J. Neurophysiol. 106, 265–273. doi:10.1152/jn.00954.2010
Bigland-Ritchie B., Jones D. A., Hosking G. P., Edwards R. H. (1978). Central and peripheral fatigue in sustained maximum voluntary contractions of human quadriceps muscle. Clin. Sci. Mol. Med. 54, 609–614. doi:10.1042/cs0540609
Boska M. D., Moussavi R. S., Carson P. J., Weiner M. W., Miller R. G. (1990). The metabolic basis of recovery after fatiguing exercise of human muscle. Neurology 40, 240–244. doi:10.1212/wnl.40.2.240
Boyas S., Guével A. (2011). Neuromuscular fatigue in healthy muscle: underlying factors and adaptation mechanisms. Ann. Phys. Rehabil. Med. 54, 88–108. doi:10.1016/j.rehab.2011.01.001
Byrne C., Eston R. (2002). Maximal-intensity isometric and dynamic exercise performance after eccentric muscle actions. J. Sports Sci. 20, 951–959. doi:10.1080/026404102321011706
Callahan D. M., Umberger B. R., Kent J. A. (2016). Mechanisms of in vivo muscle fatigue in humans: investigating age‐related fatigue resistance with a computational model. J. Physiol. 594, 3407–3421. doi:10.1113/JP271400
Callahan D. M., Umberger B. R., Kent-Braun J. A. (2013). A computational model of torque generation: neural, contractile, metabolic and musculoskeletal components. PLOS ONE 8, e56013. doi:10.1371/journal.pone.0056013
Chaubet V., Cormery B., Maitre J., Paillard T. (2013). Stimulated contractions delay and prolong central fatigue compared with voluntary contractions in men. J. Strength Cond. Res. 27, 1378–1383. doi:10.1519/JSC.0b013e318265a271
Davis J. M., Bailey S. P. (1997). Possible mechanisms of central nervous system fatigue during exercise. Med. Sci. Sports Exerc. 29, 45–57. doi:10.1097/00005768-199701000-00008
Doix A-C. M., Matkowski B., Martin A., Roeleveld K., Colson S. S. (2014). Effect of neuromuscular electrical stimulation intensity over the tibial nerve trunk on triceps surae muscle fatigue. Eur. J. Appl. Physiol. 114, 317–329. doi:10.1007/s00421-013-2780-y
Edwards R. H. T. (1981). “Human muscle function and fatigue,” in Human muscle fatigue: Physiological Mechanisms. John Wiley & Sons, 1–18.
Eriksson A., Holmberg H-C., Westerblad H. (2016). A numerical model for fatigue effects in whole-body human exercise. Math. Comput. Model. Dyn. Syst. 22, 21–38. doi:10.1080/13873954.2015.1083592
Fritz C. O., Morris P. E., Richler J. J. (2012). Effect size estimates: Current use, calculations, and interpretation. J. Exp. Psychol. Gen. 141, 2–18. doi:10.1037/a0024338
Gandevia S. C., Allen G. M., Butler J. E., Taylor J. L. (1996). Supraspinal factors in human muscle fatigue: evidence for suboptimal output from the motor cortex. J. Physiol. 490 ( Pt 2), 529–536. doi:10.1113/jphysiol.1996.sp021164
Gandevia S. C. (1998). Neural control in human muscle fatigue: changes in muscle afferents, motoneurones and motor cortical drive [corrected]. Acta Physiol. Scand. 162, 275–283. doi:10.1046/j.1365-201X.1998.0299f.x
Gandevia S. C. (2001). Spinal and supraspinal factors in human muscle fatigue. Physiol. Rev. 81, 1725–1789. doi:10.1152/physrev.2001.81.4.1725
Garland S. J., Kaufman M. P. (1995). Role of muscle afferents in the inhibition of motoneurons during fatigue. Adv. Exp. Med. Biol. 384, 271–278. doi:10.1007/978-1-4899-1016-5_21
Hachard B., Noe F., Catherine A., Zeronian Z., Paillard T. (2019). Voluntary and electrically-induced muscle fatigue differently affect postural control mechanisms in unipedal stance. Exp. Brain Res. 237, 313–323. doi:10.1007/s00221-018-5418-9
Halperin I., Aboodarda S. J., Basset F. A., Byrne J. M., Behm D. G. (2014). Pacing strategies during repeated maximal voluntary contractions. Eur. J. Appl. Physiol. 114, 1413–1420. doi:10.1007/s00421-014-2872-3
Herold J. L., Kirches C., Schlöder J. P. (2018). A phenomenological model of the time course of maximal voluntary isometric contraction force for optimization of complex loading schemes. doi:10.1101/256578
Holloszy J. O., Kohrt W. M., Hansen P. A. (1998). The regulation of carbohydrate and fat metabolism during and after exercise. Front. Biosci. 3, D1011–D1027. doi:10.2741/a342
Hunter S. K., Yoon T., Farinella J., Griffith E. E., Ng A. V. (2008). Time to task failure and muscle activation vary with load type for a submaximal fatiguing contraction with the lower leg. J. Appl. Physiol. 105, 463–472. doi:10.1152/japplphysiol.90398.2008
Jubeau M., Sartorio A., Marinone P. G., Agosti F., Hoecke J. V., Nosaka K., et al. (2008). Comparison between voluntary and stimulated contractions of the quadriceps femoris for growth hormone response and muscle damage. J. Appl. Physiol. 104, 75–81. doi:10.1152/japplphysiol.00335.2007
Kennedy D. S., Fitzpatrick S. C., Gandevia S. C., Taylor J. L. (2015). Fatigue-related firing of muscle nociceptors reduces voluntary activation of ipsilateral but not contralateral lower limb muscles. J. Appl. Physiol. 118, 408–418. doi:10.1152/japplphysiol.00375.2014
Kent-Braun J. A. (1999). Central and peripheral contributions to muscle fatigue in humans during sustained maximal effort. Eur. J. Appl. Physiol. Occup. Physiol. 80, 57–63. doi:10.1007/s004210050558
Kent-Braun J. A., Fitts R. H., Christie A. (2012). Skeletal muscle fatigue. Compr. Physiol. 2, 997–1044. doi:10.1002/cphy.c110029
Kluger B. M., Krupp L. B., Enoka R. M. (2013). Fatigue and fatigability in neurologic illnesses: proposal for a unified taxonomy. Neurology 80, 409–416. doi:10.1212/WNL.0b013e31827f07be
Koo T. K., Li M. Y. (2016). A guideline of selecting and reporting intraclass correlation coefficients for reliability research. J. Chiropr. Med. 15, 155–163. doi:10.1016/j.jcm.2016.02.012
Lanza I. R., Befroy D. E., Kent-Braun J. A. (2005). Age-related changes in ATP-producing pathways in human skeletal muscle in vivo. J. Appl. Physiol. 99, 1736–1744. doi:10.1152/japplphysiol.00566.2005
Millet G. Y., Bachasson D., Temesi J., Wuyam B., Féasson L., Vergès S., et al. (2012). Potential interests and limits of magnetic and electrical stimulation techniques to assess neuromuscular fatigue. Neuromuscul. Disord. 22, S181–S186. doi:10.1016/j.nmd.2012.10.007
Millet G. Y., Martin V., Martin A., Vergès S. (2011). Electrical stimulation for testing neuromuscular function: from sport to pathology. Eur. J. Appl. Physiol. 111, 2489–2500. doi:10.1007/s00421-011-1996-y
Moritani T., Muro M., Kijima A., Gaffney F. A., Parsons D. (1985). Electromechanical changes during electrically induced and maximal voluntary contractions: surface and intramuscular EMG responses during sustained maximal voluntary contraction. Exp. Neurol. 88, 484–499. doi:10.1016/0014-4886(85)90065-2
Muthalib M., Jubeau M., Millet G. Y., Maffiuletti N. A., Ferrari M., Nosaka K. (2010). Biceps brachii muscle oxygenation in electrical muscle stimulation. Clin. Physiol. Funct. Imaging 30, 360–368. doi:10.1111/j.1475-097X.2010.00953.x
Osada T., Mortensen S. P., Rådegran G. (2015). Mechanical compression during repeated sustained isometric muscle contractions and hyperemic recovery in healthy young males. J. Physiol. Anthropol. 34, 36. doi:10.1186/s40101-015-0075-1
Papaiordanidou M., Guiraud D., Varray A. (2010). Does central fatigue exist under low-frequency stimulation of a low fatigue-resistant muscle? Eur. J. Appl. Physiol. 110, 815–823. doi:10.1007/s00421-010-1565-9
Pethick J., Winter S. L., Burnley M. (2019). Relationship between muscle metabolic rate and muscle torque complexity during fatiguing intermittent isometric contractions in humans. Physiol. Rep. 7, e14240. doi:10.14814/phy2.14240
Place N., Maffiuletti N. A., Martin A., Lepers R. (2007). Assessment of the reliability of central and peripheral fatigue after sustained maximal voluntary contraction of the quadriceps muscle. Muscle Nerve 35, 486–495. doi:10.1002/mus.20714
Post M., Steens A., Renken R., Maurits N. M., Zijdewind I. (2009). Voluntary activation and cortical activity during a sustained maximal contraction: An fMRI study. Hum. Brain Mapp. 30, 1014–1027. doi:10.1002/hbm.20562
Robergs R. A., Pearson D. R., Costill D. L., Fink W. J., Pascoe D. D., Benedict M. A., et al. (1991). Muscle glycogenolysis during differing intensities of weight-resistance exercise. J. Appl. Physiol. 70, 1700–1706. doi:10.1152/jappl.1991.70.4.1700
Robert H., Casillas J. M., Iskandar M., D’Athis P., Antoine D., Taha S., et al. (2004). [The dijon Physical activity score: reproducibility and correlation with exercise testing in healthy elderly subjects]. Ann. Readapt. Med. Phys. 47, 546–554. doi:10.1016/j.annrmp.2004.03.005
Rockenfeller R., Günther M., Stutzig N., Haeufle D. F. B., Siebert T., Schmitt S., et al. (2020). Exhaustion of skeletal muscle fibers within seconds: incorporating phosphate kinetics into a hill-type model. Front. Physiol. 11, 306. doi:10.3389/fphys.2020.00306
Rozand V., Grosprêtre S., Stapley P. J., Lepers R. (2015). Assessment of neuromuscular function using Percutaneous electrical nerve stimulation. J. Vis. Exp. doi:10.3791/52974
Rudroff T., Justice J. N., Matthews S., Zuo R., Enoka R. M. (2010). Muscle activity differs with load compliance during fatiguing contractions with the knee extensor muscles. Exp. Brain Res. 203, 307–316. doi:10.1007/s00221-010-2233-3
Šambaher N., Aboodarda S. J., Behm D. G. (2016). Bilateral knee extensor fatigue modulates force and responsiveness of the corticospinal pathway in the non-fatigued, dominant elbow flexors. Front. Hum. Neurosci. 10, 18. doi:10.3389/fnhum.2016.00018
Schillings M. L., Hoefsloot W., Stegeman D. F., Zwarts M. J. (2003). Relative contributions of central and peripheral factors to fatigue during a maximal sustained effort. Eur. J. Appl. Physiol. 90, 562–568. doi:10.1007/s00421-003-0913-4
Sejersted O. M., Hargens A. R., Kardel K. R., Blom P., Jensen O., Hermansen L. (1984). Intramuscular fluid pressure during isometric contraction of human skeletal muscle. J. Appl. Physiol. Respir. Environ. Exerc. Physiol. 56, 287–295. doi:10.1152/jappl.1984.56.2.287
Taylor J. L., Amann M., Duchateau J., Meeusen R., Rice C. L. (2016). Neural contributions to muscle fatigue: From the Brain to the muscle and back again. Med. Sci. Sports Exerc. 48, 2294–2306. doi:10.1249/MSS.0000000000000923
Theurel J., Lepers R., Pardon L., Maffiuletti N. A. (2007). Differences in cardiorespiratory and neuromuscular responses between voluntary and stimulated contractions of the quadriceps femoris muscle. Respir. Physiol. Neurobiol. 157, 341–347. doi:10.1016/j.resp.2006.12.002
Vera-Ibáñez A., Romero-Arenas S., Marín-Pagán C., Márquez G. (2018). Concurrencia de fatiga y potenciación tras una contracción voluntaria máxima sostenida/Concurrence of Fatigue and Potentiation after A Sustained Maximal Voluntary Contraction. rimcafd. 69. doi:10.15366/rimcafd2018.69.004
Keywords: performance fatigability, maximal torque production, maximal torque sustainability, voluntary contraction, neuromuscular electrical stimulation, torque-time integral
Citation: Lebesque L, Scaglioni G and Martin A (2022) The impact of submaximal fatiguing exercises on the ability to generate and sustain the maximal voluntary contraction. Front. Physiol. 13:970917. doi: 10.3389/fphys.2022.970917
Received: 16 June 2022; Accepted: 04 August 2022;
Published: 02 September 2022.
Edited by:
Tobias Siebert, University of Stuttgart, GermanyReviewed by:
Daniel F. B. Haeufle, University of Tübingen, GermanySaied Jalal Aboodarda, University of Calgary, Canada
Copyright © 2022 Lebesque, Scaglioni and Martin. This is an open-access article distributed under the terms of the Creative Commons Attribution License (CC BY). The use, distribution or reproduction in other forums is permitted, provided the original author(s) and the copyright owner(s) are credited and that the original publication in this journal is cited, in accordance with accepted academic practice. No use, distribution or reproduction is permitted which does not comply with these terms.
*Correspondence: Loïc Lebesque, bG9pYy5sZWJlc3F1ZUB1LWJvdXJnb2duZS5mcg==