- 1Embrapa Suínos e Aves, Concórdia, Brazil
- 2Programa de Pós-Graduação em Ciências Veterinárias, Universidade Estadual do Centro-Oeste, Guarapuava, Brazil
- 3Universidade de Passo Fundo, Passo Fundo, Brazil
- 4Universidade Federal do Vale do São Francisco, UNIVASF, Petrolina, Brazil
- 5Laboratório de Biotecnologia Animal, Escola Superior de Agricultura “Luiz de Queiroz”, Universidade de SP, Piracicaba, Brazil
- 6Departamento de Genética, Faculdade de Medicina de Ribeirão Preto, Universidade de SP, Ribeirão Preto, Brazil
- 7Programa de Pós-Graduação Em Zootecnia, Universidade do Estado de SC, UDESC-Oeste, Chapecó, Brazil
- 8Faculdade de Concórdia FACC, Concórdia, Brazil
Femoral head separation (FHS) is characterized by the detachment of growth plate (GP) and articular cartilage, occurring in tibia and femur. However, the molecular mechanisms involved with this condition are not completely understood. Therefore, genes and biological processes (BP) involved with FHS were identified in 21-day-old broilers through RNA sequencing of the femoral GP. 13,487 genes were expressed in the chicken femoral head transcriptome of normal and FHS-affected broilers. From those, 34 were differentially expressed (DE; FDR ≤0.05) between groups, where all of them were downregulated in FHS-affected broilers. The main BP were enriched in receptor signaling pathways, ossification, bone mineralization and formation, skeletal morphogenesis, and vascularization. RNA-Seq datasets comparison of normal and FHS-affected broilers with 21, 35 and 42 days of age has shown three shared DE genes (FBN2, C1QTNF8, and XYLT1) in GP among ages. Twelve genes were exclusively DE at 21 days, where 10 have already been characterized (SHISA3, FNDC1, ANGPTL7, LEPR, ENSGALG00000049529, OXTR, ENSGALG00000045154, COL16A1, RASD2, BOC, GDF10, and THSD7B). Twelve SNPs were associated with FHS (p < 0.0001). Out of those, 5 were novel and 7 were existing variants located in 7 genes (RARS, TFPI2, TTI1, MAP4K3, LINK54, and AREL1). We have shown that genes related to chondrogenesis and bone differentiation were downregulated in the GP of FHS-affected young broilers. Therefore, these findings evince that candidate genes pointed out in our study are probably related to the onset of FHS in broilers.
Introduction
Leg problems are considered one of the main issues in the chicken production (Li et al., 2015). The occurrence of these disorders in commercial flocks was estimated up to 30% depending on age, density, within others, having a huge impact on animal health and welfare (Knowles et al., 2008; Federici et al., 2016). Femoral head necrosis (FHN) can affect up to 75 and 88% of commercial broilers with 28 and 42 days of age, respectively, being reported as one of the major bone pathologies (McNamee and Smyth, 2000; Durairaj et al., 2009; Wideman, 2016; Liu K. et al., 2021). Some authors classified FHN depending on its severity, such as femoral head separation (FHS), when the growth plate is separated from cartilage and femoral head separation with growth plate lacerations (FHSL), when there are lesions in the femoral growth plate (Li et al., 2015; Packialakshmi et al., 2015). This condition is also known as bacterial chondronecrosis with osteomyelitis (BCO) when it is associated with bacterial infection (Jiang et al., 2015; Al-rubaye et al., 2017; Ramser et al., 2021).
The FHS is a multifactorial disorder, which could be caused by traumatic or non-traumatic factors (Liu K. et al., 2021). It is characterized by a degenerative skeletal problem presenting focal cell death, fibrotic thickening, and atrophic changes in the cartilage (Packialakshmi et al., 2015). Furthermore, some authors consider FHS as a primary cartilage defect, since reduced chondrocyte density was observed in the GP of affected broilers (Wilson et al., 2019). These failures lead to cartilage degeneration and necrosis, which predispose animals to develop FHN (Packialakshmi et al., 2015; Wilson et al., 2019). The growth plate chondrocytes are required to the proper development and growth of endochondral bones (Hallett et al., 2019). The disruption in homeostasis of bone formation and resorption through the imbalance of catabolic and anabolic factors (Wnt signaling, HIF expression, extracellular matrix production, within others) was described in broilers with 42 and 56 days of age (Yu et al., 2020). Therefore, the understanding of the GP molecular mechanisms is essential to clarify the genes responsible for triggering FHS/FHN in chickens.
Over the years, global gene expression studies have provided a better understanding of the putative acting genes in the two tissues that are the primary sites of femoral head disorders. From transcriptomes of the femur articular cartilage (AC) and growth plate (GP) of 35-day-old broilers (Peixoto et al., 2019; Hul et al., 2021; Goldoni et al., 2022), several candidate genes were prospected. Oliveira et al. (2020) also pointed out that osteochondral downregulated genes are potentially triggering BCO in tibia of 42-day-old broilers. Most of the studies with FHN/FHS in chickens are focused on understanding the ossification processes and, generally, in animals older than 35 days of age (Li et al., 2015; Paludo et al., 2017; Peixoto et al., 2019; Hul et al., 2021; Ramser et al., 2021, 2022), since the highest incidences of this condition occur at these ages (McNamee and Smyth, 2000; Paxton et al., 2014; Packialakshmi et al., 2015). Therefore, molecular mechanisms involved with femoral head separation in young age (21 days) broilers were identified using RNA sequencing, allowing highlighting genes and BP potentially related to the onset of this condition.
Material and methods
Experimental animals and sample collection
Cobb500 male broilers used in this study were raised in a standard system for commercial growing broilers with the management conditions following the recommendations for this line, with water and feed supplied ad libitum. At 21 days of age, 15 lameness and 10 normal standard broilers were weighed and euthanized by cervical dislocation followed by exsanguination, according to the ethical guidelines of the Embrapa Swine and Poultry Ethics Committee on Animal Utilization, under the protocol number 012/2012. Immediately after the slaughter, femurs were evaluated by the presence or absence of FHS, following the methodology described by Paludo et al. (2017). Chickens were considered with FHS when there was separation of the articular cartilage from the growth plate without any visual signal of necrosis, and normal when the AC remained attached to the GP. Samples of one of the femur proximal growth plates were collected individually, immerged in liquid nitrogen and stored at −80°C for further expression analysis.
Total RNA extraction and quantification
For the RNA-Seq analysis, 4 normal and 4 FHS-affected femur growth plates were chosen and 100 mg of this tissue was homogenized using a mortar with liquid nitrogen. The total RNA was extracted using Trizol (Life Technologies, Carlsbad, United States), according to the manufacturer’s protocol, followed by a RNA cleanup step using the RNeasy mini kit (Qiagen, Hilden, Germany). RNA purity and concentration were measured using Biodrop spectrophotometer (Biodrop, UK, and integrity was evaluated using Bioanalyzer 2,100 (Agilent, Santa Clara, United States). Only samples with RIN >8.0 were considered for further analysis.
Library preparation and sequencing
Libraries were prepared using TruSeq RNA Sample Prep v2 Kit (Illumina, San Diego, United States ) with 2 μg of total RNA, following the manufacturer´s protocol. The quality of the libraries was evaluated using Bioanalyzer 2,100 (Agilent, Santa Clara, United States ). Libraries were quantified through quantitative PCR using the KAPA Library Quantification kit (KAPA Biosystems, Wilmington, United States ). After pooling the libraries, clustering and sequencing were performed in the Illumina HiSeq 2,500 sequencer (lllumina, San Diego, United States ) producing 2 × 100 bp paired-end reads, at the Functional Genomics Center from ESALQ-USP, Piracicaba, São Paulo, Brazil.
RNA-seq data analysis
The RNA-seq analysis was performed using BAQCOM, an automated pipeline available at https://github.com/hanielcedraz/BAQCOM (accession on 15th February 2022). Low quality reads (QPhred ≤20), short reads (<70 bp) and Illumina sequence adapters were trimmed using Trimmomatic v0.39 (Bolger et al., 2014). After the quality control, sequence reads of each sample were mapped against the reference chicken genome (GRCg6a, Ensembl release 105) using STAR (Dobin et al., 2013). Reads counting was performed with HTseq-count (Anders et al., 2015), which also generated a count table for statistical analysis. The differentially expressed (DE) genes and heatmap were obtained using the limma package (Ritchie et al., 2015) from the R software (R Core Team, 2021). The Benjamini-Hochberg (BH) methodology (Benjamini and Hochberg, 1995) was applied to correct for multiple testing and genes with false discovery rate (FDR) ≤ 0.05 were considered DE. Negative and positive fold-changes indicate downregulation and upregulation of the genes in the FHS-affected group compared to control group.
Functional annotation analysis
Functional annotation of DE genes was performed using clusterProfiler (Wu et al., 2021) with MSigDB (Liberzon et al., 2011) and gene ontology with Gallus gallus information (org.Gg.eg.db) available at AnnotationDBbi (Pagès et al., 2022) and also in shinyGO v 0.75 (Ge et al., 2020). A search was also performed in the DAVID database, with all genes expressed in the samples used as background, and values of EASE ≤ 0.05 and p-values ≤ 0.05 were considered significant. A gene interaction analysis was performed with the STRING database (Szklarczyk et al., 2019) in Cytoscape v 3.9 (Shannon et al., 2003) with G. gallus organism using two approaches: 1) only with DE genes and 2) with DE genes plus 5 additional gene interactors.
Additionally, in order to verify the FHS gene expression profile among different ages, Ensembl IDs of DE genes identified in this study were compared with results from transcriptome studies of Peixoto et al. (2019), Oliveira et al. (2020), Hul et al. (2021) and Goldoni et al. (2022). These studies evaluated early stages of FHS at 35 days in growth plate and articular cartilage (Peixoto et al., 2019; Hul et al., 2021; Goldoni et al., 2022) and tibia head separation at 42 days of age (Oliveira et al., 2020).
qPCR validation
The qPCR analysis was performed with the same 8 GP samples used in the RNA-seq analysis: 4 normal and 4 FHS-affected broilers with 21 days of age. The RNA was extracted as previously described and cDNA synthesis was performed using the SuperScript III First–Strand Synthesis SuperMix kit (Invitrogen, Carslbad, United States ). Primers for COL14A1, FAM180A, ANGPTL5, FBN2, TNMD, and LEPR candidate genes were used for RNA-Seq confirmation (Table 1). These genes were chosen based on their FDR, logFC, and biological function. Quantitative PCR reactions were performed in the QuantStudio 6 Flex equipment (Applied Biosystems), containing 1 × Mastermix (GoTaq qPCR Master Mix 2×, Promega), 0.16 µM of each F and R primer, 2 µl of cDNA at 1:10 dilution and ultrapure water to complete 15 µl of total reaction. The qPCR reactions were performed in duplicates and the Ct (cycle threshold) values were obtained for differential expression analysis in the REST program (Pfaffl et al., 2002), which uses a nonparametric randomization test to compare the studied groups. For this analysis, Ct means for each sample and gene were used and normalization was performed with RPL4 and RPL30 reference genes (Petry et al., 2018). Differential expression was considered significant when p ≤ 0.05.
Unmapped read analysis
Part of the reads from RNA sequencing was not mapped in the chicken genome. To verify if those reads could be from microorganisms, the unmapped sequences were submitted to a metagenome taxonomic classification analysis with Metacache software v. 2.2.1 (Müller et al., 2017) with Refseq database version 20200820. Results from family and genera were further processed using the Phyloseq v.1.34.0 package (McMurdie and Holmes, 2013) from R, in which the raw tables were concatenated and only the taxa with more than 100 mapped reads across all samples and present in more than half of the samples were maintained. Abundance analysis was performed using a zero-inflated Gaussian (ZIG) mixed model after cumulative sum scaling (CSS) normalization using the metagenomeSeq package from R (Paulson et al., 2013). Differential abundance of the taxa between groups was considered significant when FDR ≤0.05. Graphics were generated with ggplot2 v. 3.3.3 package from R (Wickham, 2016). Metagenomic classification was registered in the Brazilian National System of Genetic Resources (SISGEN) under ID A62341D.
Variant identification using RNA-Seq data
RNA sequences obtained in this study, as well as sequences from other GP and cartilage from normal and FHS-affected samples from our previous studies (Peixoto et al., 2019; Oliveira et al., 2020; Hul et al., 2021; Goldoni et al., 2022) were used to verify the presence of variants involved with FHS. To this, sequences from femoral GP and AC of 35-day-old and tibia GP from 42-day-old broilers were downloaded from SRA database bioprojects # PRJNA352962, PRJNA350521, and PRJNA352716, respectively, totaling 24 samples (9 normal and 15 FHS-affected). Sequences were trimmed as described in the RNA-Seq analysis section, and mapping against the chicken reference genome (GRCg6a, Ensembl release 105) was performed using the two pass-mode in STAR software (Dobin et al., 2013). Variant identification was performed using the Genome Analysis Tool kit 3.6 (GATK), following the best practices guidelines for transcriptome variant analysis. Firstly, the genome index, read groups assignment and marking duplicates were performed using Picard tools 2.5 (https://broadinstitute.github.io/picard/index.html). The GATK was used for CIGAR string determination (SplitNCigarReads), reassigning mapping qualities, base recalibration, variants calling and filtering. The following filters were used to select variants: FS > 30.0, MQRankSum < −12.5, SNPcluster considering 3 variants in a 35bp window, QD < 5.0, MQ < 50.0, GQ < 5.0, QUAL ≥ 30.0, ReadPosRankSum < −8.0 and DP ≥ 300.0. Once the polymorphisms were identified by GATK, they were submitted to quality control analysis in plink 1.9 (Purcell et al., 2007; Steiß et al., 2012), where SNPs with genotyping rate <0.2 and minor allele frequencies (MAF) < 0.05 were removed from further analysis. Then, a case-control association analysis using permutation was performed to verify the presence of variants related to FHS phenotype. p-values ≤ 0.0001 were considered significant. Variant annotation was performed in Variant Effect Predictor (VEP) tool (McLaren et al., 2016) from Ensembl using the G. gallus genome (GRCg6a, Ensembl 105), considering the distance of 1,000 bp for upstream/downstream transcript assignment.
Results
Sequencing and mapping
RNA-Seq data from femoral head of all 8 samples generated approximately 22 million paired-end reads (2 × 100 pb) per sample. After the quality control, about 20.3 million of paired-end reads (Supplementary Table S1) were kept for further analysis. An average of 95.81 ± 0.27% (about 19.5 million) of the reads were mapped in the reference chicken genome (GRCg6a, Ensembl 105), where 77.58% of the reads were mapped in features.
Differential expression, annotation, and pathway analysis
The clustering of samples using the DE genes highlight the differences between FHS and normal groups (Figure 1A). 13,487 genes (Supplementary Table S3) were expressed in the 21 days chicken femoral head transcriptome, corresponding to almost 55.40% of the total genes described in the G. gallus assembly GRCg6a. Out of those, 34 were differentially expressed (DE; Table 2) between the normal and FHS-affected broilers analyzed in this study. All of them were downregulated in the affected (Figure 1B) compared to the normal group.
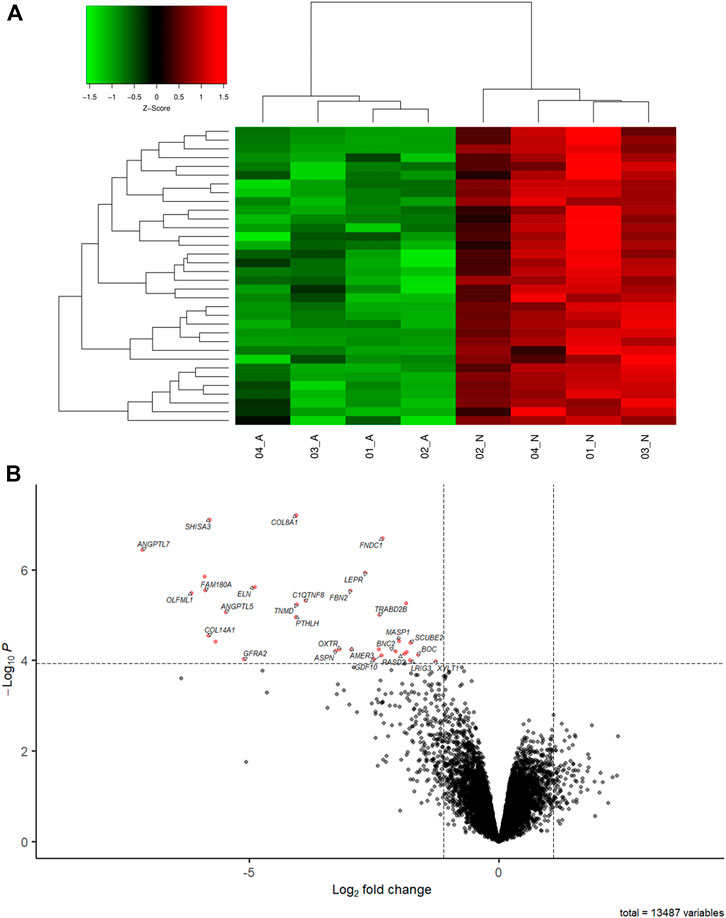
FIGURE 1. (A) Heatmap showing a hierarchical clustering of genes and samples of the DE genes between FHS-affected and control. The genes are presented in the rows and samples in the columns. Downregulated genes in the affected group are shown in red and upregulated in green. (B) Volcano plot showing the differentially expressed genes (red dot) in FHS-affected broilers.
Considering the DE genes, it was possible to observe 33 coding genes, being 29 known, 4 novel and 1 lncRNAs (ENSGALG00000045154). The sequences of 4 uncharacterized genes had similarities with fibronectin type III (ENSGALG00000015307, ENSGALG00000049529), immunoglobulin-like domain (ENSGALG00000054344), and concanavalin A-like lectin domain superfamily (ENSGALG00000054999).
In the DAVID ontology analysis, 20 genes (ANGPTL7, ASPN, GDF10, TNMD, LRIG3, LEPR, FBN2, BNC2, BOC, COL8A1, PTHLH, TRABD2B, SCUBE2, GFRA2, AMER3, and SHISA3) were attributed to biological processes, where most of them were related to receptor signaling pathways, ossification, bone mineralization and formation, and vascularization (Figure 2; Supplementary Table S4). Using the ShinyGO tool, ANGPTL7, XYLT1, MASP1, FBN2, PTHLH, SCUBE2, and ELN genes were in the extracellular region BP. The molecular functions were mostly related to calcium ion binding and matrix constituent (Figure 2, Supplementary Table S4). Furthermore, these genes were characterized in fibronectin, collagen, immunoglobulin, and epidermal growth factor protein domains (Figure 2).
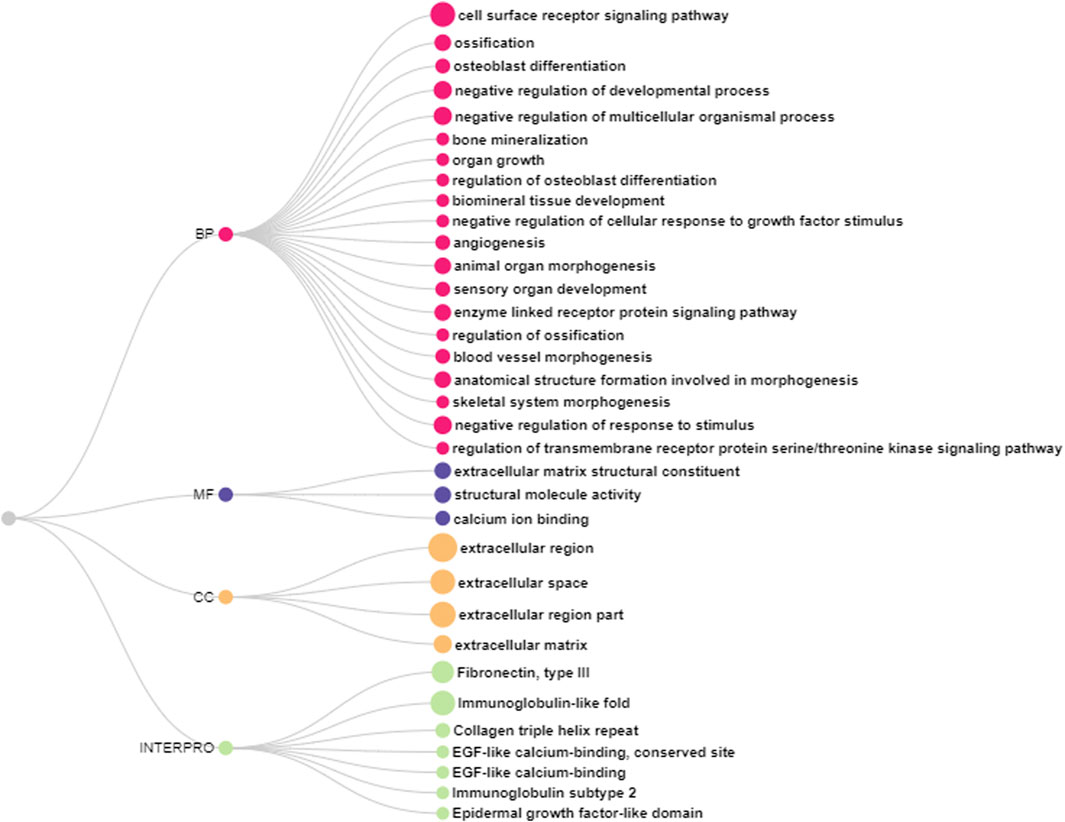
FIGURE 2. Biological processes (BP), molecular functions (MF), cell component (CC) and protein domains according to the Interpro enriched using DAVID database.
The gene network using G. gallus database was performed to verify the interactions among the DE genes, where 11 genes were grouped in two main branches (Figure 3A). Considering the gene network with the protein interactors, also 2 main branches were found (Figure 3B). Furthermore, 16 out of 34 DE genes were included in the gene network, being possible to observe that these genes are functionally associated, contributing to FHS phenotype.
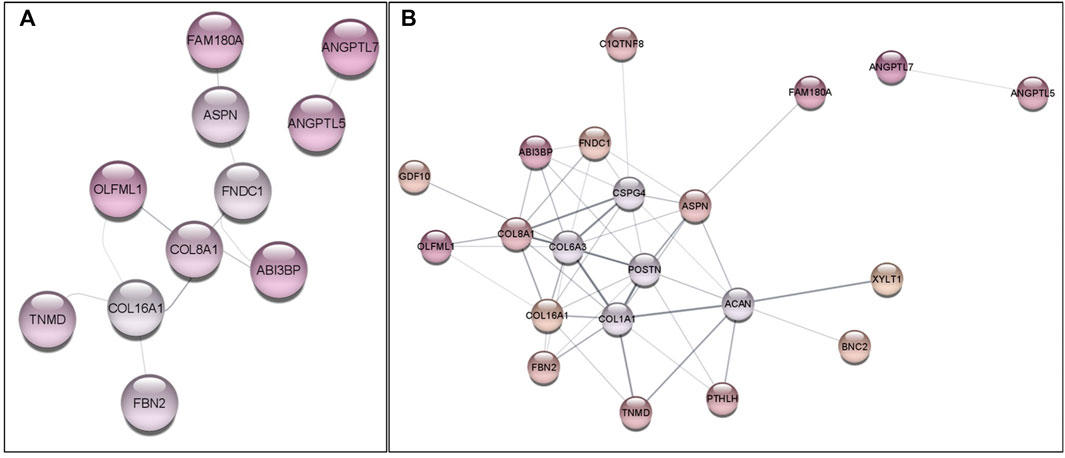
FIGURE 3. Gene network constructed with DE genes (A) and with DE genes plus up to 5 protein interactors (B) with the Gallus gallus information. Colored circles represent genes and connecting lines represent interactions among genes, according to Cytoscape Stringdb App. Colors indicate the downregulation expression levels, where darker colors have lower logFC levels in FHS-affected group. Gray circles (B) are protein interactors added to improve the gene network information.
Considering the transcriptome datasets from 21, 35, and 42 days of age, it was possible to observe that one gene was DE at all ages in the articular cartilage and growth plate (FBN2), while three genes (FBN2, C1QTNF8 and XYLT1) where DE in GP at 21, 35, and 42 days of age (Figure 4). A total of 12 genes were exclusively DE at 21 days, where 10 have already been characterized (SHISA3, FNDC1, ANGPTL7, LEPR, ENSGALG00000049529, OXTR, ENSGALG00000045154, COL16A1, RASD2, BOC, GDF10, and THSD7B).
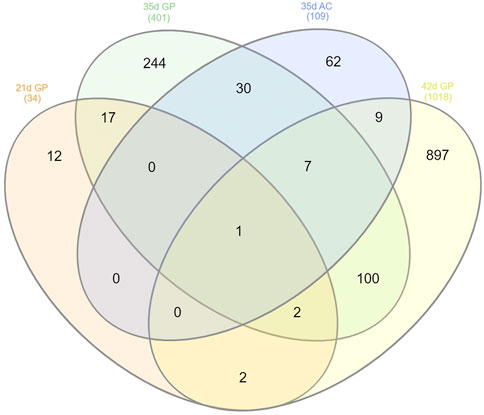
FIGURE 4. Venn diagram of DE genes in the femur GP of 21 and 35 days of age, tibia GP of 42 days of age and AC of 35 days of age.
The genes evaluated in the qPCR analysis had the same expression profile when compared to the RNA-Seq results and were also DE between groups. The COL14A1, FAM180A, ANGPTL5, FBN2, and LEPR candidate genes were downregulated in the FHS-affected when compared to normal broilers, confirming the RNA-Seq analysis results (Table 3). The TNMD gene had a low expression, showing amplification (Ct mean = 36.7) in all normal samples, but it was detected in only one sample from the FHS-affected group (Ct mean = 39.2), therefore, it was not possible to perform the statistical analysis for this gene.

TABLE 3. Relative gene expression and LogFC between FHS-affected.and control groups and results of differential expression analysis using REST.
Variant identification
A total of 223,069 variants were identified in the 24 analyzed samples after GATK filtering. For association analysis, 8,316 and 45,615 variants were removed due to genotyping rate and MAF failures, respectively. A total of 168,672 SNPs were kept for association analysis, where 12 SNPs were associated with FHS (p < 0.0001). From those, 5 were novel and 7 were existing variants being located in 7 genes (RARS, TFPI2, TTI1, MAP4K3, LINK5,4 and AREL1) (Table 4, Supplementary Table S5). The SNP located in the TTI1 gene was a missense variant, while those in RARS, TFPI2, LINK54, and AREL1 were synonymous. One SNP in the MAP4K3 gene was located in an intron and three (4:46318416, 4:82213680 and 4:82216997) were in intergenic regions (Supplementary Tables S5, S6).
Unmapped reads identification
An average of 605,218 RNA reads/sample were unmapped in the chicken genome (Supplementary Table S2) and were submitted to a metagenome taxonomic classification analysis to check if they matched with microorganisms sequences. About 23,608 (∼3.8%) reads/sample were identified in 23 different families (Supplementary Table S7) where the most abundant were Enterobacteriaceae, Comamonadaceae and Bacillaceae, (Supplementary Table S7). Considering genus, 83 were identified and the most present were Streptomyces, Pseudomonas, Proteus, Halomonas, Rhizobium, Stenotrophomonas, Shapirovirus, Corynebacterium, Acinetobacter, and Aeromonas (Supplementary Figure S1). Although several groups were found in the analyzed samples, no differences were observed between normal and FHS-affected groups.
Discussion
The complexity of the FHS/FHN etiology and pathogenesis makes this condition one of the main problems affecting fast-growing broilers production (Prisby et al., 2014). FHS is characterized by the detachment of GP and AC, occurring in tibia and femur (Durairaj et al., 2009; Packialakshmi et al., 2015). Although some studies have been published in the last years with FHS, FHN, and BCO, including by our group, there are few of them approaching the molecular mechanisms involved with these conditions, especially with early ages (Li et al., 2015; Paludo et al., 2017; Peixoto et al., 2019; Oliveira et al., 2020; Liu K. et al., 2021; Hul et al., 2021; Ramser et al., 2021, 2022; Goldoni et al., 2022; Santos et al., 2022). To the best of our knowledge, this paper is the first attempt to compare normal and FHS-affected femoral head transcriptome in broilers with 21 days of age. The results presented here can contribute to deepen the understanding of FHS in chicken, clarifying BP, genes and variants involved with this condition, as well as to prospect the presence of microorganisms in the analyzed samples. Here, most of the enriched biological processes were related to ossification, developmental processes, response to stimulus, extracellular matrix (ECM), angiogenesis, skeletal morphogenesis, within others. In these processes, collagen, angiopoietin-like, growth factors, fibronectins and tumor necrosis related genes were DE (Table 1). The skeletogenesis is initiated when mesenchymal precursor cells differentiate in cartilage and bone cells that grow by the endochondral ossification process (Goldring et al., 2006; Ağirdil, 2020). After hatch, mesenchymal cells in the GP initiate a proliferation to hypertrophic chondrocytes leading to cartilage formation, where chondrocytes will suffer chondrolysis and apoptosis, allowing vascular invasion and bone formation (Rath and Durairaj, 2022). The coordinated gene expression related to cell proliferation and differentiation, angiogenesis, apoptosis, local growth factors, hormones and cell signaling are needed (Goldring et al., 2006). In our study, 34 genes were DE between normal and FHS-affected 21 days old broilers, being downregulated in the affected group (Figure 1B; Table 2). Most of these genes were in BP related to ossification, chondrogenesis and angiogenesis (Figure 2), and the DE profile found in our study could help to understand the FHS pathogenesis. FHS has been characterized by focal death, atrophic changes in the cartilage (Packialakshmi et al., 2015), reduced chondrocyte density, presence of pyknotic nuclei and erythrocytes and the presence of inflammatory cells (Wilson et al., 2019). Here, several genes DE, such as collagens, FBN2, ANGPTLs, FNDC1, C1QTNF8, and XYLT1 may corroborate with the hypothesis suggested by those authors.
GP zones have high metabolic activity, where chondrocytes pass from resting, proliferative, hypertrophic and terminal phases, leading to a healthy osteogenesis (Ağirdil, 2020). In the first phases, it is known that collagens such as COL2A1 and COL10A1 are essential to chondrocyte maturation (Ağirdil, 2020). The major constituent of the organic bone matrix and articular cartilage is the collagen protein, where the COL2A1 is the main studied collagen (Hallett et al., 2019). This protein interacts with other collagens and proteins to create a network to form the ECM (Graham et al., 2000; Flowers et al., 2017), being highly expressed in the proliferation phase, while type X collagens are more expressed in the hypertrophic phases than in the other ones. In our study, three collagen genes, COL14A1, COL8A1, and COL16A1, were downregulated in the affected broilers, being, respectively, 7, 4, and 6.5 times less expressed in the FHS-affected than in the normal broilers (Table 1).
Furthermore, other collagen related genes, such as FBN2 and COL16A1 were also downregulated in the affected group. It is interesting to note that most of the genes in the network were grouped in one branch, where collagen genes were connectors (Figure 3). Although the endochondral ossification has been widely studied (Long and Ornitz, 2013), information on the genes involved in the endochondral ossification and FHS in chickens are still scarce. COL14A1 has already been associated to calcium ligation and cell morphogenesis (Rojas-Peña et al., 2014), being highly expressed when submitted to mechanical stress (Manon-Jensen and Karsdal, 2016). COL16A1 was associated to osteoarthritis in humans (Karlsson et al., 2010; Chou et al., 2013), while polymorphisms in COL8A gene were associated to the loss of function during embryogenesis leading to congenital vertebral malformations (Gray et al., 2014). The importance of COL2A1 in the ECM, cartilage and bone replacement formation has been highlighted in several studies. Polymorphisms in this gene were found to increase the susceptibility to FHN in humans (Li et al., 2014), and its expression is essential to the fibrils formation on epiphyseal growth plate (Pouya and Kerachian, 2015). The downregulation of these collagen genes may influence the extracellular matrix formation, affecting the integrity of the collagen network (Heuijerjans et al., 2017), as well as the chondrocyte maturation, and, possibly, preventing the bone formation and, consequently, facilitating the FHS appearance in chickens.
Lack of angiogenesis and vascularization have also been associated to FHS in chickens (Durairaj et al., 2009; Prisby et al., 2014; Li et al., 2015; Packialakshmi et al., 2015; Paludo et al., 2017; Peixoto et al., 2019; Goldoni et al., 2022) and the DE genes ANGPTL7, TNMD, LEPR, and COL8A1 were in BP related to these functions. The angiopoietin-like family is composed by 8 genes (ANGPTL1 to ANGPTL8) encoding proteins structurally similar to angiopoietins, with functions related to glucose metabolism, hematopoiesis, fat metabolism and inflammation, participating in a multitude of physiological and pathophysiological processes. ANGPTLs differ from angiopoietins because they do not bind to their receptors (Santulli, 2014). In our study, 2 out of 8 known angiopoetin-like were DE between groups: ANGPTL7 and ANGPTL5. The role of ANGPTLs in vascularization still needs to be better understood, since they may act as pro-angiogenic, anti-angiogenic and also VEGF-independent (Hato et al., 2008). ANGPTL7 and ANGPTL5 have a role in hematopoietic stem cell expansion (Zhang et al., 2006). ANGPTL7 is believed to be a potent target of the WNT/β-catenin signaling pathway, which is essential to BMPs (bone morphogenetic proteins) activation in osteoblasts (Beederman et al., 2013; Osório, 2015). Moreover, the alteration of ANGPTL7 influences the expression of several matrix proteins, such as fibronectin, collagens, myocillins and metalloproteinases (Santulli, 2014), encoded by genes that were also downregulated in our study and were grouped in enriched BP of ECM. The TNMD function is not well characterized, but this gene encodes a chondromodulin-I related protein, which increases chondrocyte growth and inhibits angiogenesis (Shukunami et al., 2005; TNMD, 2022). Another downregulated gene in broilers with 21 days of age was LEPR, which is involved with lipid metabolism and inflammatory response (Abete et al., 2009; Gogiraju et al., 2019). Mutations in this gene has already been associated to femoral head osteonecrosis in humans (Liu T. et al., 2021) and with bone integrity traits in broilers (Ibelli et al., 2014). Although there is no information on its role associated with FHS in chickens, in mice, LEPR was considered a negative modulator of bone mechanosensitivity, leading to a poor osteogenic response (Kapur et al., 2010).
The correct transportation of transmembrane adhesion molecules is important to maintain the articular cartilage and growth plate communication and, consequently, bone remodeling (Packialakshmi et al., 2015). In the current study, low expression levels of genes associated with ECM could favor the FHS condition. Among the genes DE in BP related to extracellular matrix were ASPN, FBN2, COL8A1, COL14A1, COL16A1, and ELN. The ELN was downregulated in FHS-affected broilers compared to normal ones in this study and in broilers with 35 days of age (Peixoto et al., 2019). This gene is associated with the production of a protein called tropoelastine, which is responsible for the elasticity of connective tissue found in cartilage, and acts as a precursor of osteoblast differentiation (Twine et al., 2014). Reduced ELN expression was associated to the risk of injury in the rat tendon (Kostrominova and Brooks, 2013).The ASPN gene encodes a small leucine-rich cartilage extracellular protein of proteoglycan family, regulating chondrogenesis and binding calcium to collagens (Mishra et al., 2019). ASPN is considered a biological marker for osteoarthritis development in humans and mice (Karlsson et al., 2010; Mishra et al., 2019). This gene also acts in osteoblast biomineralization activity, and its expression was increased in ECM (Zhu et al., 2012).
FHS occurrence is also affected by broilers age, increasing at older ages (Prisby et al., 2014). Our research group has been evaluating the FHS/FHN gene expression in several ages in femur (Paludo et al., 2017; Hul et al., 2021; Goldoni et al., 2022; Santos et al., 2022) and in tibia (Oliveira et al., 2020). In this way, trying to understand the genes more related with FHS onset, we have compared the common DE genes and also those exclusive to 21, 35, and 42 days of age in GP and AC. The FBN2 was the only DE gene in all studied datasets: 21 and 35 days femur GP, 42 days tibia GP and 35 days AC (Figure 4; Supplementary Table S8). This gene was annotated in 23 of the 34 ontologies enriched in this study (Figure 2; Supplementary Table S4), including ossification, regulation of TGF, extracellular matrix and calcium binding. FBN2 gene is one of the major components of ECM with a primary function of maintaining tissue structural integrity, which can affect several tissues, including skeletal system (Yu and Urban, 2013; Lee et al., 2019). In FBN2 knockout mice, a reduced osteoblast maturation was observed, preventing bone formation, and increasing bone resorption (Nistala et al., 2010), showing that this gene has an important role in stimulate osteoblast differentiation (Lee et al., 2019). In dogs, mutations in FBN2 gene were associated with hip dysplasia (Friedenberg et al., 2011). In the analyzed datasets, the FBN2 downregulation in the FHS-affected group could be preventing the optimal ossification of the GP and, since it occurred at 21, 35, and 42 days of age, this reinforces FBN2 gene as candidate for FHS/FHN.
We also observed that three genes (FBN2, XYLT1, and C1QTNF8) were downregulated in GP of FHS-group of all analyzed ages. XYLT1 encodes the xylosyltransferase enzyme, necessary for glycosaminoglycan (GAG) biosynthesis, and it is considered a key gene for chondrocyte maturation and skeletal length (Mis et al., 2014). In mice, XYLT anomalous expression, as well as mutations in this gene alters the GAG normal levels, affecting proteoglycan production and bone growth, leading to a pug dwarfism phenotype (Mis et al., 2014). Furthermore, it was demonstrated in zebrafish that bone formation around cartilage is space and time regulated, in which proteoglycans were responsible for this regulation, being crucial for skeletal development (Eames et al., 2011). There is a lack of information on the C1QTNF8 (complement C1q Tumor Necrosis Factor-Related Protein 8) gene function in chickens. C1QTNF8 is predicted to be part of a collagen trimmering and, in humans, it is considered a paralog of C1QTNF6. A DNA methylation analysis found that the C1QTNF8 was differentially methylated in osteoarthritis (OA) subchondral bone (Jeffries et al., 2016), while its paralogous is a marker for OA in mouse, acting in host defense, inflammation and glucose metabolism (Murayama et al., 2014, 2015). Moreover, when comparing DE genes in GP from different ages, we observed that through aging, more shared DE genes were found between 21 and 35 days and between 35 and 42 days of age, than when 21 and 42 days were compared (Figure 4). When looking at the 12 exclusively DE genes between normal and FHS-affected broilers at 21 days of age (SHISA3, FNDC1, ANGPTL7, LEPR, OXTR, COL16A1, BOC, ENSGALG00000049529, RASD2, GDF10 ENSGALG00000045154, and THSD7B), they were mainly related to WNT and FGF signaling (Amanatullah et al., 2014), bone metabolism (Nilsson et al., 2007; Di Benedetto et al., 2014; Lui et al., 2018), angiogenesis (Shang et al., 2015; Huang et al., 2021), chondrogenesis (Sekiya et al., 2002; Paradise et al., 2018) and Hedgehog signaling pathway (Kavran et al., 2010; Xavier et al., 2016). In this way, it is possible to highlight that DE genes between normal and affected broilers at 21 days of age were mainly related to endochondral ossification, while when GP and AC at 35 and 42 days of age were evaluated (Peixoto et al., 2019; Oliveira et al., 2020; Hul et al., 2021; Goldoni et al., 2022), there were several DE genes related to other biological processes, such as inflammation, defense response, chemotaxis, within others. Therefore, the expression profiles found at 21 days of age are in agreement with other studies that found that one of the main issues regarding FHS/FHN is related to the femur maturation and mineralization (Prisby et al., 2014; Wilson et al., 2019), possibly due to fast-growth and lack of increasing bone volume in the same rate.
Variants in all datasets analyzed here were identified in the RNA-Seq and an odds ratio analysis was performed. Twelve SNPs in seven genes (TFPI2, MAP4K3, LIN54, GRK4, AREL1, RARS, and TTI1) were associated with FHS predisposition in chickens (p < 0.0001). A new SNP annotated as missense in the TELO2 Interacting Protein (TTI1) gene had an odds ratio of 12.36. The TTI1 is an mTOR signaling that regulates cell growth and survival in response to nutrient and hormonal changes and activates the eukaryotic translation initiation factor 4E binding protein 1 (eIF4E) (Ronkina and Gaestel, 2022) from 4E-BP1, which is an inhibitor of cap-dependent translation (Katsara and Kolupaeva, 2018). The 4E-BP1 inhibition has already been associated to cartilage degeneration in rat osteoarthritic knees (Katsara and Kolupaeva, 2018). The other variants were intronic or synonymous, but it is interesting to note that, as the missense, they were found in genes mainly related to basal machinery of cells, such as RARS1, LIN54, AREL1, and TFPI2. RARS1 is an Arginyl-TRNA Synthetase 1, LIN54 is a key regulator of cell cycle genes, in which its depletion leads to growth defects (Schmit et al., 2009), the AREL1 is a negative regulator of apoptosis, while TFPI2 is related to vascular endothelial growth factor (Xu et al., 2006). Although with a small number of samples, the RNA-Seq data allowed us to identify putative functional variants that could contribute with the appearance of the analyzed condition. The SNPs discovered here should be validated in a large population to confirm them as genetic markers.
According to our results, several biological processes and genes involved with femur head separation in chickens were identified at an early age. Here, genes of the collagen and angiopoietin-like family, among others, were associated with this condition in G. gallus at 21 days of age. It was possible to show that several genes, such as FBN2, XYLT1, and C1QTNF8 have an important role in the organic matrix bone formation, hypoxia, as well as its homeostasis. This indicates that changes associated with broiler rapid growth may affect genes related to osteogenesis, especially those involved with endochondral ossification, which might contribute to the onset of femoral head necrosis. Although it is difficult to state whether these genes are causing FHS or not, the approach used in this study allows comprehending how the early molecular changes are happening. Nevertheless, further studies are needed to clarify the expression pattern of these genes over time, to elucidate whether the alteration in expression occurs since the birth of the chicks, or if it is due to their rapid growth. Moreover, SNPs in candidate genes were prospected in the femoral GP of 21-day-old broilers, which can evince new approaches to reduce this condition in chickens. Understanding the genetic factors associated with bone formation and maintenance may lead to a better comprehension on how environmental and management factors can affect the necrosis process.
Conclusion
In this study, using RNA-seq analysis, we have shown that a set of genes related to chondrogenesis and bone differentiation were downregulated in the GP of FHS-affected young broilers. Among these genes, FBN2, XYLT1, and C1QTNF8 can be highlighted since they were DE in the GP of various ages. SNPs were also identified in genes related to translation factors and cell growth, which could predispose the animals to FHS/FHN development. Furthermore, at 21 days of age, we also notice that the DE genes were more related to cartilage and bone morphogenesis, while in other ages (35 and 42 days), genes related to defense response, inflammation and chemotaxis were also found. Therefore, these findings evince that candidate genes pointed out in our study are probably related to the FHS progression in broilers.
Data availability statement
The datasets presented in this study can be found in online repositories. The names of the repository/repositories and accession number(s) can be found below: https://www.ncbi.nlm.nih.gov/, SRA Bioproject PRJNA288640.
Ethics statement
The animal study was reviewed and approved by the Ethics Committee on Animal Utilization of the Embrapa Swine and Poultry National Research Center.
Author contributions
JP, ML, RZ, and AI conceived and designed the experiment. JP, RZ, and ML were responsible for the data collection. AI, JM, MP, LC, and DM performed the laboratory experiment. AI, JG, and MC performed the data analysis. AI, JP, JG, MC, and ML interpreted the results and wrote the manuscript. All authors reviewed, edited and approved the final manuscript. JP, and ML were responsible for funding acquisition and supervision of the research.
Funding
This study was financed by the National Council for Scientific and Technological Development (CNPq), project numbers 400606/2012-7 and 407404/2013-9.
Acknowledgments
The authors are grateful to A. L. Tessmann for technical assistance. RZ was supported by a BJT grant n° 373167/2012-1 from the National Council for Scientific and Technological Development (CNPq). DEPM received a PIBIC/CNPq scholarship at Embrapa and MSDP received a FAPESC/CAPES scholarship at UDESC/Oeste. LLC, MCL, AMGI, and RZ are recipients of a productivity fellowship from CNPq. We thank the Coordenação de Aperfeiçoamento de Pessoal de Nível Superior (CAPES), Brazil—Finance Code 001 for the free access to the journals used in the literature review.
Conflict of interest
The authors declare that the research was conducted in the absence of any commercial or financial relationships that could be construed as a potential conflict of interest.
Publisher’s note
All claims expressed in this article are solely those of the authors and do not necessarily represent those of their affiliated organizations, or those of the publisher, the editors and the reviewers. Any product that may be evaluated in this article, or claim that may be made by its manufacturer, is not guaranteed or endorsed by the publisher.
Supplementary material
The Supplementary Material for this article can be found online at: https://www.frontiersin.org/articles/10.3389/fphys.2022.941134/full#supplementary-material
References
Abete I., Goyenechea E., Crujeiras A. B., Martínez J. A. (2009). Inflammatory state and stress condition in weight-lowering Lys109Arg LEPR gene polymorphism carriers. Arch. Med. Res. 40, 306–310. doi:10.1016/J.ARCMED.2009.03.005
Ağirdil Y. (2020). The growth plate: A physiologic overview. EFORT Open Rev. 5, 498–507. doi:10.1302/2058-5241.5.190088
Al-rubaye A. A. K., Ekesi N. S., Zaki S., Emami N. K., Jr R. F. W., Rhoads D. D., et al. (2017). Chondronecrosis with osteomyelitis in broilers : Further defining a bacterial challenge model using the wire flooring model. Poult. Sci. 96, 332–340. doi:10.3382/ps/pew299
Amanatullah D. F., Yamane S., Reddi A. H. (2014). Distinct patterns of gene expression in the superficial, middle and deep zones of bovine articular cartilage. J. Tissue Eng. Regen. Med. 8, 505–514. doi:10.1002/TERM.1543
Anders S., Pyl P. T., Huber W. (2015). HTSeq--a Python framework to work with high-throughput sequencing data. Bioinformatics 31, 166–169. doi:10.1093/bioinformatics/btu638
Beederman M., Lamplot J. D., Nan G., Wang J., Liu X., Yin L., et al. (2013). BMP signaling in mesenchymal stem cell differentiation and bone formation. J. Biomed. Sci. Eng. 6, 32–52. doi:10.4236/JBISE.2013.68A1004
Benjamini Y., Hochberg Y. (1995). Controlling the false discovery rate: A practical and powerful approach to multiple testing. J. R. Stat. Soc. Ser. B 57, 289–300. doi:10.1111/J.2517-6161.1995.TB02031.X
Bolger A. M., Lohse M., Usadel B. (2014). Trimmomatic: A flexible trimmer for Illumina sequence data. Bioinformatics 30, 2114–2120. doi:10.1093/bioinformatics/btu170
Chou C. H., Lee C. H., Lu L. S., Song I. W., Chuang H. P., Kuo S. Y., et al. (2013). Direct assessment of articular cartilage and underlying subchondral bone reveals a progressive gene expression change in human osteoarthritic knees. Osteoarthr. Cartil. 21, 450–461. doi:10.1016/J.JOCA.2012.11.016
Di Benedetto A., Sun L., Zambonin C. G., Tamma R., Nico B., Calvano C. D., et al. (2014). Osteoblast regulation via ligand-activated nuclear trafficking of the oxytocin receptor. Proc. Natl. Acad. Sci. U. S. A. 111, 16502–16507. doi:10.1073/PNAS.1419349111
Dobin A., Davis C. A., Schlesinger F., Drenkow J., Zaleski C., Jha S., et al. (2013). Star: Ultrafast universal RNA-seq aligner. Bioinformatics 29, 15–21. doi:10.1093/bioinformatics/bts635
Durairaj V., Okimoto R., Rasaputra K., Clark F. D., Rath N. C. (2009). Histopathology and serum clinical chemistry evaluation of broilers with femoral head separation disorder. Avian Dis. 53, 21–25. doi:10.1637/8367-051908-Reg.1
Eames B. F., Yan Y. L., Swartz M. E., Levic D. S., Knapik E. W., Postlethwait J. H., et al. (2011). Mutations in fam20b and xylt1 reveal that cartilage matrix controls timing of endochondral ossification by inhibiting chondrocyte maturation. PLoS Genet. 7, 1002246. doi:10.1371/JOURNAL.PGEN.1002246
Federici J. F., Vanderhasselt R., Sans E. C. O., Tuyttens F. A. M., Souza A. P. O., Molento C. F. M., et al. (2016). Assessment of broiler chicken welfare in southern Brazil. Rev. Bras. Cienc. Avic. 18, 133–140. doi:10.1590/18069061-2015-0022
Flowers S. A., Zieba A., Örnros J., Jin C., Rolfson O., Björkman L. I., et al. (2017). Lubricin binds cartilage proteins, cartilage oligomeric matrix protein, fibronectin and collagen II at the cartilage surface. Sci. Rep. 71 (7), 13149. doi:10.1038/s41598-017-13558-y
Friedenberg S. G., Zhu L., Zhang Z., van den Foels W. B., Schweitzer P. A., Wang W., et al. (2011). Evaluation of a fibrillin 2 gene haplotype associated with hip dysplasia and incipient osteoarthritis in dogs. Am. J. Vet. Res. 72, 530–540. doi:10.2460/AJVR.72.4.530
Ge S. X., Jung D., Jung D., Yao R. (2020). ShinyGO: A graphical gene-set enrichment tool for animals and plants. Bioinformatics 36, 2628–2629. doi:10.1093/BIOINFORMATICS/BTZ931
Gogiraju R., Hubert A., Fahrer J., Straub B. K., Brandt M., Wenzel P., et al. (2019). Endothelial leptin receptor deletion promotes cardiac autophagy and angiogenesis following pressure overload by suppressing akt/mTOR signaling. Circ. Heart Fail. 12, e005622. doi:10.1161/CIRCHEARTFAILURE.118.005622
Goldoni I., Ibelli A. M. G., Fernandes L. T., Peixoto J., Hul L. M., Cantão M. E., et al. (2022). Comprehensive analyses of bone and cartilage transcriptomes evince ion transport, inflammation and cartilage development-related genes involved in chickens’ femoral head separation. Anim 12, 788. doi:10.3390/ANI12060788
Goldring M. B., Tsuchimochi K., Ijiri K. (2006). The control of chondrogenesis. J. Cell. Biochem. 97, 33–44. doi:10.1002/JCB.20652
Graham H. K., Holmes D. F., Watson R. B., Kadler K. E. (2000). Identification of collagen fibril fusion during vertebrate tendon morphogenesis. The process relies on unipolar fibrils and is regulated by collagen-proteoglycan interaction. J. Mol. Biol. 295, 891–902. doi:10.1006/JMBI.1999.3384
Gray R. S., Wilm T. P., Smith J., Bagnat M., Dale R. M., Topczewski J., et al. (2014). Loss of col8a1a function during zebrafish embryogenesis results in congenital vertebral malformations. Dev. Biol. 386, 72–85. doi:10.1016/J.YDBIO.2013.11.028
Hallett S. A., Ono W., Ono N. (2019). growth plate chondrocytes: Skeletal development, growth and beyond. Int. J. Mol. Sci. 20, 6009. doi:10.3390/IJMS20236009
Hato T., Tabata M., Oike Y. (2008). The role of angiopoietin-like proteins in angiogenesis and metabolism. Trends cardiovasc. Med. 18, 6–14. doi:10.1016/J.TCM.2007.10.003
Heuijerjans A., Wilson W., Ito K., van Donkelaar C. C. (2017). The critical size of focal articular cartilage defects is associated with strains in the collagen fibers. Clin. Biomech. 50, 40–46. doi:10.1016/J.CLINBIOMECH.2017.09.015
Huang H., Zhang J., Ling F., Huang Y., Yang M., Zhang Y., et al. (2021). Leptin Receptor (LEPR) promotes proliferation, migration, and invasion and inhibits apoptosis in hepatocellular carcinoma by regulating ANXA7. Cancer Cell Int. 21, 4. doi:10.1186/s12935-020-01641-w
Hul L. M., Ibelli A. M. G., Savoldi I. R., Marcelino D. E. P., Fernandes L. T., Peixoto J. O., et al. (2021). Differentially expressed genes in the femur cartilage transcriptome clarify the understanding of femoral head separation in chickens. Sci. Rep. 11, 17965. doi:10.1038/s41598-021-97306-3
Ibelli A. M. G., Peixoto J. O., Marchesi J. A. P., Coutinho L. L., Ledur M. C. (2014). New insights on the influence of leptin receptor gene in bone traits in broilers. Proc. World Congr. Genet. Appl. Livest. Prod. 2014, 328.
Jeffries M. A., Donica M., Baker L. W., Stevenson M. E., Annan A. C., Beth Humphrey M., et al. (2016). Genome-Wide DNA methylation study identifies significant epigenomic changes in osteoarthritic subchondral bone and similarity to overlying cartilage. Arthritis Rheumatol. 68, 1403–1414. doi:10.1002/art.39555
Jiang T., Mandal R. K., Wideman R. F., Khatiwara A., Pevzner I., Min Kwon Y., et al. (2015). Molecular survey of bacterial communities associated with bacterial chondronecrosis with osteomyelitis (BCO) in broilers. PLoS One 10, e0124403. doi:10.1371/journal.pone.0124403
Kapur S., Amoui M., Kesavan C., Wang X., Mohan S., Baylink D. J., et al. (2010). Leptin receptor (lepr) is a negative modulator of bone mechanosensitivity and genetic variations in lepr may contribute to the differential osteogenic response to mechanical stimulation in the C57BL/6J and C3H/HeJ pair of mouse strains. J. Biol. Chem. 285, 37607–37618. doi:10.1074/JBC.M110.169714
Karlsson C., Dehne T., Lindahl A., Brittberg M., Pruss A., Sittinger M., et al. (2010). Genome-wide expression profiling reveals new candidate genes associated with osteoarthritis. Osteoarthr. Cartil. 18, 581–592. doi:10.1016/J.JOCA.2009.12.002
Katsara O., Kolupaeva V. (2018). mTOR-mediated inactivation of 4E-BP1, an inhibitor of translation, precedes cartilage degeneration in rat osteoarthritic knees. J. Orthop. Res. 36, 2728–2735. doi:10.1002/JOR.24049
Kavran J. M., Ward M. D., Oladosu O. O., Mulepati S., Leahy D. J. (2010). All mammalian hedgehog proteins interact with cell adhesion molecule, down-regulated by oncogenes (CDO) and brother of CDO (BOC) in a conserved manner. J. Biol. Chem. 285, 24584–24590. doi:10.1074/jbc.M110.131680
Knowles T. G., Kestin S. C., Haslam S. M., Brown S. N., Green L. E., Butterworth A., et al. (2008). Leg disorders in broiler chickens: Prevalence, risk factors and prevention. PLoS One 3, e1545. doi:10.1371/journal.pone.0001545
Kostrominova T. Y., Brooks S. V. (2013). Age-related changes in structure and extracellular matrix protein expression levels in rat tendons. Age 35, 2203–2214. doi:10.1007/S11357-013-9514-2
Lee Y. J., Park S. Y., Park E. K., Kim J. E. (2019). Unique cartilage matrix-associated protein regulates fibrillin-2 expression and directly interacts with fibrillin-2 protein independent of calcium binding. Biochem. Biophys. Res. Commun. 511, 221–227. doi:10.1016/J.BBRC.2019.01.128
Li N., Yu J., Cao X., Wu Q. Y., Li W. W., Li T. F., et al. (2014). A novel p. Gly630Ser mutation of COL2A1 in a Chinese family with presentations of legg–calvé–perthes disease or avascular necrosis of the femoral head. PLoS One 9, e100505. doi:10.1371/JOURNAL.PONE.0100505
Li P. F., Zhou Z. L., Shi C. Y., Hou J. F. (2015). Downregulation of basic fibroblast growth factor is associated with femoral head necrosis in broilers. Poult. Sci. 94, 1052–1059. doi:10.3382/ps/pev071
Liberzon A., Subramanian A., Pinchback R., Thorvaldsdóttir H., Tamayo P., Mesirov J. P., et al. (2011). Molecular signatures database (MSigDB) 3.0. Bioinformatics 27, 1739–1740. doi:10.1093/BIOINFORMATICS/BTR260
Liu K., Wang K., Wang L., Zhou Z. (2021a). Changes of lipid and bone metabolism in broilers with spontaneous femoral head necrosis. Poult. Sci. 100, 100808. doi:10.1016/J.PSJ.2020.10.062
Liu T., Cao Y., Han C., An F., Wang T., Sun M., et al. (2021b). Association of MIR17HG and MIR155HG gene variants with steroid-induced osteonecrosis of the femoral head in the population of northern China. J. Orthop. Surg. Res. 16, 673. doi:10.1186/s13018-021-02669-y
Long F., Ornitz D. M. (2013). Development of the endochondral skeleton. Cold Spring Harb. Perspect. Biol. 5, a008334. doi:10.1101/CSHPERSPECT.A008334
Lui J. C., Jee Y. H., Garrison P., Iben J. R., Yue S., Ad M., et al. (2018). Differential aging of growth plate cartilage underlies differences in bone length and thus helps determine skeletal proportions. PLoS Biol. 16, e2005263. doi:10.1371/JOURNAL.PBIO.2005263
Manon-Jensen T., Karsdal M. A. (2016). “Chapter 14-type XIV collagen,” in Biochemistry of Collagens, Laminins and Elastin (Cambridge, Massachusetts, EUA: Academic Press), 93–95.
McLaren W., Gil L., Hunt S. E., Riat H. S., Ritchie G. R. S., Thormann A., et al. (2016). The Ensembl variant Effect predictor. Genome Biol. 17, 122. doi:10.1186/s13059-016-0974-4
McMurdie P. J., Holmes S. (2013). phyloseq: An R package for reproducible interactive analysis and graphics of microbiome census data. PLoS One 8, e61217. doi:10.1371/JOURNAL.PONE.0061217
McNamee P. T., Smyth J. A. (2000). Bacterial chondronecrosis with osteomyelitis ('femoral head necrosis’) of broiler chickens: A review. Avian Pathol. 29, 253–270. doi:10.1080/03079450050118386
Mis E. K., Liem K. F., Kong Y., Schwartz N. B., Domowicz M., Weatherbee S. D., et al. (2014). Forward genetics defines Xylt1 as a key, conserved regulator of early chondrocyte maturation and skeletal length. Dev. Biol. 385, 67–82. doi:10.1016/J.YDBIO.2013.10.014
Mishra A., Awasthi S., Raj S., Mishra P., Srivastava R. N. (2019). Identifying the role of ASPN and COMP genes in knee osteoarthritis development. J. Orthop. Surg. Res. 14, 337. doi:10.1186/s13018-019-1391-7
Müller A., Hundt C., Hildebrandt A., Hankeln T., Schmidt B. (2017). MetaCache: Context-aware classification of metagenomic reads using minhashing. Bioinformatics 33, 3740–3748. doi:10.1093/BIOINFORMATICS/BTX520
Murayama M. A., Kakuta S., Maruhashi T., Shimizu K., Seno A., Kubo S., et al. (2014). CTRP3 plays an important role in the development of collagen-induced arthritis in mice. Biochem. Biophys. Res. Commun. 443, 42–48. doi:10.1016/J.BBRC.2013.11.040
Murayama M. A., Kakuta S., Inoue A., Umeda N., Yonezawa T., Maruhashi T., et al. (2015). CTRP6 is an endogenous complement regulator that can effectively treat induced arthritis. Nat. Commun. 61 (6), 8483. doi:10.1038/ncomms9483
Nilsson O., Parker E. A., Hegde A., Chau M., Barnes K. M., Baron J., et al. (2007). Gradients in bone morphogenetic protein-related gene expression across the growth plate. J. Endocrinol. 193, 75–84. doi:10.1677/JOE.1.07099
Nistala H., Lee-Arteaga S., Smaldone S., Siciliano G., Ramirez F. (2010). Extracellular microfibrils control osteoblast-supported osteoclastogenesis by restricting TGFβ stimulation of RANKL production. J. Biol. Chem. 285, 34126–34133. doi:10.1074/JBC.M110.125328
Oliveira H. C., Ibelli A. M. G., Guimarães S. E. F., Cantão M. E., Peixoto J. D. O., Coutinho L. L., et al. (2020). RNA-seq reveals downregulated osteochondral genes potentially related to tibia bacterial chondronecrosis with osteomyelitis in broilers. BMC Genet. 21, 58. doi:10.1186/s12863-020-00862-2
Osório J. (2015). Stem cells: Back to the origins--identifying the skeletal stem cell. Nat. Rev. Endocrinol. 113 (11), 132. doi:10.1038/nrendo.2015.14
Packialakshmi B., Rath N. C., Huff W. E., Huff G. R. (2015). Poultry femoral head separation and necrosis: A review. Avian Dis. 59, 349–354. doi:10.1637/11082-040715-Review.1
Pagès H., Carlson J., Facon S., Li L. (2022). AnnotationDbi: Manipulation of SQLite-based annotations in bioconductor. Available at: https://bioconductor.org/packages/release/bioc/html/AnnotationDbi.html (Accessed May 10, 2022).
Paludo E., Ibelli A. M. G., Peixoto J. O., Tavernari F. C., Lima-Rosa C. A. V., Pandolfi J. R. C., et al. (2017). The involvement of RUNX2 and SPARC genes in the bacterial chondronecrosis with osteomyelitis in broilers. Animal 11, 1063–1070. doi:10.1017/S1751731116002433
Paradise C. R., Galeano-Garces C., Galeano-Garces D., Dudakovic A., Milbrandt T. A., Saris D. B. F., et al. (2018). Molecular characterization of physis tissue by RNA sequencing. Gene 668, 87–96. doi:10.1016/J.GENE.2018.05.034
Paulson J. N., Colin Stine O., Bravo H. C., Pop M. (2013). Differential abundance analysis for microbial marker-gene surveys. Nat. Methods 2013, 1200–1202. doi:10.1038/nmeth.2658
Paxton H., Tickle P. G., Rankin J. W., Codd J. R., Hutchinson J. R. (2014). Anatomical and biomechanical traits of broiler chickens across ontogeny. Part II. Body segment inertial properties and muscle architecture of the pelvic limb. PeerJ 2, e473. doi:10.7717/peerj.473
Peixoto J. D. O., Savoldi I. R., Ibelli A. M. G., Cantão M. E., Jaenisch F. R. F., Giachetto P. F., et al. (2019). Proximal femoral head transcriptome reveals novel candidate genes related to epiphysiolysis in broiler chickens. BMC Genomics 20, 1031. doi:10.1186/s12864-019-6411-9
Petry B., Savoldi I. R., Ibelli A. M. G., Paludo E., de Oliveira Peixoto J., Jaenisch F. R. F., et al. (2018). New genes involved in the Bacterial Chondronecrosis with Osteomyelitis in commercial broilers. Livest. Sci. 208, 33–39. doi:10.1016/j.livsci.2017.12.003
Pfaffl M. W., Horgan G. W., Dempfle L. (2002). Relative expression software tool (REST) for group-wise comparison and statistical analysis of relative expression results in real-time PCR. Nucleic Acids Res. 30, e36. doi:10.1093/NAR/30.9.E36
Pouya F., Kerachian M. A. (2015). Avascular necrosis of the femoral head: Are any genes involved? Arch. Bone Jt. Surg. 3, 149–155.
Prisby R., Menezes T., Campbell J., Benson T., Samraj E., Pevzner I., et al. (2014). Kinetic examination of femoral bone modeling in broilers. Poult. Sci. 93, 1122–1129. doi:10.3382/PS.2013-03778
Purcell S., Neale B., Todd-Brown K., Thomas L., Ferreira M. A. R., Bender D., et al. (2007). Plink: A tool set for whole-genome association and population-based linkage analyses. Am. J. Hum. Genet. 81, 559–575. doi:10.1086/519795
R Core Team (2021). R: A language and environment for statistical computing. Available at: https://www.r-project.org.
Ramser A., Greene E., Wideman R., Dridi S. (2021). Local and systemic cytokine, chemokine, and FGF profile in bacterial chondronecrosis with osteomyelitis (BCO)-Affected broilers. Cells 10, 3174. doi:10.3390/CELLS10113174
Ramser A., Greene E., Alrubaye A. A. K., Wideman R., Dridi S. (2022). Role of autophagy machinery dysregulation in bacterial chondronecrosis with osteomyelitis. Poult. Sci. 101, 101750. doi:10.1016/J.PSJ.2022.101750
Rath N. C., Durairaj V. (2022). Avian bone physiology and poultry bone disorders. Sturkie’s Avian Physiol. 2022, 549–563. doi:10.1016/B978-0-12-819770-7.00037-2
Ritchie M. E., Phipson B., Wu D., Hu Y., Law C. W., Shi W., et al. (2015). Limma powers differential expression analyses for RNA-sequencing and microarray studies. Nucleic Acids Res. 43, e47. doi:10.1093/NAR/GKV007
Rojas-Peña M. L., Olivares-Navarrete R., Hyzy S., Arafat D., Schwartz Z., Boyan B. D., et al. (2014). Characterization of distinct classes of differential gene expression in osteoblast cultures from non-syndromic craniosynostosis bone. J. Genomics 2, 121–130. doi:10.7150/JGEN.8833
Ronkina N., Gaestel M. (2022). MAPK-activated protein kinases: Servant or partner? Annu. Rev. Biochem. 91, 505–540. doi:10.1146/ANNUREV-BIOCHEM-081720-114505
Santos C. E., Peixoto J. de O., Fernandes L. T., Marcelino D. E. P., Kawski V. L., Neis F. T., et al. (2022). Upregulated genes in articular cartilage may help to counteract femoral head separation in broilers with 21 days of age. Res. Vet. Sci. 147, 92–95. doi:10.1016/J.RVSC.2022.04.006
Santulli G. (2014). Angiopoietin-like proteins: A comprehensive look. Front. Endocrinol. 5, 4. doi:10.3389/fendo.2014.00004
Schmit F., Cremer S., Gaubatz S. (2009). LIN54 is an essential core subunit of the DREAM/LINC complex that binds to the cdc2 promoter in a sequence-specific manner. FEBS J. 276, 5703–5716. doi:10.1111/J.1742-4658.2009.07261.X
Sekiya I., Vuoristo J. T., Larson B. L., Prockop D. J. (2002). In vitro cartilage formation by human adult stem cells from bone marrow stroma defines the sequence of cellular and molecular events during chondrogenesis. Proc. Natl. Acad. Sci. U. S. A. 99, 4397–4402. doi:10.1073/PNAS.052716199
Shang J., Fan X., Shangguan L., Liu H., Zhou Y. (2015). Global gene expression profiling and alternative splicing events during the chondrogenic differentiation of human cartilage endplate-derived stem cells. Biomed. Res. Int. 2015, 604972. doi:10.1155/2015/604972
Shannon P., Markiel A., Ozier O., Baliga N. S., Wang J. T., Ramage D., et al. (2003). Cytoscape: A software environment for integrated models of biomolecular interaction networks. Genome Res. 13, 2498–2504. doi:10.1101/GR.1239303
Shukunami C., Oshima Y., Hiraki Y. (2005). Chondromodulin-I and tenomodulin: A new class of tissue-specific angiogenesis inhibitors found in hypovascular connective tissues. Biochem. Biophys. Res. Commun. 333, 299–307. doi:10.1016/J.BBRC.2005.05.133
Steiß V., Letschert T., Schäfer H., Pahl R. (2012). PERMORY-MPI: A program for high-speed parallel permutation testing in genome-wide association studies. Bioinformatics 28, 1168–1169. doi:10.1093/BIOINFORMATICS/BTS086
Szklarczyk D., Gable A. L., Lyon D., Junge A., Wyder S., Huerta-Cepas J., et al. (2019). STRING v11: Protein-protein association networks with increased coverage, supporting functional discovery in genome-wide experimental datasets. Nucleic Acids Res. 47, D607–D613. doi:10.1093/nar/gky1131
TNMD (2022). GeneCards - hum. gene database. Available at: https://www.genecards.org/cgi-bin/carddisp.pl?gene=TNMD (Accessed May 5, 2022).
Twine N. A., Chen L., Pang C. N., Wilkins M. R., Kassem M. (2014). Identification of differentiation-stage specific markers that define the ex vivo osteoblastic phenotype. Bone 67, 23–32. doi:10.1016/J.BONE.2014.06.027
Wickham H. (2016). “Elegant graphics for data analysis,” in ggplot2. 2nd ed. (Berlin, Germany: Springer Cham). doi:10.1007/978-3-319-24277-4_9
Wideman R. F. (2016). Bacterial chondronecrosis with osteomyelitis and lameness in broilers: A review. Poult. Sci. 95, 325–344. doi:10.3382/ps/pev320
Wilson F. D., Stayer P., Pace L. W., Hoerr F. J., Magee D. L. (2019). Disarticulation-associated femoral head separation in clinically normal broilers: Histologic documentation of underlying and predisposing cartilage abnormalities. Avian Dis. 63, 495–505. doi:10.1637/19-00090.1
Wu T., Hu E., Xu S., Chen M., Guo P., Dai Z., et al. (2021). clusterProfiler 4.0: A universal enrichment tool for interpreting omics data. Innov 2. doi:10.1016/J.XINN.2021.100141
Xavier G. M., Seppala M., Barrell W., Birjandi A. A., Geoghegan F., Cobourne M. T., et al. (2016). Hedgehog receptor function during craniofacial development. Dev. Biol. 415, 198–215. doi:10.1016/J.YDBIO.2016.02.009
Xu Z., Maiti D., Kisiel W., Duh E. J. (2006). Tissue factor pathway inhibitor-2 is upregulated by vascular endothelial growth factor and suppresses growth factor-induced proliferation of endothelial cells. Arterioscler. Thromb. Vasc. Biol. 26, 2819–2825. doi:10.1161/01.ATV.0000248731.55781.87
Yu J., Urban J. (2013). Immunolocalisation of fibrillin microfibrils in the calf metacarpal and vertebral growth plate. J. Anat. 223, 641–650. doi:10.1111/JOA.12123
Yu Y., Wang S., Zhou Z. (2020). Cartilage homeostasis affects femoral head necrosis induced by methylprednisolone in broilers. Int. J. Mol. Sci. 202021, 4841. doi:10.3390/IJMS21144841
Zhang C. C., Kaba M., Ge G., Xie K., Tong W., Hug C., et al. (2006). Angiopoietin-like proteins stimulate ex vivo expansion of hematopoietic stem cells. Nat. Med. 12, 240–245. doi:10.1038/NM1342
Keywords: chickens, gene expression, FBN2, FHS, femoral head necrosis, RNA-seq
Citation: Ibelli AMG, Peixoto JdO, Zanella R, Gouveia JJdS, Cantão ME, Coutinho LL, Marchesi JAP, Pizzol MSd, Marcelino DEP and Ledur MC (2022) Downregulation of growth plate genes involved with the onset of femoral head separation in young broilers. Front. Physiol. 13:941134. doi: 10.3389/fphys.2022.941134
Received: 11 May 2022; Accepted: 08 July 2022;
Published: 08 August 2022.
Edited by:
Shu-cheng Huang, Henan Agricultural University, ChinaReviewed by:
Wen-Chao Liu, Guangdong Ocean University, ChinaZhenlei Zhou, Nanjing Agricultural University, China
Zhi-Qiang Du, Yangtze University, China
Copyright © 2022 Ibelli, Peixoto, Zanella, Gouveia, Cantão, Coutinho, Marchesi, Pizzol, Marcelino and Ledur. This is an open-access article distributed under the terms of the Creative Commons Attribution License (CC BY). The use, distribution or reproduction in other forums is permitted, provided the original author(s) and the copyright owner(s) are credited and that the original publication in this journal is cited, in accordance with accepted academic practice. No use, distribution or reproduction is permitted which does not comply with these terms.
*Correspondence: Mônica Corrêa Ledur, bW9uaWNhLmxlZHVyQGVtYnJhcGEuYnI=
†Present address: Jorge Augusto Petroli Marchesi, MercoLab Laboratórios Ltda, Cascavel, Brazil