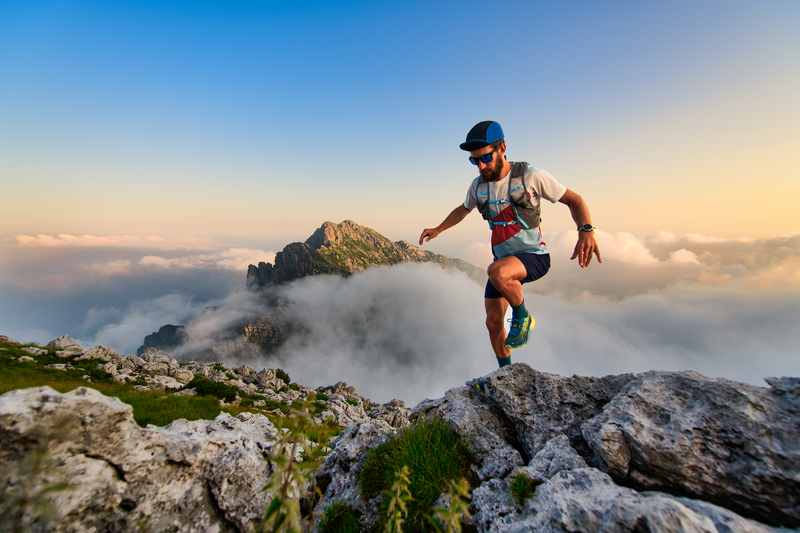
95% of researchers rate our articles as excellent or good
Learn more about the work of our research integrity team to safeguard the quality of each article we publish.
Find out more
SYSTEMATIC REVIEW article
Front. Physiol. , 15 July 2022
Sec. Clinical and Translational Physiology
Volume 13 - 2022 | https://doi.org/10.3389/fphys.2022.934731
Cerebral blood flow (CBF) is an important physiologic parameter that is vital for proper cerebral function and recovery. Current widely accepted methods of measuring CBF are cumbersome, invasive, or have poor spatial or temporal resolution. Near infrared spectroscopy (NIRS) based measures of cerebrovascular physiology may provide a means of non-invasively, topographically, and continuously measuring CBF. We performed a systematically conducted scoping review of the available literature examining the quantitative relationship between NIRS-based cerebrovascular metrics and CBF. We found that continuous-wave NIRS (CW-NIRS) was the most examined modality with dynamic contrast enhanced NIRS (DCE-NIRS) being the next most common. Fewer studies assessed diffuse correlation spectroscopy (DCS) and frequency resolved NIRS (FR-NIRS). We did not find studies examining the relationship between time-resolved NIRS (TR-NIRS) based metrics and CBF. Studies were most frequently conducted in humans and animal studies mostly utilized large animal models. The identified studies almost exclusively used a Pearson correlation analysis. Much of the literature supported a positive linear relationship between changes in CW-NIRS based metrics, particularly regional cerebral oxygen saturation (rSO2), and changes in CBF. Linear relationships were also identified between other NIRS based modalities and CBF, however, further validation is needed.
Cerebral blood flow (CBF), defined as the volume of blood flowing through a mass of brain over a period of time, is integral to brain function and a vital parameter in evaluating brain health (Fantini et al., 2016). Generally, the examination of CBF can be thought of either on the arterial scale or on the microvascular scale (arteries, capillaries, and venules). There are several existing methodologies that have been widely applied in the quantitative measurement of CBF on both scales. These included methods such as microsphere (MS) techniques that require sacrifice of the subject prior to measurement of CBF, making them only suitable for animal use. Alternatively, early non-invasive studies utilized a xenon133 clearance method (Thomas et al., 1979) while contemporary methods also exist such as positron emission tomography (PET) imaging (Frackowiak et al., 1980), perfusion weighted magnetic resonance imaging (PW-MRI; Zaro-Weber et al., 2009), arterial spin labelling MRI (ASL-MRI; Parkes et al., 2004), functional MRI derived CBF (fMRI-CBF; Chen et al., 2008), perfusion computed tomography (CTP; Hamberg et al., 1996), xenon-enhanced computed tomography (Xe-CT; Meyer et al., 1981), and single-photon emission computerized tomography (SPECT) imaging (Rootwelt et al., 1986). These methods, however, are generally cumbersome and have poor temporal resolution; some also involve the use of ionizing radiation. Temporal resolution can be improved by utilizing methods such as laser Doppler (Dirnagl et al., 1989) or thermal diffusion flowmetry (Mathieu et al., 2019). However, these methods only measure CBF in a small region of the brain and their invasive nature limits their scalability in the same subject.
Near infrared spectroscopy (NIRS) was first described as a means of evaluating cerebrovascular physiology in 1977 by Franz Jöbsis (Jöbsis, 1977). Fundamentally, this technology leverages the ability of near infrared (NIR) light (650–950 nm) to penetrate deep into tissues and be absorbed by a few physiologic chromophores including oxygenated and deoxygenated hemoglobin (OxHgB and deOxHgB respectively). Through the modified Beer-Lambert law the interaction of NIR light with tissues can be used to derive approximations of OxHgB and deOxHgB concentration (Cope, 1991). These in turn can be utilized to derive other parameters such as the total concentration of hemoglobin (tHgB) or the regional tissues oxygenation (rSO2) which is simply a function of the ratio of OxHgB to tHgB (Cope, 1991). The penetrative nature of NIRS allows it to act as a non-invasive continuous means of evaluating cerebrovascular physiology.
There are various modalities of NIRS that function on different variations of this principle. These included continuous wave NIRS (CW-NIRS), frequency-resolved NIRS (FR-NIRS), time-resolved NIRS (TR-NIRS), diffuse correlation spectroscopy (DCS), and dynamic contrast-enhanced NIRS (DCE-NIRS). CW-NIRS measures the degree of light at a fixed wavelength that is absorbed to determine a concentration of a particular chromophore. FR-NIRS modulates the intensity of its light source and measures the associated phase shift as it passes through the tissue. TR-NIRS generates ultrashort pulses of light and examines the temporal point spread function as the light emerges from the tissue. DCS examines the temporal fluctuations of reflected NIR light to produce a measure of CBF (CBFDCS). Finally, DCE-NIRS utilizes an optical tracer and applies the Fick principle to produce a measure of CBF (CBFDCE-NIRS). A detailed discussion of the technical nuances of these modalities is beyond the scope of this article. The interested reader is directed to comprehensive reviews of these technologies already present in the literature (Ferrari et al., 2004; Highton et al., 2010; Elwell and Cooper, 2011; Pellicer and Bravodel, 2011; Smith, 2011; Ghosh et al., 2012).
The non-invasive, continuous, and regional nature of NIRS makes it an ideal prospective method of evaluating cerebrovascular physiologic parameters as both the temporal evolution and topographic distribution of these parameters can be examined in a variety of settings and subjects. What is unclear is the association between these various parameters and CBF. Prior to NIRS becoming a “widely accepted” means of evaluating CBF the precise nature of this relationship must be understood. As such, the aim of this study was to provide a systematically conducted scoping review of the available human and animal literature that quantitatively examines the relationship between NIRS based cerebrovascular parameters and “widely accepted” measures of CBF.
Utilizing the methodological framework described by Arksey and O’Malley (Arksey and O’Malley, 2005), a systematically conducted scoping review of the available English language literature was conducted. The Preferred Reporting Items for Systematic Reviews and Meta-Analyses Extension for Scoping Reviews (PRISMA-ScR) guidelines were followed (Tricco et al., 2018). The methodology, including the search strategy, is similar to other scoping reviews published by our group (Froese et al., 2020a, 2020b, 2020c; Gomez et al., 2021b). Given that this is a systematic scoping review of the literature it was not eligible for registration in PROSPERO.
The review question and search strategy were decided upon by the senior author (F.A.Z.) and primary author (A.G.).
The question for this systematically conducted scoping review is as follows: What is the quantitative relationship between NIRS-based cerebrovascular metrics and CBF in humans and animals?
All English language studies, in humans or animals, that quantitatively examined the relationship between any NIRS-based cerebrovascular metric and a “widely accepted” measure of CBF were included. Any modality of NIRS was included, so long as it was aimed at measuring a cerebrovascular parameter. This included CW-NIRS, FR-NIRS, TR-NIRS, and DCE-NIRS as well as DCS. A full description of these methodologies can be found in a previous review by this group (Gomez et al., 2021c). “Widely accepted” measures of CBF included the following: Xenon133 clearance, MS techniques (radiolabelled, florescent, or coloured), laser Doppler flowmetry, thermal diffusion flowmetry, PET imaging, PW-MRI, ASL-MRI, fMRI-CBF, CTP, Xe-CT, and SPECT. The primary outcome of interest was the quantifiable relationship between NIRS-based cerebrovascular metrics and these measures of CBF.
Studies were excluded if there was no “widely accepted” measure of CBF. Notable methods that were not considered “widely accepted” measures of CBF included transcranial Doppler ultrasonography, as this method is reliant on a constant vessel diameter to be linearly related to CBF over time (Gomez et al., 2021a), and blood-oxygen-level-dependent functional magnetic resonance imaging (BOLD-fMRI), which only measures changes in oxygenated blood flow (Hillman, 2014). Additionally, studies that did not have quantitative measures of the relationship between NIRS-based cerebrovascular metrics and CBF were also excluded. Therefore, studies that only presented qualitative trends were not included. While significant advancements have been made in recent years with regards to computational models and simulations of CBF (Lan et al., 2020; Xie et al., 2022), for the purposes of this review in silico studies examining the relationship between NIRS based metrics and CBF were also excluded. Finally, review articles and non-English language articles were also excluded from consideration.
BIOSIS, Cochrane Library, EMBASE, MEDLINE, and SCOPUS were searched from the inception of each database to February 2022 using tailored search strategies for each database. As an example, the search strategy for BIOSIS, including the keywords utilized, can be seen in Supplementary Appendix A1. Similar strategies were used for each of the other databases. Search results were then combined, and deduplication was performed.
Using two reviewers (AG and AS), a two-step manual review of all articles returned by the search strategy was performed. In the first filter phase, each reviewer independently screened all studies identified using the above-described search strategy and determined if they met the inclusion criteria based on their title and abstract. The resulting list of studies was then passed through the second filter phase where once again each reviewer independently determined if the studies met the inclusion criteria, but this time based on the full text. Any discrepancies between the two reviewers were resolved by a third party (FZ). For any conference abstracts that were identified during this process an effort was made to identify associated peer-reviewed manuscripts for inclusion. Finally, the reference section of each article included in the final review were also examined to ensure no studies were missed.
Data was extracted from the final list of articles and compiled into various data fields. These fields included: the species of the subject examined and their clinical condition, the number of subjects, the experimental conditions, the “widely accepted” measure of CBF utilized, the NIRS modality or modalities utilized, the NIRS device utilized, the NIRS parameters examined, the results of the analysis of association, and any study limitations.
Given the goal of this review was to provide a comprehensive scoping overview of the available literature, a formal bias assessment was not conducted.
Due to the heterogeneity of the results/study designs and that the goal of the study was to conduct a scoping overview, no meta-analysis was performed.
The overall search and filtration results have been summarized in Figure 1 using a PRISMA flow-diagram. The combined results from the search, carried out over all five databases, yielded 15,309 articles. Following deduplication 8,187 unique articles were identified. During the first filter phase, 7,900 articles were found to not meet the inclusion/exclusion criteria based on their title and abstract. This left 287 articles for which the full text was reviewed in the second filter phase. After reviewing the full text, 242 articles were found to not meet inclusion/exclusion criteria leaving 45 articles. Examination of the reference sections of those texts yielded two additional articles resulting in 47 studies being included in this scoping review.
A number of different NIRS modalities were examined for their association with CBF. CW-NIRS was the most frequently utilized modality and was compared to CBF in 28 studies (Pryds et al., 1990, 2005; Skov et al., 1991; Bucher et al., 1993; Kaminogo et al., 1995; Kirkpatrick et al., 1995; Hock et al., 1997; Holzschuh et al., 1997; Newton et al., 1997; Villringer et al., 1997; Goddard-Finegold et al., 1998; Pellicer et al., 1999; Yamaguchi et al., 1999; Soul et al., 2000; Ogasawara et al., 2003; Huppert et al., 2006; Murata et al., 2006; Ricci et al., 2006; Naulaers et al., 2007; Themelis et al., 2007; Kim et al., 2010; Taussky et al., 2012; Alderliesten et al., 2014, 2017; Wintermark et al., 2014; Alosh et al., 2016; Polinder-Bos et al., 2020; Hashem et al., 2022). DCE-NIRS was the next most commonly used modality with its relationship to CBF examined in 12 studies (Kuebler et al., 1998; Roberts et al., 1998; Brown et al., 2001, 2002; De Visscher et al., 2003; Keller et al., 2003; Rothoerl et al., 2003; Schytz et al., 2009; Elliott et al., 2010, 2014; Saito et al., 2018; Milej et al., 2020). Of note, while most DCE-NIRS studies utilized continuous-wave technology (Kuebler et al., 1998; Roberts et al., 1998; Brown et al., 2001, 2002; De Visscher et al., 2003; Keller et al., 2003; Rothoerl et al., 2003; Schytz et al., 2009; Saito et al., 2018), two studies examined contrast signals utilizing time-resolved optical analysis (Elliott et al., 2014; Milej et al., 2020). DCS was utilized to measure CBF in seven studies (Zhou et al., 2009; Carp et al., 2010; Durduran et al., 2010; Kim et al., 2010; Shang et al., 2011; Baker et al., 2019; Giovannella et al., 2020), however five of these studies combined DCS with either FR-NIRS (Carp et al., 2010), CW-NIRS (Zhou et al., 2009; Durduran et al., 2010), or TR-NIRS (Baker et al., 2019; Giovannella et al., 2020) into a hybrid device for the purposes of improving the estimation of CBF. There was only one study that measured cerebrovascular parameters using FR-NIRS(Kurth et al., 2002) and no studies were found that compared TR-NIRS parameters and CBF directly. Table 1 Table, 2 to Table 3 summarize the findings of the various CW-NIRS, DCE-NIRS, and DCS studies respectively.
TABLE 1. Summary of articles examining continuous wave near infrared spectroscopic (CW-NIRS) cerebrovascular parameters.
TABLE 2. Summary of articles examining dynamic contrast enhanced near infrared spectroscopic (DCE-NIRS) cerebrovascular parameters.
TABLE 3. Summary of articles examining diffuse correlation spectroscopy (DCS) cerebrovascular parameters.
The number of subjects in each study varied widely from as few as one (Kirkpatrick et al., 1995; Themelis et al., 2007; Shang et al., 2011) to as many as 60 subjects (Kurth et al., 2002). The majority of studies examined human subjects (Pryds et al., 1990; Skov et al., 1991; Bucher et al., 1993; Kaminogo et al., 1995; Kirkpatrick et al., 1995; Hock et al., 1997; Holzschuh et al., 1997; Villringer et al., 1997; Yamaguchi et al., 1999; Keller et al., 2003; Ogasawara et al., 2003; Rothoerl et al., 2003; Huppert et al., 2006; Murata et al., 2006; Schytz et al., 2009; Durduran et al., 2010; Kim et al., 2010; Taussky et al., 2012; Alderliesten et al., 2014, 2017; Wintermark et al., 2014; Saito et al., 2018; Baker et al., 2019; Milej et al., 2020; Polinder-Bos et al., 2020), and while some were conducted in neonates (Pryds et al., 1990; Skov et al., 1991; Bucher et al., 1993; Durduran et al., 2010; Wintermark et al., 2014; Alderliesten et al., 2017), most were conducted in adults that were either healthy (Villringer et al., 1997; Keller et al., 2003; Huppert et al., 2006; Schytz et al., 2009; Alderliesten et al., 2017; Milej et al., 2020) or in some pathologic state (Kaminogo et al., 1995; Kirkpatrick et al., 1995; Hock et al., 1997; Holzschuh et al., 1997; Yamaguchi et al., 1999; Ogasawara et al., 2003; Rothoerl et al., 2003; Murata et al., 2006; Kim et al., 2010; Taussky et al., 2012; Saito et al., 2018; Baker et al., 2019; Polinder-Bos et al., 2020). Porcine models where the second most popular subjects, with 16 studies utilizing them (Goddard-Finegold et al., 1998; Kuebler et al., 1998; Roberts et al., 1998; Pellicer et al., 1999; Soul et al., 2000; Brown et al., 2001, 2002; Kurth et al., 2002; Ricci et al., 2006; Naulaers et al., 2007; Themelis et al., 2007; Zhou et al., 2009; Elliott et al., 2010, 2014; Alosh et al., 2016; Giovannella et al., 2020). Rodent models were relatively uncommon and were found in only five studies (De Visscher et al., 2003; Pryds et al., 2005; Carp et al., 2010; Shang et al., 2011; Hashem et al., 2022). Only one study was conducted in a canine model (Newton et al., 1997).
There was a wide variety of methods utilized to determine CBF. The majority of animal studies used microsphere (MS) techniques with 7 using radiolabeled MS (Newton et al., 1997; Goddard-Finegold et al., 1998; Kuebler et al., 1998; Roberts et al., 1998; Pellicer et al., 1999; Soul et al., 2000; Ricci et al., 2006), 3 using colored MS (De Visscher et al., 2003; Naulaers et al., 2007; Alosh et al., 2016), and one study using florescent MS (Zhou et al., 2009). This methodology requires sacrifice of the subject and were therefore limited to animal studies. Less invasive radiographic techniques were also commonly used in animal and human subjects including ASL-MRI (Huppert et al., 2006; Carp et al., 2010; Durduran et al., 2010; Wintermark et al., 2014; Alderliesten et al., 2017; Milej et al., 2020; Hashem et al., 2022), PW-MRI (Keller et al., 2003), fMRI-CBF (Alderliesten et al., 2014), CTP (Brown et al., 2001, 2002; Elliott et al., 2010, 2014; Taussky et al., 2012), XeCT (Kim et al., 2010), SPECT (Yamaguchi et al., 1999; Ogasawara et al., 2003; Murata et al., 2006; Schytz et al., 2009), and PET (Hock et al., 1997; Villringer et al., 1997; Saito et al., 2018; Giovannella et al., 2020; Polinder-Bos et al., 2020). Xenon133 clearance based methods of determining CBF were also commonly utilized, especially in earlier studies (Pryds et al., 1990; Skov et al., 1991; Bucher et al., 1993; Kaminogo et al., 1995; Holzschuh et al., 1997; Rothoerl et al., 2003). Invasive in vivo methods such as laser Doppler flowmetry (Kirkpatrick et al., 1995; Kurth et al., 2002; Pryds et al., 2005; Themelis et al., 2007; Shang et al., 2011) and thermal diffusion flowmetry (Baker et al., 2019) were also utilized.
As mentioned earlier, of the articles included in this review, CW-NIRS was the most common modality of NIRS utilized. These CW-NIRS studies are summarized in Table 1. This was likely attributable, in part, by the widespread availability of commercially produced CW-NIRS devices. Only 7 of the 28 CW-NIRS studies utilized a device developed in-house (Pryds et al., 1990, 2005; Skov et al., 1991; Goddard-Finegold et al., 1998; Huppert et al., 2006; Kim et al., 2010; Hashem et al., 2022).
The vast majority of studies identified some degree of statistically significant positive Pearson correlation between the CW-NIRS parameter examined and their measure of CBF (R = 0.247 to 0.95; Pryds et al., 1990, 2005; Skov et al., 1991; Bucher et al., 1993; Kaminogo et al., 1995; Kirkpatrick et al., 1995; Hock et al., 1997; Holzschuh et al., 1997; Newton et al., 1997; Villringer et al., 1997; Goddard-Finegold et al., 1998; Yamaguchi et al., 1999; Soul et al., 2000; Ogasawara et al., 2003; Huppert et al., 2006; Murata et al., 2006; Themelis et al., 2007; Taussky et al., 2012; Alderliesten et al., 2014, 2017; Wintermark et al., 2014; Alosh et al., 2016; Polinder-Bos et al., 2020). In those studies that found a significant correlation between CW-NIRS parameters and CBF, the majority were examining a correlation with a relative change in CBF (R = 0.247 to 0.925; Pryds et al., 1990, 2005; Kaminogo et al., 1995; Kirkpatrick et al., 1995; Hock et al., 1997; Holzschuh et al., 1997; Villringer et al., 1997; Yamaguchi et al., 1999; Soul et al., 2000; Ogasawara et al., 2003; Huppert et al., 2006; Themelis et al., 2007; Polinder-Bos et al., 2020), however 10 studies did find a correlation with absolute values of CBF (R = 0.64 to 0.95; Skov et al., 1991; Bucher et al., 1993; Newton et al., 1997; Goddard-Finegold et al., 1998; Murata et al., 2006; Taussky et al., 2012; Alderliesten et al., 2014, 2017; Wintermark et al., 2014; Alosh et al., 2016). The CW-NIRS parameter that was most commonly found to be correlated with either absolute or relative change in CBF was rSO2, which was more commonly examined in recent studies (Kaminogo et al., 1995; Yamaguchi et al., 1999; Ogasawara et al., 2003; Taussky et al., 2012; Alderliesten et al., 2014, 2017; Wintermark et al., 2014; Alosh et al., 2016; Polinder-Bos et al., 2020). However, in one of these studies a correlation was only identified in one hemisphere (Polinder-Bos et al., 2020) and in another of these studies a correlation was only present in the sickest subgroup of patients (newborns with severe hypoxic-ischemic encephalopathy; Wintermark et al., 2014). The second most common CW-NIRS parameter was tHgB, which was found to correlate with relative changes in CBF in five studies (Pryds et al., 1990, 2005; Hock et al., 1997; Villringer et al., 1997; Huppert et al., 2006) and absolute CBF in one study (Murata et al., 2006). Notably, in 4 of the earlier studies that identified a correlation with absolute values of CBF, the CW-NIRS parameter was a derived measure of CBF based on the Fick principle, with oxygen utilized as an endogenous tracer (Skov et al., 1991; Bucher et al., 1993; Newton et al., 1997; Goddard-Finegold et al., 1998).
Of note, not all studies found a positive correlation between CW-NIRS parameters and CBF. There were five studies included in this review where either no correlation was identified (Pellicer et al., 1999; Naulaers et al., 2007; Kim et al., 2010; Hashem et al., 2022) or a negative correlation was found between the CW-NIRS parameter and CBF (Ricci et al., 2006; Hashem et al., 2022).
Distinct from other modalities, DCE-NIRS requires the use of an exogenous contrast agent and application of the Fick principle (Gomez et al., 2021c). In all of the articles that leveraged this modality, indocyanine green (ICG) was utilized as the contrast agent (Kuebler et al., 1998; Roberts et al., 1998; Brown et al., 2001, 2002; De Visscher et al., 2003; Keller et al., 2003; Rothoerl et al., 2003; Schytz et al., 2009; Elliott et al., 2010, 2014; Saito et al., 2018; Milej et al., 2020). These studies are summarized in Table 2. Dissimilar to those articles that utilized CW-NIRS, commercially produced devices were used in less than half of the articles (Kuebler et al., 1998; Roberts et al., 1998; Rothoerl et al., 2003; Schytz et al., 2009; Saito et al., 2018).
Most of these studies found a significant positive Pearson correlation between CBF derived from DCE-NIRS (CBFDCE-NIRS) and that determined utilizing a “widely accepted” method (Kuebler et al., 1998; Roberts et al., 1998; Brown et al., 2001, 2002; De Visscher et al., 2003; Elliott et al., 2010, 2014; Saito et al., 2018; Milej et al., 2020). In this group CBFDCE-NIRS was correlated with absolute values of CBF (R = 0.6466 to 0.99; Kuebler et al., 1998; Roberts et al., 1998; Brown et al., 2001, 2002; De Visscher et al., 2003; Elliott et al., 2010, 2014; Milej et al., 2020), with the exception of one study which found a correlation between hemispheric ratios of CBF when measured by PET and DCE-NIRS (R = 0.618; p = 0.0004; Saito et al., 2018).
There were three studies where a CBFDCE-NIRS was found to not significantly correlate with CBF as measured by PW-MRI (Keller et al., 2003), Xenon133 clearance (Rothoerl et al., 2003), or SPECT (Schytz et al., 2009).
The studies included in this scoping review that utilized DCS, a variant of NIRS, to optically probe cerebrovascular physiology are summarized in Table 3. There are a limited number of commercially available DCS devices and as such only a single study was found to use one (Giovannella et al., 2020), while the remaining studies used devices assembled in-house (Zhou et al., 2009; Carp et al., 2010; Durduran et al., 2010; Kim et al., 2010; Shang et al., 2011; Baker et al., 2019). In 5 of the seven studies that derived a CBF utilizing DCS (CBFDCS), a strong positive Pearson correlation was found with relative changes in CBF measured by “widely accepted” techniques (R = 0.70 to 0.97; Zhou et al., 2009; Carp et al., 2010; Durduran et al., 2010; Kim et al., 2010; Shang et al., 2011). The most recent study, which was the only one to utilize a commercially available device, was also the only one to find a positive correlation with absolute values of CBF (R = 0.94; p < 0.0001; Giovannella et al., 2020). In one study, CBFDCS was not found to be correlated with CBF as measured by thermal diffusion flowmetry (R = 0.15; range -0.35 to 0.60; Baker et al., 2019). Notably, this is the only study in this scoping review that utilized thermal diffusion flowmetry to measure CBF.
There was only one study identified that utilized FR-NIRS. Kurth and others compared CBF, as measured by laser Doppler flowmetry, to rSO2, as measured by FR-NIRS, in a cohort of 60 newborn piglets subjected to hyperoxia, hypercapnia, and bilateral carotid occlusion. It was found that rSO2, as measured by their in-house FR-NIRS device, had a significant positive correlation with the percent of baseline CBF (R = 0.89; p < 0.001). The slope of this relationship was 1.75 with an intercept of -21. Notably, the FR-NIRS probe was placed directly on the skull limiting the generatability of these findings to clinical applications (Kurth et al., 2002).
There were no studies identified that examined the correlation between a purely TR-NIRS derived parameter and CBF as measured by a “widely accepted” method.
Through this systematic scoping review of the literature, 47 studies have been identified that quantitatively examine the relationship between cerebrovascular parameters, as measured by NIRS, and CBF, as measured by “widely accepted” methods. The most popular modality studied in the literature was CW-NIRS, likely attributable to the number of commercially available devices and ease of use (Pryds et al., 1990, 2005; Skov et al., 1991; Bucher et al., 1993; Kaminogo et al., 1995; Kirkpatrick et al., 1995; Hock et al., 1997; Holzschuh et al., 1997; Newton et al., 1997; Villringer et al., 1997; Goddard-Finegold et al., 1998; Pellicer et al., 1999; Yamaguchi et al., 1999; Soul et al., 2000; Ogasawara et al., 2003; Huppert et al., 2006; Murata et al., 2006; Ricci et al., 2006; Naulaers et al., 2007; Themelis et al., 2007; Kim et al., 2010; Taussky et al., 2012; Alderliesten et al., 2014, 2017; Wintermark et al., 2014; Alosh et al., 2016; Polinder-Bos et al., 2020; Hashem et al., 2022). DCE-NIRS (Kuebler et al., 1998; Roberts et al., 1998; Brown et al., 2001, 2002; De Visscher et al., 2003; Keller et al., 2003; Rothoerl et al., 2003; Schytz et al., 2009; Elliott et al., 2010, 2014; Saito et al., 2018; Milej et al., 2020) and DCS (Zhou et al., 2009; Carp et al., 2010; Durduran et al., 2010; Kim et al., 2010; Shang et al., 2011; Baker et al., 2019; Giovannella et al., 2020) methodologies were commonly reported on as well. This is despite their more complicated protocols and setups. There was only one study that examined the relationship between FR-NIRS and measures of CBF (Kurth et al., 2002), while no study compared TR-NIRS parameters directly with CBF. The relative advantages and limitations of each NIRS modality that were identified through this review have been summarized in Table 4.
TABLE 4. Summary of limitations and advantages of various NIRS modalities identified through this review.
Most of the studies identified examined human subject (Pryds et al., 1990; Skov et al., 1991; Bucher et al., 1993; Kaminogo et al., 1995; Kirkpatrick et al., 1995; Hock et al., 1997; Holzschuh et al., 1997; Villringer et al., 1997; Yamaguchi et al., 1999; Keller et al., 2003; Ogasawara et al., 2003; Rothoerl et al., 2003; Huppert et al., 2006; Murata et al., 2006; Schytz et al., 2009; Durduran et al., 2010; Kim et al., 2010; Taussky et al., 2012; Alderliesten et al., 2014, 2017; Wintermark et al., 2014; Saito et al., 2018; Baker et al., 2019; Milej et al., 2020; Polinder-Bos et al., 2020). Neonates were examined in a minority of studies which is surprising, as their thin scalp and skull are thought to render the neonatal brain particularly well suited for examination with NIRS (Dix et al., 2017). This may be a product of the strict inclusion criteria of this scoping review, that required comparison with a “widely accepted” measure of CBF, a practically challenging feat in neonates, especially those that are premature (Pryds and Edwards, 1996). Animal models were also commonly used with porcine models being the most popular in the studies included in this review (Goddard-Finegold et al., 1998; Kuebler et al., 1998; Roberts et al., 1998; Pellicer et al., 1999; Soul et al., 2000; Brown et al., 2001, 2002; Kurth et al., 2002; Ricci et al., 2006; Naulaers et al., 2007; Themelis et al., 2007; Zhou et al., 2009; Elliott et al., 2010, 2014; Alosh et al., 2016; Giovannella et al., 2020). Given that large animal models provide a closer approximation to human brains due to their similar cerebrovascular structure and gyrencephalic nature the presence of very few small animal studies can be a seen as a relative strength of this body of literature (Sorby-Adams et al., 2018). The requirement of most NIRS devices to have an emitter and detector that are separated in space may have contributed to this preference for human and large animal models. Generally, subject numbers were small with the largest study having 60 participants (Kurth et al., 2002). Once again, this is likely due to the significant resource intensive nature of any study comparing NIRS cerebrovascular parameters to a “widely accepted” measure of CBF.
A variety of “widely accepted” methods of determining true CBF were utilized in the studies included in this scoping review. Despite the heterogeneity, most methodologies either had a defined region over which CBF was being measured or the ability to define a region of interest. This is of particular importance when comparing these values to NIRS parameters as NIRS technology, by its nature, evaluates regional cerebrovascular physiology and is not a global measure. That said, not all studies aimed to match these regions of interest when evaluating associations, and as such, these studies tended to fail in identifying a relationship between parameters (Pryds et al., 1990; Skov et al., 1991; Bucher et al., 1993; Kaminogo et al., 1995; Holzschuh et al., 1997; Goddard-Finegold et al., 1998; Pellicer et al., 1999; De Visscher et al., 2003; Ricci et al., 2006; Naulaers et al., 2007; Zhou et al., 2009; Durduran et al., 2010; Giovannella et al., 2020).
There appears to be relatively robust literature supporting some degree of positive linear association between CW-NIRS parameters and CBF. Some of the most compelling associations, especially when only considering absolute values of CBF, were found when the Fick principle was utilized with oxygen as a tracer (Skov et al., 1991; Bucher et al., 1993; Newton et al., 1997; Goddard-Finegold et al., 1998). This method requires a sudden increase in oxygen saturation, and as such, can only be utilized as an intermittent method to evaluate CBF. Additionally, these studies assume a constant rate of oxygen consumption and ignored any possible effect a sharp increase in oxygenation may have on cerebral vasculature.
Several CW-NIRS parameter were examined for their association with CBF in the identified literature. This included OxHgB (the concentration of oxygenated hemoglobin in the tissue interrogated), deOxHgB (the concentration of deoxygenated hemoglobin in the tissue interrogated), tHgB (the total concentration of hemoglobin in the tissue interrogated), as well as TOI and RSO2 (which are both ratios of the concentrations of oxygenated hemoglobin to total hemoglobin in the tissue interrogated). Additionally, ΔHgB (the difference between the concentrations of OxHgB and deOxHgB in the tissue interrogated) was also examined.
The parameter of tHgB seemed to mostly be associated with changes in CBF and not absolute values of CBF (Pryds et al., 1990, 2005; Hock et al., 1997; Villringer et al., 1997; Huppert et al., 2006). This may not be entirely surprising as changes in the totally concentration of hemoglobin in a volume of brain would be expected to increase with increased CBV. Somewhat surprisingly, rSO2 was frequently found to have a linear relationship with CBF, especially when also considering changes from some baseline (Kaminogo et al., 1995; Holzschuh et al., 1997; Yamaguchi et al., 1999; Ogasawara et al., 2003; Taussky et al., 2012; Alderliesten et al., 2014, 2017; Wintermark et al., 2014; Alosh et al., 2016; Polinder-Bos et al., 2020). An interesting finding of this study is that this relationship seems to breakdown in hypothermic subjects (Naulaers et al., 2007; Wintermark et al., 2014). However, this was only found in two studies and will therefore need further validation. Given that rSO2 is the ratio of OxHgB to tHgB this may indicate that changes in CBF are primarily driven by arteriolar dilation or contraction and have a linear effect on the ratio of OxHgB to tHgB, over some range. The disproportionate change in OxHgB compared to deOxHgB with changes in CBF is further evidenced by two studies that found that changes ΔHgB correlated well with changes in CBF, once again indicating that changes in CBF my primarily be secondary to increases in arteriolar volume (Soul et al., 2000; Pryds et al., 2005).
A total of five studies failed to find any association between CBF and CW-NIRS parameters (Pellicer et al., 1999; Ricci et al., 2006; Naulaers et al., 2007; Kim et al., 2010; Hashem et al., 2022). It is important to note that in three of these studies CW-NIRS parameters were compared to global CBF as opposed to in the region of interest examined by NIRS (Pellicer et al., 1999; Ricci et al., 2006; Naulaers et al., 2007). In the study by Hashem and others, atypical methods were utilized to derive the CW-NIRS parameters that involved the assumption that tHgB was equal to deOxHgB during an induced anoxic pulse (Hashem et al., 2022). Finally, in the study by Kim and others, sample size was small, and a moderate correlation was found but failed to meet statistical significance (Kim et al., 2010).
In studies that utilized DCE-NIRS, the contrast agent of choice was ICG. Generally, there was a strong association between absolute CBFDCE-NIRS and CBF as measured by “widely accepted” methods. However, there were exceptions to this. In the study conducted by Keller and others, the limits of agreement were unacceptably large, but this is likely attributable to a small sample size and an inability to carry out measurements simultaneously with both modalities (Keller et al., 2003). In the article by Rothoerl and others, rSO2 was used to monitor the progression of the ICG contrast (Rothoerl et al., 2003). This is particularly unusual since rSO2 is a function of the ratio of OxHgB to tHgB, both of which would be affected by a change in optical density caused by ICG. Finally, it is likely that methodologic errors were at play in the article by Schytz and others as there was a failure to detect any change in CBFDCE-NIRS before and after the administration of acetazolamide (Schytz et al., 2009).
There are limitations to this methodology. As with any measure of CBF utilizing the Fick principle, DCE-NIRS is limited to only intermittent measurements of CBF. While the temporal resolution of these methods is likely limited by contrast washout rates, practically, frequent measurements may primarily be limited by the need for a contrast agent altogether. Additionally, two of the studies required radiographic imaging to determine the thickness of extracerebral tissue prior to the measurement of cerebrovascular parameters (Elliott et al., 2010; Milej et al., 2020).
Measures of change in CBF generally correlated well with changes in CBFDCS however, there were few studies that examined this relationship. The one study, by Baker and others, that failed to find any association was limited by the fact that DCS probes were not always placed on the same side as the thermal diffusion flowmetry probes (Baker et al., 2019). Additional validation of these findings will be required before any definitive conclusions can be drawn.
In the study by Kurth and others, a strong association was found between rSO2 and change in CBF from baseline. While this study contained the largest number of subjects of any study in this review, it is still only a single study. Additionally, in this study the optical probe was placed directly on the skull, limiting its generalizability to the clinical setting (Kurth et al., 2002).
It should also be noted that in the DCS study by Carp and others, FR-NIRS was utilized to determine the optical properties of the tissues and improve the measurement of CBFDCS (Carp et al., 2010).
While no studies examined the association between TR-NIRS parameters and CBF, the technology was utilized in two studies to improve the accuracy of CBFDCS measurements (Baker et al., 2019; Giovannella et al., 2020). Additionally, two studies using DCE-NIRS utilized TR-NIRS to measure changes caused by the contrast bolus (Elliott et al., 2014; Milej et al., 2020).
When considering limitations of this body of literature it is important to consider the statistical methodology utilized to evaluate the association between CBF and NIRS cerebrovascular parameters. Most studies assessed for simple correlations between parameters. In some cases, only the correlation between changes from baseline were evaluated. While this does provide evidence that NIRS parameters of cerebrovascular physiology move with CBF, any correlation analysis does not provide information about potential confounders.
One obvious confounder is interference due to extracerebral tissue. If a change in CBF is induced that has a concurrent change in scalp blood flow a positive correlation might be seen even if the NIRS signal is primarily influenced by the scalp. While some studies utilized hybrid technologies or spatially resolved techniques to handle interference from the scalp others simply removed the scalp in their animal model. Of note, one study was able to examine this relationship and found that signals from the scalp did not correlate with NIRS signals in their experimental setup (Kuebler et al., 1998).
Another source of error can be the various experimental conditions that effect CBF along with another cerebrovascular parameter that are more directly detected by NIRS. An example of this would be hypercapnic induced elevations in CBF. This experimental condition was utilized in numerous experiments and is accompanied by cerebral vasodilation, thereby increasing cerebral blood volume (CBV). It is unclear, in these experiments, if it is perhaps a change in CBV that is being detected, especially in the setting of CW-NIRS devices. The vasoactive effects of oxygen and xenon, similarly, have not been accounted for in a number of studies (Skov et al., 1991; Bucher et al., 1993; Newton et al., 1997; Goddard-Finegold et al., 1998; Kim et al., 2010). This is important to consider as it may not be the mechanism by which all increases, or decreases, in CBF occur.
CBF has a complex regulatory mechanism that can be influenced by numerous factors including neuronal activity, systemic blood pressure, pharmacologic agents, and various pathologic states (Slupe and Kirsch, 2018; Liu et al., 2020a; Korte et al., 2020). CBF can also be measured on the scale of large arteries or on the scale of the microvasculature. Given that NIRS primary examines CBF at the microvascular level, concordance with method of measuring CBF at the arterial level maybe limited, especially considering that the factors that influence CBF on the arterial scale (Leng et al., 2017; Liu et al., 2018) can be distinct from those that influence microvascular CBF (Tikhomirova et al., 2011; Liu et al., 2021).This difference in scale of measurement has not been fully explored in the literature despite NIRS becoming increasingly substituted as an alternative method of evaluating CBF in clinical studies(Yang et al., 2019; Tian et al., 2021; Guo and Chen, 2022).
The articles identified by this review generally failed to perform comparative analysis beyond a simple correlation. While some did perform a Bland-Altman analysis, it was often incomplete and did not assess a trend in the bias. Additionally, in those situations where CBF could be measured continuously, such as with laser Doppler flowmetry, alongside NIRS parameters, there was no attempt at time series analysis.
Finally, a major limitation to the articles identified by this systematically conducted scoping review is the generally small cohort sizes. As mentioned previously, this is likely due to the cumbersome nature of the methods involved. It is difficult to have confidence in conclusions drawn from the studies identified in this review with so few data points. It should be acknowledged that a wider body of literature that has examined the use of NIRS based methods to measure blood flow in other tissues does exist and should, to some degree, bolster confidence in the findings of the studies identified in this review (Choo et al., 2017; Bangalore-Yogananda et al., 2018; Dennis et al., 2021).
This systematically conducted review aimed to provide a scoping overview of the literature examining the relationship between CBF and various NIRS parameters. There are, however, inherent limitations to this review. Given the heterogeneity of the experimental designs, subjects, and modalities utilized a meta-analysis of the data was not possible. Even within similar NIRS modalities, studies were significantly different in their experimental conditions such that a meta-analysis would not be feasible or appropriate. Additionally, the decision of what methods of measuring CBF were acceptable was somewhat arbitrary. An argument could be made to include only those studies that utilized rigorous microsphere techniques and Xenon133 clearance. Conversely, it could also be argued that methods such as TCD and BOLD-fMRI provide reasonable enough methods of measuring CBF despite obvious confounders. This study aimed to strike a balance and include methods that had reasonably accurate measurements of CBF and not just a related cerebrovascular physiologic parameter. One corollary of the methods that were determined to be “widely acceptable” measures of CBF is that there are inconsistencies between what each modality is measuring. Of note, laser Doppler flowmetry is more accurately a measure of CBF velocity at the cortical surface and as such, does not entirely measure the volume of blood passing through a mass of brain tissue. The result is that certain interventions that are aimed to modulate CBF, such as administration of vasodilators, may increase CBF while reducing CBF velocity. Further to this point, certain “widely-accepted” methods of measuring CBF, such as CTP and ASL-MRI, primarily measure CBF in large cerebral vessels. This contrasts with NIRS modalities that primarily in the cerebral microvasculature (arterioles, capillaries, and venules). As a result, concordance between these methods should not entirely be strictly expected as various interventions and pathologic states may affect these differently.
Finally, given the overall globalization of scientific research, the inclusion of only English-language studies limits the available literature for inclusion in this review. This was simply made as a pragmatic decision based on the limitations of the authors involved in this study.
The results of this scoping review of the literature provide some guidance for future avenues of exploration. Given the large body of evidence supporting the association between CW-NIRS parameters and CBF, there should be a degree of confidence in research that utilizes these modalities as a means of interrogating changes in CBF as a component of cerebral multimodal monitoring. Such clinical research is already ongoing (Kim et al., 2014; Weigl et al., 2014; Trofimov et al., 2016). Beyond this, CW-NIRS parameters likely serve as an ideal non-invasive method of continuously monitoring cerebral autoregulation. These methods are continuously updating indices that examine the correlation between arterial blood pressure (ABP) and CW-NIRS parameters (Zeiler et al., 2017). These indices are a particularly well-suited application of CW-NIRS as they, by design, rely only on the relative change in CBF and not absolute values of it. They have been shown in animal models to accurately determine the lower limit of cerebral autoregulation (Brady et al., 2007, 2010). This is now being used as a method of not only measuring cerebral autoregulation in the acute setting but, due to its non-invasive nature, is being explored as a means of tracking the recovery of cerebral autoregulation in the chronic phase (Gomez et al., 2020b; 2020a).
Accurate evaluations of absolute CBF are likely to be derived from modalities of NIRS that leverage the Fick principle. This may be done through CW-NIRS methods in which oxygen is utilized as an endogenous tracer or DCE-NIRS where ICG is used as an exogenous contrast agent. These methods, as mentioned previously, are limited by their temporal resolution but may be more suitable for applications, both research and clinical, that require only “moment in time” measurement of CBF and therefore may be a viable alternative to cumbersome, low temporal resolution methods such as Xe-CT, ASL-MRI or PET imaging.
The literature around DCS, FR-NIRS, and TR-NIRS is relatively scant with regards to their ability to accurately measure CBF, or correlate with CBF changes. Prior to these modalities being accepted as non-invasive measures of CBF more work is needed. Studies involving large cohorts that utilize more sophisticated methods of comparison with “widely accepted” measures of CBF need to be conducted to validate the body of literature currently supporting their use.
Finally, given that the regulation of CBF on both the arterial and microvascular scale is complex, with numerous variables affecting flow, non-linear computational models that describe cerebral hemodynamics in various conditions may provide vital insights into the precise relationship between CBF and NIRS based metrics (Liu et al., 2020b; Xie et al., 2022). Examining NIRS based metrics in light of these computational models deserves further exploration.
In this scoping review of the literature examining the quantitative relationship between CBF and NIRS- based cerebrovascular measures, CW-NIRS was the most examined modality with DCE-NIRS, DCS, and FR-NIRS also being examined, but to a lesser degree. The identified studies almost exclusively examined this relationship with correlation analysis. There was a significant amount of literature supporting a positive linear relationship between changes in CW-NIRS based metrics, particularly rSO2, and changes in CBF. Linear relationships were also identified between other NIRS based modalities and CBF, however, further validation is needed.
The original contributions presented in the study are included in the article/Supplementary Material, further inquiries can be directed to the corresponding author.
AG: Designed the search strategy and search terms, collected articles for review, undertook review and screening of the literature, and prepared the manuscript. AS: Undertook review and screening of the literature as well as aided in preparing the manuscript. LF: Aided in preparation of the manuscript. CB: Aided in preparation of the manuscript. TS: Aided in preparation of the manuscript. KS: Aided in preparation of the manuscript. DC: Aided in preparation of the manuscript. FM: Aided in preparation of the manuscript. FZ: Responsible for conceptual development of this article, development of search strategy, undertook screening of the literature, and aided in preparation of the manuscript.
This work was directly supported through the United States National Institutes of Health (NIH) through the National Institute of Neurological Disorders and Stroke (NINDS) (Grant #: R03NS114335-01) and Natural Sciences and Engineering Council of Canada (NSERC) Discovery Grant Program (RGPIN-2022-03621). The content is solely the responsibility of the authors and does not necessarily represent the official views of the National Institutes of Health. AG is supported through the University of Manitoba Clinician Investigator Program, the University of Manitoba Dean’s Fellowship, the Manitoba Medical Services Foundation Research and Education Fellowship, and the R. Samuel McLaughlin Research Fellowship. AS is supported through the University of Manitoba, Department of Surgery GFT Research Grant. LF is supported through the University of Manitoba–Department of Surgery GFT Research Grant, the University of Manitoba Office of Research Services (ORS)—University Research Grant Program (URGP), the University of Manitoba Biomedical Engineering Fellowship Program and the Edward R. Toporeck Graduate Fellowship in Engineering. CB is supported through the Centre on Aging at the University of Manitoba. TS is supported through the University of Manitoba, Department of Surgery GFT Research Grant. FZ receives research support from the University of Manitoba–Manitoba Public Insurance (MPI) Professorship in Neuroscience, the Health Sciences Centre Foundation/MPI Neuroscience Research Operating Fund, the United States National Institutes of Health (NIH) through the National Institute of Neurological Disorders and Stroke (NINDS) (Grant #: R03NS114335-01), the Natural Sciences and Engineering Research Council of Canada (NSERC) (RGPIN-2022-03621), the Canada Foundation for Innovation (CFI) (Project #: 38583), Research Manitoba (Grant #: 3906), and the University of Manitoba VPRI Research Investment Fund (RIF).
DC is affiliated with the Pan Am Clinic Foundation which receives general education and research support from ConMed Linvatec, Ossur, Zimmer Biomet, and Arthrex.
The remaining authors declare that the research was conducted in the absence of any commercial or financial relationships that could be construed as a potential conflict of interest.
All claims expressed in this article are solely those of the authors and do not necessarily represent those of their affiliated organizations, or those of the publisher, the editors and the reviewers. Any product that may be evaluated in this article, or claim that may be made by its manufacturer, is not guaranteed or endorsed by the publisher.
The Supplementary Material for this article can be found online at: https://www.frontiersin.org/articles/10.3389/fphys.2022.934731/full#supplementary-material
Alderliesten T., De Vis J. B., Lemmers P. M. A., van Bel F., Benders M. J. N. L., Hendrikse J., et al. (2014). Simultaneous Quantitative Assessment of Cerebral Physiology Using Respiratory-Calibrated MRI and Near-Infrared Spectroscopy in Healthy Adults. Neuroimage 85 (Pt 1), 255–263. doi:10.1016/j.neuroimage.2013.07.015
Alderliesten T., De Vis J. B., Lemmers P. M., Hendrikse J., Groenendaal F., Van Bel F., et al. (2017). Brain Oxygen Saturation Assessment in Neonates Using T2-Prepared Blood Imaging of Oxygen Saturation and Near-Infrared Spectroscopy. J. Cereb. Blood Flow. Metab. 37, 902–913. doi:10.1177/0271678X16647737
Alosh H., Ramirez A., Mink R. (2016). The Correlation between Brain Near-Infrared Spectroscopy and Cerebral Blood Flow in Piglets with Intracranial Hypertension. J. Appl. Physiol. 121, 255–260. doi:10.1152/japplphysiol.00760.2015
Arksey H., O'Malley L. (2005). Scoping Studies: towards a Methodological Framework. Int. J. Soc. Res. Methodol. 8, 19–32. doi:10.1080/1364557032000119616
Baker W. B., Balu R., He L., Kavuri V. C., Busch D. R., Amendolia O., et al. (2019). Continuous Non-invasive Optical Monitoring of Cerebral Blood Flow and Oxidative Metabolism after Acute Brain Injury. J. Cereb. Blood Flow. Metab. 39, 1469–1485. doi:10.1177/0271678X19846657
Bangalore-Yogananda C.-G., Rosenberry R., Soni S., Liu H., Nelson M. D., Tian F. (2018). Concurrent Measurement of Skeletal Muscle Blood Flow during Exercise with Diffuse Correlation Spectroscopy and Doppler Ultrasound. Biomed. Opt. Express 9, 131. doi:10.1364/BOE.9.000131
Brady K. M., Lee J. K., Kibler K. K., Smielewski P., Czosnyka M., Easley R. B., et al. (2007). Continuous Time-Domain Analysis of Cerebrovascular Autoregulation Using Near-Infrared Spectroscopy. Stroke 38, 2818–2825. doi:10.1161/STROKEAHA.107.485706
Brady K. M., Mytar J. O., Kibler K. K., Hogue C. W., Lee J. K., Czosnyka M., et al. (2010). Noninvasive Autoregulation Monitoring with and without Intracranial Pressure in the Naïve Piglet Brain. Anesth. Analgesia 111, 191–195. doi:10.1213/ANE.0b013e3181e054ba
Brown D. W., Picot P. A., Gharavi Naeini J., Springett R., Delpy D. T., Lee T.-Y. (2002). Quantitative Near Infrared Spectroscopy Measurement of Cerebral Hemodynamics in Newborn Piglets. Pediatr. Res. 51, 564–570. doi:10.1203/00006450-200205000-00004
Brown D. W., Picot P. A., Springett R., Delpy D. T., Lee T.-Y. (2001). Comparison of Near-Infrared Spectroscopy with CT Cerebral Blood Flow Measurements in Newborn Piglets. Proc. SPIE - Int. Soc. Opt. Eng. 4321, 168–176. doi:10.1117/12.428134
Bucher H.-U., Edwards A. D., Lipp A. E., Duc G. (1993). Comparison between Near Infrared Spectroscopy and 133xenon Clearance for Estimation of Cerebral Blood Flow in Critically Ill Preterm Infants. Pediatr. Res. 33, 56–60. doi:10.1203/00006450-199301000-00012
Carp S. A., Dai G. P., Boas D. A., Franceschini M. A., Kim Y. R. (2010). Validation of Diffuse Correlation Spectroscopy Measurements of Rodent Cerebral Blood Flow with Simultaneous Arterial Spin Labeling MRI; towards MRI-Optical Continuous Cerebral Metabolic Monitoring. Biomed. Opt. Express 1, 553–565. doi:10.1364/BOE.1.000553
Chen J. J., Wieckowska M., Meyer E., Pike G. B. (2008). Cerebral Blood Flow Measurement Using fMRI and PET: a Cross-Validation Study. Int. J. Biomed. Imaging 2008, 516359. doi:10.1155/2008/516359
Choo H. C., Nosaka K., Peiffer J. J., Ihsan M., Yeo C. C., Abbiss C. R. (2017). Reliability of Laser Doppler, Near-Infrared Spectroscopy and Doppler Ultrasound for Peripheral Blood Flow Measurements during and after Exercise in the Heat. J. Sports Sci. 35, 1715–1723. doi:10.1080/02640414.2016.1235790
Cope M. (1991). The Development of a Near Infrared Spectroscopy System and its Application for Non Invasive Monitoring of Cerebral Blood and Tissue Oxygenation in the Newborn Infants. College London (United Kingdom): University of London, University.
De Visscher G., Leunens V., Borgers M., Reneman R. S., Flameng W., Van Rossem K. (2003). NIRS Mediated CBF Assessment: Validating the Indocyanine Green Bolus Transit Detection by Comparison with Coloured Microsphere Flowmetry. Adv. Exp. Med. Biol. 540. 37–45. doi:10.1007/978-1-4757-6125-2_7
Dennis J. J., Wiggins C. C., Smith J. R., Isautier J. M. J., Johnson B. D., Joyner M. J., et al. (2021). Measurement of Muscle Blood Flow and O2 Uptake via Near-Infrared Spectroscopy Using a Novel Occlusion Protocol. Sci. Rep. 11, 918. doi:10.1038/s41598-020-79741-w
Dirnagl U., Kaplan B., Jacewicz M., Pulsinelli W. (1989). Continuous Measurement of Cerebral Cortical Blood Flow by Laser-Doppler Flowmetry in a Rat Stroke Model. J. Cereb. Blood Flow. Metab. 9, 589–596. doi:10.1038/jcbfm.1989.84
Dix L. M. L., van Bel F., Lemmers P. M. A. (2017). Monitoring Cerebral Oxygenation in Neonates: An Update. Front. Pediatr. 5, 46. doi:10.3389/fped.2017.00046
Durduran T., Zhou C., Buckley E. M., Kim M. N., Yu G., Choe R., et al. (2010). Optical Measurement of Cerebral Hemodynamics and Oxygen Metabolism in Neonates with Congenital Heart Defects. J. Biomed. Opt. 15, 037004. doi:10.1117/1.3425884
Elliott J. T., Diop M., Morrison L. B., d'Esterre C. D., Lee T.-Y., St. Lawrence K. (2014). Quantifying Cerebral Blood Flow in an Adult Pig Ischemia Model by a Depth-Resolved Dynamic Contrast-Enhanced Optical Method. NeuroImage 94, 303–311. doi:10.1016/j.neuroimage.2014.03.023
Elliott J. T., Diop M., Tichauer K. M., Lee T.-Y., Lawrence K. S. K. (2010). Quantitative Measurement of Cerebral Blood Flow in a Juvenile Porcine Model by Depth-Resolved Near-Infrared Spectroscopy. J. Biomed. Opt. 15, 037014. doi:10.1117/1.3449579
Elwell C. E., Cooper C. E. (2011). Making Light Work: Illuminating the Future of Biomedical Optics. Phil. Trans. R. Soc. A 369, 4358–4379. doi:10.1098/rsta.2011.0302
Fantini S., Sassaroli A., Tgavalekos K. T., Kornbluth J. (2016). Cerebral Blood Flow and Autoregulation: Current Measurement Techniques and Prospects for Noninvasive Optical Methods. Neurophoton 3, 031411. doi:10.1117/1.NPh.3.3.031411
Ferrari M., Mottola L., Quaresima V. (2004). Principles, Techniques, and Limitations of Near Infrared Spectroscopy. Can. J. Appl. Physiol. 29, 463–487. doi:10.1139/h04-031
Frackowiak R. S. J., Lenzi G.-L., Jones T., Heather J. D. (1980). Quantitative Measurement of Regional Cerebral Blood Flow and Oxygen Metabolism in Man Using 15O and Positron Emission Tomography. J. Comput. Assisted Tomogr. 4, 727–736. doi:10.1097/00004728-198012000-00001
Froese L., Dian J., Batson C., Gomez A., Unger B., Zeiler F. A. (2020a). Cerebrovascular Response to Propofol, Fentanyl, and Midazolam in Moderate/Severe Traumatic Brain Injury: A Scoping Systematic Review of the Human and Animal Literature. Neurotrauma Rep. 1, 100–112. doi:10.1089/neur.2020.0040
Froese L., Dian J., Gomez A., Unger B., Zeiler F. A. (2020b). Cerebrovascular Response to Phenylephrine in Traumatic Brain Injury: A Scoping Systematic Review of the Human and Animal Literature. Neurotrauma Rep. 1, 46–62. doi:10.1089/neur.2020.0008
Froese L., Dian J., Gomez A., Unger B., Zeiler F. A. (2020c). The Cerebrovascular Response to Norepinephrine: A Scoping Systematic Review of the Animal and Human Literature. Pharmacol. Res. Perspect. 8, e00655. doi:10.1002/prp2.655
Ghosh A., Elwell C., Smith M. (2012). Cerebral Near-Infrared Spectroscopy in Adults. Anesth. Analg. 115, 1373–1383. doi:10.1213/ANE.0b013e31826dd6a6
Giovannella M., Andresen B., Andersen J. B., El-Mahdaoui S., Contini D., Spinelli L., et al. (2020). Validation of Diffuse Correlation Spectroscopy against 15O-Water PET for Regional Cerebral Blood Flow Measurement in Neonatal Piglets. J. Cereb. Blood Flow. Metab. 40, 2055–2065. doi:10.1177/0271678X19883751
Goddard-Finegold J., Louis P. T., Rodriguez D. L., David Y., Contant C. F., Rolfe P. (1998). Correlation of Near Infrared Spectroscopy Cerebral Blood Flow Estimations and Microsphere Quantitations in Newborn Piglets. Neonatology 74, 376–384. doi:10.1159/000014056
Gomez A., Batson C., Froese L., Sainbhi A. S., Zeiler F. A. (2021a). Utility of Transcranial Doppler in Moderate and Severe Traumatic Brain Injury: A Narrative Review of Cerebral Physiologic Metrics. J. Neurotrauma 38, 2206–2220. doi:10.1089/neu.2020.7523
Gomez A., Dian J., Froese L., Zeiler F. A. (2020a). Near-Infrared Cerebrovascular Reactivity for Monitoring Cerebral Autoregulation and Predicting Outcomes in Moderate to Severe Traumatic Brain Injury: Proposal for a Pilot Observational Study. JMIR Res. Protoc. 9, e18740. doi:10.2196/18740
Gomez A., Dian J., Zeiler F. A. (2020b). Continuous and Entirely Non-invasive Method for Cerebrovascular Reactivity Assessment: Technique and Implications. J. Clin. Monit. Comput. 35, 307–315. doi:10.1007/s10877-020-00472-4
Gomez A., Froese L., Sainbhi A. S., Batson C., Zeiler F. A. (2021b). Transcranial Doppler Based Cerebrovascular Reactivity Indices in Adult Traumatic Brain Injury: A Scoping Review of Associations with Patient Oriented Outcomes. Front. Pharmacol. 12, 690921. doi:10.3389/fphar.2021.690921
Gomez A., Sainbhi A. S., Froese L., Batson C., Alizadeh A., Mendelson A. A., et al. (2021c). Near Infrared Spectroscopy for High-Temporal Resolution Cerebral Physiome Characterization in TBI: A Narrative Review of Techniques, Applications, and Future Directions. Front. Pharmacol. 12, 719501. doi:10.3389/fphar.2021.719501
Guo Z., Chen F. (2022). Idle-state Detection in Motor Imagery of Articulation Using Early Information: A Functional Near-Infrared Spectroscopy Study. Biomed. Signal Process. Control 72, 103369. doi:10.1016/j.bspc.2021.103369
Hamberg L. M., Hunter G. J., Halpern E. F., Hoop B., Gazelle G. S., Wolf G. L. (1996). Quantitative High-Resolution Measurement of Cerebrovascular Physiology with Slip-Ring CT. AJNR Am. J. Neuroradiol. 17, 639–650.
Hashem M., Shafqat Q., Wu Y., Rho J. M., Dunn J. F. (2022). Abnormal Oxidative Metabolism in the Cuprizone Mouse Model of Demyelination: An In Vivo NIRS-MRI Study. NeuroImage 250, 118935. doi:10.1016/j.neuroimage.2022.118935
Highton D., Elwell C., Smith M. (2010). Noninvasive Cerebral Oximetry: Is There Light at the End of the Tunnel? Curr. Opin. Anaesthesiol. 23, 576–581. doi:10.1097/aco.0b013e32833e1536
Hillman E. M. C. (2014). Coupling Mechanism and Significance of the BOLD Signal: a Status Report. Annu. Rev. Neurosci. 37, 161–181. doi:10.1146/annurev-neuro-071013-014111
Hock C., Villringer K., Heekeren H., Schuh-Hofer S., Hofmann M., Minoshima S., et al. (1997). Decrease in Parietal Cerebral Hemoglobin Oxygenation during Performance of a Verbal Fluency Task in Patients with Alzheimer’s Disease Monitored by Means of Near-Infrared Spectroscopy (NIRS) — Correlation with Simultaneous rCBF-PET Measurements. Brain Res. 755 (2), 293–303. doi:10.1016/s0006-8993(97)00122-4
Holzschuh M., Woertgen C., Metz C., Brawanski A., Cohadon F., Reulen H., et al. (1997). Comparison of Changes in Cerebral Blood Flow and Cerebral Oxygen Saturation Measured by Near Infrared Spectroscopy (NIRS) after Acetazolamide. Acta Neurochir. 139, 58–62. doi:10.1007/bf01850869
Huppert T. J., Hoge R. D., Diamond S. G., Franceschini M. A., Boas D. A. (2006). A Temporal Comparison of BOLD, ASL, and NIRS Hemodynamic Responses to Motor Stimuli in Adult Humans. NeuroImage 29, 368–382. doi:10.1016/j.neuroimage.2005.08.065
Jöbsis F. F. (1977). Noninvasive, Infrared Monitoring of Cerebral and Myocardial Oxygen Sufficiency and Circulatory Parameters. Science 198, 1264–1267. doi:10.1126/science.929199
Kaminogo M., Ichikura A., Shibata S., Toba T., Yonekura M. (1995). Effect of Acetazolamide on Regional Cerebral Oxygen Saturation and Regional Cerebral Blood Flow. Stroke 26, 2358–2360. doi:10.1161/01.STR.26.12.2358
Keller E., Nadler A., Alkadhi H., Kollias S. S., Yonekawa Y., Niederer P. (2003). Noninvasive Measurement of Regional Cerebral Blood Flow and Regional Cerebral Blood Volume by Near-Infrared Spectroscopy and Indocyanine Green Dye Dilution. NeuroImage 20, 828–839. doi:10.1016/S1053-8119(03)00315-X
Kim M. N., Durduran T., Frangos S., Edlow B. L., Buckley E. M., Moss H. E., et al. (2010). Noninvasive Measurement of Cerebral Blood Flow and Blood Oxygenation Using Near-Infrared and Diffuse Correlation Spectroscopies in Critically Brain-Injured Adults. Neurocrit Care 12, 173–180. doi:10.1007/s12028-009-9305-x
Kim M. N., Edlow B. L., Durduran T., Frangos S., Mesquita R. C., Levine J. M., et al. (2014). Continuous Optical Monitoring of Cerebral Hemodynamics during Head-Of-Bed Manipulation in Brain-Injured Adults. Neurocrit Care 20, 443–453. doi:10.1007/s12028-013-9849-7
Kirkpatrick P. J., Smielewski P., Czosnyka M., Menon D. K., Pickard J. D. (1995). Near-infrared Spectroscopy Use in Patients with Head Injury. J. Neurosurg. 83, 963–970. doi:10.3171/jns.1995.83.6.0963
Korte N., Nortley R., Attwell D. (2020). Cerebral Blood Flow Decrease as an Early Pathological Mechanism in Alzheimer's Disease. Acta Neuropathol. 140, 793–810. doi:10.1007/s00401-020-02215-w
Kuebler W. M., Sckell A., Habler O., Kleen M., Kuhnle G. E. H., Welte M., et al. (1998). Noninvasive Measurement of Regional Cerebral Blood Flow by Near-Infrared Spectroscopy and Indocyanine Green. J. Cereb. Blood Flow. Metab. 18, 445–456. doi:10.1097/00004647-199804000-00013
Kurth C. D., Levy W. J., McCann J. (2002). Near-infrared Spectroscopy Cerebral Oxygen Saturation Thresholds for Hypoxia-Ischemia in Piglets. J. Cereb. Blood Flow. Metab. 22, 335–341. doi:10.1097/00004647-200203000-00011
Lan L., Leng X., Ip V., Soo Y., Abrigo J., Liu H., et al. (2020). Sustaining Cerebral Perfusion in Intracranial Atherosclerotic Stenosis: The Roles of Antegrade Residual Flow and Leptomeningeal Collateral Flow. J. Cereb. Blood Flow. Metab. 40, 126–134. doi:10.1177/0271678X18805209
Leng X., Lan L., Ip V. H. L., Liu H., Abrigo J., Liebeskind D. S., et al. (2017). Noninvasive Fractional Flow in Intracranial Atherosclerotic Stenosis: Reproducibility, Limitations, and Perspectives. J. Neurological Sci. 381, 150–152. doi:10.1016/j.jns.2017.08.3239
Liu H., Chen F., Hartmann V., Khalid S. G., Hughes S., Zheng D. (2020a). Comparison of Different Modulations of Photoplethysmography in Extracting Respiratory Rate: from a Physiological Perspective. Physiol. Meas. 41, 094001. doi:10.1088/1361-6579/abaaf0
Liu H., Lan L., Abrigo J., Ip H. L., Soo Y., Zheng D., et al. (2021). Comparison of Newtonian and Non-newtonian Fluid Models in Blood Flow Simulation in Patients with Intracranial Arterial Stenosis. Front. Physiol. 12, 718540. doi:10.3389/fphys.2021.718540
Liu H., Lan L., Leng X., Ip H. L., Leung T. W. H., Wang D., et al. (2018). Impact of Side Branches on the Computation of Fractional Flow in Intracranial Arterial Stenosis Using the Computational Fluid Dynamics Method. J. Stroke Cerebrovasc. Dis. 27, 44–52. doi:10.1016/j.jstrokecerebrovasdis.2017.02.032
Liu H., Wang D., Leng X., Zheng D., Chen F., Wong L. K. S., et al. (2020b). State-of-the-Art Computational Models of Circle of Willis with Physiological Applications: A Review. IEEE Access 8, 156261–156273. doi:10.1109/ACCESS.2020.3007737
Mathieu F., Khellaf A., Thelin E. P., Zeiler F. A. (2019). Continuous Thermal Diffusion-Based Cerebral Blood Flow Monitoring in Adult Traumatic Brain Injury: A Scoping Systematic Review. J. Neurotrauma 36, 1707–1723. doi:10.1089/neu.2018.6309
Meyer J. S., Hayman L. A., Amano T., Nakajima S., Shaw T., Lauzon P., et al. (1981). Mapping Local Blood Flow of Human Brain by CT Scanning during Stable Xenon Inhalation. Stroke 12, 426–436. doi:10.1161/01.str.12.4.426
Milej D., He L., Abdalmalak A., Baker W. B., Anazodo U. C., Diop M., et al. (2020). Quantification of Cerebral Blood Flow in Adults by Contrast-Enhanced Near-Infrared Spectroscopy: Validation against MRI. J. Cereb. Blood Flow. Metab. 40, 1672–1684. doi:10.1177/0271678x19872564
Murata Y., Sakatani K., Hoshino T., Fujiwara N., Kano T., Nakamura S., et al. (2006). Effects of Cerebral Ischemia on Evoked Cerebral Blood Oxygenation Responses and BOLD Contrast Functional MRI in Stroke Patients. Stroke 37, 2514–2520. doi:10.1161/01.STR.0000239698.50656.3b
Naulaers G., Meyns B., Miserez M., Leunens V., Van Huffel S., Casaer P., et al. (2007). Use of Tissue Oxygenation Index and Fractional Tissue Oxygen Extraction as Non-invasive Parameters for Cerebral Oxygenation. Neonatology 92, 120–126. doi:10.1159/000101063
Newton C. R. J. C., Wilson D. A., Gunnoe E., Wagner B., Cope M., Traystman R. J. (1997). Measurement of Cerebral Blood Flow in Dogs with Near Infrared Spectroscopy in the Reflectance Mode Is Invalid. J. Cereb. Blood Flow. Metab. 17, 695–703. doi:10.1097/00004647-199706000-00011
Ogasawara K., Konno H., Yukawa H., Endo H., Inoue T., Ogawa A. (2003). Transcranial Regional Cerebral Oxygen Saturation Monitoring during Carotid Endarterectomy as a Predictor of Postoperative Hyperperfusion. Neurosurgery 53, 309–315. discussion 314-5. doi:10.1227/01.neu.0000073547.86747.f3
Parkes L. M., Rashid W., Chard D. T., Tofts P. S. (2004). Normal Cerebral Perfusion Measurements Using Arterial Spin Labeling: Reproducibility, Stability, and Age and Gender Effects. Magn. Reson. Med. 51, 736–743. doi:10.1002/mrm.20023
Pellicer A., Aparicio M., Cabañas F., Valverde E., Quero J., Stiris T. A. (1999). Effect of the Cyclo-Oxygenase Blocker Ibuprofen on Cerebral Blood Volume and Cerebral Blood Flow during Normocarbia and Hypercarbia in Newborn Piglets. Acta Paediatr. 88, 82–88. doi:10.1080/08035259950170664
Pellicer A., Bravodel M. d. C. C. (2011). Near-infrared Spectroscopy: A Methodology-Focused Review. Seminars Fetal Neonatal Med. 16, 42–49. doi:10.1016/j.siny.2010.05.003
Polinder-Bos H. A., Elting J. W. J., Aries M. J., García D. V., Willemsen A. T., van Laar P. J., et al. (2020). Changes in Cerebral Oxygenation and Cerebral Blood Flow during Hemodialysis - A Simultaneous Near-Infrared Spectroscopy and Positron Emission Tomography Study. J. Cereb. Blood Flow. Metab. 40, 328–340. doi:10.1177/0271678x18818652
Pryds A., Tønnesen J., Pryds O., Knudsen G. M., Greisen G. (2005). Cerebral Pressure Autoregulation and Vasoreactivity in the Newborn Rat. Pediatr. Res. 57, 294–298. doi:10.1203/01.PDR.0000148714.51310.5E
Pryds O., Edwards A. D. (1996). Cerebral Blood Flow in the Newborn Infant. Archives Dis. Child. - Fetal Neonatal Ed. 74, F63–F69. doi:10.1136/fn.74.1.f63
Pryds O., Greisen G., Skov L. L., Friis-Hansen B. (1990). Carbon Dioxide-Related Changes in Cerebral Blood Volume and Cerebral Blood Flow in Mechanically Ventilated Preterm Neonates: Comparison of Near Infrared Spectrophotometry and 133Xenon Clearance. Pediatr. Res. 27, 445–449. doi:10.1203/00006450-199005000-00006
Ricci M., Lombardi P., Schultz S., Galindo A., Coscarella E., Vasquez A., et al. (2006). Near-infrared Spectroscopy to Monitor Cerebral Oxygen Saturation in Single-Ventricle Physiology. J. Thorac. Cardiovasc. Surg. 131, 395–402. doi:10.1016/j.jtcvs.2005.07.039
Roberts I. G., Fallon P., Kirkham F. J., Kirshbom P. M., Cooper C. E., Elliott M. J., et al. (1998). Measurement of Cerebral Blood Flow during Cardiopulmonary Bypass with Near-Infrared Spectroscopy. J. Thorac. Cardiovasc. Surg. 115, 94–102. doi:10.1016/S0022-5223(98)70447-7
Rootwelt K., Dybevold S., Nyberg-Hansen R., Russell D. (1986). Measurement of Cerebral Blood Flow with 133Xe Inhalation and Dynamic Single Photon Emission Computer Tomography. Normal Values. Scand. J. Clin. Lab. Invest Suppl. 184, 97–105.
Rothoerl R. D., Schebesch K. M., Faltermeier R., Woertgen C., Brawanski A. (2003). Lack of Correlation between Xenon133and Near Infrared Spectroscopy/indocyanine Green rCBF Measurements. Neurological Res. 25, 528–532. doi:10.1179/016164103101201779
Saito H., Ishikawa T., Tanabe J., Kobayashi S., Moroi J. (2018). Bedside Assessment of Regional Cerebral Perfusion Using Near-Infrared Spectroscopy and Indocyanine Green in Patients with Atherosclerotic Occlusive Disease. Sci. Rep. 8, 1242. doi:10.1038/s41598-018-19668-5
Schytz H. W., Wienecke T., Jensen L. T., Selb J., Boas D. A., Ashina M. (2009). Changes in Cerebral Blood Flow after Acetazolamide: An Experimental Study Comparing Near-Infrared Spectroscopy and SPECT. Eur. J. Neurology 16, 461–467. doi:10.1111/j.1468-1331.2008.02398.x
Shang Y., Chen L., Toborek M., Yu G. (2011). Diffuse Optical Monitoring of Repeated Cerebral Ischemia in Mice. Opt. Express 19, 20301–20315. doi:10.1364/OE.19.020301
Skov L., Pryds O., Greisen G. (1991). Estimating Cerebral Blood Flow in Newborn Infants: Comparison of Near Infrared Spectroscopy and 133Xe Clearance. Pediatr. Res. 30, 570–573. doi:10.1203/00006450-199112000-00016
Slupe A. M., Kirsch J. R. (2018). Effects of Anesthesia on Cerebral Blood Flow, Metabolism, and Neuroprotection. J. Cereb. Blood Flow. Metab. 38, 2192–2208. doi:10.1177/0271678X18789273
Smith M. (2011). Shedding Light on the Adult Brain: a Review of the Clinical Applications of Near-Infrared Spectroscopy. Phil. Trans. R. Soc. A 369, 4452–4469. doi:10.1098/rsta.2011.0242
Sorby-Adams A. J., Vink R., Turner R. J. (2018). Large Animal Models of Stroke and Traumatic Brain Injury as Translational Tools. Am. J. Physiology-Regulatory, Integr. Comp. Physiology 315, R165–R190. doi:10.1152/ajpregu.00163.2017
Soul J. S., Taylor G. A., Wypij D., Duplessis A. J., Volpe J. J. (2000). Noninvasive Detection of Changes in Cerebral Blood Flow by Near-Infrared Spectroscopy in a Piglet Model of Hydrocephalus. Pediatr. Res. 48, 445–449. doi:10.1203/00006450-200010000-00005
Taussky P., O'Neal B., Daugherty W. P., Luke S., Thorpe D., Pooley R. A., et al. (2012). Validation of Frontal Near-Infrared Spectroscopy as Noninvasive Bedside Monitoring for Regional Cerebral Blood Flow in Brain-Injured Patients. Foc 32, E2. doi:10.3171/2011.12.FOCUS11280
Themelis G., D’Arceuil H., Diamond S. G., Thaker S., Huppert T. J., Boas D. A., et al. (2007). Near-infrared Spectroscopy Measurement of the Pulsatile Component of Cerebral Blood Flow and Volume from Arterial Oscillations. J. Biomed. Opt. 12, 014033. doi:10.1117/1.2710250
Thomas D. J., Zilkha E., Redmond S., Du Boulay G. H., Marshall J., Ross Russell R. W., et al. (1979). An Intravenous 133Xenon Clearance Technique for Measuring Cerebral Blood Flow. J. Neurological Sci. 40, 53–63. doi:10.1016/0022-510X(79)90008-X
Tian X., Liu Y., Guo Z., Cai J., Tang J., Chen F., et al. (2021). Cerebral Representation of Sound Localization Using Functional Near-Infrared Spectroscopy. Front. Neurosci. 15, 739706. doi:10.3389/fnins.2021.739706
Tikhomirova I. A., Oslyakova A. O., Mikhailova S. G. (2011). Microcirculation and Blood Rheology in Patients with Cerebrovascular Disorders. Clin. Hemorheol. Microcirc. 49, 295–305. doi:10.3233/CH-2011-1480
Tricco A. C., Lillie E., Zarin W., O'Brien K. K., Colquhoun H., Levac D., et al. (2018). PRISMA Extension for Scoping Reviews (PRISMA-ScR): Checklist and Explanation. Ann. Intern Med. 169, 467–473. doi:10.7326/M18-0850
Trofimov A. O., Kalentiev G., Voennov O., Grigoryeva V. (2016). Comparison of Cerebral Oxygen Saturation and Cerebral Perfusion Computed Tomography in Cerebral Blood Flow in Patients with Brain Injury. Adv. Exp. Med. Biol. 876, 145–149. doi:10.1007/978-1-4939-3023-4_18
Villringer K., Minoshima S., Hock C., Obrig H., Ziegler S., Dirnagl U., et al. (1997). Assessment of Local Brain Activation: A Simultaneous PET and Near- Infrared Spectroscopy Study. Springer, 149–153. doi:10.1007/978-1-4899-0056-2_16
Weigl W., Milej D., Gerega A., Toczylowska B., Kacprzak M., Sawosz P., et al. (2014). Assessment of Cerebral Perfusion in Post-traumatic Brain Injury Patients with the Use of ICG-Bolus Tracking Method. Neuroimage 85 (Pt 1), 555–565. doi:10.1016/j.neuroimage.2013.06.065
Wintermark P., Hansen A., Warfield S. K., Dukhovny D., Soul J. S. (2014). Near-infrared Spectroscopy versus Magnetic Resonance Imaging to Study Brain Perfusion in Newborns with Hypoxic-Ischemic Encephalopathy Treated with Hypothermia. NeuroImage 85, 287–293. doi:10.1016/j.neuroimage.2013.04.072
Xie J., Cheng Z., Gu L., Wu B., Zhang G., Shiu W., et al. (2022). Evaluation of Cerebrovascular Hemodynamics in Vascular Dementia Patients with a New Individual Computational Fluid Dynamics Algorithm. Comput. Methods Programs Biomed. 213, 106497. doi:10.1016/j.cmpb.2021.106497
Yamaguchi H., Yamauchi H., Hazama S., Hamamoto H., Inoue N. (1999). Correlation between Cerebral Oxygen Metabolism and Cerebral Blood Flow Simultaneously Measured before and after Acetazolamide Administration. J. Biomed. Opt. 4, 418–423. doi:10.1117/1.429946
Yang M., Yang Z., Yuan T., Feng W., Wang P. (2019). A Systemic Review of Functional Near-Infrared Spectroscopy for Stroke: Current Application and Future Directions. Front. Neurol. 10, 58. doi:10.3389/fneur.2019.00058
Zaro-Weber O., Moeller-Hartmann W., Heiss W.-D., Sobesky J. (2009). The Performance of MRI-Based Cerebral Blood Flow Measurements in Acute and Subacute Stroke Compared with 15O-Water Positron Emission Tomography. Stroke 40, 2413–2421. doi:10.1161/STROKEAHA.108.540914
Zeiler F. A., Donnelly J., Menon D. K., Smielewski P., Zweifel C., Brady K., et al. (2017). Continuous Autoregulatory Indices Derived from Multi-Modal Monitoring: Each One Is Not like the Other. J. Neurotrauma 34, 3070–3080. doi:10.1089/neu.2017.5129
Keywords: biomedical optics, cerebral blood flow (CBF), cerebrovascular physiology, near infrared spectroscopy, scoping review
Citation: Gomez A, Sainbhi AS, Froese L, Batson C, Slack T, Stein KY, Cordingley DM, Mathieu F and Zeiler FA (2022) The Quantitative Associations Between Near Infrared Spectroscopic Cerebrovascular Metrics and Cerebral Blood Flow: A Scoping Review of the Human and Animal Literature. Front. Physiol. 13:934731. doi: 10.3389/fphys.2022.934731
Received: 03 May 2022; Accepted: 09 June 2022;
Published: 15 July 2022.
Edited by:
Xiuyun Liu, Johns Hopkins University, United StatesReviewed by:
Alexander Ruesch, Carnegie Mellon University, United StatesCopyright © 2022 Gomez, Sainbhi, Froese, Batson, Slack, Stein, Cordingley, Mathieu and Zeiler. This is an open-access article distributed under the terms of the Creative Commons Attribution License (CC BY). The use, distribution or reproduction in other forums is permitted, provided the original author(s) and the copyright owner(s) are credited and that the original publication in this journal is cited, in accordance with accepted academic practice. No use, distribution or reproduction is permitted which does not comply with these terms.
*Correspondence: Alwyn Gomez, Z29tZXphMzVAbXl1bWFuaXRvYmEuY2E=
Disclaimer: All claims expressed in this article are solely those of the authors and do not necessarily represent those of their affiliated organizations, or those of the publisher, the editors and the reviewers. Any product that may be evaluated in this article or claim that may be made by its manufacturer is not guaranteed or endorsed by the publisher.
Research integrity at Frontiers
Learn more about the work of our research integrity team to safeguard the quality of each article we publish.