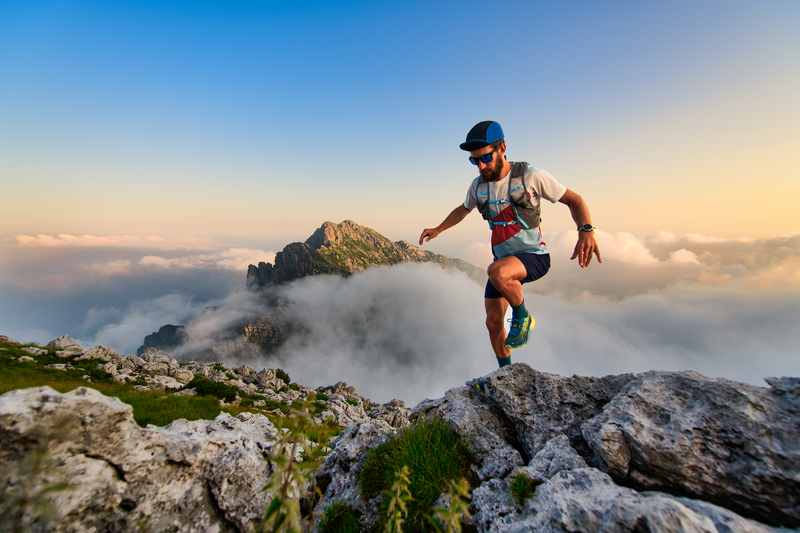
95% of researchers rate our articles as excellent or good
Learn more about the work of our research integrity team to safeguard the quality of each article we publish.
Find out more
ORIGINAL RESEARCH article
Front. Physiol. , 05 September 2022
Sec. Autonomic Neuroscience
Volume 13 - 2022 | https://doi.org/10.3389/fphys.2022.934372
This article is part of the Research Topic Insights in Autonomic Neuroscience: 2021 View all 10 articles
Background: Periodic breathing (PB) is a cyclical breathing pattern composed of alternating periods of hyperventilation (hyperpnea, HP) and central apnea (CA). Differences in PB phenotypes mainly reside in HP length. Given that respiration modulates muscle sympathetic nerve activity (MSNA), which decreases during HP and increases during CA, the net effects of PB on MSNA may critically depend on HP length.
Objectives: We hypothesized that PB with shorter periods of HP is associated with increased MSNA and decreased heart rate variability.
Methods: 10 healthy participants underwent microelectrode recordings of MSNA from the common peroneal nerve along with non-invasive recording of HRV, blood pressure and respiration. Following a 10-min period of tidal breathing, participants were asked to simulate PB for 3 min following a computed respiratory waveform that emulated two PB patterns, comprising a constant CA of 20 s duration and HP of two different lengths: short (20 s) vs long (40 s). Results: Compared to (3 min of) normal breathing, simulated PB with short HP resulted in a marked increase in mean and maximum MSNA amplitude (from 3.2 ± 0.8 to 3.4 ± 0.8 µV, p = 0.04; from 3.8 ± 0.9 to 4.3 ± 1.1 µV, p = 0.04, respectively). This was paralleled by an increase in LF/HF ratio of heart rate variability (from 0.9 ± 0.5 to 2.0 ± 1.3; p = 0.04). In contrast, MSNA response to simulated PB with long HP did not change as compared to normal breathing. Single CA events consistently resulted in markedly increased MSNA (all p < 0.01) when compared to the preceding HPs, while periods of HP, regardless of duration, decreased MSNA (p < 0.05) when compared to normal breathing.
Conclusion: Overall, the net effects of PB in healthy subjects over time on MSNA are dependent on the relative duration of HP: increased sympathetic outflow is seen during PB with a short but not with a long period of HP.
Periodic breathing (PB) is a cyclical pattern of ventilation characterised by alternating phases of hyperventilation (hyperpnea, HP) and transient cessation of respiratory effort and breathing, i. e. central apnea (CA) (Somers et al., 1989a; Hall et al., 1996; Solin et al., 2003; Giannoni et al., 2016; Giannoni et al., 2019a). Beyond occurring at high altitude, PB is frequently found in patients with systolic heart failure (Heart failure with reduced ejection fraction; HFrEF), where phases of hyperventilation alternate with CA in a crescendo-decrescendo pattern that is known as Hunter-Cheyne-Stokes respiration (Somers et al., 1989a; Hall et al., 1996; Solin et al., 2003; Giannoni et al., 2016; Giannoni et al., 2019a).
Apneic events are known to increase sympathetic outflow as measured by intraneural recording of muscle sympathetic nerve activity (MSNA), reflecting chemoreflex stimulation by hypercapnia and hypoxia and (possibly) loss of ventilation-related vagal inputs (Solin et al., 2003; Giannoni et al., 2016; Giannoni et al., 2019a). Chemoreflex hypersensitivity is often observed and usually considered the main mechanism behind Cheyne-Stokes respiration (Somers et al., 1989a; Somers et al., 1989b; Hall et al., 1996; Wedewardt et al., 2010; Seitz et al., 2013) On the contrary, HP is usually accompanied by a decrease in sympathetic outflow, at least in healthy individuals (Oldenburg et al., 2015).
Obstructive apneas have been consistently shown to increase sympathetic outflow, assessed by means of plasma catecholamines, heart rate variability, and MSNA (Fatouleh et al., 2014). In obstructive sleep apnea periodicity is less evident and the HP usually shorter than in PB or Cheyne-Stokes respiration, also due to anatomical factors preventing the reopening of upper airways (Fatouleh et al., 2014; Butler et al., 2019; Hietakoste et al., 2020). On the other hand, the apnea length is more variable and may reflect individual differences in anatomical factors, chemoreflex sensitivity (low or high) or arousability (low or high) (Fatouleh et al., 2014; Butler et al., 2019; Hietakoste et al., 2020). While shorter apneas have been associated with increased mortality (Butler et al., 2019), longer ones have been associated with higher ultra-short term HRV (Hietakoste et al., 2020).
In patients with HFrEF and Cheyne-Stokes respiration apnea duration is usually less heterogenous, while HP length may vary, and this has been mainly attributed to differences in cardiac output and circulation time (Hall et al., 1996; Giannoni et al., 2019b; Gentile et al., 2022). Regarding the impact of CA on sympathetic outflow in the context of CSR the current evidence is contradictory (Lorenzi-Filho et al., 1999; Fatouleh et al., 2014; Oldenburg et al., 2015; Giannoni et al., 2019b). While some authors found CA to increase sympathetic outflow, others have attributed sympathetic outflow to depend solely on background hemodynamics and hence HFrEF severity (Burnum et al., 1954; Somers et al., 1989a; Maze et al., 1989; Hall et al., 1996; Solin et al., 2003; Oldenburg et al., 2007; Bitter et al., 2009; Wedewardt et al., 2010; Yumino et al., 2013; Cowie et al., 2015; Magalang and Pack, 2015; Cagnoni et al., 2016; Eulenburg et al., 2016; Emdin et al., 2017; Perger et al., 2017). Intermittent HP is an integral part of Hunter-Cheyne-Stokes respiration and may counterbalance the effects of CA on sympathetic outflow, possibly by ensuing hypocapnia-hyperoxia, but also by increasing cardiac output (Somers et al., 1989a; Somers et al., 1989b; Hall et al., 1996; Wedewardt et al., 2010; Seitz et al., 2013; Oldenburg et al., 2015). Thus, the overall impact of PB on sympathetic outflow, a major driver of HFrEF progression, may depend on the net effect of CA and HP and particularly by the relative duration of HP within the PB cycle.
This is of major clinical interest as two large multicentric studies using either continuous positive airway pressure or assisted servo-ventilation to treat CA in HFrEF showed neutral or even detrimental impact on survival (Bradley et al., 2005; Cowie et al., 2015; Eulenburg et al., 2016) In patients with HFrEF and Hunter-Cheyne-Stokes respiration apnea duration is usually less heterogenous, while HP length may vary, and this has been mainly attributed to differences in cardiac output and circulation time (Hall et al., 1996; Giannoni et al., 2019b; Gentile et al., 2022). Therefore, to investigate whether the duration of HP may specifically impact on sympathetic outflow, we instructed a group of healthy subjects to simulate two different oscillatory patterns of PB while measuring sympathetic outflow directly by means of intraneural MSNA recordings (Velez-Roa et al., 2004; Macefield, 2012; Macefield and Wallin, 2018; Macefield and Henderson, 2019) and indirectly via non-invasive assessment of heart rate variability (Oldenburg et al., 2015).
Ten healthy participants (36 ± 9 [standard deviation] years, 5 male, 5 female, BMI 25 ± 2 kg/m2) were recruited. The study protocol conformed with the 1975 Declaration of Helsinki, other than registration in a database, and was approved by the institution’s Human Research Ethics Committee. All participants provided informed written consent to the studies, which were conducted in the Human Autonomic Neurophysiology laboratory at The Baker Heart and Diabetes Institute.
All participants underwent a thorough physical examination and evaluation of their past medical history to ensure the absence of previous diseases potentially affecting MSNA (Gratze et al., 1998; Maceira et al., 2006; Ponikowski et al., 2016).
Participants lay semi-recumbent in a chair with their back at 45o and their legs supported horizontally. Electrocardiographic activity was recorded with Ag-AgCl surface electrodes (BioAmp, PowerLab, ADInstruments, Sydney, Australia) on the chest and sampled at 2 kHz (bandpass 0.3 Hz–1 kHz). Continuous blood pressure was recorded non-invasively by finger pulse plethysmography, sampled at 400 Hz (DC-200 Hz), and calibrated with an integrated sphygmomanometer cuff on the opposite upper arm (NOVA; Finapres Medical System BV, Amsterdam, Netherlands). Respiration was sampled at 100 Hz (DC-100 Hz) using a respiratory belt transducer (ADInstruments). All physiological signals were stored on computer via a data acquisition and analysis system (PowerLab 16SP™ hardware device and LabChart™ for Macintosh, v7.1.2.5 software; ADInstruments).
For assessment of sympathovagal balance heart rate variability was analysed using a 3-lead electrocardiogram (sampling rate 200 Hz). HRV (expressed as ms2 based on continuously recorded variability in RR intervals) reflects sympathovagal balance and heart-brain interactions. Data were computed by frequency domain analysis and presented as the high frequency component (HF; 0.15–0.40 Hz), the low frequency component (LF; 0.04–0.15 Hz) and their relative ratio (LF/HF) (ESC Guidelines, 1996; Davies et al., 1999; Karemaker, 2017; Woehrle et al., 2017; Spiesshoefer et al., 2020; Spiesshoefer et al., 2021).
Figure 1 displays the methodology to obtain and analyse MSNA measurements. Participants lay semi-recumbent in a chair with their back at 45o and their legs supported horizontally. Electrical pulses (2–10 mA; Stimulus Isolator, ADInstruments, Sydney, Australia) were delivered via a 2 mm surface probe to locate the course of the right common peroneal nerve at the level of the fibular head. After identifying the optimal stimulation site, a sterile insulated tungsten microelectrode (Frederick Haer and Co., Bowdoin, United States) was inserted into the skin; an electrode with a larger uninsulated tip inserted at 1 cm distance served as the reference electrode. Weak electrical stimulation delivered through the active microelectrode (0.2 ms, 1 Hz, 0.01–1.0 mA) was used to guide manipulation of its tip into the nerve; evoked muscle twitches at 0.01–0.02 mA, without radiating paraesthesia, indicated that the tip had penetrated a muscle fascicle of the nerve.
FIGURE 1. Multi-unit recording of muscle SNA from a healthy participant via a tungsten microelectrode inserted percutaneously into a muscle fascicle of the peroneal nerve, shown schematically on the left. Muscle SNA occurs as bursts with a clear cardiac rhythmicity. Heart rate and diastolic blood pressure variability can be derived from the setup used through ECG and continuous (finger) blood pressure all being recorded simultaneously.
Neural activity was amplified (gain 2 × 104, bandpass 0.3–5.0 kHz) via an isolated amplifier and headstage (NeuroAmpEX, ADInstruments) and stored on computer (10 kHz sampling). Spontaneous or stretch-evoked activity of muscle spindle afferents, without afferent activity produced by light stroking of the leg or foot, confirmed the identity of the muscle fascicle of the nerve. The microelectrode was further manipulated until spontaneous bursts of muscle sympathetic nerve activity (MSNA) with clear cardiac rhythmicity, increasing during a maximal inspiratory apnoea, were observed.
A mean voltage neurogram of the filtered nerve signal was displayed from the root-mean square processed signal (200 ms moving average).
MSNA was analysed from the root mean square-processed nerve signal using the “Cyclic Measurements” and “Peak Parameters” features of the LabChart™ software: burst frequency (bursts per minute), burst incidence (bursts per 100 heart beats), burst width, burst area and time to peak (Figure 1) (Bradley et al., 2005; Macefield, 2012; Macefield and Henderson, 2019). In addition, amplitude distribution was determined: burst amplitudes were normalized to the highest burst amplitude seen during 1 minute of normal breathing (Bradley et al., 2005; Macefield, 2012; Macefield and Henderson, 2019).
After recording MSNA and cardiorespiratory signals during 10 min of normal breathing, participants were asked to follow different patterns of respiration displayed on a computer monitor in front of them. Each pattern was composed of sinusoidal waveforms of either constant amplitude and frequency (control) or two different types of PB as described below. These ventilatory patterns were generated in the LabChart™ software and displayed in the computer monitor so that participants could follow them in real time. Simulation of short and long HP cycle length were randomized in order. The patterns are shown in Figure 1. During the experiments participants’ own respiratory excursions were displayed below the emulated patterns. The different ventilatory manoeuvres were separated by 3 min of spontaneous breathing until MSNA, heart rate and blood pressure had returned to baseline.
Subjects were instructed to alternate HPs with CAs, thus simulating the typical crescendo-decrescendo pattern of PB. PB was simulated with 2 different HP lengths (20 and 40 s) while keeping the CA phase constant at 20 s duration, as in spontaneous PB in HFrEF, where PB cycle length depends on variable duration of the HP phase rather than apnea length (Solin et al., 2003; Magalang and Pack, 2015; Cagnoni et al., 2016; Perger et al., 2017). In addition, the simulated breathing patterns were specifically designed in such a way that apneas always started at end-expiration (referred to as a “negative PB breathing pattern” below), subsequently causing lung volume to fall below functional residual capacity during the hyperventilation phase. Participants were trained to practice several PB cycles by means of visual feedback before actual recordings were started (Figure 2).
FIGURE 2. Impact of short simulated periodic breathing on muscle sympathetic nerve activity. Multi-unit recording of muscle SNA. RMS, root mean square, BP, blood pressure; Resp flow, respiratory flow.
Assuming a two-sided significance level of 0.05 (alpha) and 80% power (beta), a sample size of at least 10 patients was calculated for detection of a 10% change in MSNA burst rate and amplitude, respectively (mean values and standard deviations of MSNA burst rate and amplitudes for power calculations were derived from preliminary data). Secondary endpoints comprised the MSNA burst amplitude distribution (% amplitude as a ratio of the greatest MSNA burst seen in 1 min of normal breathing), burst width, integral, time to peak, time distance from the R wave and time distance among one another. Other secondary endpoints comprised systolic, diastolic and mean arterial blood pressure, heart rate and heart rate variability frequency domain metrics, and oxygen saturation.
All analyses were performed using Sigma Plot software (Version 13.0, Systat Software GmbH, Erkrath, Germany), PASS 14 Power Analysis and Sample Size Software 2015, respectively (NCSS, LLC. Kaysville, Utah, United States). Results were expressed as mean and standard deviation for continuous variables with a normal distribution, and median and interquartile range for continuous variables with a skewed distribution.
MSNA and cardiorespiratory parameters were compared using paired t-test or Wilcoxon Rank Sum Test, as appropriate. Before comparison, data were averaged across the 3-min intervals of the different PB (either short or long HP) manoeuvres, and the last 3 min of normal breathing that preceded the first respiratory task. When comparing the effects of CAs against HPs, data were again averaged including all phases of CAs, and all phases of HPs during the simulation task. Heart rate variability-derived metrics were also compared with MSNA-derived metrics employing Pearson correlation and Bland-Altman graphs. For all tests, a p-value ≤0.05 was considered statistically significant.
The main results are shown in Figures 2–4 as graphical summaries.
All participants were normotensive, in sinus rhythm and without ectopic beats during all the recordings. No subject was taking any kind of medication possibly influencing sympathetic drive.
In all participants and across all breathing manoeuvres a total of 1570 MSNA bursts were analysed. MSNA data, as well as heart rate variability-derived metrics, are shown in Table 1. Notably, during normal breathing, no correlations were found between spectral indices of heart rate variability and any of the MSNA-derived metrics.
TABLE 1. Comparison of sympathetic outflow, hemodynamic and respiratory data between normal breathing and periodic breathing with a short hyperpnea length.
Table 1 and Figure 2 provides an analysis of sympathetic outflow during simulation of PB with a short (20 s) HP length. An increase in both the mean and the highest burst amplitude was observed between the phases of normal breathing and simulated PB.
Other than this no differences were found in other MSNA parameters between normal breathing and simulated PB with short HP length, including burst frequency, incidence, width, integral, time to peak, or variability.
Despite the increase in respiratory rate and depth, there was no effect on blood pressure. On the contrary, an increase in heart rate, HF, LF, and LF/HF ratio was observed during PB with a short HP length. Likewise, respiratory rate, and oxygen saturation increased significantly during simulated PB (p < 0.01).
Table 2 and Figure 3 provides an analysis of sympathetic outflow response to simulation of PB with a long (40 s) HP length. Simulated PB with a long HP length decreased mean burst area as well as its standard deviation but otherwise had no effects on other MSNA or heart rate variability parameters. Heart rate and HF component of heart rate variability, respiratory rate and oxygen saturation increased significantly, while there was no change in blood pressure when comparing normal breathing to PB with long HP length.
TABLE 2. Comparison of sympathetic outflow, hemodynamic and respiratory data between normal breathing and periodic breathing with a long hyperpnea length.
FIGURE 3. Impact of long simulated periodic breathing on muscle sympathetic nerve activity. Multi-unit recording of muscle SNA. RMS, root mean square, BP, blood pressure; Resp flow, respiratory flow.
Table 3 and Figure 4 provides an analysis of MSNA changes between CAs and HPs during simulated PB. MSNA increased significantly during the apneic phase irrespective of HP length: bursts during apneas had a higher frequency and incidence and an increased burst area compared to the preceding hyperpneas.
TABLE 3. Comparison of sympathetic outflow and hemodynamic data between central apneas and hyperpnea phases during periodic breathing with a short and a long hyperpnea length.
FIGURE 4. Impact of apneas compared to hyperpneas on muscle sympathetic nerve activity. Multi-unit recording of muscle SNA. RMS, root mean square, BP, blood pressure; Resp flow, respiratory flow.
This is the first study to determine the impact of simulated PB of different hyperpnea lengths as well as the immediate effects of CAs on sympathetic outflow in healthy subjects using MSNA, the gold standard technique for sympathetic outflow assessment. We demonstrated that end-expiratory CAs are associated with increased sympathetic outflow compared to HP, and that shorter PB cycles, in contrast to longer ones, are associated with an increase of sympathetic outflow, too. This seems to involve mainly the mean and the highest burst amplitude, at least in healthy individuals. Thus, the net effect of PB, measured over the entire respiratory cycle, derives from the equilibrium between the excitatory drive provided by CAs and the inhibitory effect provided by HPs: when the HP duration is short the relative balance of excitation vs. inhibition shift towards the first one.
This is of importance as PB is a highly prevalent phenomenon with a presumed incremental effect on sympathetic drive in HFrEF, as well as in conditions in the absence of HFrEF, with detrimental effects if left untreated (Burnum et al., 1954; Maze et al., 1989; Yumino et al., 2013; Magalang and Pack, 2015; Eulenburg et al., 2016; Perger et al., 2017). However, a previous study combining polysomnography and right heart catheterisation showed that the increase of sympathetic drive was related to the severity of HFrEF rather than to that of Cheyne-Stokes respiration (as reflected by the apnea-hypopnea index) (Mansfield et al., 2003a; Berry RB Gamaldo et al., 2012). Moreover, the unexpected results of the SERVE-HF trial suggested that treatment of CAs with adaptive servo-ventilation is associated with increased rather than decreased mortality in HFrEF patients with CAs, especially in those with severe HFrEF, namely with left ventricular ejection fraction lower than 30% (Cowie et al., 2015; Woehrle et al., 2017). This group of patients is likely to have also a decreased cardiac output and a longer circulatory time.
This has given rise to the hypothesis that CAs and PB potentially exert compensatory rather than detrimental effects in patients with HFrEF. Indeed, during hyperventilation, venous return and thus cardiac output are increased, and sympathetic drive is known to be inhibited by phasic (but not static) changes in lung volume and by hypocapnia (Burnum et al., 1954; Somers et al., 1989b; Hall et al., 1996; Wedewardt et al., 2010; Seitz et al., 2013; Oldenburg et al., 2015). Conversely, apneas are presumed to lead to increased sympathetic drive mainly in response to apnea-related hypoxemia and hypercapnia (Solin et al., 2003; Giannoni et al., 2016), but also to a lack of inhibitory drive from the stretch receptors located in the airways, lungs and chest wall.
In HFrEF, prolonged circulatory time per se prolongs both CA and HP duration (Macefield, 2012). Notably, patients with HFrEF and PB/Cheyne-Stokes respiration show longer HP and cycle length but similar apnea duration when compared with patients with HFrEF and obstructive sleep apnea (Macefield and Henderson, 2019), who usually have a less compromised heamodynamic profile (Hall et al., 1996; Wedewardt et al., 2010; Perger et al., 2017).
The present work suggests that in PB, HP duration may contribute to the net effect of sympathetic outflow.
Simulation of PB (in contrast to assessing its effects when it occurs spontaneously) has the advantage that the length and composition of PB cycles can be standardized, differently from the rather erratic and variable composition observed during spontaneous PB (central and obstructive apneas, mixed apneas, and variable cycle length). Thus, it is possible to precisely predefine the duration of the hyperventilation phase, while keeping CA constant in length. Second, the net effects of PB could be studied having phases of normal breathing as a reliable comparator. Our findings therefore, based on this approach, confirm that CA likely impacts negatively while HP likely impacts positively on sympathetic outflow. It is therefore, that a long cycle length of PB where CAs can be found embedded in long HP impacts neutral on sympathetic outflow, whereas this all results in increased sympathetic outflow should the same CAs be embedded into shorter HP. Furthermore, the present study also directly proved that CAs per se increase sympathetic outflow when compared directly against HP.
Indeed, CA may cause an increase in sympathetic outflow by several mechanisms: by deactivating pulmonary stretch receptors and stimulating peripheral and central chemoreceptors by hypoxia and hypercapnia (Floras, 2009). Furthermore, cortical activation following apneas may further rise central sympathetic outflow plus vagal withdrawal (Floras, 2009). The pathophysiological concept of CAs further increasing SNA is further supported by findings showing that patients with CAs are characterized by increased SNA as reflected by increased HR (Szollosi et al., 2007), decreased heart rate and blood pressure variability (Szollosi et al., 2007), enhanced serum and urinary catecholamine levels (Solin et al., 2003), as well as with increased MSNA (Solin et al., 2003) compared to patients without CAs. This theory is also further supported by studies linking the presence of CAs to worse prognosis in HFrEF with the presumed mechanism being increased SNA through CAs causing arrhythmias and HFrEF related death (Poletti et al., 2009). This has been shown for daytime CA and in HFrEF in particular (Emdin et al., 2017). Another exploratory approach to this key pathophysiological issue is to evaluate the effects of CA treatment on SNA. Indeed, reducing CA through administration of oxygen is also associated with SNA decrease (Staniforth et al., 1998). As for mask-based therapies based on positive pressure ventilation, despite their positive effect on CA in HF, there have been conflicting evidences on their impact on SNA too. While it was shown that cPAP and ASV lead to decreases in SNA as reflected by increased heart rate and blood pressure variability (Iwaya et al., 2014), decreased serum and urinary catecholamine levels (Hetzenecker et al., 2016; Toyama et al., 2017), as well as decreased MSNA (Koyama et al., 2010) other authors as well as a large scale RCT challenged these hypotheses (Cowie et al., 2015; Woehrle et al., 2017).
Nonetheless, the real impact of hyperpnea length may vary in patients with HFrEF, in whom several different pathophysiological mechanisms (chemoreflex gain, plant gain, circulation time, other sources of sympathetic outflow related to HFrEF itself or other altered circuits) may drive to different results. Therefore, an effort to study MSNA and other sympathetic outflow metrics should be attempted also in this relevant clinical setting before exporting our results to improve patients selection and referral to specific PB treatment. Ultimately, it should be noted that also conflicting evidence by other authors has been provided showing that CAs per se may not always result in increased sympathetic outflow (Mansfield et al., 2003b; Grimm et al., 2015).
Our study has several limitations. MSNA was obtained in 10 healthy participants with no cardiovascular or respiratory disease. Nevertheless, despite it being an invasive technique, one of the advantages of MSNA is undoubtedly its stability over time, requiring smaller sample size (Macefield, 2012; Macefield and Wallin, 2018; Macefield and Henderson, 2019) especially when studying the same participants in different experimental conditions.
Secondly, in our study PB was simulated. As noted above, PB cycle length is rather irregular in various pathologies and might therefore have a different impact on sympathetic outflow. Similarly, cortical influences secondary to simulation cannot be excluded. Studies focusing on spontaneous PB are therefore needed for more definite conclusions.
Third, the duration of each ventilatory manoeuvre was limited to 3 min. Therefore, we cannot exclude the possibility that longer periods of PB may exert different effects on the variables evaluated in this study, and that a drift in the average value of each haemodynamic measure may occur. Longer recordings are needed to clarify this point.
Fourth, considering that increasing the respiratory rate, the HF component may have been moved towards higher frequencies (>0.40 Hz underestimating HF component, by using the same frequency for everybody). Therefore a potential future study might use a wavelet analysis or a Wigner-Ville that may correct for non-stationarity of the signals (considering the respiratory maneuvers performed).
Apneas per se consistently resulted in markedly increased sympathetic outflow compared to phases of hyperventilation. However, at least in healthy subject simulating PB, the overall net effects of PB on on sympathetic outflow depend on hyperpnea length, with shorter duration of the hyperpneic phase resulting in increased sympathoexcitation. Future research should focus on the differential impact of longer recordings and focusing on patients with spontaneous PB during either wakefulness or sleep, considering at the same time the impact of several covariates including background haemodynamics, pulmonary mechanics, and the degree of feedback resetting.
The original contributions presented in the study are included in the article/Supplementary Material, further inquiries can be directed to the corresponding author.
The study protocol has been approved by the Human Research Ethics Committee of the Alfred Hospital and Governance of the Baker Heart and Diabetes Institute. The participants provided their written informed consent to participate in this study.
JS, AG and MB designed the study. JS, TD and VM were responsible for data collection. IH and JS performed the statistical analyses, and JS, AG and VM prepared the manuscript which was critically revised and amended by all other authors.
This work was supported by a travel grant awarded by the German Heart Foundation (Deutsche Herzstiftung e.V.). Mit Fördermitteln der Deutschen Herzstiftung e.V”. The funders had no role in study design, data collection and analysis, preparation of the manuscript, or the submission process. This study was supported in part by the the Tuscany Region through the Tuscany Network for Bioelectronic Approaches in Medicine: AI-based predictive algorithms for fine-tuning of electroceutical treatments in neurological, cardiovascular and endocrinological diseases (TUNE-BEAM, H14I20000300002).
We gratefully acknowledge Gerold Kiersteins, Kevin Evans and Stefan Bornscheins (all AD Instruments, Oxford, United Kingdom) kind technical help in collecting, analysing and interpreting our data. Language editing assistance was provided by Nicola Ryan, independent medical writer.
The authors declare that the research was conducted in the absence of any commercial or financial relationships that could be construed as a potential conflict of interest.
All claims expressed in this article are solely those of the authors and do not necessarily represent those of their affiliated organizations, or those of the publisher, the editors and the reviewers. Any product that may be evaluated in this article, or claim that may be made by its manufacturer, is not guaranteed or endorsed by the publisher.
Berry Rb Gamaldo C. E., Harding S. M., Lloyd R. M., Marcus C. L.Vaughn BV for the American Academy of Sleep Medicine BR (2012). The AASM manual for the scoring of sleep and associated events. J Clin Sleep Med 8, 597–619.
Bitter T., Faber L., Hering D., Langer C., Horstkotte D., Oldenburg O. (2009). Sleep-disordered breathing in heart failure with normal left ventricular ejection fraction. Eur. J. Heart Fail. 11, 602–608. doi:10.1093/eurjhf/hfp057
Bradley T. D., Logan A. G., Kimoff R. J., Series F., Morrison D., Ferguson K., et al. (2005). CANPAP InvestigatorsContinuous positive airway pressure for central sleep apnea and heart failure. N. Engl. J. Med. 353, 2025–2033. doi:10.1056/NEJMoa051001
Burnum J. F., Hickam J. B., McIntosh H. D. (1954). The effect of hypocapnia on arterial blood pressure. Circulation 9, 89–95. doi:10.1161/01.cir.9.1.89
Butler M. P., Emch J. T., Rueschman M., Sands S. A., Shea S. A., Wellman A., et al. (2019). Apnea-hypopnea event duration predicts mortality in men and women in the sleep heart health study. Am. J. Respir. Crit. Care Med. 199, 903–912. doi:10.1164/rccm.201804-0758OC
Cagnoni F., Destro M., Bontempelli E., Locatelli G., Gering D., Schlaich M. (2016). Central sympathetic inhibition: a neglegted approach for treatment of cardiac arrhythmias. Curr. Hypertens. Rep. 18, 13. doi:10.1007/s11906-015-0619-0
Cowie M. R., Woehrle H., Wegscheider K., Angermann C., d'Ortho M. P., Erdmann E., et al. (2015). Adaptive servo-ventilation for central sleep apnea in systolic heart failure. N. Engl. J. Med. 373, 1095–1105. doi:10.1056/NEJMoa1506459
Davies L. C., Francis D., Jurák P., Kara T., PiepoliM., , Coats A. J. (1999). Reproducibility of methods for assessing baroreflex sensitivity in normal controls and in patients with chronic heart failure. Clin. Sci. (Lond). 97, 515–522. doi:10.1042/cs19990135
Emdin M., Mirizzi G., Giannoni A., Poletti R., Iudice G., Bramanti F., et al. (2017). Prognostic significance of central apneas throughout a 24-hour period in patients with heart failure. J. Am. Coll. Cardiol. 70, 1351–1364. doi:10.1016/j.jacc.2017.07.740
Eulenburg C., Wegscheider K., Woehrle H., Angermann C., d'Ortho M. P., Erdmann E., et al. (2016). Mechanisms underlying increased mortality risk in patients with heart failure and reduced ejection fraction randomly assigned to adaptive servoventilation in the SERVE-HF study: Results of a secondary multistate modelling analysis. Lancet. Respir. Med. 4, 873–881. doi:10.1016/S2213-2600(16)30244-2
Fatouleh R., Mckenzie D. K., Macefield V. G. (2014). Respiratory modulation of muscle sympathetic nerve activity in obstructive sleep apnoea. Exp. Physiol. 99 (10), 1288–1298. PMID: 24887112. doi:10.1113/expphysiol.2013.077511
Floras J. S. (2009). Sympathetic nervous system Activation in human heart failure. Clinical implications of an updated model. J. Am. Coll. Cardiol. 54, 375–385. doi:10.1016/j.jacc.2009.03.061
Gentile F., Borrelli C., Sciarrone P., Buoncristiani F., Spiesshoefer J., Bramanti F., et al. (2022). Central apneas are more detrimental in female than in male patients with heart failure. J. Am. Heart Assoc. 11, e024103. doi:10.1161/JAHA.121.024103
Giannoni A., Gentile F., Navari A., Borrelli C., Mirizzi G., Catapano G., et al. (2019). Contribution of the lung to the genesis of Cheyne-Stokes respiration in heart failure: Plant gain beyond chemoreflex gain and circulation time. J. Am. Heart Assoc. 8, e012419. doi:10.1161/JAHA.119.012419
Giannoni A., Raglianti V., Mirizzi G., Taddei C., Del Franco A., Iudice G., et al. (2016). Influence of central apneas and chemo reflex activation on pulmonary artery pressure in chronic heart failure. Int. J. Cardiol. 202, 200–206. doi:10.1016/j.ijcard.2015.09.007
Giannoni A., Raglianti V., Taddei C., Borrelli C., Chubuchny V., Vergaro G., et al. (2019). Cheyne-Stokes respiration related oscillations in cardiopulmonary hemodynamics in patients with heart failure. Int. J. Cardiol. 289, 76–82. doi:10.1016/j.ijcard.2019.03.033
Gratze G., Fortin J., Holler A., Pfurtscheller G., Wach P. (1998). A software package for non-invasive, real-time beat-to-beat monitoring of stroke volume, blood pressure, total peripheral resistance and for assessment of autonomic function. Comput. Biol. Med. 28, 121–142. doi:10.1016/s0010-4825(98)00005-5
Grimm W., Sosnovskaya A., Timmesfeld N., Hildebrandt O., Koehler U. (2015). Prognostic impact of central sleep apnea in patients with heart failure. J. Card. Fail. 21, 126–133. doi:10.1016/j.cardfail.2014.10.017
Hall M. J., Xie A., Rutherford R., Ando S., Floras J. S., Bradley T. D. (1996). Cycle length of periodic breathing in patients with and without heart failure. Am. J. Respir. Crit. Care Med. 154, 376–381. doi:10.1164/ajrccm.154.2.8756809
Hetzenecker A., Roth T., Birner C., Maier L., Pfeifer M., Arzt M. (2016). Adaptive servo-ventilation therapy of central sleep apnoea and its effect on sleep quality. Clin. Res. Cardiol. 105, 189–195. doi:10.1007/s00392-015-0904-6
Hietakoste S., Korkalainen H., Kainulainen S., Sillanmaki S., Nikkonen S., Myllymaa S., et al. (2020). Longer apneas and hypopneas are associated with greater ultra-short-term HRV in obstructive sleep apnea. Sci. Rep. 10, 21556. doi:10.1038/s41598-020-77780-x
Iwaya S., Yoshihisa A., Nodera M., Owada T., Yamada S., Sato T., et al. (2014). Suppressive effects of adaptive servo-ventilation on ventricular premature complexes with attenuation of sympathetic nervous activity in heart failure patients with sleep-disordered breathing. Heart Vessels 29, 470–477. doi:10.1007/s00380-013-0394-2
Karemaker J. M. (2017). An introduction into autonomic nervous function. Physiol. Meas. 38, R89–R118. doi:10.1088/1361-6579/aa6782
Koyama T., Watanabe H., Yusuke K., Makabe S., Munehisa Y., Iino K., et al. (2010). Beneficial effects of adaptive servo ventilation in patients with chronic heart failure. Circ. J. 74, 2118–2124. doi:10.1253/circj.cj-10-0082
Lorenzi-Filho G., Dajani H. R., Leung R. S., Floras J. S., Bradley T. D. (1999). Entrainment of blood pressure and heart rate oscillations by periodic breathing. Am. J. Respir. Crit. Care Med. 159, 1147–1154. doi:10.1164/ajrccm.159.4.9806081
Macefield V. G. (2012). Firing patterns of muscle vasoconstrictor neurons in respiratory disease. Front. Physiology 3, 153. PMID: 22654767. doi:10.3389/fphys.2012.00153
Macefield V. G., Henderson L. A. (2019). Identification of the human sympathetic connectome involved in blood pressure regulation. NeuroImage 202, 116119. PMID: 31446130. doi:10.1016/j.neuroimage.2019.116119
Macefield V. G., Wallin B. G. (2018). Physiological and pathophysiological firing properties of single postganglionic sympathetic neurons in humans. J. Neurophysiol. 119, 944–956. PMID: 29142091. doi:10.1152/jn.00004.2017
Maceira A. M., Prasad S. K., Khan M., Pennell D. J. (2006). Reference right ventricular systolic and diastolic function normalized to age, gender and body surface area from steady-state free precession cardiovascular magnetic resonance. Eur. Heart J. 27, 2879–2888. doi:10.1093/eurheartj/ehl336
Magalang U. J., Pack A. I. (2015). Heart failure and sleep-disordered breathing—the Plot thickens. N. Engl. J. Med. 373, 1166–1167. doi:10.1056/NEJMe1510397
Mansfield D., Kaye D., La R., Solin P., Esler M. D., Naughton M. T. (2003). Raised sympathetic nerve activity in heart failure and central sleep apnea is due to heart failure severity. Circulation 107, 1396–1400. doi:10.1161/01.cir.0000056520.17353.4f
Mansfield D., Kaye D. M., La Rocca H. B., Solin P., Esler M. D., Naughton M. T. (2003). Raised sympathetic nerve activity in heart failure and central sleep apnea is due to heart failure severity. Circulation 107, 1396–1400. doi:10.1161/01.cir.0000056520.17353.4f
Maze S. S., Kotler M. N., Parry W. R. (1989). Doppler evaluation of changing cardiac dynamics during Cheyne-Stokes respiration. Chest 95, 525–529. doi:10.1378/chest.95.3.525
Oldenburg O., Lamp B., Faber L., Teschler H., Horstkotte D., Topfer V. (2007). Sleep-disordered breathing in patients with symptomatic heart failure. A contemporary study of prevalence in and characteristics of 700 patients. Eur. J. Heart Fail. 9, 251–257. doi:10.1016/j.ejheart.2006.08.003
Oldenburg O., Spießhöfer J., Fox H., Bitter T., Horstkotte D. (2015). Cheyne-Stokes respiration in heart failure: friend or foe? Hemodynamic effects of hyperventilation in heart failure patients and healthy volunteers. Clin. Res. Cardiol. 104, 328–333. doi:10.1007/s00392-014-0784-1
Perger E., Inami T., Lyons O. D., Alshaer H., Smith S., Floras J. S., et al. (2017). Distinct patterns of hyperpnea during cheyne-stokes respiration: Implication for cardiac function in patients with heart failure. J. Clin. Sleep. Med. 13, 1235–1241. doi:10.5664/jcsm.6788
Poletti R., Passino C., Giannoni A., Zyw L., Prontera C., Bramanti F., et al. (2009). Risk factors and prognostic value of daytime Cheyne – Stokes respiration in chronic heart failure patients. Int. J. Cardiol. 137, 47–53. doi:10.1016/j.ijcard.2008.06.028
Ponikowski P., Voors A. A., Anker S. D., Bueno H., Cleland J. G. F., Coats A. J. S., et al. (2016). 2016 ESC Guidelines for the diagnosis and treatment of acute and chronic heart failure: The Task Force for the diagnosis and treatment of acute and chronic heart failure of the European Society of Cardiology (ESC)Developed with the special contribution of the Heart Failure Association (HFA) of the ESC. Eur. Heart J. 37, 2129–2200. doi:10.1093/eurheartj/ehw128
Seitz M. J., Brown R., Macefield V. G. (2013). Inhibition of augmented muscle vasoconstrictor drive following asphyxic apnoea in awake human subjects is not affected by relief of chemical drive. Exp. Physiol. 98, 405–414. doi:10.1113/expphysiol.2012.067421
Solin P., Kaye D. M., Little P. J., Bergin P., Richardson M., Naughton M. T. (2003). Impact of sleep apnea on sympathetic nervous system activity in heart failure. Chest 123, 1119–1126. doi:10.1378/chest.123.4.1119
Somers V. K., Mark A. L., Zavala D. C., Aboud F. M. (1989). Contrasting effects of hypoxia and hypercapnia on ventilation and sympathetic activity in humans. J. Appl. Physiol. 67, 2101–2106. doi:10.1152/jappl.1989.67.5.2101
Somers V. K., Mark A. L., Zavala D. C., Aboud F. M. (1989). Influence of ventilation and hypocapnia on sympathetic nerve responses to hypoxia in normal humans. J. Appl. Physiol. 67, 2095–2100. doi:10.1152/jappl.1989.67.5.2095
Spiesshoefer J., Bannwitz B., Mohr M., Simon H., Winfried R., Paolo S., et al. (2020). Effects of nasal high flow on sympathovagal balance, sleep, and sleep-related breathing in patients with precapillary pulmonary hypertension. Sleep. Breath. 25, 705–717. PMID: 32827122. doi:10.1007/s11325-020-02159-1
Spiesshoefer J., Hegerfeld N., Gerdes M. F., Sören K., Martha G., Robert R., et al. (2021). Effects of central apneas on sympathovagal balance and hemodynamics at night: impact of underlying systolic heart failure. Sleep. Breath. 25 (2), 965–977. PMID: 32700287. doi:10.1007/s11325-020-02144-8
Staniforth A. D., Kinnear W. J. M., Starling R., Hetmanski D. J., Cowley A. J. (1998). Effect of oxygen on sleep quality, cognitive function and sympathetic activity in patients with chronic heart failure and Cheyne-Stokes respiration. Eur. Heart J. 19, 922–928. doi:10.1053/euhj.1997.0861
Szollosi I., Krum H., Kaye D., Naughton M. T. (2007). Sleep apnea in heart failure increases heart rate variability and sympathetic dominance. Sleep 30, 1509–1514. doi:10.1093/sleep/30.11.1509
Toyama T., Hoshizaki H., Kasama S. (2017). Adaptive servo-ventilation therapy improves cardiac sympathetic nerve activity , cardiac function , exercise capacity , and symptom in patients with chronic heart failure and Cheyne- Stokes respiration. J. Nucl. Cardiol. 24 (6), 1926–1937. doi:10.1007/s12350-016-0529-9
Velez-Roa S., Ciarka A., Najem B., Vachiery J. L., Naeije R., van de Borne P. (2004). Increased sympathetic nerve activity in pulmonary artery hypertension. Circulation 110, 1308–1312. doi:10.1161/01.CIR.0000140724.90898.D3
Wedewardt J., Bitter T., Prinz C., Faber L., Horstkotte D., Oldenburg O. (2010). Cheyne-Stokes respiration in heart failure: Cycle length is dependent on left ventricular ejection fraction. Sleep. Med. 11, 137–142. doi:10.1016/j.sleep.2009.09.004
Woehrle H., Cowie M. R., Eulenburg C., Suling A., Angermann C., d'Ortho M. P., et al. (2017). Adaptive servo ventilation for central sleep apnoea in heart failure: SERVE-HF on-treatment analysis. Eur. Respir. J. 50, 1601692. doi:10.1183/13993003.01692-2016
Keywords: central sleep apnea, physiology, muscle sympathetic nerve activity, risk factor, hyperventilation
Citation: Spiesshoefer J, Giannoni A, Borrelli C, Sciarrone P, Husstedt I, Emdin M, Passino C, Kahles F, Dawood T, Regmi B, Naughton M, Dreher M, Boentert M and Macefield VG (2022) Effects of hyperventilation length on muscle sympathetic nerve activity in healthy humans simulating periodic breathing. Front. Physiol. 13:934372. doi: 10.3389/fphys.2022.934372
Received: 02 May 2022; Accepted: 10 August 2022;
Published: 05 September 2022.
Edited by:
Nick Spencer, Flinders University, AustraliaReviewed by:
Eugene Nalivaiko, The University of Newcastle, AustraliaCopyright © 2022 Spiesshoefer, Giannoni, Borrelli, Sciarrone, Husstedt, Emdin, Passino, Kahles, Dawood, Regmi, Naughton, Dreher, Boentert and Macefield. This is an open-access article distributed under the terms of the Creative Commons Attribution License (CC BY). The use, distribution or reproduction in other forums is permitted, provided the original author(s) and the copyright owner(s) are credited and that the original publication in this journal is cited, in accordance with accepted academic practice. No use, distribution or reproduction is permitted which does not comply with these terms.
*Correspondence: Jens Spiesshoefer, anNwaWVzc2hvZWZlckB1a2FhY2hlbi5kZQ==
†These authors have contributed equally to this work
Disclaimer: All claims expressed in this article are solely those of the authors and do not necessarily represent those of their affiliated organizations, or those of the publisher, the editors and the reviewers. Any product that may be evaluated in this article or claim that may be made by its manufacturer is not guaranteed or endorsed by the publisher.
Research integrity at Frontiers
Learn more about the work of our research integrity team to safeguard the quality of each article we publish.