- 1CRMBM, CNRS, Aix Marseille University, Marseille, France
- 2Laboratoire Interuniversitaire de Biologie de la Motricité, Université Savoie Mont Blanc, Chambéry, France
Hydroxyurea (HU) is a ribonucleotide reductase inhibitor most commonly used as a therapeutic agent in sickle cell disease (SCD) with the aim of reducing the risk of vaso-occlusion and improving oxygen transport to tissues. Previous studies suggest that HU may be even beneficial in mild anemia. However, the corresponding effects on skeletal muscle energetics and function have never been reported in such a mild anemia model. Seventeen mildly anemic HbAA Townes mice were subjected to a standardized rest-stimulation (transcutaneous stimulation)-protocol while muscle energetics using 31Phosphorus magnetic resonance spectroscopy and muscle force production were assessed and recorded. Eight mice were supplemented with hydroxyurea (HU) for 6 weeks while 9 were not (CON). HU mice displayed a higher specific total force production compared to the CON, with 501.35 ± 54.12 N/mm3 and 437.43 ± 57.10 N/mm3 respectively (+14.6%, p < 0.05). Neither the total rate of energy consumption nor the oxidative metabolic rate were significantly different between groups. The present results illustrated a positive effect of a HU chronic supplementation on skeletal muscle function in mice with mild anemia.
1 Introduction
Hydroxyurea, also known as hydroxycarbamide (HU) is a ribonucleotide reductase inhibitor, leading to a reduced availability of intracellular deoxynucleotide triphosphate which is needed for DNA synthesis. It reactivates γ-globin synthesis and consequently fetal hemoglobin (HbF) production (Cokic et al., 2003; Almeida et al., 2012).
HU is the most commonly used treatment in sickle cell disease (SCD) for decades (Platt et al., 1984; McGann and Ware, 2015). Indeed by inducing HbF production, HU contributes to reduce the abnormal sickle hemoglobin (HbS) proportion thereby alleviating symptoms of SCD patients i.e., anemia, vaso-occlusive crisis, acute chest syndrome and transfusion requirement (Charache et al., 1995). Interestingly, HU is also used in other types of anemias such as β-thalassemia major (Algiraigri et al., 2017, 2014) or E/β-thalassemia (Algiraigri and Kassam, 2017) in which anemia is expressed together with microcytosis (Rees et al., 1998; Muncie and Campbell, 2009). For these β-thalassemias, HU could also enhance HbF production thereby increasing total hemoglobin (Hb) level and reducing anemia (Fucharoen et al., 1996; Arruda et al., 1997; Singer et al., 2005). The corresponding improved effectiveness of erythropoiesis has been related to the decreased disease severity (Fucharoen et al., 1996; Singer et al., 2005; Algiraigri et al., 2017; Algiraigri and Kassam, 2017).
Severe anemia (<7 g/dl) is accompanied by multiple consequences of oxygen reduction. More particularly, anemia affects the oxygen-carrying capacity of blood with a recognized impact on oxygen supply and utilization by the active muscles which ultimately impairs exercise capacity (Woodson et al., 1978). In that respect, HU has been able to substantially enhance quality of life of patients (Fucharoen et al., 1996; Singer et al., 2005; Ballas et al., 2006; Algiraigri et al., 2017).
Interestingly, Algiraigri and colleagues reported that “the more severe the type of β-thalassemia, the milder the response to HU” (Algiraigri et al., 2014). From that point of view and considering 1) the relationship between oxygenation, muscle energetics and muscle function (including fatigue) (Woodson et al., 1978; Haseler et al., 1999), 2) the potential beneficial effects of HU on Hb level and local blood flow previously reported (Fucharoen et al., 1996; Cokic et al., 2003; Green and Barral, 2014), one could expect that a chronic HU supplementation could improve muscle energetics and function even in mild anemia. Interestingly, humanized hα/hα::βA/βA Townes mice exhibit a slightly lower Hb concentration compared to wild type mice (Wu et al., 2006) and thus constitutes an excellent model of mild anemia.
In the present study, we intended to assess the effects of a chronic HU supplementation on muscle energetics and function during a standardized rest-exercise-recovery protocol in a mild anemic murine model.
2 Methods
2.1 Animal Model, Care and Feeding
Seventeen humanized hα/hα::βA/βA Townes mice of the same litter/lineage and two (±0.5) months old have been used in the present study (Jackson Laboratories, Bar Harbor, United States). These mice are characterized by a lower Hb concentration (mild anemia) and a reduced MCV as compared to wild type counterparts (Wu et al., 2006). The study was performed in conformity with the French guidelines for animal care and in conformity with the European convention for the protection of vertebrate animals used for experimental purposes and institutional guidelines n° 86/609/CEE 24 November 1986. All animal experiments were approved by the Institutional Animal Care Committee of Aix-Marseille University (permit number #1841-2019011016466656). Mice were housed in a controlled-environment facility (12–12 h light–dark cycle, 22°C) and received water and standard food ad libitum. Eight out of seventeen mice were supplemented with HU for 6 weeks (HU). Hydroxyurea was given every day (50 mg/kg/day) (Lebensburger et al., 2010) in drinking water and the concentration was adjusted daily according to the water consumption, we ensured that mice never lacked water. Previous studies have reported a positive effect of a similar dose provided acutely or for a 6 week-period on hematological characteristics (Platt et al., 1984; Lebensburger et al., 2010). Control mice (CON, n = 9) received water only over the same period. No animals died during the experiments.
2.2 Study Design
All mice were investigated after the 6-week period. Mice were anesthetized and weighted then muscle energetics and function were assessed in HU and CON mice in response to a standardized Rest-Stimulation (1 Hz)-Recovery protocol. One week later, mice were anesthetized in an induction chamber with isoflurane and then euthanized by cervical dislocation. Gastrocnemius muscle was harvested and weighted.
2.3 Non-Invasive Investigation of Posterior Hindlimb Muscles Function and Bioenergetics
Investigations were conducted using an innovative homebuilt experimental setup which has been designed to be operational inside the PharmaScan® AVANCE™ III HD 70/16 US equipped with a 90 mm BGA09S (760 mT/m) gradient insert (Bruker BioSpin MRI Gmbh, Ettlinge, Germany). The setup allows 1) magnetic resonance imaging (MRI), 2) muscle force measurements and 3) 31P-magnetic resonance spectroscopy (31P-MRS) (Giannesini et al., 2010). MRI was used in order to get anatomical information about the hindlimb. The posterior hindlimb muscles mechanical performance was assessed with a dedicated homemade ergometer consisting of a foot pedal coupled to a force transducer (Giannesini et al., 2010). 31P-MRS was used to dynamically monitor the levels of high-energy phosphorylated compounds and acidosis. We have chosen to study posterior hindlimb muscles, which form the belly of the calf, because it is clearly distinct from the other muscles of the leg, easily accessible for MR coils and large enough to give 31P-MRS spectra with a good signal to noise ratio in a short time. It has been shown that the gastrocnemius muscle was preferentially activated using our experimental protocol (Giannesini et al., 2010).
2.4 Animal Preparation
Mice were anesthetized in an induction chamber (Equipement vétérinaire Minerve, Esternay, France) using an air flow (3 L/min) containing 4% isoflurane. The left hindlimb was shaved and electrode cream was applied at the knee and heel regions to optimize electrical contacts. The anesthetized animal was then placed supine in the experimental setup (Giannesini et al., 2010). Corneas were protected from drying by applying ophthalmic cream, and the animal’s head was placed in a facemask continuously supplied with 1-2% isoflurane mixed in 66% room air (1 L/min) and 33% O2 (0.5 L/min). Breathing rate was monitored during the protocol and kept between 100 and 130 breaths/min due to isoflurane concentration adjustment. The foot was positioned on the ergometer’s pedal and the hindlimb was centered inside a 16-mm-diameter 1H Helmholtz coil while the belly of the gastrocnemius muscle was located above an elliptic (8 × 6 mm2) 31P surface coil. Body temperature was controlled and maintained at a physiological level throughout the experiment using a feedback loop including an electrical heating blanket, a temperature control unit (ref. 507137, Harvard Apparatus, Holliston, MA, United States) and a homemade rectal thermometer.
2.5 Induction of Muscle Contraction and Contractile Force Measurement
Muscle contractions were induced by electrostimulation using two transcutaneous surface electrodes connected to a constant-current stimulator (DS7A, Digitimer, Herthfordshire, United Kingdom). One electrode was placed at the heel level and the other one was located just above the knee joint. Electrical signal coming out from the force transducer of the ergometer was amplified (Two operational amplifiers: AD620 and OP497, Analog Devices, Norwood, MA, United States, with a total dB gain: 70 dB at 0-5 kHz bandwidth) and continuously monitored and recorded on a personal computer using a Powerlab 16/36 data acquisition system (AD Instruments, Sydney, Australia) and a dedicated software (LabChart 8, AD Instruments, Sydney, Australia). The digital signal was converted to force according to a linear calibration curve and expressed in mN.
2.6 Moderate Exercise Protocol
Function and bioenergetics of the posterior hindlimb muscles were evaluated throughout a 6-min moderate exercise consisting of maximal isometric contractions repeated at a frequency of 1Hz, induced with square wave pulses (0.5 ms duration).
Force parameters were acquired with LabChart software (AD Instruments, Oxford, United Kingdom). Peak force (Pf, mN) was quantified. Total force production (TFP) was computed as the sum of each twitch. Specific values (Pf, TFP) were normalized to gastrocnemius muscle volume (cm3 or mm3) computed from anatomic hindlimb MR images.
2.7 Preliminary Adjustments
Before the onset of MR acquisition, the muscle was passively stretched at rest by adjusting the angle between the foot and the hindlimb to produce maximal twitch tension in response to supramaximal square waves pulses (0.5 ms duration). Individual maximal stimulation intensity was determined by a progressive increase of the stimulus intensity until there was no further force increase.
2.8 Multimodal MR Data Acquisition
Ten consecutive non-contiguous axial slices (1 mm thickness, spaced 0.25 mm) covering the region from the knee to the ankle were selected across the lower hindlimb. RARE images (Rare factor = 8, effective echo time = 35.29 ms, actual echo time = 11.76 ms, repetition time = 5,000 ms, one accumulation, 20 × 20 mm field of view, 256 × 192 matrix size, acquisition time = 3 min 14 s) were recorded at rest in order to assess muscle volume. Posterior hindlimb muscles region was manually delineated on each of the 6 largest slices. Then, muscle volume of each slice was extracted using the FSLeyes software (McCarthy, 2020). Total muscle volume (mm3) was calculated using the truncated cone volume formula considering slice and gap volumes.
Multiecho T2-weighted images (16 echo times equally spaced from 7.28 ms to 116.59 ms, 2000-ms repetition time, one accumulation, 20 × 30 mm field of view, 256 × 256 matrix size, total acquisition time = 8.32 min, slice thickness = 1 mm, slice gap = 1 mm) were recorded at rest. T2-weighted images were processed to generate T2-maps on a pixel by pixel basis by fitting the corresponding data with a single exponential function. Mean T2 values of posterior hindlimb muscles were measured on T2 maps and averaged on the 2 largest consecutive slices from the outlined regions of interest. The corresponding values were quantified in total gastrocnemius (GA) muscle, in the tibialis anterior (TA) region.
31P-MRS spectra (8 kHz sweep width; 2048 data points) from posterior hindlimb muscles were continuously acquired before (rest period; 4.7 min duration), during and after (recovery period; 16 min duration) the 6 min moderate exercise. Spectra acquisition was gated to muscle electrostimulation in order to reduce potential motion artifacts due to contraction. A total of 800 saturated free induction decays (FID, 1.875 s repetition time) were acquired. The first 140 FID were acquired in resting muscle and summed together. The next 450 FIDs were acquired during exercise and were summed as blocks of 15, allowing a 30 s temporal resolution. The remaining 210 FIDS were obtained during the recovery period and were summed as blocks of 30 FIDs.
2.9 MRS Data Processing
MRS data were processed using IDL-based (Interactive Data Language, Exelis, Visual Information Solutions, Boulder, CO,United States) custom-written routines (Le Fur et al., 2010) integrating the AMARES Fortran code (Vanhamme et al., 1997) from jMRUI (http://www.jmrui.eu/).
Relative concentrations of phosphocreatine (PCr), inorganic phosphate (Pi) and ATP were obtained from 31P-MRS spectra using the AMARES-MRUI-based time domain fitting routine including appropriate prior knowledge for the ATP multiplets. Absolute amounts of phosphorylated compounds were expressed taking into account a 5 mM β-ATP basal concentration (Giannesini et al., 2013). PCr relative concentration was expressed relative to the PCr content at rest which was set at 100%. Intracellular pH was calculated from the chemical shift of the Pi signal relative to PCr according to the formula (Arnold et al., 1984): pH = 6.75 + log [(3.27-δPi)/(δPi-5.69)]. Cytosolic ADP concentration was calculated, as previously described (Kemp et al., 1993; Harkema and Meyer, 1997): [ADP] = ([Cr] x [ATP])/([PCr]x10−pH x KCK), using the creatine kinase equilibrium constant (KCK = 1.66 × 109 M−1) (Kemp et al., 2001a) and total creatine [Cr] calculated by assuming that PCr represents ∼85% of total Cr (Kemp et al., 2007). The rate of PCr degradation (VPCrstim, in mM/min) at the onset of exercise was calculated as VPCrstim = ΔPCr/τPCrstim, where ΔPCr is the extent of PCr depletion measured at the exercise-end (relative to basal value) and τPCrstim is the time constant of PCr degradation. τPCrstim was determined by fitting the time course of PCr to a mono-exponential function using a least-means-squared algorithm. Force-normalized non-oxidative ATP synthesis (PCrcost in µmol/N) was calculated as the ratio between VPCrstim and the amount of force produced at the beginning of exercise, considering at that time that PCr is the only source of ATP production. Similarly, the PCr recovery kinetic parameters (τPCrrec) were determined during the post-exercise period by fitting the time course of PCr resynthesis to a mono-exponential function. Force-normalized oxidative ATP synthesis (PCrrec in mM/N) was calculated as the ratio between VPCrrec and the amount of force produced at the end of exercise. As previously suggested, VPCrrec was considered as the rate of oxidative ATP production at end of exercise as VPCrrec = ΔPCr/τPCrrec (Boska, 1991; Prompers et al., 2014). The maximal rate of PCr recovery, Vmax, has been calculated as Vmax = VPCrrec (1 + (Km/[ADPend])), where Km is the affinity constant of the mitochondria for ADP (30 μM in gastrocnemius muscle of the mice), and [ADPend] is the ADP concentration at the end of exercise.
2.10 Statistics
All values are presented as means ± SD except on graphics for which SE are displayed for the sake of clarity. Sample distribution was tested with the Shapiro-Wilk test and visual distribution (qqnorm plots). Significant differences were assessed using non-parametric Wilcoxon tests or Welch t-tests. All statistics were performed using R, on RStudio (4.0.2). The significance level was set at p < 0.05.
3 Results
3.1 Morphological Characteristics and Muscle Imaging
Body weight differed significantly between CON and HU groups. As indicated Table 1, HU mice were heavier (34.26 ± 1.94 g) than CON (30.91 ± 2.83 g, p = 0.013). Otherwise, relative and absolute gastrocnemius weights and volumes did not differ between groups.
T2 values of the gastrocnemius were significantly lower in HU (29.43 ± 0.84 ms) as compared to CON (30.79 ± 1.70 ms, p = 0.0328, Table 2). Regarding the tibialis anterior, T2 values were not significantly different between HU (25.33 ± 0.55 ms) and CON (25.74 ± 0.63 ms) groups (p = 0.0952, Table 2).
3.2 Posterior Hindlimb Muscles Bioenergetics
Intracellular pH at rest was similar in HU (7.27 ± 0.09) and CON (7.25 ± 0.08) mice (p = 0.6765, Table 3). During the 6-min moderate-intensity stimulation period, a transient pHi elevation was observed, followed by a pHi reduction. This reduction occurred in both groups with an initial faster decrease followed by a slower phase (Figure 1A). At the end of the exercise period, pHi decreased of 0.25 ± 0.10 and 0.28 ± 0.09 pH unit in HU and CON group respectively (p = 0.58). The initial recovery phase started in a steady-state and continued with a progressive increase towards the resting value (Figure 1A). At the end of the recovery period, pHi was not significantly different in HU and CON (7.24 ± 0.04 and 7.31 ± 0.11, respectively, p = 0.0953). The end of exercise ADP was not significantly different between HU (55.17 ± 12.06 μM) and CON (48.94 ± 8.46 μM, p = 0.23, Table 3) groups.
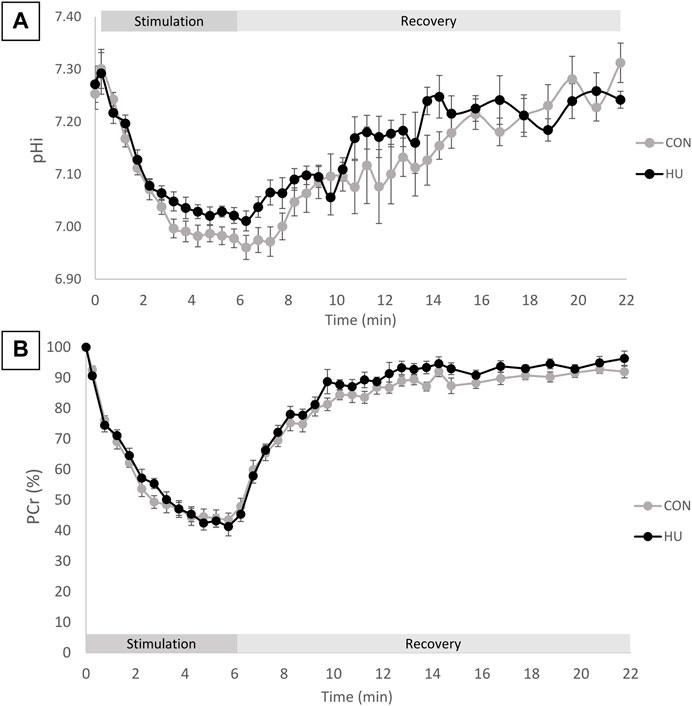
FIGURE 1. (A) pHi time course during the rest-stimulation-recovery protocol in CON and HU. Results are presented as means ± SEM. (B) Relative PCr time-course during the rest-stimulation-recovery protocol in CON and HU. Results are presented as means ± SEM.
As illustrated in Figure 1B, the relative PCr time course can be divided in 3 phases. An initial progressive decrease occurred during the first 4 min of exercise, followed by a plateau from minute 4 to 6 reaching a depletion of −58.68% ± 8.12 and −56.45% ± 6.14 for HU and CON group respectively (p = 0.53, Table 3). The recovery period was characterized by a progressive return towards the baseline values.
The rate of PCr degradation (VPCrstim) and the rate of PCr resynthesis (VPCrrec) were not significantly modified between HU (4.82 ± 1.25 mM/min and 3.69 ± 1.28 mM/min, respectively) and CON (5.44 ± 1.03 mM/min and 3.08 ± 0.61 mM/min, respectively) group (p = 0.2924 and p = 0.2512).
The time constant of PCr degradation (τPCrstim) was not significantly different between HU (2.03 ± 0.90 min) and CON (1.57 ± 0.39 min) groups (p = 0.2109). The time constant of PCr resynthesis (τPCrrec) was similar in HU (2.12 ± 0.43 min) and CON (2.19 ± 0.42 min) groups (p = 0.7459).
The force-normalized non-oxidative ATP synthesis i.e., PCrcost strongly tended to be lower in HU compared to CON (Figure 2A, p = 0.0583). The force-normalized oxidative ATP synthesis i.e. PCrrec was similar in HU and CON mice (Figure 2B, p = 0.5216).
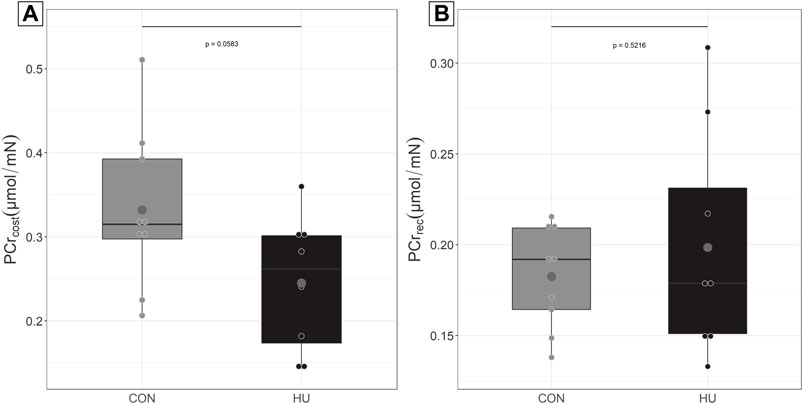
FIGURE 2. (A) PCrcost at the beginning of the moderate exercise in CON (grey, n = 9) and HU (black, n = 8). results are presented in boxplot. (B) PCrrec at the end of moderate exercise, results are presented in boxplot.
The maximal rate of PCr recovery, Vmax, was similar (p = 0.3) between CON (4.99 ± 0.86 mM/min) and HU (5.68 ± 1.68 mM/min) groups.
3.3 Muscle Function
At the onset of stimulation, specific peak force was significantly higher in HU mice (1,348.17 ± 165.82 N/cm3) as compared to CON mice (1,121.78 ± 140.78 N/cm3, p = 0.0093, Figure 3).
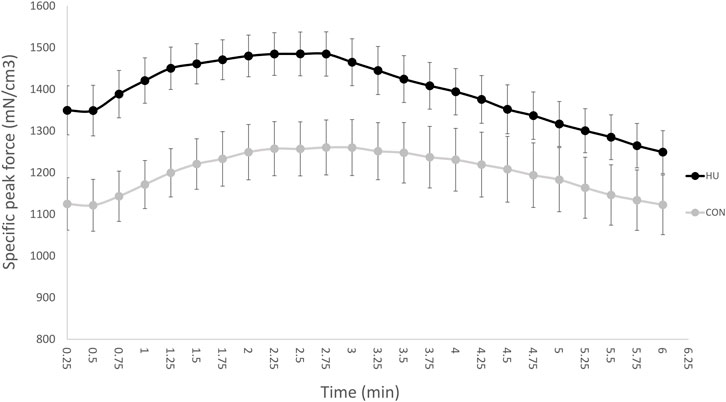
FIGURE 3. Time dependent changes in specific peak force throughout the moderate exercise in CON (grey, n = 9) and HU-treated mice (black, n = 8). Results are presented as means ± SEM.
As illustrated in Figure 3, specific peak force time-course was biphasic with an initial increase and a peak reached after a 90 s stimulation period. Afterwards, specific peak force progressively decreased until the end of stimulation.
As illustrated in Figure 4, HU mice (501.35 ± 54.12 N/mm3) produced a significantly larger specific total force production over the 6-min stimulation period as compared to controls (437.43 ± 57.10 N/mm3, p = 0.0318).
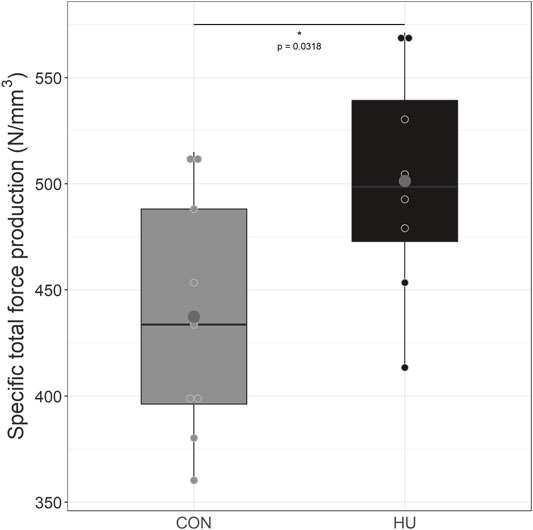
FIGURE 4. Specific total force production from during the 6 minutes moderate exercise in CON (grey, n = 9) and HU (black, n = 8) groups, results are presented in boxplot. * significantly different from HU group.
4 Discussion
In the present study, we aimed at characterizing the changes occurring in skeletal muscle energetics and function in response to a 6-week chronic hydroxyurea supplementation in mildly anemic mice. Our results showed that HU supplementation 1) strongly tended to enhance PCrcost, 2) decreased posterior hindlimb averaged T2 values and 3) increased specific total force production.
4.1 Effect of HU on Skeletal Muscle Oxygenation
Considering the well-known effect of HU on fetal Hb production, we hypothesized that HU could improve muscle oxidative capacity as a consequence of an increased oxygen delivery. This hypothesis was supported by the fact that a beneficial effect of HU treatment has been established on aerobic capacities. Indeed, sickle cell anemia (SCA) patients receiving HU decreased their heart rate in response to a standardized exercise compared to untreated SCA patients (Hackney et al., 1997). The authors suggested an improved cardiovascular efficiency without mentioning underlying mechanisms. In addition, HU supplementation has been related to an increased NO bioavailability which can have a vasorelaxant effect and improve muscle oxygenation (Chen et al., 2008; Roberson and Bennett-Guerrero, 2012; Green and Barral, 2014).
As pH and ADP concentration were similar at the end of exercise, the initial rate of PCr resynthesis, VPCrrec should reflect Vmax as previously suggested (Roussel et al., 2000). Therefore, only VPCrrec will be discussed. In the present study, the unchanged rate of PCr resynthesis, VPCrrec, quantified during the post-stimulation period did not support this hypothesis. The rate of PCr resynthesis and the corresponding kinetic metrics have been largely acknowledged as illustrative of muscle oxidative function (McCully et al., 1993; Kemp and Radda, 1994; Conley et al., 2000; Praet et al., 2007; Lanza et al., 2011). In addition, it has been shown that PCr recovery kinetics could be modulated by the oxygenation status. More specifically, PCr recovery kinetics can be accelerated in hyperoxic conditions in a trained population (Haseler et al., 1999), whereas hypoxia can reduce the corresponding rate in a sedentary and trained population (Haseler et al., 2007, 2004).
Along the same line, it has been previously reported that patient with peripheral arterial disease (PAD) who suffer from a decreased skeletal muscle O2 supply displayed a significant reduced PCr recovery kinetics (Kemp et al., 2001b). Of interest, it has been reported that hyperoxia was not sufficient to improve the recovery kinetics in PAD patients (Hart et al., 2018). However, an improved mitochondrial function was observed when hyperoxia was combined to reactive hyperemia (Hart et al., 2018) thereby suggesting that both convective and diffusive O2 supply may limit mitochondrial oxidative capacity in PAD patients (Hart et al., 2018). These results are totally supportive of the present results obtained in anemic conditions. While HU is expected to increase oxygen supply in the same way as hyperoxia in PAD patients, we could suggest that the unchanged PCr recovery kinetics in HU-treated mice is indicating that anemic muscle is limited not only by oxygen convection but also by oxygen diffusion to muscle cells.
One can also wonder if the lack of effect of HU in the present study may be related to the low impact of mild anemia observed in the used model on the muscle oxidative capacity and mitochondrial function. Moreover, it cannot be excluded that the lack of difference may be related to compensatory mechanisms affecting cardiac output and microvascular blood flow could occur in mild anemic conditions thereby limiting the deleterious effects of the reduced oxygen delivery (Roberson and Bennett-Guerrero, 2012). Overall, these compensatory changes would account for the similar oxidative capacity in treated and untreated mice. Additional measurements are warranted in order to decipher the exact mechanisms.
Nevertheless, PCrcost, an index of the non-oxidative ATP production, tended to be reduced by HU supplementation (p = 0.0583). This can be understood as a reduced glycolytic contribution and/or a larger oxidative production in exercising muscle which could support our primary hypothesis. It would have then to be determined why a potential improved oxygenation could modulate the aerobic ATP production during exercise and not during the post-exercise recovery phase.
4.2 Effect of HU on the ATP Cost of Contraction
The ATP cost of contraction represents the rate of ATP utilization for a given muscle activity. The rate of ATP utilization is actually supported by both oxidative and non-oxidative contributions. In the present study and as previously mentioned, PCrcost strongly tended to be reduced in HU mice compared to CON counterparts (p = 0.058). These results support those from Bailey et al who obtained a reduced PCr consumption in humans during an incremental knee-extension test after beetroot juice (a nitrate provider) supplementation (Bailey et al., 2010).
This decreased PCrcost can be understood as a lower rate of ATP utilization for a given force or a faster rate of ATP resynthesis which mainly relies on oxidative capacity. In the study of Bailey et al, the PCrcost reduction was explained as a reduced rate of ATP hydrolysis for a given work (Bailey et al., 2010). Of note, ATP demand is conditioned by both contractile and non-contractile processes keeping in mind that non-contractile processes such as sarcoplasmic Ca2+ pumping can represent up to 20-50% of the total ATP turnover (Bergstrom and Hultman, 1988).
Nevertheless, a faster oxidative rate of ATP resynthesis by oxidative pathway cannot be totally excluded. Ferguson et al (2015) reported a higher microvascular partial pressure of oxygen (PO2mv) after a dietary nitrate supplementation (Ferguson et al., 2015) which could be expected to increase the rate of oxidative wing of ATP production thereby reducing the exercise-induced PCr consumption (Haseler et al., 1998; Vanhatalo et al., 2011; Ferguson et al., 2015). Neither the present results nor those from Bailey et al (2010) indicated an improved rate of PCr resynthesis, VPCrrec.
Moreover, oxidative stress, which has been reported as elevated in various anemias (Akohoue et al., 2007; Fibach and Rachmilewitz, 2008; Nur et al., 2011; Voskou et al., 2015; Vinhaes et al., 2020), has been previously reported to impair mitochondrial function (Halestrap et al., 1993; Guo et al., 2013). Of interest, it has been previously reported that SCA patients treated with HU displayed a reduced degree of oxidative perturbation as compared to untreated SCA patients (Vinhaes et al., 2020). If such a phenomenon was occurring in HU-treated mice, our results indicate that it did not affect mitochondrial function.
The reduced PCrcost could result from a reduced rate of ATP hydrolysis. Accordingly, Hernandez et al suggested that the larger force production resulting from nitrate ingestion would result from changes in Ca2+ handling (Hernández et al., 2012), indicating that the non-contractile ATP demand might be reduced.
4.3 Effect of HU on Force Production
Specific total peak force (total peak force scaled to muscle volume) developed by HU-treated mice was significantly higher as compared to CON. Such a direct effect of HU on force production has never been reported in mild anemic mice. However, Wali & Moheeb reported a higher absolute force production of the right hand and leg in HU-supplemented children compared to healthy controls (Wali and Moheeb, 2011). HU has also been associated to a decreased heart rate during a treadmill exercise together with a longer exercise time before exhaustion both indicative of an improved exercise capacity (Wali and Moheeb, 2011). A similar beneficial effect on exercise capacity has already been described in HU-treated SCA patients with an improved performance for the Wingate test (Hackney et al., 1997). Both results have been directly related to the increased fetal hemoglobin level with a corresponding reduction in the HbS level and a reduction of clinical symptoms.
Of interest, in vitro measurements could suggest some accounting factors of the force increase. An increased contractile force has been reported in vitro in fast-twitch fibers (dominant fiber type) in mice supplemented with nitrate, an important NO precursor (Hernández et al., 2012). Of interest, HU supplementation has been shown to increase NO production and bioavailability (Jiang et al., 1997; Glover et al., 1999; Huang et al., 2002). More specifically, HU can oxidize oxy and deoxy-hemoglobin to methemoglobin, which can then react with other HU molecules and form nitrosylhemoglobin (HbNO) slowly releasing NO (Jiang et al., 1997).
Alternatively, this increased force production might be related to a larger relative amount of fast-twitch fibers in HU mice. This means that HU would induce a slow to fast-twitch fiber transition. The reduced T2 values we quantified in HU mice compared to CON in the present study might be supportive of this hypothesis since lower T2 values have been related to a larger quantity of fast-twitch glycolytic fibers (Hatakenaka et al., 2001). The averaged T2 of water protons illustrates the molecular environment together with the water mobility. Previous studies have mainly reported T2 changes (mainly increase) in a variety of conditions such as eccentric exercise, inflammatory disorders etc. (Fisher et al., 1990; Marqueste et al., 2008; Esposito et al., 2013; Bryant et al., 2014; Zaccagnini et al., 2015), and reduced T2 values have been scarcely reported.
Considering the beneficial effects of HU in daily activity of patients (Fucharoen et al., 1996; Singer et al., 2005; Ballas et al., 2006; Algiraigri and Kassam, 2017), cage activity of mice in the present study might have increased thereby accounting for the improved muscle function (Bowden Davies et al., 2019).
4.4 Effect Mediated by Nitric Oxide
Overall, HU-supplementation was related to a likely reduced non oxidative cost of contraction and an increased skeletal muscle force production. On the basis of literature results and of the well-known effects of HU on NO production, we strongly pointed out the increased NO bioavailability as a strong potential accounting factor for the present results. Although NO measurements would have to be performed as a confirmatory support, potential underlying effects mediated by NO are of interest to mention.
The increased bioavailability of NO would improve local blood flow, fatigue resistance and fiber contractility (Jones et al., 2016). In this way, the increased contractility may have increased force production and decreased the cost of non-contractile processes, inevitably reducing ATP consumption.
5 Conclusion
In the present study, we observed a potential ergogenic effect of a chronic supplementation of hydroxyurea in a group of mice with mild anemia. HU supplementation improved force production, modified bioenergetics and reduced the average T2 values. Further studies are necessary to confirm the underlying mechanisms of the observed changes.
Data Availability Statement
The raw data supporting the conclusions of this article will be made available by the authors, without undue reservation.
Ethics Statement
The animal study was reviewed and approved by Institutional Animal Care Committee of Aix-Marseille University (permit number #1841-2019011016466656).
Author Contributions
CM, LM, and DB contributed to conception and design of the study; CM, BG, CV, and YF performed experiments; CM analyzed data; CM, LM, BC, and DB interpreted results of experiments; CM prepared figures; CM, LM, BG, and DB drafted manuscript; CM, LM, BC, BG, CV, YF, and DB edited and revised manuscript; CM, LM, BC, BG, CV, YF, and DB approved final version of manuscript.
Funding
This work was partly funded by France Life Imaging (grant ANR-11-INBS-0006), AFM and Region sud and by the European Union's Horizon 2020 research and innovation programme under grant agreement No 736937.
Conflict of Interest
The authors declare that the research was conducted in the absence of any commercial or financial relationships that could be construed as a potential conflict of interest.
Publisher’s Note
All claims expressed in this article are solely those of the authors and do not necessarily represent those of their affiliated organizations, or those of the publisher, the editors and the reviewers. Any product that may be evaluated in this article, or claim that may be made by its manufacturer, is not guaranteed or endorsed by the publisher.
Acknowledgments
Hydroxyurea has been kindly provided by the ADDMEDICA company.
References
Akohoue S. A., Shankar S., Milne G. L., Morrow J., Chen K. Y., Ajayi W. U., et al. (2007). Energy Expenditure, Inflammation, and Oxidative Stress in Steady-State Adolescents with Sickle Cell Anemia. Pediatr. Res. 61, 233–238. doi:10.1203/pdr.0b013e31802d7754
Algiraigri A. H., Kassam A. (2017). Hydroxyurea for Hemoglobin E/β-Thalassemia: A Systematic Review and Meta-Analysis. Int. J. Hematol. 106, 748–756. doi:10.1007/s12185-017-2307-0
Algiraigri A. H., Wright N. A., Kassam A. (2014). Hydroxyurea for β-Thalassemia Major: A Meta-Analysis. Blood 124, 4894. doi:10.1182/blood.V124.21.4894.4894
Algiraigri A. H., Wright N. A. M., Paolucci E. O., Kassam A. (2017). Hydroxyurea for Lifelong Transfusion-Dependent β-Thalassemia: A Meta-Analysis. Pediatr. Hematol. Oncol. 34, 435–448. doi:10.1080/08880018.2017.1354948
Almeida C. B., Scheiermann C., Jang J.-E., Prophete C., Costa F. F., Conran N., et al. (2012). Hydroxyurea and a cGMP-Amplifying Agent Have Immediate Benefits on Acute Vaso-Occlusive Events in Sickle Cell Disease Mice. Blood 120, 2879–2888. doi:10.1182/blood-2012-02-409524
Arnold D. L., Matthews P. M., Radda G. K. (1984). Metabolic Recovery After Exercise and the Assessment of Mitochondrial Functionin Vivo in Human Skeletal Muscle by Means of 31P NMR. Magn. Reson. Med. 1, 307–315. doi:10.1002/mrm.1910010303
Arruda V. R., Lima C. S. P., Saad S. T. O., Costa F. F. (1997). Successful Use of Hydroxyurea in β-Thalassemia Major. N. Engl. J. Med. 336, 964–965. doi:10.1056/NEJM199703273361318
Bailey S. J., Fulford J., Vanhatalo A., Winyard P. G., Blackwell J. R., DiMenna F. J., et al. (2010). Dietary Nitrate Supplementation Enhances Muscle Contractile Efficiency During Knee-Extensor Exercise in Humans. J. Appl. Physiology 109, 135–148. doi:10.1152/japplphysiol.00046.2010
Ballas S. K., Barton F. B., Waclawiw M. A., Swerdlow P., Eckman J. R., Pegelow C. H., et al. (2006). Hydroxyurea and Sickle Cell Anemia: Effect on Quality of Life. Health Qual. Life Outcomes 4, 59. doi:10.1186/1477-7525-4-59
Bergstrom M., Hultman E. (1988). Energy Cost and Fatigue During Intermittent Electrical Stimulation of Human Skeletal Muscle. J. Appl. Physiology 65, 1500–1505. doi:10.1152/jappl.1988.65.4.1500
Boska M. (1991). Estimating the ATP Cost of Force Production in the Human Gastrocnemius/Soleus Muscle Group Using 31P MRS and 1H MRI. NMR Biomed. 4, 173–181. doi:10.1002/nbm.1940040404
Bowden Davies K. A., Pickles S., Sprung V. S., Kemp G. J., Alam U., Moore D. R., et al. (2019). Reduced Physical Activity in Young and Older Adults: Metabolic and Musculoskeletal Implications. Ther. Adv. Endocrinol. 10, 204201881988882. doi:10.1177/2042018819888824
Bryant N. D., Li K., Does M. D., Barnes S., Gochberg D. F., Yankeelov T. E., et al. (2014). Multi-Parametric MRI Characterization of Inflammation in Murine Skeletal Muscle. NMR Biomed. 27, 716–725. doi:10.1002/nbm.3113
Charache S., Terrin M. L., Moore R. D., Dover G. J., Barton F. B., Eckert S. V., et al. (1995). Effect of Hydroxyurea on the Frequency of Painful Crises in Sickle Cell Anemia. N. Engl. J. Med. 332, 1317–1322. doi:10.1056/NEJM199505183322001
Chen K., Pittman R. N., Popel A. S. (2008). Nitric Oxide in the Vasculature: Where Does it Come from and Where Does It Go? A Quantitative Perspective. Antioxidants Redox Signal. 10, 1185–1198. doi:10.1089/ars.2007.1959
Cokic V. P., Smith R. D., Beleslin-Cokic B. B., Njoroge J. M., Miller J. L., Gladwin M. T., et al. (2003). Hydroxyurea Induces Fetal Hemoglobin by the Nitric Oxide-Dependent Activation of Soluble Guanylyl Cyclase. J. Clin. Invest. 111, 231–239. doi:10.1172/JCI200316672
Conley K. E., Jubrias S. A., Esselman P. C. (2000). Oxidative Capacity and Ageing in Human Muscle. J. Physiology 526, 203–210. doi:10.1111/j.1469-7793.2000.t01-1-00203.x
Esposito A., Campana L., Palmisano A., De Cobelli F., Canu T., Santarella F., et al. (2013). Magnetic Resonance Imaging at 7T Reveals Common Events in Age-Related Sarcopenia and in the Homeostatic Response to Muscle Sterile Injury. PLoS ONE 8, e59308. doi:10.1371/journal.pone.0059308
Ferguson S. K., Holdsworth C. T., Wright J. L., Fees A. J., Allen J. D., Jones A. M., et al. (2015). Microvascular Oxygen Pressures in Muscles Comprised of Different Fiber Types: Impact of Dietary Nitrate Supplementation. Nitric Oxide 48, 38–43. doi:10.1016/j.niox.2014.09.157
Fibach E., Rachmilewitz E. (2008). The Role of Oxidative Stress in Hemolytic Anemia. Cmm 8, 609–619. doi:10.2174/156652408786241384
Fisher M. J., Meyer R. A., Adams G. R., Foley J. M., Potchen E. J. (1990). Direct Relationship Between Proton T2 and Exercise Intensity in Skeletal Muscle MR Images. Investig. Radiol. 25, 480–485. doi:10.1097/00004424-199005000-00003
Fucharoen S., Siritanaratkul N., Winichagoon P., Chowthaworn J., Siriboon W., Muangsup W., et al. (1996). Hydroxyurea Increases Hemoglobin F Levels and Improves the Effectiveness of Erythropoiesis in Beta-Thalassemia/Hemoglobin E Disease. Blood 87, 887–892. doi:10.1182/blood.v87.3.887.bloodjournal873887
Giannesini B., Vilmen C., Amthor H., Bernard M., Bendahan D. (2013). Lack of Myostatin Impairs Mechanical Performance and ATP Cost of Contraction in Exercising Mouse Gastrocnemius Muscle In Vivo. Am. J. Physiology-Endocrinology Metabolism 305, E33–E40. doi:10.1152/ajpendo.00651.2012
Giannesini B., Vilmen C., Le Fur Y., Dalmasso C., Cozzone P. J., Bendahan D. (2010). A Strictly Noninvasive MR Setup Dedicated to Longitudinal Studies of Mechanical Performance, Bioenergetics, Anatomy, and Muscle Recruitment in Contracting Mouse Skeletal Muscle. Magn. Reson. Med. 64, 262–270. doi:10.1002/mrm.22386
Glover R. E., Ivy E. D., Orringer E. P., Maeda H., Mason R. P. (1999). Detection of Nitrosyl Hemoglobin in Venous Blood in the Treatment of Sickle Cell Anemia with Hydroxyurea. Mol. Pharmacol. 55, 1006–1010. doi:10.1124/mol.55.6.1006
Green N. S., Barral S. (2014). Emerging Science of Hydroxyurea Therapy for Pediatric Sickle Cell Disease. Pediatr. Res. 75, 196–204. doi:10.1038/pr.2013.227
Guo C., Sun L., Chen X., Zhang D. (2013). Oxidative Stress, Mitochondrial Damage and Neurodegenerative Diseases. Neural Regen. Res. 8, 2003–2014. doi:10.3969/j.issn.1673-5374.2013.21.009
Hackney A. C., Hezier W., Gulledge T. P., Jones S., Strayhorn D., Busby M., et al. (1997). Effects of Hydroxyurea Administration on the Body Weight, Body Composition and Exercise Performance of Patients with Sickle-Cell Anaemia. Clin. Sci. 92, 481–486. doi:10.1042/cs0920481
Halestrap A. P., Griffiths E. J., Connern C. P. (1993). Mitochondrial Calcium Handling and Oxidative Stress. Biochem. Soc. Trans. 21, 353–358. doi:10.1042/bst0210353
Harkema S. J., Meyer R. A. (1997). Effect of Acidosis on Control of Respiration in Skeletal Muscle. Am. J. Physiology-Cell Physiology 272, C491–C500. doi:10.1152/ajpcell.1997.272.2.C491
Hart C. R., Layec G., Trinity J. D., Le Fur Y., Gifford J. R., Clifton H. L., et al. (2018). Oxygen Availability and Skeletal Muscle Oxidative Capacity in Patients with Peripheral Artery Disease: Implications from In Vivo and In Vitro Assessments. Am. J. Physiology-Heart Circulatory Physiology 315, H897–H909. doi:10.1152/ajpheart.00641.2017
Haseler L. J., Hogan M. C., Richardson R. S. (1999). Skeletal Muscle Phosphocreatine Recovery in Exercise-Trained Humans Is Dependent on O2 availability. J. Appl. Physiology 86, 2013–2018. doi:10.1152/jappl.1999.86.6.2013
Haseler L. J., Lin A., Hoff J., Richardson R. S., (2007). Oxygen Availability and PCr Recovery Rate in Untrained Human Calf Muscle: Evidence of Metabolic Limitation in Normoxia. Am. J. Physiology-Regulatory, Integr. Comp. Physiology 293, R2046–R2051. doi:10.1152/ajpregu.00039.2007
Haseler L. J., Lin A. P., Richardson R. S. (2004). Skeletal Muscle Oxidative Metabolism in Sedentary Humans: 31P-MRS Assessment of O2 Supply and Demand Limitations. J. Appl. Physiology 97, 1077–1081. doi:10.1152/japplphysiol.01321.2003
Haseler L. J., Richardson R. S., Videen J. S., Hogan M. C. (1998). Phosphocreatine Hydrolysis During Submaximal Exercise: the Effect of F I O 2. J. Appl. Physiology 85, 1457–1463. doi:10.1152/jappl.1998.85.4.1457
Hatakenaka M., Ueda M., Ishigami K., Otsuka M., Masuda K. (2001). Effects of Aging on Muscle T2 Relaxation Time. Investig. Radiol. 36, 692–698. doi:10.1097/00004424-200112000-00003
Hernández A., Schiffer T. A., Ivarsson N., Cheng A. J., Bruton J. D., Lundberg J. O., et al. (2012). Dietary Nitrate Increases Tetanic [Ca2+]i and Contractile Force in Mouse Fast-Twitch Muscle. J. Physiol. 590, 3575–3583. doi:10.1113/jphysiol.2012.232777
Huang J., Hadimani S. B., Rupon J. W., Ballas S. K., Kim-Shapiro D. B., King S. B. (2002). Iron Nitrosyl Hemoglobin Formation From the Reactions of Hemoglobin and Hydroxyurea. Biochemistry 41, 2466–2474. doi:10.1021/bi011470o
Jiang J., Jordan S. J., Barr D. P., Gunther M. R., Maeda H., Mason R. P. (1997). In Vivo Production of Nitric Oxide in Rats after Administration of Hydroxyurea. Mol. Pharmacol. 52, 1081–1086. doi:10.1124/mol.52.6.1081
Jones A. M., Ferguson S. K., Bailey S. J., Vanhatalo A., Poole D. C. (2016). Fiber Type-Specific Effects of Dietary Nitrate. Exerc. Sport Sci. Rev. 44, 53–60. doi:10.1249/JES.0000000000000074
Kemp G. J., Radda G. K. (1994). Quantitative Interpretation of Bioenergetic Data from 31P and 1H Magnetic Resonance Spectroscopic Studies of Skeletal Muscle: An Analytical Review. Magn. Reson. Q. 10, 43–63.
Kemp G. J., Meyerspeer M., Moser E. (2007). Absolute Quantification of Phosphorus Metabolite Concentrations in Human Musclein Vivo by31P MRS: a Quantitative Review. NMR Biomed. 20, 555–565. doi:10.1002/nbm.1192
Kemp G. J., Roberts N., Bimson W. E., Bakran A., Harris P. L., Gilling-Smith G. L., et al. (2001a). Mitochondrial Function and Oxygen Supply in Normal and in Chronically Ischemic Muscle: A Combined 31P Magnetic Resonance Spectroscopy and Near Infrared Spectroscopy Study In Vivo. J. Vasc. Surg. 34, 1103–1110. doi:10.1067/mva.2001.117152
Kemp G. J., Roussel M., Bendahan D., Fur Y. L., Cozzone P. J. (2001b). Interrelations of ATP Synthesis and Proton Handling in Ischaemically Exercising Human Forearm Muscle Studied by31P Magnetic Resonance Spectroscopy. J. Physiology 535, 901–928. doi:10.1111/j.1469-7793.2001.00901.x
Kemp G. J., Taylor D. J., Radda G. K. (1993). Control of Phosphocreatine Resynthesis during Recovery from Exercise in Human Skeletal Muscle. NMR Biomed. 6, 66–72. doi:10.1002/nbm.1940060111
Lanza I. R., Bhagra S., Nair K. S., Port J. D. (2011). Measurement of Human Skeletal Muscle Oxidative Capacity by 31P-MR Spectroscopy: A Cross-Validation with In Vitro Measurements. J. Magn. Reson. Imaging 34, 1143–1150. doi:10.1002/jmri.22733
Le Fur Y., Nicoli F., Guye M., Confort-Gouny S., Cozzone P. J., Kober F. (2010). Grid-free Interactive and Automated Data Processing for MR Chemical Shift Imaging Data. Magn. Reson Mater Phy 23, 23–30. doi:10.1007/s10334-009-0186-y
Lebensburger J. D., Pestina T. I., Ware R. E., Boyd K. L., Persons D. A. (2010). Hydroxyurea Therapy Requires HbF Induction for Clinical Benefit in a Sickle Cell Mouse Model. Haematologica 95, 1599–1603. doi:10.3324/haematol.2010.023325
Marqueste T., Giannesini B., Fur Y. L., Cozzone P. J., Bendahan D. (2008). Comparative MRI Analysis of T2 Changes Associated with Single and Repeated Bouts of Downhill Running Leading to Eccentric-Induced Muscle Damage. J. Appl. Physiology 105, 299–307. doi:10.1152/japplphysiol.00738.2007
McCarthy P. (2020). FSLeyes. Oxford, United-Kingdom: University of Oxford. doi:10.5281/ZENODO.3858136
McCully K. K., Fielding R. A., Evans W. J., Leigh J. S., Posner J. D. (1993). Relationships between In Vivo and In Vitro Measurements of Metabolism in Young and Old Human Calf Muscles. J. Appl. Physiology 75, 813–819. doi:10.1152/jappl.1993.75.2.813
McGann P. T., Ware R. E. (2015). Hydroxyurea Therapy for Sickle Cell Anemia. Expert Opin. Drug Saf. 14, 1749–1758. doi:10.1517/14740338.2015.1088827
Nur E., Biemond B. J., Otten H.-M., Brandjes D. P., Schnog J.-J. B., CURAMA Study G. (2011). Oxidative Stress in Sickle Cell Disease; Pathophysiology and Potential Implications for Disease Management. Am. J. Hematol. 86, 484–489. doi:10.1002/ajh.22012
Platt O. S., Orkin S. H., Dover G., Beardsley G. P., Miller B., Nathan D. G. (1984). Hydroxyurea Enhances Fetal Hemoglobin Production in Sickle Cell Anemia. J. Clin. Invest. 74, 652–656. doi:10.1172/JCI111464
Praet S. F. E., Feyter H. M. M. D., Jonkers R. A. M., Nicolay K., Van Pul C., Kuipers H., et al. (2007). 31P MR Spectroscopy and In Vitro Markers of Oxidative Capacity in Type 2 Diabetes Patients. Magn. Reson Mater Phy 19, 321–331. doi:10.1007/s10334-006-0060-0
Prompers J. J., Wessels B., Kemp G. J., Nicolay K. (2014). MITOCHONDRIA: Investigation of In Vivo Muscle Mitochondrial Function by 31P Magnetic Resonance Spectroscopy. Int. J. Biochem. Cell Biol. 50, 67–72. doi:10.1016/j.biocel.2014.02.014
Rees D. C., Styles L., Vichinsky E. P., Clegg J. B., Weatherall D. J. (1998). The Hemoglobin E Syndromes. Ann. N. Y. Acad. Sci. 850, 334–343. doi:10.1111/j.1749-6632.1998.tb10490.x
Roberson R. S., Bennett-Guerrero E. (2012). Impact of Red Blood Cell Transfusion on Global and Regional Measures of Oxygenation. Mt. Sinai J. Med. 79, 66–74. doi:10.1002/msj.21284
Roussel M., Bendahan D., Mattei J. P., Le Fur Y., Cozzone P. J. (2000). 31P Magnetic Resonance Spectroscopy Study of Phosphocreatine Recovery Kinetics in Skeletal Muscle: the Issue of Intersubject Variability. Biochimica Biophysica Acta (BBA) - Bioenergetics 1457, 18–26. doi:10.1016/s0005-2728(99)00111-5
Singer S. T., Kuypers F. A., Olivieri N. F., Weatherall D. J., Mignacca R., Coates T. D., et al. (2005). The E/beta0 Thalassaemia Study GroupFetal Haemoglobin Augmentation in E/beta0 Thalassaemia: Clinical and Haematological Outcome. Br. J. Haematol. 131, 378–388. doi:10.1111/j.1365-2141.2005.05768.x
Vanhamme L., van den Boogaart A., Van Huffel S. (1997). Improved Method for Accurate and Efficient Quantification of MRS Data with Use of Prior Knowledge. J. Magnetic Reson. 129, 35–43. doi:10.1006/jmre.1997.1244
Vanhatalo A., Fulford J., Bailey S. J., Blackwell J. R., Winyard P. G., Jones A. M. (2011). Dietary Nitrate Reduces Muscle Metabolic Perturbation and Improves Exercise Tolerance in Hypoxia. J. Physiol. 589, 5517–5528. doi:10.1113/jphysiol.2011.216341
Vinhaes C. L., Teixeira R. S., Monteiro-Júnior J. A. S., Tibúrcio R., Cubillos-Angulo J. M., Arriaga M. B., et al. (2020). Hydroxyurea Treatment Is Associated with Reduced Degree of Oxidative Perturbation in Children and Adolescents with Sickle Cell Anemia. Sci. Rep. 10, 18982. doi:10.1038/s41598-020-76075-5
Voskou S., Aslan M., Fanis P., Phylactides M., Kleanthous M. (2015). Oxidative Stress in β-thalassaemia and Sickle Cell Disease. Redox Biol. 6, 226–239. doi:10.1016/j.redox.2015.07.018
Wali Y. A., Moheeb H. (2011). Effect of Hydroxyurea on Physical Fitness Indices in Children with Sickle Cell Anemia. Pediatr. Hematol. Oncol. 28, 43–50. doi:10.3109/08880018.2010.524278
Woodson R. D., Wills R. E., Lenfant C. (1978). Effect of Acute and Established Anemia on O2 Transport at Rest, Submaximal and Maximal Work. J. Appl. Physiology 44, 36–43. doi:10.1152/jappl.1978.44.1.36
Wu L.-C., Sun C.-W., Ryan T. M., Pawlik K. M., Ren J., Townes T. M. (2006). Correction of Sickle Cell Disease by Homologous Recombination in Embryonic Stem Cells. Blood 108, 1183–1188. doi:10.1182/blood-2006-02-004812
Keywords: hydroxyurea, anemia, skeletal muscle function, skeletal muscle energetics, magnetic resonance imaging, magnetic resonance spectroscopy
Citation: Michel CP, Messonnier LA, Giannesini B, Chatel B, Vilmen C, Le Fur Y and Bendahan D (2022) Effects of Hydroxyurea on Skeletal Muscle Energetics and Function in a Mildly Anemic Mouse Model. Front. Physiol. 13:915640. doi: 10.3389/fphys.2022.915640
Received: 08 April 2022; Accepted: 16 May 2022;
Published: 15 June 2022.
Edited by:
Enrique Jaimovich, University of Chile, ChileReviewed by:
Roland Pittman, Virginia Commonwealth University, United StatesCorey R. Hart, Wright-Patterson Air Force Base, United States
Copyright © 2022 Michel, Messonnier, Giannesini, Chatel, Vilmen, Le Fur and Bendahan. This is an open-access article distributed under the terms of the Creative Commons Attribution License (CC BY). The use, distribution or reproduction in other forums is permitted, provided the original author(s) and the copyright owner(s) are credited and that the original publication in this journal is cited, in accordance with accepted academic practice. No use, distribution or reproduction is permitted which does not comply with these terms.
*Correspondence: Constance P. Michel, Y29uc3RhbmNlLm1pY2hlbEB1bml2LWFtdS5mcg==