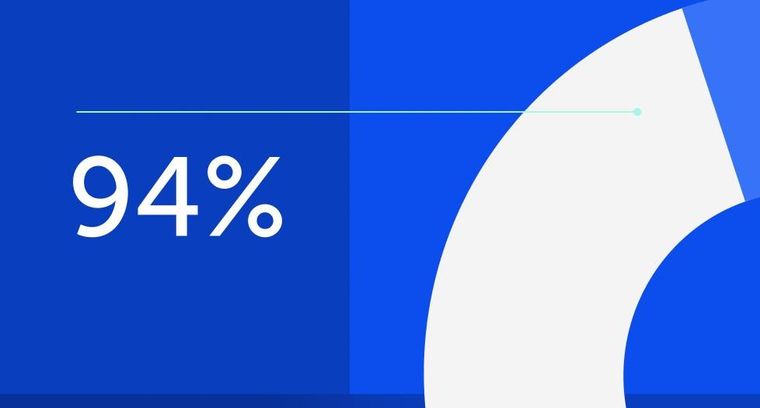
94% of researchers rate our articles as excellent or good
Learn more about the work of our research integrity team to safeguard the quality of each article we publish.
Find out more
REVIEW article
Front. Physiol., 27 April 2022
Sec. Invertebrate Physiology
Volume 13 - 2022 | https://doi.org/10.3389/fphys.2022.905441
Insect life cycle is coordinated by hormones and their downstream effectors. Krüppel homolog1 (Kr-h1) is one of the crucial effectors which mediates the actions of the two critical hormones of insects, the juvenile hormone (JH) and 20-hydroxyecdysone (20E). It is a transcription factor with a DNA-binding motif of eight C2H2 zinc fingers which is found to be conserved among insect orders. The expression of Kr-h1 is fluctuant during insect development with high abundance in juvenile instars and lower levels in the final instar and pupal stage, and reappearance in adults, which is governed by the coordination of JH, 20E, and miRNAs. The dynamic expression pattern of Kr-h1 is closely linked to its function in the entire life of insects. Over the past several years, accumulating studies have advanced our understanding of the role of Kr-h1 during insect development. It acts as a universal antimetamorphic factor in both hemimetabolous and holometabolous species by directly inhibiting the transcription of 20E signaling genes Broad-Complex (Br-C) and Ecdysone induced protein 93F (E93), and steroidogenic enzyme genes involved in ecdysone biosynthesis. Meanwhile, it promotes vitellogenesis and ovarian development in the majority of studied insects. In addition, Kr-h1 regulates insect behavioral plasticity and caste identity, neuronal morphogenesis, maturation of sexual behavior, as well as embryogenesis and metabolic homeostasis. Hence, Kr-h1 acts as a cornerstone regulator in insect life.
Insects represent the largest and most diverse group of animals, with over 70% of all species. They are the first to master flight and establish social societies (Misof et al., 2014). The complex life cycles of insects, which include an abrupt ontogenetic change in an individual’s morphology, physiology, and behavior, undeniably play an important role during insect evolution. These changing life patterns were intimately linked to two hormonal systems, the 20-hydroxyecdysone (20E) and juvenile hormone (JH), which function in both embryonic and postembryonic phases. Generally, pulses of 20E initiate each of the major developmental transitions, while JH is classically viewed as a “status quo” and gonadotropic hormone (Riddiford, 2012; Truman, 2019). 20E induces and directs its functions through the ecdysone receptor (EcR), and a cascade of transcription factors. JH directs 20E action through binding the nuclear receptor methoprene-tolerant (Met) (Jindra et al., 2015) or unidentified membrane receptor (Liu et al., 2015) to control the expression of transcription factor Krüppel homolog1 (Kr-h1) which in turn regulates other stage-specifying genes. However, Kr-h1 is also regulated by 20E and involved in the 20E-mediated events. Hence, it is a major factor that is critical to life of the insects and that has been repurposed during evolution to regulate many processes. The aim of this review is to summarize the significant advances that have been made in the molecular action of Kr-h1 in insect life during the past few years to get a general understandingof the functions of Kr-h1.
Kr-h1 was initially discovered in Drosophila melanogaster by characterizing a P-element induced mutant that dies during the prepupal period and fails to complete head eversion. Molecular studies revealed that the Kr-h1 locus gives rise to two major transcripts (Kr-h1α and Kr-h1β) which derive from two distinct promoters. The Kr-h1α encodes a 791 amino acid protein while Kr-h1β has an additional 54 residues at the N-terminus. Both protein isoforms contain a central domain with eight C2H2 zinc fingers belonging to the Krüppel-like family of transcription factors (Schuh et al., 1986; Pecasse et al., 2000). Subsequently, Kr-h1 has been cloned in numerous insects from the holometabolan species Apis mellifera (Grozinger et al., 2003), Tribolium castaneum (Minakuchi et al., 2009), Aedes aegypti (Zhu et al., 2010), Bombyx mori (Kayukawa et al., 2012), Helicoverpa armigera (Zhang W.-N. et al., 2018), Bactrocera dorsalis (Yue et al., 2018), Sitodiplosis mosellana (Cheng et al., 2020), Chilo suppressalis (Tang et al., 2020), Colaphellus bowringi (Guo et al., 2021), and Harmoniaaxyridis (Han et al., 2022) to the hemimetabolous species Blattella germanica (Lozano and Belles, 2011), Pyrrhocoris apterus (Konopova et al., 2011), Rhodnius prolixus (Konopova et al., 2011), Locusta migratoria (Song et al., 2014), Nilaparvata lugens (Li et al., 2018)and Sogatella furcifera (Hu et al., 2020). More recently, Kr-h1 has also been characterized in two most-ancestral insect orders, the Ephemeroptera Cloeon dipterum (Kamsoi et al., 2021) and the Odonata Ischnura senegalensis and Pseudothemis zonata (Okude et al., 2022), which belong to paleopterans. Alignments of the full protein sequences of the orthologs reveal that the eight zinc-finger DNA binding domains are remarkably well conserved among insect orders, as are two additional regions (LPL/PRKR and RXXSVIXXA) at the extreme C-terminus which are proved to be involved in the transcriptional inhibitory activity of Kr-h1 (Kayukawa et al., 2012; Kayukawa et al., 2016; Li et al., 2018; Cheng et al., 2020). However, the number of amino acids located between the first and second Zn finger, and that between LPL/PRKR and RXXSVIXXA varies with the taxon, especially distinguished from the dipterans. In the dipteran lineage, the sequence between Z1 and Z2 ranges from 34–65 aa, whereas in other taxa, it varies from 9–14 aa. Also, the sequence between LPL/PRKR and RXXSVIXXA is longer in the dipteran lineage (28–60 aa) relative to 14–19 aa in other taxa.
In D. melanogaster, the stage and tissue expression patterns of DmKr-h1α and DmKr-h1β are distinguished. DmKr-h1β is the major transcript in embryogenesis with a broad peak of expression between 8 and 12 h after egg laying and localized to specific neuronal cells (Beck et al., 2004), whereas DmKr-h1α predominates throughout larval life. DmKr-h1α transiently peaks from the onset of pupariationuntil 6–8 h after pupariation (Pecasse et al., 2000; He et al., 2020), and finally reappears in adults (Pecasse et al., 2000). Unlike DmKr-h1β, DmKr-h1α expression spreads to most larval and prepupal tissues, including diploid imaginal discs that are set aside in the larva to later form adult structures (Pecasse et al., 2000; Beck et al., 2005). In B. mori, Kr-h1 also encodes two isoforms (BmKr-h1α and BmKr-h1β), but the overall expression patterns of these two isoforms are very similar, although the levels of BmKr-h1β are far lower than BmKr-h1α. The expression of Kr-h1 is fluctuant during B. mori development. Both BmKr-h1α and BmKr-h1β are continuously expressed in B. mori epidermis during the larval stages but disappear completely upon ecdysis to the final larval instar, reappear and peak during the prepupal stage, then disappear again in the pupae. From the late pupal stage, they increase gradually and maintain high levels during the adult stage (Kayukawa et al., 2014).
In other insects, temporal expression profiles of Kr-h1 mRNA also show abundant levels in larval or nymphal instars, but sudden decrease during the final juvenile stage, particularly in pupae of holometabolan species, such as T. castaneum (Minakuchi et al., 2009) and H. armigera (Zhang W.-N. et al., 2018), or in the last nymphal instar of hemimetabolous species, such as B. germanica (Lozano and Belles, 2011), P. apterus (Konopova et al., 2011) and N. lugens (Li et al., 2018), and the expression remains undetectable for several days until the adult molt. It is remarkable to note that a transient peak of Kr-h1 appearsat the prepupal stage of holometabolans or at the mid-end of the penultimate nymphal instar of hemimetabolans respectively, which is of paramount importance that we will discuss in the following section.
Kr-h1 was initially reported to be a 20E-inducible gene in D. melanogaster. Several Kr-h1 mutants die at the prepupal-pupal transition, or shortly after hatching to a first instar larva. The mutations lead to defects in the timing and level of expression of the key 20E-response genes during metamorphosis or embryogenesis (Pecasse et al., 2000; Beck et al., 2004). Treating salivary glands from wandering larvae or prepupae with 20E increases Kr-h1 transcript levels by approximately fivefold. This induction seems to be a primary response because it does not require new protein synthesis (Beckstead et al., 2005). Importantly, the regulation effect of 20E on Kr-h1 expressionhas also been observed in other insects, such as T. castaneum (Xu et al., 2018), H. armigera (Zhang W.-N. et al., 2018), and B. mori (Kayukawa et al., 2014; Zhu Z. et al., 2021). In B. mori, the expression patterns of BmKr-h1α/β are closely correlated with the titer of ecdysteroids. Treating ovaries or BmN cells of B. mori with 20E induced the expression of BmKr-h1 (Kayukawa et al., 2014; Zhu Z. et al., 2021). Very recently, two 20E cis-regulatory regions (CRE) in the BmKr-h1 promoter had been identified, which also exist in the promoter region of Kr-h1 genes in other insects, like D. melanogaster, T. castaneum, A. mellifera, A. aegypti, and A. pisum (Zhu Z. et al., 2021). A Kr-h1 regulatory protein named BmKRP had been isolated and demonstrated to be able to bind this CRE. Overexpressing BmKRP enhanced the promoter activity of BmKr-h1, whereas RNAi knocking-down BmKRP decreased the activity. The expression of BmKRP itself was upregulated by 20E through EcR/USP receptor complex interacting with the potential EcRE in BmKRP promoter. So the study indicates that 20E stimulates BmKr-h1 transcription by inducing the expressionof BmKRP which then activates BmKr-h1 by binding the 20E CRE in its promoter (Zhu Z. et al., 2021). However, the regulatory mechanism of 20E-induced Kr-h1 expression in other insects needs to be confirmed in the future.
It is well known that Kr-h1 acts as the JH primary response gene in various insect species. The abundance of Kr-h1 in larval or nymphal stages and the decline of its mRNA levels in pupae or the final nymphal instar coincide well with the JH titer in the hemolymph (Lozano and Belles, 2011; Belles and Santos, 2014; Kayukawa et al., 2014; Jindra, 2019; Truman, 2019; Martin et al., 2021) (Figure 1). Treatment with the JH mimic (JHAM) at the time of onset of metamorphosis or at the end of the penultimate nymphal instar causes rapid and large induction of Kr-h1 transcripts (Minakuchi et al., 2008b; Minakuchi et al., 2009; Konopova et al., 2011). While suppression of JH biosynthesis or JH signaling in the larval stage or adult leads to a decrease of Kr-h1 (Minakuchi et al., 2009; Abdou et al., 2011; Konopova et al., 2011; Smykal et al., 2014a; Smykal et al., 2014b; Daimon et al., 2015; Jindra et al., 2015; Nouzova et al., 2021). Moreover, the JH response elements (JHREs) in the Kr-h1 promoter of several insects have been identified. The JHRE sequences contain a canonical E-box CACGTG or an E-box like motif CACGCG (Kayukawa et al., 2012; Kayukawa et al., 2013; Cui et al., 2014; He et al., 2014), which are the typical binding elements for bHLH-PAS proteins (Kewley et al., 2004). In the presence of JH, the JH receptor Met, belonging to the bHLH-PAS transcriptional family, directly binds to the E box and activates Kr-h1 transcription in a form of associating with another bHLH-PAS transcription factor known as Taiman/AaFISC/TcSRC (Li et al., 2011; Zhang et al., 2011; Kayukawa et al., 2012), which recently has been demonstrated to be a 1:1 heterodimer (Jindra et al., 2021). This activation initially requires Met to enter the nucleus, which is achieved through interaction with the chaperone heat shock protein Hsp83 and the nucleoporin Nup358 (He et al., 2014; He et al., 2017). JH stimulates the chaperone protein Hsp83 interacting with the bHLH and PAS-B domains of Met in the cytoplasm, and then the association between Hsp83 and the tetratricopeptide repeat (TPR) domain of Nup358 facilitates the nuclear transportation of the entire complex through the nuclear pore (He et al., 2014; He et al., 2017). However, recent studies by Jindra et al. (2021) demonstrated that addition of JHM to the Sf9 cell cultures coexpressed with T. castaneum Met and Taiman promoted Met/Taiman dimerization and reduced the interaction between Met and Hsp83. This discrepancy could be attributed to the fact that Jindra et al. (2021) coexpressed Met and Taiman in cells, and the excess Taiman could effectively displace Hsp83 from their overlapping binding sites in the PAS B domain of Met while JH stimulates the interaction of Taiman and Met.
FIGURE 1. Transcriptional regulation of Kr-h1 in holometabolous insects (Top panel) and hemimetabolous insects (bottom panel). The expression pattern of Kr-h1 during insect life history is shown in the purple line. The middle is the regulatory mechanism during each stage of development. The approximate times of 20E pulses and JH levels in holometabolous insects and hemimetabolous insects are shown in red and yellow boxes at the top or bottom of each panel, respectively. Gene expression profiles for Kr-h1 refers to Belles and Santos (2014) and Martin et al. (2021). JH and 20E titers are from Martin et al. (2021).
Besides the intracellular signaling, the studies in A. aegypti showed that JH could activate Kr-h1 expression through a hypothetical cell membrane-associated JH receptor via PLC signaling. This signaling phosphorylates Met and Taiman by a calcium/calmodulin-dependent protein kinase II (CaMKII), which then positively regulates their binding with JHRE, thus activating the target gene expression (Liu et al., 2015). Therefore, both the cell membrane-based and the intracellular nuclear pathways play important roles in JH regulated Kr-h1 expression.
In addition to the hormone-receptor regulation mode, JH could induce Kr-h1 expression through epigenetic modification (Fernandez-Nicolas and Belles, 2017; Roy et al., 2017; Roy and Palli, 2018; George et al., 2019). Studies in B. germanica and T. castaneum had shown that RNAi depletion of CREB-binding protein (CBP), a protein with acetyltransferase activity (HAT), reduces the JH-driven increase of Kr-h1 (Fernandez-Nicolas and Belles, 2017; Roy et al., 2017; Roy and Palli, 2018). And Exposure of T. castaneum cells to Trichostatin A, a histone deacetylase (HDAC) inhibitor, causes an increase in expression of Kr-h1 (Roy and Palli, 2018). These suggest that acetylation and deacetylation mediated by CBP and HDACs play an important role in JH induction of Kr-h1 expression. A recent publication showed that JH suppressed HDAC1, leading to increased levels of histone acetylation and consequently promoting Kr-h1 transcription (George et al., 2019). In the absence of JH, HDAC1 forms a repressor complex with SIN3 and deacetylates histones that are associated with the Kr-h1 promoter and then suppress Kr-h1 expression. When JH is present, the repressor complex is likely replaced by an activator complex which acetylates the core histones at the Kr-h1 promoter and then facilitates Kr-h1 expression (George et al., 2019). Recently, this activator is presumed to be CBP (Gaddelapati et al., 2020).
Another factor that needs to be considered regarding Kr-h1 transcriptional regulation is E93. E93 is a helix-turn-helix transcription factor containing a Pip-squeak (Psq) motif, which was first reported to be involved in the regulation of programmed cell death in D. melanogaster during the prepupal stage (Baehrecke and Thummel, 1995; Lee et al., 2000). The studies by Urena et al. (2014) found that E93 mRNA was expressed in the last nymphal instar of B. germanica or during the entire pupal period in T. castaneum and D. melanogaster in a pattern opposite to that of Kr-h1. RNAi knockdown of E93 prevented the decline of Kr-h1 expression during the final nymphal instar of B. germanica or pupal stage of T. castaneum, even though the decline of JH titer occurred normally. Such observation strongly suggests that E93 is necessary for the downregulation of Kr-h1 at the beginning of the pre-adult stage (Urena et al., 2014). Importantly, these findings have been confirmed in other insects, such as Cimex lectularius (Gujar and Palli, 2016b), N. lugens (Li et al., 2018), and Gryllus bimaculatus (Ishimaru et al., 2019).
Besides the transcriptional regulation, microRNA (miRNA) has emerged as a critical post-transcriptional regulator in Kr-h1 expression. It was initially reported in B. germanica that miR-2 bound the 3′UTR of BgKr-h1mRNA and scavenged Kr-h1 transcripts during the transition from nymph to adult. Depletion of miR-2 prevents the normal elimination of Kr-h1. This study suggests that the abrupt decrease of Kr-h1 mRNA levels in the final nymphal instar results not only from the decline of JH titer but also from the action of miR-2 (Lozano et al., 2015) (Figure 1). However, this miR-2 binding site in the 3′UTR of Kr-h1 mRNA was only found to be conserved in hemimetabolous species. Recently, our studies in D. melanogaster found that DmKr-h1 transcription could also be regulated by miRNA. Two miR-927 binding sites were identified in the 3′UTR of DmKr-h1 mRNA, overexpression of miR-927 decreased the transcriptional levels of DmKr-h1 and resulted in higher lethality during embryogenesis and metamorphosis (He et al., 2020), which was similar to DmKr-h1 mutants (Pecasse et al., 2000). Moreover, the expression of miR-927 was found to be repressed by JH, thus forming a positive regulatory loop (He et al., 2020). Similarly, the JH/miRNA/Kr-h1 regulatory axis has also been reported in L. migratoria, where let-7 and miR-278 downregulate LmKr-h1 and JH represses the expression of these two miRNAs (Song et al., 2018). This suggests that the cooperation between hormones and miRNAs ensures the proper regulation of Kr-h1 expression, and the regulatory axis is conserved during insect evolution.
As stated above, the general expression pattern of Kr-h1 in hemimetabolous and holometabolous insect development is coordinatedly regulated by the hormones, miRNA, and E93, which could be summarized as follows. 1) in the early juvenile stages, the continuous presence of JH and the low levels of miRNA maintain high expression levels of Kr-h1. 2) in the final larval stage of holometabolans, the decrease of JH and the increased expression of miRNA lead to the downregulation of Kr-h1. 3) at the prepupal stage or the mid-late stage of the penultimate nymphal instar, a surge of 20E and JH gives rise to the transient peak of Kr-h1; 4) upon pupation or ecdysis to the final nymphal instar, gradually decreasing JH and increasing levels of E93 and miRNA lead to the disappearance of Kr-h1 (Figure 1).
It has been well documented that Kr-h1 functions as a transducer of the antimetamorphic action of JH in both hemimetabolous and holometabolous insects. JH precludes insect metamorphosis during the pre-ultimate immature stages, its disappearance in the final instar then permits a metamorphic molt that transforms larvae to adults directly (hemimetaboly) or via a pupal stage (holometaboly) (Riddiford, 1994; Belles, 2019; Jindra, 2019; Truman, 2019; Belles, 2020a; Martin et al., 2021). In most insects except for the higher Diptera such as D. melanogaster and A. aegypti where the “status quo” action of JH is subtle (Liu et al., 2009; Riddiford et al., 2010; Abdou et al., 2011), administration of JH or JHM to the final-instar nymphs, larvae, or pupae leads to repetition of that stage (Riddiford, 1994; Minakuchi et al., 2009; Konopova et al., 2011; Jindra et al., 2013). Conversely, experimental removal of JH at earlier instars causes a precocious metamorphic molt, manifested by heterochronic development of adult characters such as wings and external genitals or formation of miniature pupae in holometabolans (Tan et al., 2005; Minakuchi et al., 2008a; Daimon et al., 2012; Daimon et al., 2015). Regardless of the distinct metamorphic responses to JH among species, exogenous JHM treatment or depletion of JH could increased or decreased the expression of Kr-h1, respectively, in the studied insects (Minakuchi et al., 2008a; Minakuchi et al., 2009; Konopova et al., 2011; Smykal et al., 2014b; Daimon et al., 2015), even in D. melanogaster and A. aegypti (Minakuchi et al., 2008b; Abdou et al., 2011; Nouzova et al., 2021). Moreover, the “status quo” action of JH can be counteracted when the Kr-h1 expression is blocked. Depletion of Kr-h1 by RNAi before the JHM treatment at the pupal stage or the final-instar nymphal stage leads to normal adult development instead of repeating the pupal or larval program (Minakuchi et al., 2009; Konopova et al., 2011). Additionally, RNAi knockdown of Kr-h1 at juvenile stages, such as the fourth instar in T. castaneum (Minakuchi et al., 2009), antepenultimate or penultimate instar in B. germanica (Lozano and Belles, 2011) or penultimate instar in P. apterus (Konopova et al., 2011; Smykal et al., 2014b) induces precocious pupation or premature adult development. Meanwhile, as mentioned above, a transient peak of Kr-h1 at the prepupal stage of holometabolous insects, which may be regulated by a combination of JH and 20E, has been demonstrated to be essential for the formation of the pupa (Urena et al., 2016). Depletion of this specific Kr-h1 peak triggers a direct transformation of the larva into adult form, bypassing the pupal stage (Urena et al., 2016). On the other hand, studies in B. mori showed that constitutive overexpression of Kr-h1 caused prepupal arrest with unusually thin-skinned cocoon (Kayukawa et al., 2014). In D. melanogaster, ectopic expression of Kr-h1 in the histoblast during metamorphosis blocks normal differentiation of the abdominal epidermis and causes missing or short dorsal midline bristles in the adult, a phenotype that resembles the effect of exogenous JH treatment (Minakuchi et al., 2008b). In summary, all these data demonstrate that Kr-h1 functions as a universal JH-dependent repressor of metamorphosis in insects undergoing both holometaboly and hemimetaboly.
Regarding the molecular mechanism by which Kr-h1 mediates the anti-metamorphic effect of JH, various results show that it mainly depends on the direct repression of the expression of the pupal specifier gene Broad-Complex (Br-C) and the adult specifier gene Ecdysone induced protein 93F (E93) (Figure 2). The role of Br-C as the pupal specifier has been extensively confirmed in the holometabolan species. For example, in D. melanogaster, Br-C null mutation results in death at the time of pupariation (Kiss et al., 1976; Kiss et al., 1988), and in other insects RNAi depletion of Br-C results in the failure of larvae to undergo normal pupal transition or a mix feature of larva, pupa and adult (Zhou and Riddiford, 2002; Uhlirova et al., 2003; Konopova and Jindra, 2008; Parthasarathy et al., 2008; Suzuki et al., 2008; Ding et al., 2020). JH prevents pupation by restricting Br-C expression in young larvae until the onset of the final larval instar when the decline of JH allows the induction of Br-C by 20E, which then triggers the pupal formation. Removal of the JH-producing corpora allata glands causes ectopic Br-C induction as well as precocious pupation, whereas exposure of larvae to JH prevents both Br-C transcription and pupation (Zhou et al., 1998; Reza et al., 2004). Likewise, Kr-h1 mutations result in precocious Br-C expression, and the precocious expression could not be suppressed by exogenous JHM (Huang et al., 2011). This indicates that Kr-h1 mediates the JH-inhibited precocious Br-C expression during larval stages.
FIGURE 2. Molecular mechanisms of the anti-metamorphic action of Kr-h1. JH prevents insect metamorphosis by activating Kr-h1 expression. Kr-h1 binds directly to the KBS in the promoters of pupal specifier gene Br-C or adult specifier gene E93 to inhibit their expression, which in turn prevent pupal metamorphosis or adult metamorphosis. This action requires the phosphorylation of Kr-h1 and the recruitment of cofactors. Kr-h1 could also prevent metamorphosis via repressing the expression of enzymes involved in 20E biosynthesis, such as Spok. The binding of Kr-h1 to the KBS in the promoter of Spok induces the adjacent DNA methylation by unknown factors, which leads to the repression of Spok expression. Whether this action depends on the phosphorylation of Kr-h1and the association with cofactors is unknown. KBS, Kr-h1 binding site; p, phosphorylation modification; c, cytosine; PG, prothoracic gland.
The conception that E93 functions as a universal adult specifier in both hemimetabolous and holometabolous insects is afforded by the research of Urena et al. The authors found that E93 is highly upregulated during the final nymphal instar or the entire pupal period. RNAi depletion of E93 in the penultimate nymphs of B. germanicaor mid final instar larvaeof T. castaneum prevents the nymphal-adult or pupal-adult metamorphic transition, inducing multiple reiterations of nymphal molts or producing a supernumerary second pupa, even in the absence of JH (Urena et al., 2014). At the same time, Belles and Santos (2014) found that Kr-h1 repressed E93 expression in B. germanica, and later, Urena et al. (2016), confirmed the same interaction in T. castaneum, thus indicating that repression of Kr-h1 upon E93 prevented the precocious adult metamorphosis. In the same way, Urena et al. (2016) used RNAi experiment to deplete the transient peak of Kr-h1 expression at the prepupal stage in T. castaneum and found that this led to a precocious upregulation of E93 and hence to a direct transformation of the larva into the adult, bypassing the pupal stage (Urena et al., 2016). Thus, the MEKRE93 pathway which comprises Met, Kr-h1, and E93 is the essential axis regulating adult morphogenesis (Belles and Santos, 2014; Belles, 2020b). Subsequent research in Shinoda lab elucidated that Kr-h1 mediated Br-C and E93 transcriptional suppression via directly binding to the Kr-h1 binding sites (KBS, TGACCTNNNNYAAC) in their promoters (Figure 2). When KBS was deleted from the promoter, the repression was abolished (Kayukawa et al., 2016; Kayukawa et al., 2017). Thus Kr-h1 prevents precocious metamorphosis of juvenile insects to adults by directly repressing the transcriptional expression of Br-C and E93.
Remarkably, it is paradoxical that the repression of Kr-h1 on Br-C in larvae seems not to operate during the larva-pupa transition as the pulse of Br-C parallels that of Kr-h1, and it is strongly suppressed after Kr-h1 RNAi, as shown in T. castaneum (Urena et al., 2016). Consistently, overexpression of Kr-h1 in B. mori during this stage does not prevent the Br-C pulse (Kayukawa et al., 2014). Furthermore, exogenous JHM treatment in the prepupae of D. melanogaster and T. castaneum induces both Kr-h1 and Br-C expression (Minakuchi et al., 2008b; Minakuchi et al., 2009). One explanation is that the pulse of Kr-h1 during the prepupal stage inhibits the upregulation of E93, which is also a strong repressor of Br-C. So the induction effect of Kr-h1 on Br-C during the prepupal stage may result from the repression of E93. This explanation seems to be plausible. However, the expression of E93 is always downregulated in juvenile stages. Thus according to this explanation, Kr-h1 should also stimulate Br-C expression in early larval stages, which is obviously not the case. Another possibility might be that the stage-specific regulatory effect of Kr-h1 on Br-C results from differences in the cell-autonomous factors at each stage, such as different cofactors (co-repressor/co-activator), epigenetic modification of the promoter, or post-translational modification of Kr-h1. A study in mosquitoes showed that Kr-h1 acts either as a transcriptional activator or a repressor in female reproduction through directly binding to the regulatory regions of its target genes in a promoter-specific manner (Ojani et al., 2018). Recently, Wu et al. (2021) demonstrated that Kr-h1 recruited different cofactors to function as activators and repressors at distinct developmental stages. During the juvenile stage, Kr-h1 recruits a corepressor called C-terminal binding protein (CtBP) to repress the transcription of E93 and prevent adult formation. While in the adult stage, Kr-h1 recruits the coactivator CBP to promote vitellogenesis, and the phosphorylation status of Kr-h1 is indispensable for the cofactors recruitment (Figure 2). Nevertheless, more research is still needed to gain a unified understanding of the stage-specific regulatory effect of Kr-h1 on Br-C.
Besides antagonizing ecdysone action through decreasing the expression of Br-C and E93 to mediate the anti-metamorphic action of JH, Kr-h1 could also inhibit ecdysone biosynthesis in the prothoracic gland by directly suppressing the expression of steroidogenic enzyme genes (Zhang T. et al., 2018; Liu et al., 2018). Kr-h1 binds to the KBSs in the promoter of Spok which encodes a rate-limiting enzyme involved in ecdysteriodogenesis and then induces DNA methylation at cytosines around the KBS. This action in turn inhibits transcription of Spok and negatively regulates ecdysone biosynthesis (Figure 2). Hence, Kr-h1 transduces the anti-metamorphic action of JH through repressing both 20E signaling and 20E biosynthesis (Figure 2).
In addition to repressing metamorphosis, Kr-h1 has also been found to function in female reproduction. In most insects, vitellogenesis is the central event of female reproduction, which involves the production and secretion of vitellogenin (Vg) by the fat body, followed by transportation of Vg through the intercellular spaces in the follicular epithelium (known as patency) and subsequent uptake by maturing oocytes via Vg receptor-mediated endocytosis (Valle, 1993; Roy et al., 2018; Santos et al., 2019; Wu et al., 2020). It has been well established that insect female reproduction is coordinately regulated by JH, 20E, nutrients, and miRNA (Roy et al., 2018; Wu et al., 2020). In hemimetabolous and basal holometabolous insects, JH is the main hormone that stimulates vitellogenesis, while 20E takes over the role of the leading hormonal regulator among the insect orders of Diptera, some hymenopterans, and lepidopterans (Raikhel and Dhadialla, 1992; Valle, 1993; Wyatt and Davey, 1996; Roy et al., 2018; Santos et al., 2019). As mentioned above, Kr-h1 is regulated by both JH and 20E. Hence, Kr-h1 is also involved in female reproduction. However, the role of Kr-h1 in insect vitellogenesis varies across species. In L. migratoria, Kr-h1 RNAi prevents JH-induced Vg expression in the fat body along with blocked oocyte maturation and arrested ovarian development. Meanwhile, the JH-induced intercellular space in the follicular epithelium fails to occur after Kr-h1 depletion. Kr-h1 RNAi blocks lipid accumulation in the primary oocytes (Song et al., 2014). Similarly, Kr-h1 knockdown leads to reduction of Vg transcript levels and impaired oocyte maturation in H. armigera (Zhang W.-N. et al., 2018), B. dorsalis (Yue et al., 2018), C. suppressalis (Tang et al., 2020), S. furcifera (Hu et al., 2020), C. bowringi (Guo et al., 2021) and H. axyridis (Han et al., 2022). However, in the hemipteran linden bug P. apterus, RNAi knockdown of Kr-h1 neither reduced Vg expression nor affected ovarian development, whereas JH deprivation or knockdown of Met or Tai did (Smykal et al., 2014a). Likewise, in the common bed bug C. lectularius, knockdown of Kr-h1 did not affect Vg synthesis and fecundity (Gujar and Palli, 2016a). This suggests that in the true bugs (Heteroptera) other transcriptional factors might mediate JH-regulated vitellogenesis. Studies in the coleopteran T. castaneum showed that RNAi depletion of Kr-h1 only resulted in about 30% reduction in Vg mRNA levels while knocking down the expression of JHAMT, a key enzyme involved in JH biosynthesis, or Met caused about 80–90% reduction (Parthasarathy et al., 2010). Later studies demonstrated that JH functions through an insulin-like peptide (ILP) signaling pathway to stimulate Vg production (Sheng et al., 2011). Application of JH to previtellogenic female beetles induced the expression of ILP2 and ILP3 which acts through the insulin-like peptide signaling pathway to phosphorylate FOXO, leading to its export from the nucleus, thus triggering the expression of Vg. Knockdown of JHAMT or Met decreased ILP expression and induced FOXO nuclear localization, resulting in the binding of FOXO to the FOXO response element within the Vg promoter, thus repressing Vg synthesis (Sheng et al., 2011). This research indicates that in T. castaneum, JH stimulates Vg synthesis through inducing ILP expression and activating the ILP signaling pathway, but whether this mechanism is conserved among insects where vitellogenesis is regulated by JH remains to be confirmed. The role of Kr-h1 in this regulatory pathway remains unclear.
In the dipterans such as D. melanogaster and Ae. aegypti, Vg expression is mainly induced by 20E (Bownes, 1982; Valle, 1993). In Ae. aegypti, Vg synthesis in the fat body is stimulated by a blood meal which triggers the release of the ovarian ecdysteroidogenic hormone (OEH) from the medial neurosecretory cells of the brain. The OEH activates follicular cells of the ovary to produce ecdysone, which is converted into the active 20E in the fat body. 20E then stimulates Vg expression via the ecdysone pathway. The 5′ upstream regulatory region of the mosquito Vg contains several binding sites for EcR-USP and the early and early-late 20E-inducible genes, such as E74B, E75A, Br-C-Z2, βFTZ-F1, and HR3, which indicates a combinatorial control of Vg expression by 20E (Kokoza et al., 2001; Martin et al., 2001; Chen et al., 2004; Sun et al., 2004; Zhu et al., 2006; Cruz et al., 2012; Mane-Padros et al., 2012). The role of JH in mosquito reproduction is to make the fat body competent for Vg synthesis during the previtellogenic stage. The translation of the competence factor βFTZ-F1 that potentiates the activation of 20E-responsive genes is controlled by JH (Zhu et al., 2003; Zhu et al., 2006). Neither blood meal nor 20E treatment can induce the expression of Vg when βFTZ-F1 is depleted (Zhu et al., 2003). Besides, JH acts via Met to activate ribosomal biogenesis in the fat body to facilitate the massive production of Vg (Wang et al., 2017). Kr-h1 functions as a transcriptional activator or a repressor in JH/Met-regulated female mosquito reproduction through directly binding to the regulatory regions of target genes (Ojani et al., 2018). RNAi depletion of Kr-h1 remarkably reduces the length of primary follicles and egg production after blood feeding (Ojani et al., 2018; Saha et al., 2019). Additionally, Kr-h1 could act synergistically with Hairy in the repression of JH/Met target genes during previtellogenic development. Knockdown of Kr-h1 and Hairy resulted in overlapping changes at the transcriptomes level. Only a simultaneous knockdown of Kr-h1 and Hairy could fully phenocopy the effect of Met RNAi (Saha et al., 2019). Recently, in the brown planthopper N. lugens, it has been demonstrated that the N-terminal zinc-finger domains of Kr-h1 interact directly with Hairy in modulating gene transcription (Mao et al., 2020).
In D. melanogaster, it has been well documented that 20E is responsible for oogenesis and the high rate of Vg synthesis in the fat body and JH is essential for the uptake of Vg into oocytes from the hemolymph (Bownes, 1982; Soller et al., 1999). However, JH signaling is also involved in oogenesis. The decreased egg-laying rate was observed in Met or Gce null mutants and JH deficient flies (Abdou et al., 2011), which later was found to be caused by the accumulation of mature eggs in the ovary (Luo et al., 2021). Using the JHRR-LacZ which recapitulates the responsiveness of Kr-h1 to JH and Met/Gce as an activity indicator of JH signaling (He et al., 2014), Luo et al. (2021) found that JH signaling was activated in ovarian muscle cells and adult fat body. RNAi knockdown of Met/Gce or Kr-h1 in ovaries or adult fat body significantly decreased fecundity, with reduced oviposition, increased ovary size, and accumulation of mature eggs in the ovary, along with reduced egg length. Meanwhile, the expression levels of laminin and collagen IV that participate in the assembly of ovarian muscle extracellular matrix (ECM) were strongly decreased in the JH signaling-deficient flies. The ECM components are indispensable for ovarian muscle contraction that eventually generates a mechanical force. Therefore, JH signaling promotes ovulation and maintains egg shape by inducing the expression of ECM genes (Luo et al., 2021). Besides, the authors demonstrated that JH signaling could directly regulate germline stem cells (GSCs) maintenance in the ovary. RNAi knockdown of Met and Gce or Kr-h1 targeted to the cap cells of the ovary led to significantly declined numbers of GSCs (Luo et al., 2020). These experiments indicate that the JH/Met/Kr-h1 signaling also functions in D. melanogaster oogenesis.
In lepidopterans, hormone control of reproduction appears to have evolved independently in different lineages. For example, in H. armigera and Manduca sexta, which synthesize Vg in adults, JH plays a significant role in regulating female reproduction, whereas in B. mori, where vitellogenesis proceeds before adult emergence in the absence of JH, 20E is the essential hormone controlling egg development (Roy et al., 2018; Khalid et al., 2021). Recent findings in B. mori showed that Kr-h1 was highly expressed in ovaries during the late pupal and adult stages and the expression was induced by 20E (Zhu Z. et al., 2021). RNAi-mediated depletion of Kr-h1 in female pupae during the late pupal stage resulted in abnormal oocytes with less yolk protein deposition and partially transparent chorion. Kr-h1 exerts this function through upregulating the expression of the Vg receptor which affects the yolk protein absorption process, or probably via activating the transcription factorAp-1and FOXG1 which then regulate genes involved in the metabolic-related pathways and the nutrient absorption process to modulate the oocyte maturation (Zhu Z. et al., 2021; Zhu Z. D. et al., 2021).
In summary, among insect orders, the female reproduction is coregulated by a complex interaction between JH, 20E, and nutritional signaling pathways, and Kr-h1 plays crucial roles among these regulatory pathways.
It is interesting to note that Kr-h1 functions as a transcriptional repressor in insect metamorphosis and switches as an activator in adult reproduction. As mentioned previously, the studies by Wu et al.(2021)demonstrated that the dual functions of Kr-h1 depend on the property of the recruited cofactors. The high expression levels of the corepressor CtBP and coactivator CBP in the juvenile stage and adult stage, respectively, might explain the distinct Kr-h1 complexes forming in these two stages. However, whether other factors are involved in stimulating the specific complexes remains to be investigated.
In addition to the roles in insect metamorphosis and reproduction, Kr-h1 is also necessary for many other aspects of insect development, such as neuron fate specification and morphogenesis (Shi et al., 2007; Hewes, 2008; Fichelson et al., 2012; Marchetti and Tavosanis, 2019), social hierarchy and behavioral plasticity (Whitfield et al., 2003; Grozinger and Robinson, 2007; Shpigler et al., 2010; Gospocic et al., 2021), maturation of sexual behavior (Duportets et al., 2012; Gassias et al., 2021), embryogenesis (Fernandez-Nicolas and Belles, 2017) and metabolic homeostasis (Kang et al., 2017). Most of these physiological processes are also regulated by JH and 20E signaling.
In D. melanogaster, Kr-h1 is downregulated in mushroom body (MB) neurons both at the time of initial morphological differentiation and during metamorphic neurite remodeling. Overexpression of Kr-h1 in developing MB neurons inhibits axon remodeling and blocks MB neuron morphogenesis (Shi et al., 2007). During development, four major types of MB neurons (γ, α'β', pioneer αβ and αβ) are sequentially produced, with γ, α'β' neurons arising during early larval stages or late larval stages respectively, and pioneer αβ and αβ are generated during early metamorphosis (Lee et al., 1999). TGF-β signaling promotes the expression of EcR-B1, which is required in MB neuronal progenitors to consolidate the α'β' fate. Kr-h1 antagonizes the effect of TGF-β signaling, leading to decreased levels of EcR-B1 and subsequently a significant reduction in the number of α'β' neurons. This suggests that Kr-h1 negatively modulates the 20E signaling response to maintain the correct MB neuronal identity (Marchetti and Tavosanis, 2019). Similarly, during D. melanogaster photoreceptor maturation, transient repression of Kr-h1 by the conserved homeobox protein Orthodenticle (Otd) and EcR is required to enable neuronal maturation to proceed normally (Fichelson et al., 2012). Thus, Kr-h1 appears to exhibit an antimorphogenetic activity in developing and remodeling neurons. However, in the honey bee, the increased expression levels of Kr-h1 accompany the neurite outgrowth and synapse formation in the MB (Grozinger et al., 2003; Whitfield et al., 2003), which indicates that Kr-h1 acts as a positive regulator in honey bee neurite outgrowth.
Behavioral plasticity is key to animal survival. Social insects provide an excellent model system for this study. The adult honey bee possesses a remarkable trait that is the behavioral transition from inhive tasks to outside tasks. Upon emergence, worker honey bees begin the task of “nursing” behavior, where they are responsible for the feeding and care of the queen and brood. Approximately 1 week later, they change to new roles, such as storing and processing food; when they are 3 weeks of age, worker bees begin to forage fornectar, pollenor water outside of the hive (Robinson, 1992). Increased levels of Kr-h1 in the brain are associated with the behavior transition from nursing to foraging. The expression levels of Kr-h1 are found to be significantly higher in foragers than in nurses and all other behavioral groups (Whitfield et al., 2003; Grozinger and Robinson, 2007). The factors that regulate Kr-h1 expression, such as age, JH, and brain octopamine which could accelerate the transition to foraging, have been extensively examined. But the results show that Kr-h1 expression is not regulated by any of these factors, although JH reduces the effect of queen mandibular pheromone on decreasing Kr-h1 expression (Grozinger et al., 2003; Grozinger and Robinson, 2007). Later, using behavioral manipulations and pharmacological treatments, Fussnecker and Grozinger demonstrated that high Kr-h1 levels in foragers resulted from the cGMP-mediated physiological changes in the brain that occurred early in the transition, and the Kr-h1 promoter contained a conserved potential cGMP response element (Fussnecker and Grozinger, 2008). However, in contrast to the honey bee, bumble bee foragers do not have higher Kr-h1 levels relative to nurses. Instead, in bumble bees, Kr-h1 is associated with JH-mediated division of worker reproduction and dominance rank (Shpigler et al., 2010; Pandey and Bloch, 2021). Bumble bees are primitively eusocial insects that there are no obvious morphological differences between the queen and the workers. Direct contact with the queen is required to inhibit worker reproduction (Breed, 1976; Brown et al., 2003). However, JH treatment of workers causes a dose-dependent increase in oocyte length, even in the presence of the inhibitory queen, but does not affect worker task. High levels of JH are associated with worker ovary activation and reproduction, and high dominance rank in queenless workers. An individual with reduced JH levels is less likely to acquire a high dominance rank in queenless workers, and this effect is fully reverted by topical treatment with JH (Bloch et al., 2000; Pandey et al., 2020). Thus, in bumble bee, JH influences reproduction as well as other behaviors related to dominance. Kr-h1 levels are found to be higher in dominant workers with active ovaries relative to subordinated individuals with undeveloped ovaries in queenless groups (Shpigler et al., 2010). Knockdown Kr-h1 expression in bumble bees by a perfluorocarbon nanoparticles-based RNA interference protocol showed that bees with reduced Kr-h1 transcript abundance exhibited significantly reduced ovarian activity, less dominance behavior, and had lower dominance rank (Pandey and Bloch, 2021). Therefore, Kr-h1 is associated with JH-mediated regulation of social organization in both bees, from labor division in honey bees to reproduction division and dominance rank in bumble bees. As a transcription factor, the target genes of Kr-h1 involved in these processes need to beidentified in the future.
Recently, in the ant Harpegnathos saltator, Kr-h1 has been demonstrated to regulate caste identity by acting as a core transcriptional regulator in response to the caste-specific JH and 20E signaling (Gospocic et al., 2021). H. saltator ants can switch between worker and queen-like status. When the queen dies or is removed from a colony, workers enter a dueling tournament until a few become reproductive individuals that are called gamergates (Sasaki et al., 2016). The gamergate abandons worker tasks such as hunting, begins to lay eggs, and exhibits dominant behaviors toward workers. This behavioral transition is accompanied by a reconfiguration of gene expression and cellular composition of the brain, and neurohormonal changes, such as the levels of JH3 and 20E (Penick et al., 2011; Sheng et al., 2020). By analyzing the transcriptional and behavioral response to JH3 and 20E in vivo and in vitro, the authors concluded that JH3 and 20E were responsible for the caste identities of workers and gamergates respectively and that Kr-h1 was required to maintain the caste boundaries (Gospocic et al., 2021). ChIP-seq and RNA-seq analyses demonstrated that Kr-h1 functioned as a caste-specific transcriptional repressor by repressing “socially inappropriate” patterns of gene expression in the brain. In workers, Kr-h1 is induced by JH3 and downregulates gamergate-biased genes. In gamergates, Kr-h1 is downstream of 20E signaling and is involved in maintaining gamergate identity through the repression of worker-biased genes. Knockdown of Kr-h1 in workers stimulated expression of gamergate-biased genes and inhibited hunting, whereas loss of Kr-h1 in gamergates led to upregulation of worker-biased genes and promoted hunting (Gospocic et al., 2021).
Besides regulating behavioral plasticity in females, Kr-h1 is also involved in the maturation of male sexual behavior and male accessory glands (MAGs). In the noctuid moth, Agrotis ipsilon, newly emerged males are sexually immature and unable to behaviorally respond to the female-produced sex pheromone, and theirMAGs are undifferentiated and have a low protein content. Three to five days after emergence, males become sexually mature and highly attracted to the female sex pheromone; their MAGs exhibit elevated protein synthesis. This increase in behavioral responsiveness is well known to be JH-dependent (Duportets et al., 1998; Anton and Gadenne, 1999). Removal of the JH-producing CA induces a co-inhibition of the sex pheromone-triggered orientation flight and the MAG protein synthesis, which can be subsequently restored if operated males are injected with JH (Anton and Gadenne, 1999). The expression levels of Kr-h1 and Met in A. ipsilon males are predominant in the antennal lobe and MAGs, wheretheir amount increased concomitantly with age, in parallel with sex pheromone responses and maturation of the MAGs (Duportets et al., 2012; Gassias et al., 2021). RNAi experiments show that males injected with either Met or Kr-h1 dsRNA exhibited a significantly reduced MAG length and protein content (Gassias et al., 2021). Thus, Kr-h1 and Met are critical for modulating JH-regulated male reproductive maturation.
The initial studies on the role of Kr-h1 in insect embryogenesis were conducted by Pecasse et al. (2000) and Beck et al. (2004) in D. melanogaster. Two deletion mutants (Df(2L) Kr-h17.1 and Kr-h17id), obtained by the mobilization of the P element in one of the Kr-h1 mutations (Kr-h17), that removed coding sequences common to both DmKr-h1α and DmKr-h1β rarely eclosed as larvae. However, the mutants showed normal embryonic development and elaborated larval structures by the end of embryogenesis, with occasional ectopic neurons (Pecasse et al., 2000; Beck et al., 2004). Later, in B. mori, Daimon et al. (2015) used the genome-editing tool TALENs (targeted mutagenesis mediated by transcription activator-like effector nucleases) to generate knockout silkworms with null mutations in JHAMT or Met. The transcriptional levels of Kr-h1 were reduced dramatically in both mutants during the embryonic stage. Phenotypes examination found that the JH-deficient or JH signaling-deficient mutants showed high lethality in embryos and delayed embryonic development. Similar to the findings in D. melanogaster, embryogenesis appeared to be completed in the unhatched embryos, which were able to emerge as apparently normal first instar larvae if they were artificially dechorionated (Daimon et al., 2015). These results suggest that in the holometabolan, JH or JH signaling (JH-Met-Kr-h1) does not have a morphogenetic role in embryogenesis. On the contrary, studies by Fernandez-Nicolas and Belles (2017) in B. germanica showed that JH and its signaling have relevant functions during cockroach embryo development. Knockdown of Kr-h1, JHAMT, or Met by maternal RNAi technique not only caused impaired hatchability of embryos but also resulted in morphological malformations, such as failure formation of the germ-band anlage, imperfectly sealed dorsal closure, reduced abdomen, and intensely sclerotized cuticle (Fernandez-Nicolas and Belles, 2017). Therefore, involvement of JH and JH signaling in hatching appears to be conserved in hemimetabolans and holometabolans, whereas the morphogenetic functions were lost in holometabolan embryos. It is presumed that Br-C might be an important factor accounting for this distinction. In the B. germanica embryo, Br-C is consistently expressed during the period of JH production (Piulachs et al., 2010), its expression is reduced after JH or JH signaling is depleted (Fernandez-Nicolas and Belles, 2017). And the phenotypes observed in JH or Kr-h1 depleted embryos of B. germanica are similar to those found in Br-C depleted embryos (Piulachs et al., 2010). On the contrary, in B. mori embryos, Br-C mRNA showed low levels during the cycle of JH production (Daimon et al., 2015). So it is supposed that the expression of Br-C would be induced prematurely in JH-deficient or JH signaling-deficient mutant Bombyx larvae. However, the Br-C expression levels remained very low in these mutations during embryonic development (Daimon et al., 2015). Even so, whether Br-C is the crucial factor determining the different functions of JH and JH signaling (JH-Met-Kr-h1) in embryogenesis between hemimetabolans and holometabolans remains to be investigated.
Metabolic homeostasis plays important roles in insect development. Kr-h1 has also been reported to control lipid metabolism. Studies in D. melanogaster found that Kr-h1 mutations showed delayed larval development and reduced triglyceride (Kang et al., 2017). Under normal fed condition, Kr-h1 acted on lipogenesis, whereas upon fasting, Kr-h1 regulated lipolysis. Further, the authors demonstrated that insulin signaling was inhibited in Kr-h1 mutant larvae and Kr-h1 could physically and genetically interact with FOXO to repress the transcriptional activity of FOXO on adipose lipase brummer (Kang et al., 2017). Previous studies by Mirth et al. (2014) showed that JH deficient Drosophila larvae pupariated at smaller size and FOXO is necessary for the size reduction. The co-regulation of lipid metabolism by Kr-h1 and FOXO contributes to a novel mechanism through which JH interacts with insulin signaling to integrate metabolism and growth during larval development.
Kr-h1 is a conserved transcription factor among insect orders. It acts as a cornestone regulator in insect life, from preventing metamorphosis to promoting female reproduction. In addition, it modulates social insects’ caste identity and behavior plasticity and the associated neuronal morphogenesis. Hence, Kr-h1 could be a promising target in pest control in the future. However, despite the rapidly mounting evidence for the molecular and developmental functions of Kr-h1, many outstanding questions still need to be answered. The most pressing issues are: 1) What is the precise mechanism of Kr-h1 acting as both transcriptional repressor and activator? Is it dependent on the recruited cofactors? And what are these cofactors? So far, interactions of Kr-h1 with the coactivator CBP and the corepressor CtBP important for activating vitellogenesis and preventing metamorphosis have thus far been studied in L. migratoria. As Kr-h1 plays many roles in insect life, we speculate that Kr-h1 interacts with multiple distinct partners, each time regulating a different aspect of insect biology. But how does Kr-h1 discriminate against these partners at different developmental stages? And how is their interaction stimulated? Moreover, besides phosphorylation, whether other post-translational modifications affect Kr-h1 binding with these cofactors? Does the opposite stage-specific regulatory effect of Kr-h1 on Br-C depend on the property of partners or the post-translational modification of Kr-h1? In addition, Krüppel-like transcription factors can epigenetically induce DNA methylation or demethylation of the promoters of their target genes to inhibit or promote their transcription respectively. Does Kr-h1 exert similar functions? If so, what are the DNA methyltransferases and demethylase? 2) The functions of the eight zinc finger domains need to be dissected. Do all of them engage DNA? or which of them are involved in DNA binding and protein interaction? Do different zinc fingers recognize specific DNA sequences? 3) Apart from the already known functions, is Kr-h1 involved in other JH or 20E-regulated physiologies, such as spermatogenesis, testicular development, female mating, and sex pheromone production?In mammals, Krüppel-like transcription factors are critical in many physiological and pathological processes including cell proliferation, differentiation, inflammation, and apoptosis. Does Kr-h1 perform similar functions in insects? With the advent of new genomic technologies and the capacity of using different insect species, we will be in an excellent situation to deepen our understanding of the functions of Kr-h1 and properly exploit it as the target of pest control.
QH and YZ collected the references. QH and YZ wrote the manuscript. All authors have read and agreed to the published version of the manuscript.
This work was supported by the National Natural Science Foundation of China (31970465); University Nursing Program for Young Scholars with Creative Talents in Heilongjiang Province (UNPYSCT-2018080) and Scientific Research Foundation for Postdoctors in Heilongjiang Province of China (LBH-Q19165).
The authors declare that the research was conducted in the absence of any commercial or financial relationships that could be construed as a potential conflict of interest.
All claims expressed in this article are solely those of the authors and do not necessarily represent those of their affiliated organizations, or those of the publisher, the editors and the reviewers. Any product that may be evaluated in this article, or claim that may be made by its manufacturer, is not guaranteed or endorsed by the publisher.
Abdou M. A., He Q., Wen D., Zyaan O., Wang J., Xu J., et al. (2011). Drosophila Met and Gce Are Partially Redundant in Transducing Juvenile Hormone Action. Insect Biochem. Mol. Biol. 41 (12), 938–945. doi:10.1016/j.ibmb.2011.09.003
Anton S., Gadenne C. (1999). Effect of Juvenile Hormone on the central Nervous Processing of Sex Pheromone in an Insect. Proc. Natl. Acad. Sci. U.S.A. 96 (10), 5764–5767. doi:10.1073/pnas.96.10.5764
Baehrecke E. H., Thummel C. S. (1995). The Drosophila E93 Gene from the 93F Early Puff Displays Stage- and Tissue-specific Regulation by 20-hydroxyecdysone. Developmental Biol. 171 (1), 85–97. doi:10.1006/dbio.1995.1262
Beck Y., Dauer C., Richards G. (2005). Dynamic Localisation of KR-H during an Ecdysone Response in Drosophila. Gene Expr. Patterns 5 (3), 403–409. doi:10.1016/j.modgep.2004.09.008
Beck Y., Pecasse F., Richards G. (2004). Krüppel-homolog Is Essential for the Coordination of Regulatory Gene Hierarchies in Early Drosophila Development. Developmental Biol. 268 (1), 64–75. doi:10.1016/j.ydbio.2003.12.017
Beckstead R. B., Lam G., Thummel C. S. (2005). The Genomic Response to 20-hydroxyecdysone at the Onset of Drosophila Metamorphosis. Genome Biol. 6 (12), R99. doi:10.1186/gb-2005-6-12-r99
Belles X. (2020). Insect Metamorphosis. From Natural History to Regulation of Development and Evolution. London: Academic Press.
Belles X. (2020). Krüppel Homolog 1 and E93: The Doorkeeper and the Key to Insect Metamorphosis. Arch. Insect Biochem. Physiol. 103 (3), e21609. doi:10.1002/arch.21609
Belles X., Santos C. G. (2014). The MEKRE93 (Methoprene Tolerant-Krüppel Homolog 1-E93) Pathway in the Regulation of Insect Metamorphosis, and the Homology of the Pupal Stage. Insect Biochem. Mol. Biol. 52, 60–68. doi:10.1016/j.ibmb.2014.06.009
Belles X. (2019). The Innovation of the Final Moult and the Origin of Insect Metamorphosis. Phil. Trans. R. Soc. B 374 (1783), 20180415. doi:10.1098/rstb.2018.0415
Bloch G., Borst D. W., Huang Z.-Y., Robinson G. E., Cnaani J., Hefetz A. (2000). Juvenile Hormone Titers, Juvenile Hormone Biosynthesis, Ovarian Development and Social Environment in Bombus terrestris. J. Insect Physiol. 46 (1), 47–57. doi:10.1016/s0022-1910(99)00101-8
Bownes M. (1982). Hormonal and Genetic Regulation of Vitellogenesis in Drosophila. Q. Rev. Biol. 57 (3), 247–274. doi:10.1086/412802
Breed M. D. (1976). The Evolution of Social Behavior in Primitively Social Bees: A Multivariate Analysis. Evolution 30 (2), 234–240. doi:10.1111/j.1558-5646.1976.tb00906.x
Brown M. J. F., Schmid‐Hempel R., Schmid‐Hempel P. (2003). Queen‐controlled Sex Ratios and Worker Reproduction in the Bumble Bee Bombus Hypnorum , as Revealed by Microsatellites. Mol. Ecol. 12 (6), 1599–1605. doi:10.1046/j.1365-294x.2003.01840.x
Chen L., Zhu J., Sun G., Raikhel A. S. (2004). The Early Gene Broad Is Involved in the Ecdysteroid Hierarchy Governing Vitellogenesis of the Mosquito Aedes aegypti. J. Mol. Endocrinol. 33 (3), 743–761. doi:10.1677/jme.1.01531
Cheng W. N., Li X. J., Zhao J. J., Zhu‐Salzman K. (2020). Cloning and Characterization of Methoprene‐tolerant (Met) and Krüppel Homolog 1 (Kr‐h1) Genes in the Wheat Blossom Midge, Sitodiplosis Mosellana. Insect Sci. 27 (2), 292–303. doi:10.1111/1744-7917.12638
Cruz J., Mane-Padros D., Zou Z., Raikhel A. S. (2012). Distinct Roles of Isoforms of the Heme-Liganded Nuclear Receptor E75, an Insect Ortholog of the Vertebrate Rev-Erb, in Mosquito Reproduction. Mol. Cell Endocrinol. 349 (2), 262–271. doi:10.1016/j.mce.2011.11.006
Cui Y., Sui Y., Xu J., Zhu F., Palli S. R. (2014). Juvenile Hormone Regulates Aedes aegypti Krüppel Homolog 1 through a Conserved E Box Motif. Insect Biochem. Mol. Biol. 52, 23–32. doi:10.1016/j.ibmb.2014.05.009
Daimon T., Uchibori M., Nakao H., Sezutsu H., Shinoda T. (2015). Knockout Silkworms Reveal a Dispensable Role for Juvenile Hormones in Holometabolous Life Cycle. Proc. Natl. Acad. Sci. U.S.A. 112 (31), E4226–E4235. doi:10.1073/pnas.1506645112
Ding N., Wang Z., Geng N., Zou H., Zhang G., Cao C., et al. (2020). Silencing Br-C Impairs Larval Development and Chitin Synthesis in Lymantria dispar Larvae. J. Insect Physiol. 122, 104041. doi:10.1016/j.jinsphys.2020.104041
Duportets L., Bozzolan F., Abrieux A., Maria A., Gadenne C., Debernard S. (2012). The Transcription Factor Krüppel Homolog 1 Is Linked to the Juvenile Hormone-dependent Maturation of Sexual Behavior in the Male Moth, Agrotis Ipsilon. Gen. Comp. Endocrinol. 176 (2), 158–166. doi:10.1016/j.ygcen.2012.01.005
Duportets L., Dufour M., Couillaud F., Gadenne C. (1998). Biosynthetic Activity of Corpora Allata, Growth of Sex Accessory Glands and Mating in the Male Moth Agrotis Ipsilon (Hufnagel). J. Exp. Biol. 201 (Pt 16), 2425–2432. doi:10.1242/jeb.201.16.2425
Fernandez-Nicolas A., Belles X. (2017). Juvenile Hormone Signaling in Short Germ-Band Hemimetabolan Embryos. Development 144 (24), 4637–4644. doi:10.1242/dev.152827
Fichelson P., Brigui A., Pichaud F. (2012). Orthodenticle and Kruppel Homolog 1 Regulate Drosophila Photoreceptor Maturation. Proc. Natl. Acad. Sci. U S A. 109 (20), 7893–7898. doi:10.1073/pnas.1120276109
Fussnecker B., Grozinger C. (2008). Dissecting the Role ofKr-H1brain Gene Expression in Foraging Behavior in Honey Bees (Apis mellifera). Insect Mol. Biol. 17 (5), 515–522. doi:10.1111/j.1365-2583.2008.00819.x
Gaddelapati S. C., Dhandapani R. K., Palli S. R. (2020). CREB-binding Protein Regulates Metamorphosis and Compound Eye Development in the Yellow Fever Mosquito, Aedes aegypti. Biochim. Biophys. Acta (Bba) - Gene Regul. Mech. 1863 (8), 194576. doi:10.1016/j.bbagrm.2020.194576
Gassias E., Maria A., Couzi P., Demondion E., Durand N., Bozzolan F., et al. (2021). Involvement of Methoprene-Tolerant and Krüppel Homolog 1 in Juvenile Hormone-Signaling Regulating the Maturation of Male Accessory Glands in the Moth Agrotis Ipsilon. Insect Biochem. Mol. Biol. 132, 103566. doi:10.1016/j.ibmb.2021.103566
George S., Gaddelapati S. C., Palli S. R. (2019). Histone Deacetylase 1 Suppresses Krüppel Homolog 1 Gene Expression and Influences Juvenile Hormone Action in Tribolium castaneum. Proc. Natl. Acad. Sci. U.S.A. 116 (36), 17759–17764. doi:10.1073/pnas.1909554116
Gospocic J., Glastad K. M., Sheng L., Shields E. J., Berger S. L., Bonasio R. (2021). Kr-h1 Maintains Distinct Caste-specific Neurotranscriptomes in Response to Socially Regulated Hormones. Cell 184 (23), 5807–5823. e5814. doi:10.1016/j.cell.2021.10.006
Grozinger C. M., Robinson G. E. (2007). Endocrine Modulation of a Pheromone-Responsive Gene in the Honey Bee Brain. J. Comp. Physiol. A. 193 (4), 461–470. doi:10.1007/s00359-006-0202-x
Grozinger C. M., Sharabash N. M., Whitfield C. W., Robinson G. E. (2003). Pheromone-mediated Gene Expression in the Honey Bee Brain. Proc. Natl. Acad. Sci. U.S.A. 100 (2), 14519–14525. doi:10.1073/pnas.2335884100
Gujar H., Palli S. R. (2016a). Juvenile Hormone Regulation of Female Reproduction in the Common Bed Bug, Cimex Lectularius. Sci. Rep. 6, 35546. doi:10.1038/srep35546
Gujar H., Palli S. R. (2016b). Krüppel Homolog 1 and E93 Mediate Juvenile Hormone Regulation of Metamorphosis in the Common Bed Bug, Cimex Lectularius. Sci. Rep. 6, 26092. doi:10.1038/srep26092
Guo S., Wu Q.-W., Tian Z., Zhu L., King-Jones K., Zhu F., et al. (2021). Krüppel Homolog 1 Regulates Photoperiodic Reproductive Plasticity in the Cabbage Beetle Colaphellus Bowringi. Insect Biochem. Mol. Biol. 134, 103582. doi:10.1016/j.ibmb.2021.103582
Han H., Feng Z., Han S., Chen J., Wang D., He Y. (2022). Molecular Identification and Functional Characterization of Methoprene-Tolerant (Met) and Krüppel-Homolog 1 (Kr-H1) in Harmonia axyridis (Coleoptera: Coccinellidae). J. Econ. Entomol. 115 (1), 334–343. doi:10.1093/jee/toab252
He Q., Wen D., Jia Q., Cui C., Wang J., Palli S. R., et al. (2014). Heat Shock Protein 83 (Hsp83) Facilitates Methoprene-Tolerant (Met) Nuclear Import to Modulate Juvenile Hormone Signaling. J. Biol. Chem. 289 (40), 27874–27885. doi:10.1074/jbc.M114.582825
He Q., Zhang Y., Dong W. (2020). MicroRNA miR ‐927 Targets the Juvenile Hormone Primary Response Gene Krüppel Homolog1 to Control Drosophila Developmental Growth. Insect Mol. Biol. 29 (6), 545–554. doi:10.1111/imb.12662
He Q., Zhang Y., Zhang X., Xu D., Dong W., Li S., et al. (2017). Nucleoporin Nup358 Facilitates Nuclear Import of Methoprene-Tolerant (Met) in an Importin β- and Hsp83-dependent Manner. Insect Biochem. Mol. Biol. 81, 10–18. doi:10.1016/j.ibmb.2016.12.005
Hewes R. S. (2008). The Buzz on Fly Neuronal Remodeling. Trends Endocrinol. Metab. 19 (9), 317–323. doi:10.1016/j.tem.2008.07.008
Hu K., Tian P., Yang L., Tang Y., Qiu L., He H., et al. (2020). Molecular Characterization of the Krüppel-Homolog 1 and its Role in Ovarian Development in Sogatella Furcifera (Hemiptera: Delphacidae). Mol. Biol. Rep. 47 (2), 1099–1106. doi:10.1007/s11033-019-05206-7
Huang J., Tian L., Peng C., Abdou M., Wen D., Wang Y., et al. (2011). DPP-mediated TGFβ Signaling Regulates Juvenile Hormone Biosynthesis by Activating the Expression of Juvenile Hormone Acid Methyltransferase. Development 138 (11), 2283–2291. doi:10.1242/dev.057687
Ishimaru Y., Tomonari S., Watanabe T., Noji S., Mito T. (2019). Regulatory Mechanisms Underlying the Specification of the Pupal-Homologous Stage in a Hemimetabolous Insect. Phil. Trans. R. Soc. B 374 (1783), 20190225. doi:10.1098/rstb.2019.0225
Jindra M., McKinstry W. J., Nebl T., Bittova L., Ren B., Shaw J., et al. (2021). Purification of an Insect Juvenile Hormone Receptor Complex Enables Insights into its post-translational Phosphorylation. J. Biol. Chem. 297 (6), 101387. doi:10.1016/j.jbc.2021.101387
Jindra M., Palli S. R., Riddiford L. M. (2013). The Juvenile Hormone Signaling Pathway in Insect Development. Annu. Rev. Entomol. 58 (1), 181–204. doi:10.1146/annurev-ento-120811-153700
Jindra M., Uhlirova M., Charles J.-P., Smykal V., Hill R. J. (2015). Genetic Evidence for Function of the bHLH-PAS Protein Gce/Met as a Juvenile Hormone Receptor. PLOS Genet. 11, e1005394. doi:10.1371/journal.pgen.1005394
Jindra M. (2019). Where Did the Pupa Come from? the Timing of Juvenile Hormone Signalling Supports Homology between Stages of Hemimetabolous and Holometabolous Insects. Phil. Trans. R. Soc. B 374 (1783), 20190064. doi:10.1098/rstb.2019.0064
Kamsoi O., Ventos-Alfonso A., Casares F., Almudi I., Belles X. (2021). Regulation of Metamorphosis in Neopteran Insects Is Conserved in the Paleopteran Cloeon Dipterum (Ephemeroptera). Proc. Natl. Acad. Sci. U S A. 118 (34), e2105272118. doi:10.1073/pnas.2105272118
Kang P., Chang K., Liu Y., Bouska M., Birnbaum A., Karashchuk G., et al. (20172017). Drosophila Kruppel Homolog 1 Represses Lipolysis through Interaction with dFOXOMolecular Mechanism Underlying Juvenile Hormone-Mediated Repression of Precocious Larval-Adult Metamorphosis. Sci. Repproc Natl. Acad. Sci. U S A. 7114 (15), 163691057–163691062. doi:10.1038/s41598-017-16638-110.1073/pnas.1615423114
Kayukawa T., Minakuchi C., Namiki T., Togawa T., Yoshiyama M., Kamimura M., et al. (2012). Transcriptional Regulation of Juvenile Hormone-Mediated Induction of Krüppel Homolog 1, a Repressor of Insect Metamorphosis. Proc. Natl. Acad. Sci. U.S.A. 109 (29), 11729–11734. doi:10.1073/pnas.1204951109
Kayukawa T., Murata M., Kobayashi I., Muramatsu D., Okada C., Uchino K., et al. (2014). Hormonal Regulation and Developmental Role of Krüppel Homolog 1, a Repressor of Metamorphosis, in the Silkworm Bombyx mori. Developmental Biol. 388 (1), 48–56. doi:10.1016/j.ydbio.2014.01.022
Kayukawa T., Nagamine K., Ito Y., Nishita Y., Ishikawa Y., Shinoda T. (2016). Krüppel Homolog 1 Inhibits Insect Metamorphosis via Direct Transcriptional Repression of Broad-Complex, a Pupal Specifier Gene. J. Biol. Chem. 291 (4), 1751–1762. doi:10.1074/jbc.M115.686121
Kayukawa T., Tateishi K., Shinoda T. (2013). Establishment of a Versatile Cell Line for Juvenile Hormone Signaling Analysis in Tribolium castaneum. Sci. Rep. 3, 1570. doi:10.1038/srep01570
Kewley R. J., Whitelaw M. L., Chapman-Smith A. (2004). The Mammalian Basic helix-loop-helix/PAS Family of Transcriptional Regulators. Int. J. Biochem. Cell Biol. 36 (2), 189–204. doi:10.1016/s1357-2725(03)00211-5
Khalid M. Z., Ahmad S., Ngegba P. M., Zhong G. (2021). Role of Endocrine System in the Regulation of Female Insect Reproduction. Biology 10 (7), 614. doi:10.3390/biology10070614
Kiss I., Beaton A. H., Tardiff J., Fristrom D., Fristrom J. W. (1988). Interactions and Developmental Effects of Mutations in the Broad-Complex of Drosophila melanogaster. Genetics 118 (2), 247–259. doi:10.1093/genetics/118.2.247
Kiss I., Bencze G., Fodor A., Szabad J., Fristrom J. W. (1976). Prepupal Larval Mosaics in Drosophila melanogaster. Nature 262 (5564), 136–138. doi:10.1038/262136a0
Kokoza V. A., Martin D., Mienaltowski M. J., Ahmed A., Morton C. M., Raikhel A. S. (2001). Transcriptional Regulation of the Mosquito Vitellogenin Gene via a Blood Meal-Triggered cascade. Gene 274 (1-2), 47–65. doi:10.1016/s0378-1119(01)00602-3
Konopova B., Jindra M. (2008). Broad-complex Acts Downstream of Met in Juvenile Hormone Signaling to Coordinate Primitive Holometabolan Metamorphosis. Development 135 (3), 559–568. doi:10.1242/Dev.016097
Konopova B., Smykal V., Jindra M. (2011). Common and Distinct Roles of Juvenile Hormone Signaling Genes in Metamorphosis of Holometabolous and Hemimetabolous Insects. PLoS One 6 (12), e28728. doi:10.1371/journal.pone.0028728
Lee C.-Y., Wendel D. P., Reid P., Lam G., Thummel C. S., Baehrecke E. H. (2000). E93 Directs Steroid-Triggered Programmed Cell Death in Drosophila. Mol. Cell 6 (2), 433–443. doi:10.1016/s1097-2765(00)00042-3
Lee T., Lee A., Luo L. (1999). Development of the Drosophila Mushroom Bodies: Sequential Generation of Three Distinct Types of Neurons from a Neuroblast. Development 126 (18), 4065–4076. doi:10.1242/dev.126.18.4065
Li K. L., Yuan S. Y., Nanda S., Wang W. X., Lai F. X., Fu Q., et al. (2018). The Roles of E93 and Kr-H1 in Metamorphosis of Nilaparvata Lugens. Front. Physiol. 9, 1677. doi:10.3389/fphys.2018.01677
Li M., Mead E. A., Zhu J. (2011). Heterodimer of Two bHLH-PAS Proteins Mediates Juvenile Hormone-Induced Gene Expression. Proc. Natl. Acad. Sci. U.S.A. 108 (2), 638–643. doi:10.1073/pnas.1013914108
Liu P., Peng H.-J., Zhu J. (2015). Juvenile Hormone-Activated Phospholipase C Pathway Enhances Transcriptional Activation by the Methoprene-Tolerant Protein. Proc. Natl. Acad. Sci. U.S.A. 112 (15), E1871–E1879. doi:10.1073/pnas.1423204112
Liu S., Li K., Gao Y., Liu X., Chen W., Ge W., et al. (2018). Antagonistic Actions of Juvenile Hormone and 20-hydroxyecdysone within the Ring Gland Determine Developmental Transitions in Drosophila. Proc. Natl. Acad. Sci. U.S.A. 115 (1), 139–144. doi:10.1073/pnas.1716897115
Liu Y., Sheng Z., Liu H., Wen D., He Q., Wang S., et al. (2009). Juvenile Hormone Counteracts the bHLH-PAS Transcription Factors MET and GCE to Prevent Caspase-dependent Programmed Cell Death inDrosophila. Development 136 (12), 2015–2025. doi:10.1242/Dev.033712
Lozano J., Belles X. (2011). Conserved Repressive Function of Krüppel Homolog 1 on Insect Metamorphosis in Hemimetabolous and Holometabolous Species. Sci. Rep. 1, 163. doi:10.1038/srep00163
Lozano J., Montañez R., Belles X. (2015). MiR-2 Family Regulates Insect Metamorphosis by Controlling the Juvenile Hormone Signaling Pathway. Proc. Natl. Acad. Sci. U.S.A. 112 (12), 3740–3745. doi:10.1073/pnas.1418522112
Luo W., Liu S., Zhang W., Yang L., Huang J., Zhou S., et al. (2021). Juvenile Hormone Signaling Promotes Ovulation and Maintains Egg Shape by Inducing Expression of Extracellular Matrix Genes. Proc. Natl. Acad. Sci. U.S.A. 118 (39). doi:10.1073/pnas.2104461118
Luo W., Veeran S., Wang J., Li S., Li K., Liu S. N. (2020). Dual Roles of Juvenile Hormone Signaling during Early Oogenesis in Drosophila. Insect Sci. 27 (4), 665–674. doi:10.1111/1744-7917.12698
Mane-Padros D., Cruz J., Cheng A., Raikhel A. S. (2012). A Critical Role of the Nuclear Receptor HR3 in Regulation of Gonadotrophic Cycles of the Mosquito Aedes aegypti. PLoS One 7 (9), e45019. doi:10.1371/journal.pone.0045019
Mao Y., Li Y., Gao H., Lin X. (2020). Krüppel Homologue 1 Interacts Directly with Hairy and Regulates Ecdysis in the Brown Planthopper. Insect Mol. Biol. 29 (3), 293–300. doi:10.1111/imb.12635
Marchetti G., Tavosanis G. (2019). Modulators of Hormonal Response Regulate Temporal Fate Specification in the Drosophila Brain. Plos Genet. 15 (12), e1008491. doi:10.1371/journal.pgen.1008491
Martín D., Wang S. F., Raikhel A. S. (2001). The Vitellogenin Gene of the Mosquito Aedes aegypti Is a Direct Target of Ecdysteroid Receptor. Mol. Cell Endocrinol 173 (1-2), 75–86. doi:10.1016/s0303-7207(00)00413-5
Martín D., Chafino S., Franch-Marro X. (2021). How Stage Identity Is Established in Insects: the Role of the Metamorphic Gene Network. Curr. Opin. Insect Sci. 43, 29–38. doi:10.1016/j.cois.2020.10.002
Minakuchi C., Namiki T., Shinoda T. (2009). Krüppel Homolog 1, an Early Juvenile Hormone-Response Gene Downstream of Methoprene-Tolerant, Mediates its Anti-metamorphic Action in the Red Flour Beetle Tribolium castaneum. Developmental Biol. 325 (2), 341–350. doi:10.1016/j.ydbio.2008.10.016
Minakuchi C., Namiki T., Yoshiyama M., Shinoda T. (2008a). RNAi-mediated Knockdown of Juvenile Hormone Acid O-Methyltransferase Gene Causes Precocious Metamorphosis in the Red Flour Beetle Tribolium castaneum. FEBS J. 275 (11), 2919–2931. doi:10.1111/j.1742-4658.2008.06428.x
Minakuchi C., Zhou X., Riddiford L. M. (2008b). Krüppel Homolog 1 (Kr-H1) Mediates Juvenile Hormone Action during Metamorphosis of Drosophila melanogaster. Mech. Development 125 (1-2), 91–105. doi:10.1016/j.mod.2007.10.002
Mirth C. K., Tang H. Y., Makohon-Moore S. C., Salhadar S., Gokhale R. H., Warner R. D., et al. (2014). Juvenile Hormone Regulates Body Size and Perturbs Insulin Signaling in Drosophila. Proc. Natl. Acad. Sci. U.S.A. 111 (19), 7018–7023. doi:10.1073/pnas.1313058111
Misof B., Liu S., Meusemann K., Peters R. S., Donath A., Mayer C., et al. (2014). Phylogenomics Resolves the Timing and Pattern of Insect Evolution. Science 346 (6210), 763–767. doi:10.1126/science.1257570
Nouzova M., Edwards M. J., Michalkova V., Ramirez C. E., Ruiz M., Areiza M., et al. (2021). Epoxidation of Juvenile Hormone Was a Key Innovation Improving Insect Reproductive Fitness. Proc. Natl. Acad. Sci. U.S.A. 118 (45). doi:10.1073/pnas.2109381118
Ojani R., Fu X., Ahmed T., Liu P., Zhu J. (2018). Krüppel Homologue 1 Acts as a Repressor and an Activator in the Transcriptional Response to Juvenile Hormone in Adult Mosquitoes. Insect Mol. Biol. 27 (2), 268–278. doi:10.1111/imb.12370
Pandey A., Bloch G. (2021). Krüppel-homologue 1 Mediates Hormonally Regulated Dominance Rank in a Social Bee. Biology 10 (11), 1188. doi:10.3390/biology10111188
Pandey A., Motro U., Bloch G. (2020). Juvenile Hormone Interacts with Multiple Factors to Modulate Aggression and Dominance in Groups of Orphan Bumble Bee (Bombus Terrestris) Workers. Horm. Behav. 117, 104602. doi:10.1016/j.yhbeh.2019.104602
Parthasarathy R., Sun Z., Bai H., Palli S. R. (2010). Juvenile Hormone Regulation of Vitellogenin Synthesis in the Red Flour Beetle, Tribolium castaneum. Insect Biochem. Mol. Biol. 40 (5), 405–414. doi:10.1016/j.ibmb.2010.03.006
Parthasarathy R., Tan A., Bai H., Palli S. R. (2008). Transcription Factor Broad Suppresses Precocious Development of Adult Structures during Larval-Pupal Metamorphosis in the Red Flour Beetle, Tribolium castaneum. Mech. Development 125 (3-4), 299–313. doi:10.1016/j.mod.2007.11.001
Pecasse F., Beck Y., Ruiz C., Richards G. (2000). Krüppel-homolog, a Stage-specific Modulator of the Prepupal Ecdysone Response, Is Essential for Drosophila Metamorphosis. Developmental Biol. 221 (1), 53–67. doi:10.1006/dbio.2000.9687
Penick C. A., Liebig J., Brent C. S. (2011). Reproduction, Dominance, and Caste: Endocrine Profiles of Queens and Workers of the Ant Harpegnathos Saltator. J. Comp. Physiol. A. 197 (11), 1063–1071. doi:10.1007/s00359-011-0667-0
Piulachs M.-D., Pagone V., Bellés X. (2010). Key Roles of the Broad-Complex Gene in Insect Embryogenesis. Insect Biochem. Mol. Biol. 40 (6), 468–475. doi:10.1016/j.ibmb.2010.04.006
Raikhel A. S., Dhadialla T. S. (1992). Accumulation of Yolk Proteins in Insect Oocytes. Annu. Rev. Entomol. 37, 217–251. doi:10.1146/annurev.en.37.010192.001245
Reza A. M. S., Kanamori Y., Shinoda T., Shimura S., Mita K., Nakahara Y., et al. (2004). Hormonal Control of a Metamorphosis-specific Transcriptional Factor Broad-Complex in Silkworm. Comp. Biochem. Physiol. B: Biochem. Mol. Biol. 139 (4), 753–761. doi:10.1016/j.cbpc.2004.09.009
Riddiford L. M. (1994). Cellular and Molecular Actions of Juvenile Hormone I. General Considerations and Premetamorphic Actions. Adv. Insect Physiol. 24, 213–274. doi:10.1016/s0065-2806(08)60084-3
Riddiford L. M. (2012). How Does Juvenile Hormone Control Insect Metamorphosis and Reproduction? Gen. Comp. Endocrinol. 179 (3), 477–484. doi:10.1016/j.ygcen.2012.06.001
Robinson G. E. (1992). Regulation of Division of Labor in Insect Societies. Annu. Rev. Entomol. 37, 637–665. doi:10.1146/annurev.en.37.010192.003225
Roy A., George S., Palli S. R. (2017). Multiple Functions of CREB-Binding Protein during Postembryonic Development: Identification of Target Genes. BMC Genomics 18 (1), 996. doi:10.1186/s12864-017-4373-3
Roy A., Palli S. R. (2018). Epigenetic Modifications Acetylation and Deacetylation Play Important Roles in Juvenile Hormone Action. BMC Genomics 19 (1), 934. doi:10.1186/s12864-018-5323-4
Roy S., Saha T. T., Zou Z., Raikhel A. S. (2018). Regulatory Pathways Controlling Female Insect Reproduction. Annu. Rev. Entomol. 63, 489–511. doi:10.1146/annurev-ento-020117-043258
Saha T. T., Roy S., Pei G., Dou W., Zou Z., Raikhel A. S. (2019). Synergistic Action of the Transcription Factors Krüppel Homolog 1 and Hairy in Juvenile hormone/Methoprene-Tolerant-Mediated Gene-Repression in the Mosquito Aedes aegypti. Plos Genet. 15 (10), e1008443. doi:10.1371/journal.pgen.1008443
Santos C. G., Humann F. C., Hartfelder K. (2019). Juvenile Hormone Signaling in Insect Oogenesis. Curr. Opin. Insect Sci. 31, 43–48. doi:10.1016/j.cois.2018.07.010
Sasaki T., Penick C. A., Shaffer Z., Haight K. L., Pratt S. C., Liebig J. (2016). A Simple Behavioral Model Predicts the Emergence of Complex Animal Hierarchies. The Am. Naturalist 187 (6), 765–775. doi:10.1086/686259
Schuh R., Aicher W., Gaul U., Côte S., Preiss A., Maier D., et al. (1986). A Conserved Family of Nuclear Proteins Containing Structural Elements of the finger Protein Encoded by Krüppel, a Drosophila Segmentation Gene. Cell 47 (6), 1025–1032. doi:10.1016/0092-8674(86)90817-2
Sheng L., Shields E. J., Gospocic J., Glastad K. M., Ratchasanmuang P., Berger S. L., et al. (2020). Social Reprogramming in Ants Induces Longevity-Associated Glia Remodeling. Sci. Adv. 6 (34), eaba9869. doi:10.1126/sciadv.aba9869
Sheng Z., Xu J., Bai H., Zhu F., Palli S. R. (2011). Juvenile Hormone Regulates Vitellogenin Gene Expression through Insulin-like Peptide Signaling Pathway in the Red Flour Beetle, Tribolium castaneum. J. Biol. Chem. 286 (49), 41924–41936. doi:10.1074/jbc.M111.269845
Shi L., Lin S., Grinberg Y., Beck Y., Grozinger C. M., Robinson G. E., et al. (2007). Roles ofDrosophila Kruppel-Homolog 1 in Neuronal Morphogenesis. Devel Neurobio 67 (12), 1614–1626. doi:10.1002/dneu.20537
Shpigler H., Patch H. M., Cohen M., Fan Y., Grozinger C. M., Bloch G. (2010). The Transcription Factor Krüppel Homolog 1is Linked to Hormone Mediated Social Organization in Bees. BMC Evol. Biol. 10, 120. doi:10.1186/1471-2148-10-120
Smykal V., Bajgar A., Provaznik J., Fexova S., Buricova M., Takaki K., et al. (2014a). Juvenile Hormone Signaling during Reproduction and Development of the linden Bug, Pyrrhocoris apterus. Insect Biochem. Mol. Biol. 45, 69–76. doi:10.1016/j.ibmb.2013.12.003
Smykal V., Daimon T., Kayukawa T., Takaki K., Shinoda T., Jindra M. (2014b). Importance of Juvenile Hormone Signaling Arises with Competence of Insect Larvae to Metamorphose. Developmental Biol. 390 (2), 221–230. doi:10.1016/j.ydbio.2014.03.006
Soller M., Bownes M., Kubli E. (1999). Control of Oocyte Maturation in Sexually MatureDrosophilaFemales. Developmental Biol. 208 (2), 337–351. doi:10.1006/dbio.1999.9210
Song J., Li W., Zhao H., Gao L., Fan Y., Zhou S. (2018). MicroRNA Let-7 and miR-278 Regulate Insect Metamorphosis and Oogenesis via Targeting Juvenile Hormone Early Response Gene Krüppel-Homolog 1. Development 145 (24). doi:10.1242/dev.170670
Song J., Wu Z., Wang Z., Deng S., Zhou S. (2014). Krüppel-homolog 1 Mediates Juvenile Hormone Action to Promote Vitellogenesis and Oocyte Maturation in the Migratory Locust. Insect Biochem. Mol. Biol. 52, 94–101. doi:10.1016/j.ibmb.2014.07.001
Sun G., Zhu J., Raikhel A. S. (2004). The Early Gene E74B Isoform Is a Transcriptional Activator of the Ecdysteroid Regulatory Hierarchy in Mosquito Vitellogenesis. Mol. Cell Endocrinol. 218 (1-2), 95–105. doi:10.1016/j.mce.2003.12.014
Suzuki Y., Truman J. W., Riddiford L. M. (2008). The Role of Broad in the Development ofTribolium Castaneum:implications for the Evolution of the Holometabolous Insect Pupa. Development 135 (3), 569–577. doi:10.1242/Dev.015263
Tan A., Tanaka H., Tamura T., Shiotsuki T. (2005). Precocious Metamorphosis in Transgenic Silkworms Overexpressing Juvenile Hormone Esterase. Proc. Natl. Acad. Sci. U.S.A. 102 (33), 11751–11756. doi:10.1073/pnas.0500954102
Tang Y., He H., Qu X., Cai Y., Ding W., Qiu L., et al. (2020). RNA Interference‐mediated Knockdown of the Transcription Factor Krüppel Homologue 1 Suppresses Vitellogenesis in Chilo Suppressalis. Insect Mol. Biol. 29 (2), 183–192. doi:10.1111/imb.12617
Truman J. W. (2019). The Evolution of Insect Metamorphosis. Curr. Biol. 29 (23), R1252–R1268. doi:10.1016/j.cub.2019.10.009
Uhlirova M., Foy B. D., Beaty B. J., Olson K. E., Riddiford L. M., Jindra M. (2003). Use of Sindbis Virus-Mediated RNA Interference to Demonstrate a Conserved Role of Broad-Complex in Insect Metamorphosis. Proc. Natl. Acad. Sci. U.S.A. 100 (26), 15607–15612. doi:10.1073/pnas.2136837100
Ureña E., Chafino S., Manjón C., Franch-Marro X., Martín D. (2016). The Occurrence of the Holometabolous Pupal Stage Requires the Interaction between E93, Krüppel-Homolog 1 and Broad-Complex. Plos Genet. 12 (5), e1006020. doi:10.1371/journal.pgen.1006020
Ureña E., Manjón C., Franch-Marro X., Martín D. (2014). Transcription Factor E93 Specifies Adult Metamorphosis in Hemimetabolous and Holometabolous Insects. Proc. Natl. Acad. Sci. U.S.A. 111 (19), 7024–7029. doi:10.1073/pnas.1401478111
Valle D. (1993). Vitellogenesis in Insects and Other Groups: a Review. Mem. Inst. Oswaldo Cruz 88 (1), 1–26. doi:10.1590/s0074-02761993000100005
Wang J.-L., Saha T. T., Zhang Y., Zhang C., Raikhel A. S. (2017). Juvenile Hormone and its Receptor Methoprene-Tolerant Promote Ribosomal Biogenesis and Vitellogenesis in the Aedes aegypti Mosquito. J. Biol. Chem. 292 (24), 10306–10315. doi:10.1074/jbc.M116.761387
Whitfield C. W., Cziko A.-M., Robinson G. E. (2003). Gene Expression Profiles in the Brain Predict Behavior in Individual Honey Bees. Science 302 (5643), 296–299. doi:10.1126/science.1086807
Wu Z., Yang L., He Q., Zhou S. (2020). Regulatory Mechanisms of Vitellogenesis in Insects. Front. Cell Dev. Biol. 8, 593613. doi:10.3389/fcell.2020.593613
Wu Z., Yang L., Li H., Zhou S. (2021). Krüppel-homolog 1 Exerts Anti-metamorphic and Vitellogenic Functions in Insects via Phosphorylation-Mediated Recruitment of Specific Cofactors. BMC Biol. 19 (1), 222. doi:10.1186/s12915-021-01157-3
Wyatt G. R., Davey K. G. (1996). Cellular and Molecular Actions of Juvenile Hormone. II. Roles of Juvenile Hormone in Adult Insects. Adv. Insect Physiol. 26, 1–155. doi:10.1016/s0065-2806(08)60030-2
Xu J., Roy A., Palli S. R. (2018). CREB-binding Protein Plays Key Roles in Juvenile Hormone Action in the Red Flour Beetle, Tribolium Castaneum. Sci. Rep. 8 (1), 1426. doi:10.1038/s41598-018-19667-6
Yue Y., Yang R.-L., Wang W.-P., Zhou Q.-H., Chen E.-H., Yuan G.-R., et al. (2018). Involvement of Met and Kr-H1 in JH-Mediated Reproduction of Female Bactrocera Dorsalis (Hendel). Front. Physiol. 9, 482. doi:10.3389/fphys.2018.00482
Zhang T., Song W., Li Z., Qian W., Wei L., Yang Y., et al. (2018a). Krüppel Homolog 1 Represses Insect Ecdysone Biosynthesis by Directly Inhibiting the Transcription of Steroidogenic Enzymes. Proc. Natl. Acad. Sci. U.S.A. 115 (15), 3960–3965. doi:10.1073/pnas.1800435115
Zhang W.-N., Ma L., Liu C., Chen L., Xiao H.-J., Liang G.-M. (2018b). Dissecting the Role ofKrüppel Homolog 1in the Metamorphosis and Female Reproduction of the Cotton bollworm,Helicoverpa Armigera. Insect Mol. Biol. 27 (4), 492–504. doi:10.1111/imb.12389
Zhang Z., Xu J., Sheng Z., Sui Y., Palli S. R. (2011). Steroid Receptor Co-activator Is Required for Juvenile Hormone Signal Transduction through a bHLH-PAS Transcription Factor, Methoprene Tolerant. J. Biol. Chem. 286 (10), 8437–8447. doi:10.1074/jbc.M110.191684
Zhou B., Hiruma K., Shinoda T., Riddiford L. M. (1998). Juvenile Hormone Prevents Ecdysteroid-Induced Expression of Broad Complex RNAs in the Epidermis of the Tobacco Hornworm,Manduca Sexta. Developmental Biol. 203 (2), 233–244. doi:10.1006/dbio.1998.9059
Zhou X., Riddiford L. M. (2002). Broad Specifies Pupal Development and Mediates the 'Status Quo' Action of Juvenile Hormone on the Pupal-Adult Transformation inDrosophilaandManduca. Development 129 (9), 2259–2269. doi:10.1242/dev.129.9.2259
Zhu J., Busche J. M., Zhang X. (2010). Identification of Juvenile Hormone Target Genes in the Adult Female Mosquitoes. Insect Biochem. Mol. Biol. 40 (1), 23–29. doi:10.1016/j.ibmb.2009.12.004
Zhu J., Chen L., Raikhel A. S. (2003). Posttranscriptional Control of the Competence Factor βFTZ-F1 by Juvenile Hormone in the Mosquito Aedes aegypti. Proc. Natl. Acad. Sci. U.S.A. 100 (23), 13338–13343. doi:10.1073/pnas.2234416100
Zhu J., Chen L., Sun G., Raikhel A. S. (2006). The Competence Factor βFtz-F1 Potentiates Ecdysone Receptor Activity via Recruiting a P160/SRC Coactivator. Mol. Cell Biol 26 (24), 9402–9412. doi:10.1128/MCB.01318-06
Zhu Z. D., Hu Q. H., Tong C. M., Yang H. G., Zheng S. C., Feng Q. L., et al. (2021b). Transcriptomic Analysis Reveals the Regulation Network of BmKrüppel Homolog 1 in the Oocyte Development of Bombyx mori. Insect Sci. 28 (1), 47–62. doi:10.1111/1744-7917.12747
Keywords: Kr-h1, metamorphosis, reproduction, juvenile hormone, ecdysone, behavioral plastisity, neuronal morphogenesis, embryogenesis
Citation: He Q and Zhang Y (2022) Kr-h1, a Cornerstone Gene in Insect Life History. Front. Physiol. 13:905441. doi: 10.3389/fphys.2022.905441
Received: 27 March 2022; Accepted: 13 April 2022;
Published: 27 April 2022.
Edited by:
Milena Damulewicz, Other, Krakow, PolandReviewed by:
Marek Jindra, Academy of Sciences of the Czech Republic (ASCR), CzechiaCopyright © 2022 He and Zhang. This is an open-access article distributed under the terms of the Creative Commons Attribution License (CC BY). The use, distribution or reproduction in other forums is permitted, provided the original author(s) and the copyright owner(s) are credited and that the original publication in this journal is cited, in accordance with accepted academic practice. No use, distribution or reproduction is permitted which does not comply with these terms.
*Correspondence: Qianyu He, aGVxaWFueXUyMDA1QDE2My5jb20=
†These authors have contributed equally to this work and share first authorship
Disclaimer: All claims expressed in this article are solely those of the authors and do not necessarily represent those of their affiliated organizations, or those of the publisher, the editors and the reviewers. Any product that may be evaluated in this article or claim that may be made by its manufacturer is not guaranteed or endorsed by the publisher.
Research integrity at Frontiers
Learn more about the work of our research integrity team to safeguard the quality of each article we publish.