- Departamento de Genética, Fisiología y Microbiología, Unidad Docente de Fisiología Animal, Facultad de Biología, Universidad Complutense de Madrid, Madrid, Spain
The circadian system is formed by a network of oscillators located in central and peripheral tissues that are tightly linked to generate rhythms in vertebrates to adapt the organism to the cyclic environmental changes. The nuclear receptors PPARs, REV-ERBs and RORs are transcription factors controlled by the circadian system that regulate, among others, a large number of genes that control metabolic processes for which they have been proposed as key genes that link metabolism and temporal homeostasis. To date it is unclear whether these nuclear receptors show circadian expression and which zeitgebers are important for their synchronization in fish. Therefore, the objective of this study was to investigate whether the two main zeitgebers (light-dark cycle and feeding time) could affect the synchronization of central (hypothalamus) and peripheral (liver) core clocks and nuclear receptors in goldfish. To this aim, three experimental groups were established: fish under a 12 h light-12 h darkness and fed at Zeitgeber Time 2; fish with the same photoperiod but randomly fed; and fish under constant darkness and fed at Circadian Time 2. After one month, clock genes and nuclear receptors expression in hypothalamus and liver and circulating glucose were studied. Clock genes displayed daily rhythms in both tissues of goldfish if the light-dark cycle was present, with shifted-acrophases of negative and positive elements, as expected for proper functioning clocks. In darkness-maintained fish hypothalamic clock genes were fully arrhythmic while the hepatic ones were still rhythmic. Among studied nuclear receptors, in the hypothalamus only nr1d1 was rhythmic and only when the light-dark cycle was present. In the liver all nuclear receptors were rhythmic when both zeitgebers were present, but only nr1d1 when one of them was removed. Plasma glucose levels showed significant rhythms in fish maintained under random fed regimen or constant darkness, with the highest levels at 1-h postprandially in all groups. Altogether these results support that hypothalamus is mainly a light-entrained-oscillator, while the liver is a food-entrained-oscillator. Moreover, nuclear receptors are revealed as clear outputs of the circadian system acting as key elements in the timekeeping of temporal homeostasis, particularly in the liver.
Introduction
Nuclear receptors (NRs) belong to a superfamily of ligand-activated transcription factors that regulate gene transcription by modulating essential physiological processes such as growth, development, immunity, and metabolic homeostasis (Kojetin and Burris, 2014; Ribas-Latre and Eckel-Mahan, 2016; Lee et al., 2021). All NRs described up to the present share a great homology in their amino acid sequence with highly conserved domain structures (Solt et al., 2011). Some classical NRs are currently well-known, such as steroid or thyroid receptors. However, there are also a huge number of other less known NRs recently described. Recent studies have advanced in the knowledge of three subfamilies of NRs participating as critical regulators of circadian clock, with significant roles in the regulation of metabolism (Solt et al., 2011; Kojetin and Burris, 2014; Berthier et al., 2021; Lee et al., 2021). These three subfamilies are the nuclear receptor subfamily 1 group C (nr1c), commonly named as PPAR; the nuclear receptor subfamily 1 group D (nr1d) or REV-ERB, and the nuclear receptor subfamily 1 group F (nr1f) or retinoic acid-related orphan receptor (ROR) (Nuclear Receptors Nomenclature Committee, 1999; Weikum et al., 2018).
Most NRs bind to DNA as dimers with another member of the NRs superfamily, and this is the case of PPAR receptors, which form heterodimers with the retinoid X receptors (RXRs). Once the linkage has occurred, the heterodimer PPAR/RXR binds to the PPAR response elements (PPRE) in the promoter of its target genes, inducing their expression (Solt et al., 2011; Chen and Yang, 2014). On their side, REV-ERB and ROR are particular NRs, as they act usually as monomers (Kojetin and Burris, 2014; Cook et al., 2015). REV-ERB competes with ROR for binding to the retinoic orphan receptor binding elements–RORE–, acting REV-ERB as a repressor and ROR as an inductor of genes containing RORE elements (Solt et al., 2011; Chen and Yang, 2014; Ribas-Latre and Eckel-Mahan, 2016; Albrecht and Ripperger, 2018).
As regulators of genes involved in lipid and glucose metabolism (lipid storage and lipogenesis, hepatic fatty acid oxidation, gluconeogenesis, or ketogenesis among several functions), these three subfamilies of proteins provide an essential key for the maintenance of energy homeostasis in metabolic tissues (Chen and Yang, 2014; Pawlak et al., 2015; Vieira et al., 2015; Duszka and Wahli, 2020; Berthier et al., 2021; Lee et al., 2021). Although they were considered orphan receptors for a long time, different endogenous compounds have been emerged as natural ligands for these NRs. Specifically, PPAR ligands are endogenous fatty acids, such as N-acylethanolamines and eicosanoids (Chen and Yang, 2014), being the heme group the main endogenous molecule which reversibly binds to REV-ERBs (Yin et al., 2007; Kojetin and Burris, 2014), while cholesterol is suggested to be a potential reversibly ligand for RORs (Solt et al., 2011). In this sense, these nuclear receptors could act as metabolic sensors.
It is well known that PPARs and REV-ERBs/RORs are the main NRs that link metabolism and circadian system (Vieira et al., 2015; Ribas-Latre and Eckel-Mahan, 2016; Albrecht and Ripperger, 2018). The circadian system is formed by a wide variety of oscillators located in central and peripheral tissues that provides the temporal organization for physiological and behavioral activities enabling the animals to predict environmental cyclic changes. These clocks are closely inter-communicated and generate circadian endogenous rhythms with a period close, but generally not equal, to 24 h (Albrecht, 2012; Schibler et al., 2015; Isorna et al., 2017). The molecular functioning of this set of oscillators is based on transcriptional-translational feedback loops of named clock genes. The main loop is formed by two pairs of clock genes that define two different limbs. The positive limb is represented by the circadian locomotor output cycles kaput (CLOCK) and the brain and muscle ARNT-like 1 (BMAL1) proteins, whose heterodimer acts as an activator transcription factor binding to an E-box rich region in the promoter of the negative limb genes period (per) and cryptochrome (cry). Then, the heterodimer of PER:CRY represses the CLOCK:BMAL1 transactivation (Nader et al., 2010; Schibler et al., 2015). The functioning of this molecular mechanisms is well conserved in vertebrates, although several copies of these clock genes have been reported in fishes (Sánchez-Bretaño et al., 2015a; Isorna et al., 2017).
The outputs of the clock occur through the CLOCK:BMAL1 heterodimer which not only promotes the expression of the negative limb genes, but also induces the expression of genes known as clock-controlled genes (CCG), by binding to an E-box rich region in their respective promoters (Albrecht and Ripperger, 2018). These CCGs, which expression follows a 24-h pattern, are considered the outputs of the clocks, and include a wide variety of metabolic, endocrine, and feeding-related genes. Many transcription factors are considered as CCGs, such as the NRs subject of the present study, PPARs, REV-ERBs, and RORs. Besides their role as outputs of endogenous oscillator, these transcription factors also constitute auxiliary loops of the core clock, that stabilize the main loop giving more robustness to the cycling and synchronization of the circadian system (Schibler et al., 2015; Albrecht and Ripperger, 2018), at least in mammals. The possible circadian rhythmicity of these NRs in teleost has not been explored to date.
Although clock circadian oscillations are endogenous, the synchronization of the circadian system to external cues is essential to keeping temporal organization and to predict environmental cyclic changes. The environmental signals that entrain endogenous clocks are named zeitgebers, “time giver” in German (Albrecht, 2012; Tsang et al., 2014; Challet, 2015), being the light-dark (LD) cycle the most important one, that dominantly entrains the central clocks. Clocks synchronized by this zeitgeber are named Light-Entrainable Oscillators or LEOs (Mendoza and Challet, 2009; Tahara and Shibata, 2018). The effects of the LD cycle on peripheral organs are not as evident as in the brain. However, feeding time is considered one of the most potent zeitgeber, that especially synchronizes the peripheral clocks of the gastrointestinal tract in mammals (Tahara and Shibata, 2018) and in fish (Nisembaum et al., 2012; Isorna et al., 2017; Gómez-Boronat et al., 2018). These peripheral clocks entrained by the feeding-fasting cycles are known as Feeding-Entrainable Oscillators or FEOs (Stephan, 2002; Mendoza and Challet, 2009) and allow tissues to anticipate the food arrival in order to optimize the processes demanded for food digestion, metabolism, and energy storage and utilization (Lamia et al., 2008). In fish, the effects of feeding time on physiological and behavioral aspects are well studied. Feeding time affects daily locomotor activity rhythms (Aranda et al., 2001; Cavallari et al., 2011; Feliciano et al., 2011; Gómez-Boronat et al., 2018); clock genes expression in liver, gut, and brain (López-Olmeda et al., 2009; López-Olmeda et al., 2010; Feliciano et al., 2011; Nisembaum et al., 2012; Tinoco et al., 2014; Gómez-Boronat et al., 2018); and daily profile of circulating cortisol (Montoya et al., 2010; Cowan et al., 2017; Gómez-Boronat et al., 2018; Saiz et al., 2021). However, it is unknown whether feeding time, in addition to entrain clock genes, also drives daily rhythms in PPARs, REV-ERBs, and RORs transcription factors in teleost.
Therefore, the aims of this work were first to study if the NRs rhythms could be of circadian nature in central and peripheral clocks in goldfish (Carassius auratus), and second to investigate whether such rhythms are synchronized by the two main zeitgebers (LD cycle and feeding time). To this end, we have studied the effects of an absence of the LD cycle and feeding schedule on 24-h daily patterns of clock genes (per1a, per1b, per2a, per3, clock1a, and bmal1a) and NRs transcripts (pparα, pparγ, nr1d1, nr1d2 b, and rorα a) in the hypothalamus and the liver in this teleost species. Daily pattern in plasma glucose, as an important metabolic output of the circadian system, was also studied.
Material and Methods
Animals and Housing
Goldfish with a body weight (bw) of 13.9 ± 0.4 g were obtained from a local commercial supplier (ICA). Fish were housed in 60 L aquaria with filtered and aerated fresh water (21 ± 2°C), under a 12L:12D photoperiod (lights on at 8 a.m., considered as Zeitgeber Time 0, ZT 0). Fish were daily fed at ZT 2 with food pellets (1% bw; Sera Pond Biogranulat). Animals were acclimated during 3 weeks under these conditions before the experiment. All the animal procedures complied with the Guidelines of the European Union Council (UE63/2010) and the Spanish Government (RD53/2013) for the use of animals in research and was approved by the Community of Madrid (PROEX 170.6/20).
Experimental Design
Fish (n = 147, 7/aquaria) were divided into three groups. One group (Group I; n = 49) was maintained under a 12L:12D photoperiod and scheduled fed at ZT 2. A second group (Group II; n = 49) was maintained under a 12L:12D photoperiod and was randomly fed at fasting-eating intervals of a maximum 24 h. Random feeding times were provided by the random number generator software (RAND function, Microsoft Excel®). The third group (Group III; n = 49) was maintained at constant dark (24D) and scheduled fed at circadian time 2 (CT 2). One month later, goldfish were sampled every 4 h throughout a 24-h cycle (n = 7 fish/sampling time; ZT 3, ZT 7, ZT 11, ZT 15, ZT 19, ZT 23, and ZT 3 on next day—named ZT 3b). Food was offered as scheduled (ZT 2/CT 2) the first day of the experiment, but not the second day before last sampling time (ZT 3b). Thus, the possible effect of food intake on gene expression and circulating glucose levels was tested by comparing fish sampled at the same daily time, but 1-h postprandial (ZT 3) or 25-h fasting (ZT 3b). Blood was collected from the caudal vein of anesthetized animals (tricaine methanesulfonate, MS-222, 0.14 g/L; Sigma-Aldrich), and plasma was obtained after blood centrifugation and stored at −80°C until assay. Fish were then sacrificed by anesthetic overdose (MS-222, 0.28 g/L), and hypothalamus and liver were quickly collected, frozen in liquid nitrogen, and stored at −80°C until analysis.
Quantification of Plasma Glucose
The circulating glucose was determined using an enzymatic/colorimetric method with commercial kit (GOD-POP; Spinreact), adapted to microplates (Gómez-Boronat et al., 2020).
Analysis of mRNA Abundance
Total RNA from hypothalamus and liver were isolated using TRI® Reagent (Sigma-Aldrich) and treated with RQ1 RNase-Free DNase (Promega), according to the manufacturer’s instructions. Then, 0.3 μg of total RNA was reverse transcribed into cDNA in a 25 μL reaction volume using random primers (Invitrogen), RNase inhibitor (Promega), and SuperScript II Reverse Transcriptase (Invitrogen). Real-Time quantitative PCRs (RT-qPCRs) were carried out in duplicates in a CFX96™ Real-Time System (Bio-Rad Laboratories, Hercules, USA), using iTaq™ Universal SYBR® Green Supermix (Bio-Rad Laboratories) into a 96-well plate loaded with 1 μL of cDNA and 0.5 μM of each forward and reverse primer in a final volume of 10 μL. Each PCR run also included a 4-points serial dilution curve and non-retrotranscribed-RNA and water as negative control. The RT-qPCR cycling conditions consisted of an initial denaturation at 95°C for 30 s and 40 cycles of a two-step amplification program (95°C for 5 s and 60°C for 30 s). A melting curve was systematically monitored (temperature gradient at 0.5°C/5 s from 70 to 90°C) at the end of each run, to confirm the specificity of the amplification reaction. The Gene Data Bank reference numbers and the primers (Sigma-Aldrich) sequences employed for target genes (clock genes: per1a, per1b, per2a, per3, clock1a, and bmal1a; clock-related NRs: pparα, pparγ, nr1d1, nr1d2 b, and rorα a) and the reference gene elongation factor 1α (eef-1a1) are shown in Table 1. The 2−ΔΔCt method (Livak and Schmittgen, 2001) was used to determine the relative mRNA abundance (fold change), normalizing to the group with the lowest level in each gene.
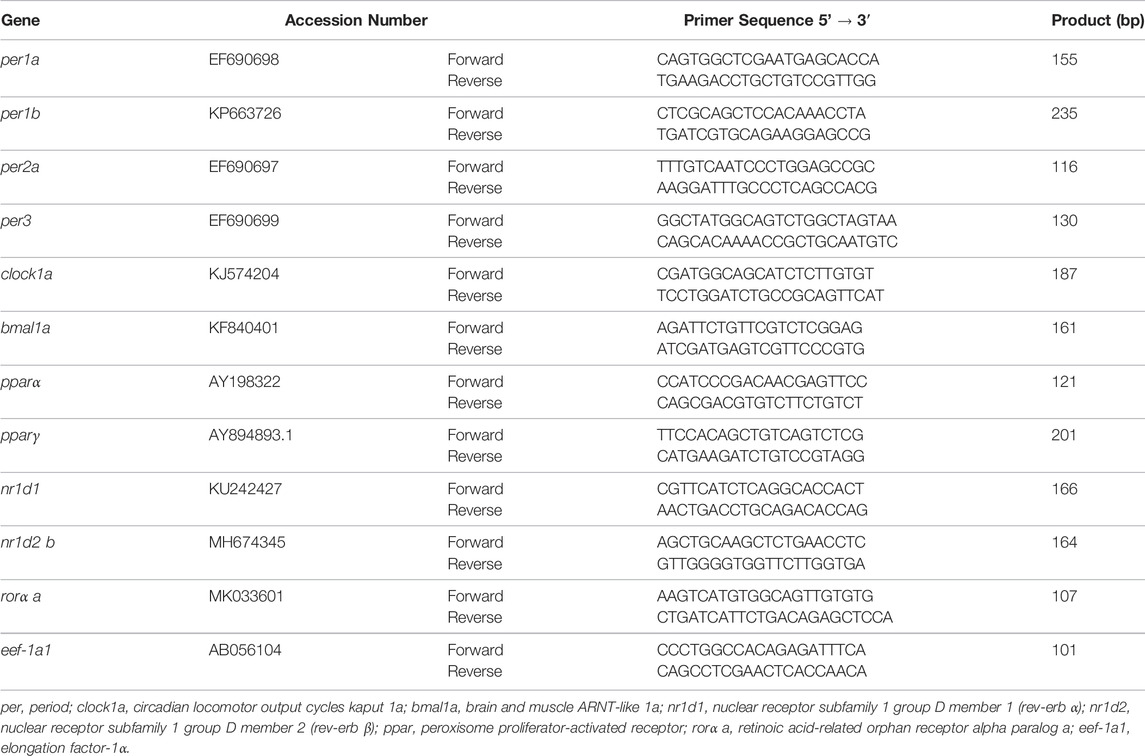
TABLE 1. Accession numbers of the genes and primers sequences employed in quantitative RT-qPCR studies.
Data Analysis
A one-way ANOVA followed by the post hoc Student-Newman-Keuls (SNK) test was performed to compare gene expression at different sampling points (SigmaPlot 12.0 statistics package). When necessary, data were transformed to logarithmic or square root scale to normalize and to obtain homoscedasticity. A probability level of p < 0.05 was considered statistically significant in all tests. Daily (24-h) significant rhythms were determined by Cosinor analysis fitting the data to sinusoidal functions by the least squares method (Duggleby, 1981). The formula used was f(t) = M + A*cos(t*π/12-Φ), where f(t) is the gene expression level at a given time, the mesor (M) is the mean value, A is the sinusoidal amplitude of oscillation, t is time in hours, and Φ is the acrophase. Non-linear regression allows the estimation of M, A, Φ and their standard errors (SE), which are calculated on the residual sum of squares in the least-squares fit (Duggleby, 1981; Delgado et al., 1993). Significance of Cosinor analysis was defined by the noise/signal of amplitude calculated from the ratio SE(A)/A (Nisembaum et al., 2012). Data were considered to display a significant daily rhythm if fulfil these criteria: p < 0.05 by ANOVA, SE(A)/A < 0.3 by Cosinor analysis, and A > 1 by Cosinor analysis.
Results
In the hypothalamus of the animals maintained under 12L:12D and scheduled feeding at ZT 2 (Group I) the mRNA abundance of all of the studied clock genes (per1a, per1b, per2a, per3, and bmal1a) displayed significant 24-h rhythms (Figures 1A–D,F), except clock1a, whose transcript showed significant changes through the 24-h (ANOVA) but not a significant rhythm by the Cosinor analysis (Figure 1E). The acrophases of daily rhythms of per1 genes took place at the end of the dark phase (ZT 22.3 for per1a and ZT 23.1 for per1b) or at the light onset (ZT 1.0) for per3. These rhythmic profiles were in antiphase with that shown by bmal1a (ZT 8.8). Hypothalamic per2a expression peaked at ZT 4.3 (Figure 1C). The expression profiles of clock genes in the Group II (12L:12D, random feeding) also exhibited daily rhythms in the hypothalamus (Figures 1G–J,L), except for clock1a (Figure 1K). The acrophases of all the studied genes were similar to those observed in the Group I, while the amplitudes were slightly reduced. Finally, the hypothalamic rhythmicity of clock genes of the Group III (24D, scheduled feeding at CT 2) was totally lost (Figures 1M–P,R,S).
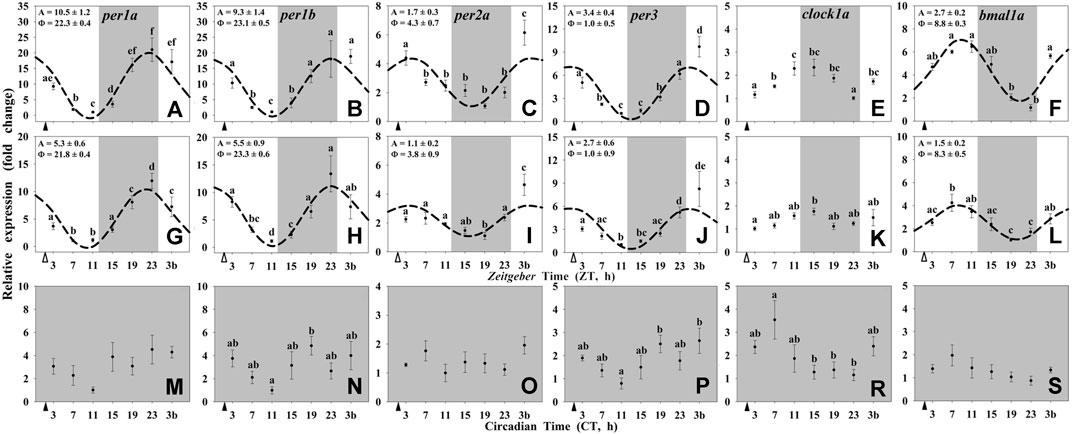
FIGURE 1. Daily profile of clock genes transcripts in the hypothalamus of goldfish maintained under different lighting conditions and feeding protocols. Grey area indicates the dark period. Feeding time is indicated by triangles in the x-axis (solid, ▲, scheduled feeding at ZT/CT 2; white, ∆, random feeding with the last meal at ZT 2). Data are shown as mean ± SEM (n = 5–7) in relative units (2−ΔΔCt method). Different letters indicate significant differences (ANOVA p < 0.05, SNK post hoc test). When Cosinor (SE(A)/A < 0.3) was significant and A > 1, periodic sinusoidal functions were represented as dashed curves with the corresponding amplitudes and acrophases (A and Φ, respectively).
Most of the clock genes in the liver of goldfish (per1a, per1b, per3, bmal1a, and clock1a) were rhythmically expressed in the three experimental groups (Figure 2), except for per2a which did not show daily rhythmicity in any of the studied groups (Figures 2C,I,O), and clock1a which lost its 24-h rhythm under constant dark (Group III; Figure 2R). The acrophases of the rhythms of per genes in the liver of goldfish reared under 12L:12D and fed at ZT2 (group I) were found at the end of the dark phase (ZT 22.7 for per1a and ZT 22.5 for per1b; Figures 2A,B) and at the light onset for per3 (ZT 0.5; Figure 2D), while that for clock1a was found at ZT 5.4 (Figure 2E) and at ZT 7.7 for bmal1a (Figure 2F). These rhythmic profiles of hepatic clock genes (Figures 2A–F) were similar to those observed in the hypothalamus from the same animals (Figures 1A–F), while the amplitudes were higher than in brain tissue.
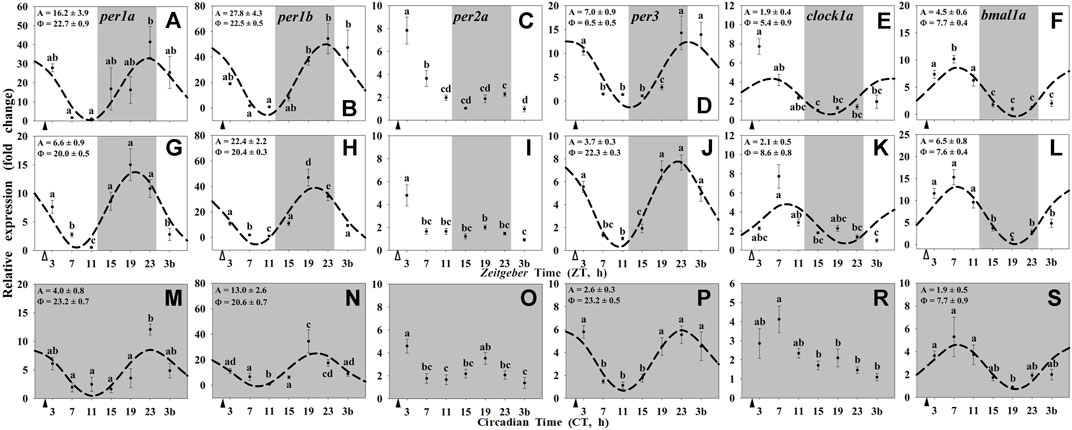
FIGURE 2. Daily profile of clock genes transcripts in the liver of goldfish maintained under different lighting conditions and feeding protocols. Grey area indicates the dark period. Feeding time is indicated by triangles in the x-axis (solid, ▲, scheduled feeding at ZT/CT 2; white, ∆, random feeding with the last meal at ZT 2). Data are shown as mean ± SEM (n = 5–7) in relative units (2−ΔΔCt method). Different letters indicate significant differences (ANOVA p < 0.05, SNK post hoc test). When Cosinor (SE(A)/A < 0.3) was significant and A > 1, periodic sinusoidal functions were represented as dashed curves with the corresponding amplitudes and acrophases (A and Φ, respectively).
In the randomly fed fish (Group II), the acrophases of per genes in the liver (Figures 2G–J) were slightly advanced (2 h) compared with the hypothalamus (Figures 1G–J), while the transcripts of clock1a peaked 3 h later and the rhythmic profile of bmal1a remained unchanged (Figure 2K, L vs. Figures 1K,L). Additionally, the amplitudes of clock genes rhythms in randomly fed fishes were reduced (per genes; Figures 2G–J) or remained almost unchanged (clock1a and bmal1a genes; Figures 2K,L) respect to Group I (Figures 2A–F). Regarding the constant dark conditions (Group III), the acrophases of per genes were found at the end of the subjective scotophase (CT 21-23; Figures 2M,N,P), and the profile were in antiphase with bmal1a (CT 7.7; Figure 2S). Amplitudes of all rhythmic clock genes in the liver of fish under constant darkness (Figures 2M–P,R,S) were strongly reduced compared with animals maintained under a LD cycle (Figures 2A–L).
In the hypothalamus of goldfish, only the NR nr1d1 showed a significant daily rhythmic expression with a peak at middark (around ZT 17-18; Figures 3). Moreover, such rhythm only occurred when the LD cycle was present (Groups I and II; Figures 3C,H), and showed a high 4-fold reduction in the amplitude in randomly fed animals. Under constant darkness (Group III), any of the NRs studied expressed 24-h rhythmic variations in the hypothalamus of goldfish (Figures 3K–O).
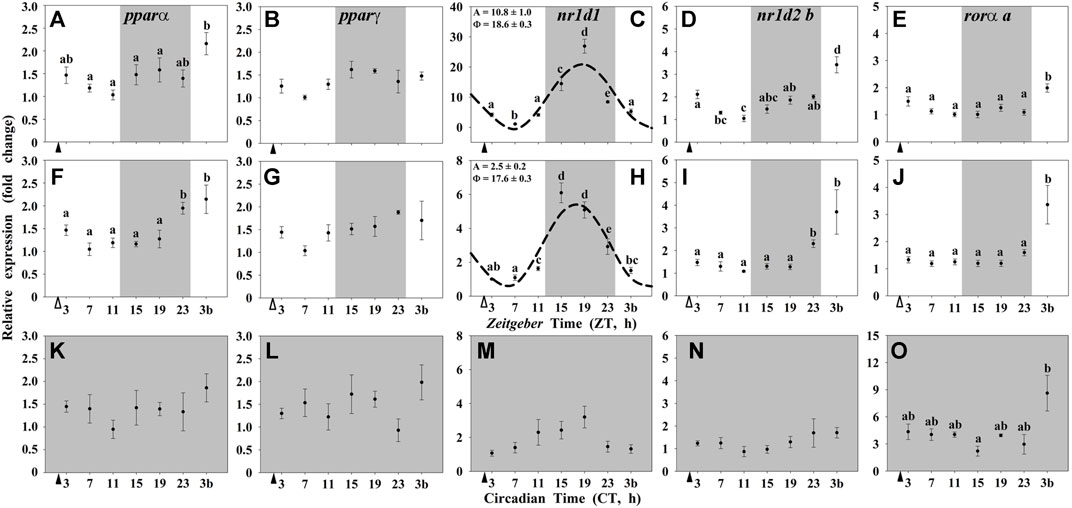
FIGURE 3. Daily profile of NRs transcripts in the hypothalamus of goldfish maintained under different lighting conditions and feeding protocols. Grey area indicates the dark period. Feeding time is indicated by triangles in the x-axis (solid, ▲, scheduled feeding at ZT/CT 2; white, ∆, random feeding with the last meal at ZT 2). Data are shown as mean ± SEM (n = 5–7) in relative units (2−ΔΔCt method). Different letters indicate significant differences (ANOVA p < 0.05, SNK post hoc test). When Cosinor (SE(A)/A < 0.3) was significant and A > 1, periodic sinusoidal functions were represented as dashed curves with the corresponding amplitudes and acrophases (A and Φ, respectively).
By contrast, most of the studied NRs in the liver (pparα, nr1d1, nr1d2 b, and rorα a) showed daily significant rhythms when both zeitgebers were present (Group I; Figures 4A–E). The acrophases of pparα and nr1d2 b rhythms occurred at the light onset (ZT 0.3 and ZT 0.1, respectively; Figures 4A,D), nr1d1 peaked at the middle of the dark period (ZT 17.5; Figure 4C), and rorα a displayed its maximum of expression in the early morning (ZT 2.9; Figure 4E). The acrophase of nr1d1 occurred during the scotophase in the liver (Figure 4C), as in the hypothalamus (Figure 3C), while the amplitude was twice in the peripheral vs. the encephalic tissue. These daily patterns of expression remained as significant rhythms only for pparα and nr1d1 in fish randomly fed (Group II, Figures 4F–J), with the acrophases during the dark phase (ZT 22 for pparα and ZT 16.9 for nr1d1). Comparing with the hypothalamus, the peak of nr1d1 remained unchanged, but the amplitude was reduced. In Group III (24D, scheduled feeding CT 2), only nr1d1 was still rhythmic (Figure 4K–O) with similar acrophases and amplitudes as in Group I (Figure 4C).
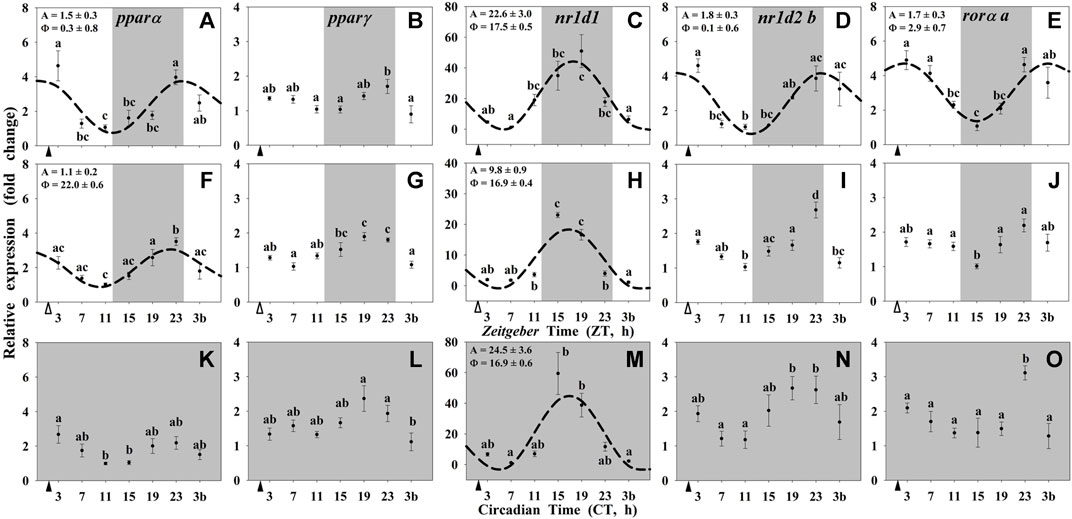
FIGURE 4. Daily profile of NRs transcripts in the liver of goldfish maintained under different lighting conditions and feeding protocols. Grey area indicates the dark period. Feeding time is indicated by triangles in the x-axis (solid, ▲, scheduled feeding at ZT/CT 2; white, ∆, random feeding with the last meal at ZT 2). Data are shown as mean ± SEM (n = 5–7) in relative units (2−ΔΔCt method). Different letters indicate significant differences (ANOVA p < 0.05, SNK post hoc test). When Cosinor (SE(A)/A < 0.3) was significant and A > 1, periodic sinusoidal functions were represented as dashed curves with the corresponding amplitudes and acrophases (A and Φ, respectively).
Finally, the circulating levels of glucose are shown in Figure 5. In the three experimental conditions, the highest levels of circulating glucose were observed at 1-h postprandial, while the lowest was found during the dark (resting) period. At the end of the night (or subjective night) an increase in glucose levels, that was extended to the beginning of the photophase (3b sampling point), was observed in all the animals, including unfed fish. However, significant daily rhythms were only found in randomly fed (Figure 5B) and constant darkness maintained animals (Figure 5C).
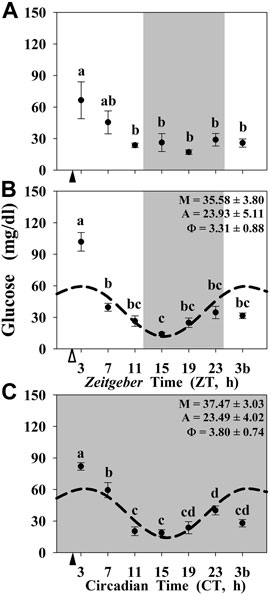
FIGURE 5. Daily profile of plasma glucose levels in goldfish maintained under different lighting conditions and feeding protocols. Grey area indicates the dark period. Feeding time is indicated by triangles in the x-axis (solid, ▲, scheduled feeding at ZT/CT 2; white, ∆, random feeding with the last meal at ZT 2). Data are shown as mean ± SEM (n = 5–7). Different letters indicate significant differences (ANOVA p < 0.05, SNK post hoc test). When Cosinor (SE(A)/A < 0.3) was significant and A > 1, periodic sinusoidal functions were represented as dashed curves with the corresponding amplitudes and acrophases (A and Φ, respectively).
Discussion
Results obtained in this work evidence that the absence of one of the main zeitgebers (LD or feeding-fasting cycles) impairs the clock circuitry in a tissue-dependent manner when comparing a central oscillator (hypothalamus) with a peripheral one (liver) in goldfish. Our data also support the idea that the hypothalamus behaves as an authentic LEO, while the liver acts as a true FEO in goldfish (Sánchez-Bretaño et al., 2015a; Isorna et al., 2017; Gómez-Boronat et al., 2018). Nevertheless, the presence of both zeitgebers increases the amplitudes of rhythms in both tissues, probably giving them more robustness to act as timekeepers.
Regarding the core clock of the oscillators, when both zeitgebers are present, both the hypothalamic and the hepatic clocks displayed significant daily rhythms of the negative-limb clock genes (per) with acrophases at the end of the scotophase or at the onset of the photophase, while the daily expression rhythms of the positive-limb clock genes (clock1a and bmal1a) peaked at the end of the photophase. These results show that both the positive and the negative-limb genes are in antiphase, suggesting that these are functional clocks as expected, in accordance with previous results in goldfish also maintained in 12L:12D photoperiod and fed during the photophase (Velarde et al., 2009; Sánchez-Bretaño et al., 2015b; Gómez-Boronat et al., 2018). However, the hypothalamic clock of fish under constant darkness was impaired, as daily rhythms of clock genes expression were lost, making a non-functional oscillator, even when maintaining the scheduled feeding. This is an unexpected result, since a scheduled feeding time under constant light was able to synchronize the daily rhythms of clock genes in central tissues (optic tectum and hypothalamus) of this teleost (goldfish; Feliciano et al., 2011). A possible explanation may lie in the clock gene per2. This is a light-inducible gene and a key regulator of the circadian molecular clock in mammals (Kim et al., 2018) and fish (Vatine et al., 2009; Beale et al., 2013; Frøland Steindal et al., 2018). The absence of light is probably the responsible of the very low abundance of per2 in fish reared under constant darkness, and under these conditions, this gene could not regulate the circadian clock machinery, turning the hypothalamus into a non-rhythmic oscillator. In constant light, a high abundance of per2 expression is expected (Vatine et al., 2009; Isorna et al., 2017), thus this element would be present, and the clockwork could be not so altered as in constant darkness. On the other hand, the lack of the scheduled feeding did not modify the expression of any hypothalamic clock genes when the LD cycle was present. Together these data are consistent with those observed in mammals (Mendoza and Challet, 2009), and previously reported in fish (López-Olmeda et al., 2010; Gómez-Boronat et al., 2018), and point out that the hypothalamus of goldfish is entrained by the LD cycle behaving as a LEO.
Conversely, the hepatic clock of the fish maintained in darkness seems to remain functional, as daily rhythms of clock genes were preserved. This result agrees with previous results in goldfish under constant light, where a scheduled feeding synchronizes daily rhythms of clock genes in liver and intestine (Feliciano et al., 2011; Nisembaum et al., 2012). However, when the LD cycle was present, such rhythms exhibited greater amplitudes, supporting that both external zeitgebers (LD and feeding-fasting cycles) work together sustaining the molecular clock mechanism, at least in the gastrointestinal tissues (Nisembaum et al., 2012; Sánchez-Bretaño et al., 2015a). All current data support the idea that the liver is entrained by the feeding-fasting cycle, acting as a FEO in both, mammals and fish (Mendoza and Challet, 2009; López-Olmeda et al., 2010; Gómez-Boronat et al., 2018). In fact a single temporal change in the food availability can resynchronize peripheral clocks, such as the liver in fish (Feliciano et al., 2011; Gómez-Boronat et al., 2018) as well as in mammals (Lamia et al., 2008; Patton and Mistlberger, 2013).
It is worth noting that food-derived molecules, lipid-soluble nutrients, and hormones can activate transcription factors of the nuclear receptor family, such as REV-ERBs, RORs, and PPARs, among many others (Duez and Staels, 2009; Albrecht and Ripperger, 2018). Therefore, it was expected that these nuclear receptors, that are key elements of the biological clocks’ machinery, were also driven by feeding time. This seems to be the case in the liver, but not in the hypothalamus of goldfish, where the studied nuclear receptors, as clock-controlled genes, seem to be mainly driven by the clock itself, instead of by putative metabolic ligands. Our results showed that in the hypothalamus, only nr1d1 (rev-erbα) displayed significant daily rhythms under a LD cycle in both feeding conditions, scheduled and random feeding. Recently a rhythm in nr1d1 has been reported in the whole brain in Atlantic salmon (Salmo salar) reared under a 12L:12D photoperiod with acrophases during the middle-late scotophase (Bolton et al., 2021) as in goldfish. Interestingly, when goldfish were maintained under constant darkness, such rhythm disappeared, and any nuclear receptor mRNA had oscillations, which coincides with the loss of the clock gene rhythms in these animals and reinforces the hypothesis that the hypothalamus is a classical LEO. The existence of nr1d1 rhythms driven by the LD cycle in the hypothalamus, but not entrained by feeding-fasting cycle, suggest that other functions beyond the known metabolic role of REV-ERBα are probably linked to this transcription factor in the hypothalamus. Among such putative functions, it has been proposed that REV-ERBα acts as a repressor of the key enzyme for dopamine synthesis, tyrosine hydroxylase, in mammals (Chung et al., 2014; Mendoza, 2019). This action could be a link for a crosstalk between the circadian system and brain functioning by modulating anxiety, addiction and the hedonic system, among others (Banerjee et al., 2014; Chung et al., 2014; Mendoza, 2019). Putative targets of REV-ERBα in fish brain and its relevance for the temporal control of neural functions need to be explored in the future.
On the other hand, all the nuclear receptors showed daily rhythmic patterns of expression in the liver of goldfish when both zeitgebers were present, except pparγ. Interestingly, in random-fed fish only pparα and nr1d1 were rhythmic and the latter in the 24D fish. The scheduled feeding in both conditions, 12L:12D and 24D, preserved high amplitudes of expression of nr1d1, while in random-fed fish the amplitude was considerably diminished. These results also support the idea that the liver of goldfish woks as a FEO mainly driven by feeding time (Isorna et al., 2017; Gómez-Boronat et al., 2018), and that REV-ERBα is an important element to maintain the temporal homeostasis in this organ.
In the last decades, it is increasingly being developed and established the idea that the circadian system not only controls the metabolic regulation as an output, but also the metabolic status can signals back to the clocks in order to reinforce circadian rhythmicity (Reinke and Asher, 2016; Kolbe et al., 2018; Reinke and Asher, 2019). Indeed, at least 20% of the whole transcriptome in peripheral organs of mouse are rhythmic (Zhang et al., 2014; Panda, 2016), including genes involved in lipid and glucose metabolism, and most of the transcription factors belonging to the nuclear receptor family (Yang et al., 2006). In fish, around 15% of 13,939 genes studied in sea bream also were also rhythmic, including genes of the molecular clock, metabolic genes, and nuclear receptors (Yúfera et al., 2017).
The daily rhythms shown by nuclear receptors in the liver of goldfish, and particularly, the rhythmic expression of nr1d1 even in constant darkness, suggest their possible implication in the regulation of the temporal homeostasis. Complementary to their contribution to the circadian system, all the described nuclear receptors exert multiple regulatory functions on several metabolic pathways, being the most important REV-ERBα and PPARα (Yang et al., 2007; Kojetin and Burris, 2014; Pawlak et al., 2015; Berthier et al., 2018). These nuclear receptors could play a key role in the control of hepatic glucose and lipid metabolism, which is supported by the preserved rhythmic expression of REV-ERBα in the liver, but not in the hypothalamus of goldfish under constant darkness. In this sense, daily rhythm of nr1d1 expression in the liver of goldfish peaked at the mid-scotophase (or subjective), i.e., during the rest phase, as these animals were diurnal (personal observations). In addition, the lowest levels of plasma glucose occurred during the scotophase, matching with this maximum hepatic expression of nr1d1, which might be explained by the effects that this nuclear receptor exerts on glucose metabolism during fasting/resting phase observed in mammals, downregulating the expression of the gluconeogenic enzymes (PEPCK and G6Pase) and modulating the actions of insulin in mammals (Yin et al., 2007; Vieira et al., 2015; Berthier et al., 2018) and fish (Saiz et al., 2022). On the other hand, plasma glucose raised at the end of the night peaking few hours after the mealtime. Such increment before light onset coincides with the daily rhythm of rorα in the liver of goldfish in presence of both zeitgebers. In rorα−/- mice a reduced blood glucose levels has been proposed (Kang et al., 2007). It could be possible that hepatic RORα increases plasmatic glucose also in goldfish.
The other nuclear receptor largely involved in the hepatic metabolism is the pparα, which showed a daily rhythm of expression with the acrophase placed only a few hours before the last meal in fish exposed to a light/dark cycle, with either scheduled or random feeding. These results are in agreement with those observed in mammals, and support the possible role of this nuclear receptor as responsible for preparing the liver to be able to respond to the lipids that income with the food (Berger and Moller, 2002; Chakravarthy et al., 2009; Liu et al., 2014; Pawlak et al., 2015). Moreover, PPARα is upregulated during the rest phase of the animals, peaking at the end of daytime in nocturnal mammals (Yang et al., 2006; Chen et al., 2010), or at the end of nighttime in diurnal fish (Paredes et al., 2014). During this resting and fasting states, animals obtain energy from increasing the hepatic fatty oxidation (Ribas-Latre and Eckel-Mahan, 2016), metabolic process that is promoted by PPARα in mammals (Chen and Yang, 2014; Liu et al., 2014). Current PPARα expression rhythms confirm our previous results (Gómez-Boronat et al., 2019) and are in agreement to those observed in mammals, suggesting preserved functions throughout the phylogeny.
Interestingly, we observed that the postprandial peak of circulating glucose is overlapped with the basal daily rhythms of this metabolite, which can be observed only when one zeitgeber (LD cycle or scheduled feeding) was present. In the three experimental conditions, we detect significant differences between 1-h postprandial (ZT 3/CT 3) and 25-h fasting (ZT 3b/CT 3b) regardless of the photoperiod and scheduled feeding conditions. These data suggest that daily glucose rhythms are directly controlled by the feeding in fish, as seen in mammals (Kasanen et al., 2018). However, this is not the case for NRs expression. In contrast to circulating glucose, we did not obtain any difference between 1-h postprandial and 25-h-fasting in the gene expression of NRs either in the liver or in the hypothalamus of goldfish. Indeed, in the 24D fish neither the hypothalamic core clock genes nor the studied NRs show daily oscillations. However, the hepatic clock genes and nr1d1 were rhythmic in these animals reared in 24D as well as in the absence of a scheduled feeding. All these obtained data point to NRs being clear outputs of the circadian system, being influenced by the core clock of the oscillators, and not being masked by the feeding-fasting cycle.
In summary, our results evidence the circadian nature of the hepatic oscillator as well as some nuclear clock-related receptors in teleost fish and reinforce the idea that the liver is mainly synchronized by the feeding-fasting cycle, while the light-dark cycle is the main zeitgeber entraining the clock genes in the hypothalamus of goldfish. In addition, and bearing in mind the many implications they have, nuclear receptors are being discovered as real outputs of the circadian system acting as very important molecules for the timekeeping of temporal homeostasis, and independently of the energy status in fish.
Data Availability Statement
The datasets presented in this study can be found in online repositories. The names of the repository/repositories and accession number(s) can be found below: https://www.ncbi.nlm.nih.gov/genbank/, MK033601 https://www.ncbi.nlm.nih.gov/genbank/, MH674345.
Ethics Statement
The animal study was reviewed and approved by Community of Madrid (PROEX 170.6/20).
Author Contributions
EI, MG-B, MD, and ND conceived and designed the experiments, interpreted findings, drafted, and revised the manuscript. MG-B, AA-G, and EI analyzed the samples. All authors participated in sampling animals.
Funding
This study was supported by grants from Ministerio de Ciencia e Innovación to MD and ND (PID2019-103969RB-C32). MG-B was recipient of a predoctoral fellow from Ministerio de Ciencia e Innovación (BES-2014-068103). AA-G, EI, MD and ND belong to a network on fish chronobiology (RED2018-102487-T).
Conflict of Interest
The authors declare that the research was conducted in the absence of any commercial or financial relationships that could be construed as a potential conflict of interest.
Publisher’s Note
All claims expressed in this article are solely those of the authors and do not necessarily represent those of their affiliated organizations, or those of the publisher, the editors and the reviewers. Any product that may be evaluated in this article, or claim that may be made by its manufacturer, is not guaranteed or endorsed by the publisher.
References
Albrecht U., Ripperger J. A. (2018). Circadian Clocks and Sleep: Impact of Rhythmic Metabolism and Waste Clearance on the Brain. Trends Neurosci. 41, 677–688. doi:10.1016/j.tins.2018.07.007
Albrecht U. (2012). Timing to Perfection: The Biology of Central and Peripheral Circadian Clocks. Neuron 74, 246–260. doi:10.1016/j.neuron.2012.04.006
Aranda A., Madrid J. A., Sánchez-Vázquez F. J. (2001). Influence of Light on Feeding Anticipatory Activity in Goldfish. J. Biol. Rhythms 16, 50–57. doi:10.1177/074873040101600106
Banerjee S., Wang Y., Solt L. A., Griffett K., Kazantzis M., Amador A., et al. (2014). Pharmacological Targeting of the Mammalian Clock Regulates Sleep Architecture and Emotional Behaviour. Nat. Commun. 5, 5759. doi:10.1038/ncomms6759
Beale A., Guibal C., Tamai T. K., Klotz L., Cowen S., Peyric E., et al. (2013). Circadian Rhythms in Mexican Blind Cavefish Astyanax Mexicanus in the Lab and in the Field. Nat. Commun. 4, 2769. doi:10.1038/ncomms3769
Berger J., Moller D. E. (2002). The Mechanisms of Action of PPARs. Annu. Rev. Med. 53, 409–435. doi:10.1146/annurev.med.53.082901.104018
Berthier A., Johanns M., Zummo F. P., Lefebvre P., Staels B. (2021). PPARs in Liver Physiology. Biochim. Biophys. Acta BBA - Mol. Basis Dis. 1867, 166097. doi:10.1016/j.bbadis.2021.166097
Berthier A., Vinod M., Porez G., Steenackers A., Alexandre J., Yamakawa N., et al. (2018). Combinatorial Regulation of Hepatic Cytoplasmic Signaling and Nuclear Transcriptional Events by the OGT/REV-ERBα Complex. Proc. Natl. Acad. Sci. U.S.A. 115, E11033–E11042. doi:10.1073/pnas.1805397115
Bolton C. M., Bekaert M., Eilertsen M., Helvik J. V., Migaud H. (2021). Rhythmic Clock Gene Expression in Atlantic Salmon Parr Brain. Front. Physiol. 12, 761109. doi:10.3389/fphys.2021.761109
Cavallari N., Frigato E., Vallone D., Fröhlich N., Lopez-Olmeda J. F., Foà A., et al. (2011). A Blind Circadian Clock in Cavefish Reveals that Opsins Mediate Peripheral Clock Photoreception. PLOS Biol. 9, e1001142. doi:10.1371/journal.pbio.1001142
Chakravarthy M. V., Lodhi I. J., Yin L., Malapaka R. R. V., Xu H. E., Turk J., et al. (2009). Identification of a Physiologically Relevant Endogenous Ligand for PPARα in Liver. Cell 138, 476–488. doi:10.1016/j.cell.2009.05.036
Challet E. (2015). Keeping Circadian Time with Hormones. Diabetes Obes. Metab. 17, 76–83. doi:10.1111/dom.12516
Chen L., Yang G. (2014). PPARs Integrate the Mammalian Clock and Energy Metabolism. PPAR Res. 2014, 1–6. doi:10.1155/2014/653017
Chen P., Kakan X., Zhang J. (2010). Altered Circadian Rhythm of the Clock Genes in Fibrotic Livers Induced by Carbon Tetrachloride. FEBS Lett. 584, 1597–1601. doi:10.1016/j.febslet.2010.03.019
Chung S., Lee E. J., Yun S., Choe H. K., Park S.-B., Son H. J., et al. (2014). Impact of Circadian Nuclear Receptor REV-ERBα on Midbrain Dopamine Production and Mood Regulation. Cell 157, 858–868. doi:10.1016/j.cell.2014.03.039
Cook D. N., Kang H. S., Jetten A. M. (2015). Retinoic Acid-Related Orphan Receptors (RORs): Regulatory Functions in Immunity, Development, Circadian Rhythm, and Metabolism. Nucl. Recept. Res. 2, 101185. doi:10.11131/2015/101185
Cowan M., Azpeleta C., López-Olmeda J. F. (2017). Rhythms in the Endocrine System of Fish: A Review. J. Comp. Physiol. B 187, 1057–1089. doi:10.1007/s00360-017-1094-5
Delgado M. J., Aeonso-Gómez A. L., Gancedo B., de Pedro N., Valenciano A. I., Alonso-Bedate M. (1993). Serotonin N-Acetyltransferase (NAT) Activity and Melatonin Levels in the Frog Retina are not Correlated during the Seasonal Cycle. Gen. Comp. Endocrinol. 92, 143–150. doi:10.1006/gcen.1993.1151
Duez H., Staels B. (2009). Rev-erb-α: An Integrator of Circadian Rhythms and Metabolism. J. Appl. Physiol. 107, 1972–1980. doi:10.1152/japplphysiol.00570.2009
Duggleby R. G. (1981). A Nonlinear Regression Program for Small Computers. Anal. Biochem. 110, 9–18. doi:10.1016/0003-2697(81)90104-4
Duszka K., Wahli W. (2020). Peroxisome Proliferator-Activated Receptors as Molecular Links between Caloric Restriction and Circadian Rhythm. Nutrients 12, 3476. doi:10.3390/nu12113476
Feliciano A., Vivas Y., de Pedro N., Delgado M. J., Velarde E., Isorna E. (2011). Feeding Time Synchronizes Clock Gene Rhythmic Expression in Brain and Liver of Goldfish (Carassius Auratus). J. Biol. Rhythms 26, 24–33. doi:10.1177/0748730410388600
Frøland Steindal I. A., Beale A. D., Yamamoto Y., Whitmore D. (2018). Development of the Astyanax Mexicanus Circadian Clock and Non-Visual Light Responses. Dev. Biol. 441, 345–354. doi:10.1016/j.ydbio.2018.06.008
Gómez-Boronat M., Isorna E., Armirotti A., Delgado M. J., Piomelli D., de Pedro N. (2019). Diurnal Profiles of N-Acylethanolamines in Goldfish Brain and Gastrointestinal Tract: Possible Role of Feeding. Front. Neurosci. 13, 450. doi:10.3389/fnins.2019.00450
Gómez-Boronat M., Isorna E., Conde-Sieira M., Delgado M. J., Soengas J. L., De Pedro N. (2020). First Evidence on the Role of Palmitoylethanolamide in Energy Homeostasis in Fish. Horm. Behav. 117, 104609. doi:10.1016/j.yhbeh.2019.104609
Gómez-Boronat M., Sáiz N., Delgado M. J., de Pedro N., Isorna E. (2018). Time-lag in Feeding Schedule Acts as a Stressor that Alters Circadian Oscillators in Goldfish. Front. Physiol. 9, 1749. doi:10.3389/fphys.2018.01749
Isorna E., de Pedro N., Valenciano A. I., Alonso-Gómez Á. L., Delgado M. J. (2017). Interplay between the Endocrine and Circadian Systems in Fishes. J. Endocrinol. 232, R141–R159. doi:10.1530/JOE-16-0330
Kang H. S., Angers M., Beak J. Y., Wu X., Gimble J. M., Wada T., et al. (2007). Gene Expression Profiling Reveals a Regulatory Role for RORα and RORγ in Phase I and Phase II Metabolism. Physiol. Genomics 31, 281–294. doi:10.1152/physiolgenomics.00098.2007
Kasanen I., Inhilä K., Savontaus E., Voipio H.-M., Õkva K., Mering S., et al. (2018). Diurnal Rhythms of Blood Glucose, Serum Ghrelin, Faecal IgA and Faecal Corticosterone in Rats Subjected to Restricted Feeding Using the Diet Board. Lab. Anim. 52, 29–37. doi:10.1177/0023677217709850
Kim M., de la Peña J. B., Cheong J. H., Kim H. J. (2018). Neurobiological Functions of the Period Circadian Clock 2 Gene, Per2. Biomol. Ther. 26, 358–367. doi:10.4062/biomolther.2017.131
Kojetin D. J., Burris T. P. (2014). REV-ERB and ROR Nuclear Receptors as Drug Targets. Nat. Rev. Drug Discov. 13, 197–216. doi:10.1038/nrd4100
Kolbe I., Brehm N., Oster H. (2018). Interplay of Central and Peripheral Circadian Clocks in Energy Metabolism Regulation. J. Neuroendocrinol. 31, e12659. doi:10.1111/jne.12659
Lamia K. A., Storch K.-F., Weitz C. J. (2008). Physiological Significance of a Peripheral Tissue Circadian Clock. Proc. Natl. Acad. Sci. U.S.A. 105, 15172–15177. doi:10.1073/pnas.0806717105
Lee J. M., Kim H., Baek S. H. (2021). Unraveling the Physiological Roles of Retinoic Acid Receptor-Related Orphan Receptor α. Exp. Mol. Med. 53, 1278–1286. doi:10.1038/s12276-021-00679-8
Liu S., Alexander R. K., Lee C.-H. (2014). Lipid Metabolites as Metabolic Messengers in Inter-Organ Communication. Trends Endocrinol. Metab. TEM 25, 356–363. doi:10.1016/j.tem.2014.05.002
Livak K. J., Schmittgen T. D. (2001). Analysis of Relative Gene Expression Data Using Real-Time Quantitative PCR and the 2−ΔΔCT Method. Methods 25, 402–408. doi:10.1006/meth.2001.1262
López-Olmeda J. F., Montoya A., Oliveira C., Sánchez-Vázquez F. J. (2009). Synchronization to Light and Restricted-Feeding Schedules of Behavioral and Humoral Daily Rhythms in Gilthead Sea Bream (Sparus Aurata). Chronobiol. Int. 26, 1389–1408. doi:10.3109/07420520903421922
López-Olmeda J. F., Tartaglione E. V., de la Iglesia H. O., Sánchez-Vázquez F. J. (2010). Feeding Entrainment of Food-Anticipatory Activity and Per1 Expression in the Brain and Liver of Zebrafish under Different Lighting and Feeding Conditions. Chronobiol. Int. 27, 1380–1400. doi:10.3109/07420528.2010.501926
Mendoza J., Challet E. (2009). Brain Clocks: From the Suprachiasmatic Nuclei to a Cerebral Network. Neuroscientist 15, 477–488. doi:10.1177/1073858408327808
Mendoza J. (2019). Food Intake and Addictive-Like Eating Behaviors: Time to Think about the Circadian Clock(s). Neurosci. Biobehav. Rev. 106, 122–132. doi:10.1016/j.neubiorev.2018.07.003
Montoya A., López-Olmeda J. F., Garayzar A. B. S., Sánchez-Vázquez F. J. (2010). Synchronization of Daily Rhythms of Locomotor Activity and Plasma Glucose, Cortisol and Thyroid Hormones to Feeding in Gilthead Seabream (Sparus Aurata) under a Light–Dark Cycle. Physiol. Behav. 101, 101–107. doi:10.1016/j.physbeh.2010.04.019
Nader N., Chrousos G. P., Kino T. (2010). Interactions of the Circadian CLOCK System and the HPA Axis. Trends Endocrinol. Metab. TEM 21, 277–286. doi:10.1016/j.tem.2009.12.011
Nisembaum L. G., Velarde E., Tinoco A. B., Azpeleta C., de Pedro N., Alonso-Gómez A. L., et al. (2012). Light-dark Cycle and Feeding Time Differentially Entrains the Gut Molecular Clock of the Goldfish (Carassius Auratus). Chronobiol. Int. 29, 665–673. doi:10.3109/07420528.2012.686947
Nuclear Receptors Nomenclature Committee (1999). A Unified Nomenclature System for the Nuclear Receptor Superfamily. Cell 97, 161–163. doi:10.1016/S0092-8674(00)80726-6
Panda S. (2016). Circadian Physiology of Metabolism. Science 354, 1008–1015. doi:10.1126/science.aah4967
Paredes J. F., Vera L. M., Martinez-Lopez F. J., Navarro I., Sánchez Vázquez F. J. (2014). Circadian Rhythms of Gene Expression of Lipid Metabolism in Gilthead Sea Bream Liver: Synchronisation to Light and Feeding Time. Chronobiol. Int. 31, 613–626. doi:10.3109/07420528.2014.881837
Patton D. F., Mistlberger R. E. (2013). Circadian Adaptations to Meal Timing: Neuroendocrine Mechanisms. Front. Neurosci. 7, 185. doi:10.3389/fnins.2013.00185
Pawlak M., Lefebvre P., Staels B. (2015). Molecular Mechanism of PPARα Action and its Impact on Lipid Metabolism, Inflammation and Fibrosis in Non-Alcoholic Fatty Liver Disease. J. Hepatol. 62, 720–733. doi:10.1016/j.jhep.2014.10.039
Reinke H., Asher G. (2016). Circadian Clock Control of Liver Metabolic Functions. Gastroenterology 150, 574–580. doi:10.1053/j.gastro.2015.11.043
Reinke H., Asher G. (2019). Crosstalk between Metabolism and Circadian Clocks. Nat. Rev. Mol. Cell Biol. 20, 227–241. doi:10.1038/s41580-018-0096-9
Ribas-Latre A., Eckel-Mahan K. (2016). Interdependence of Nutrient Metabolism and the Circadian Clock System: Importance for Metabolic Health. Mol. Metab. 5, 133–152. doi:10.1016/j.molmet.2015.12.006
Saiz N., Gómez-Boronat M., De Pedro N., Delgado M. J., Isorna E. (2021). The Lack of Light-Dark and Feeding-Fasting Cycles Alters Temporal Events in the Goldfish (Carassius Auratus) Stress Axis. Animals 11, 669. doi:10.3390/ani11030669
Saiz N., Herrera-Castillo L., Isorna E., Delgado M. J., Conde-Sieira M., Soengas J. L., et al. (2022). REV-ERBα Agonist SR9009 Promotes a Negative Energy Balance in Goldfish. Int. J. Mol. Sci. 23, 2921. doi:10.3390/ijms23062921
Sánchez-Bretaño A., Alonso-Gómez Á. L., Delgado M. J., Isorna E. (2015a). The Liver of Goldfish as a Component of the Circadian System: Integrating a Network of Signals. Gen. Comp. Endocrinol. 221, 213–216. doi:10.1016/j.ygcen.2015.05.001
Sánchez-Bretaño A., Gueguen M.-M., Cano-Nicolau J., Kah O., Alonso-Gómez Á. L., Delgado M. J., et al. (2015b). Anatomical Distribution and Daily Profile of Gper1b Gene Expression in Brain and Peripheral Structures of Goldfish (Carassius Auratus). Chronobiol. Int. 32, 889–902. doi:10.3109/07420528.2015.1049615
Schibler U., Gotic I., Saini C., Gos P., Curie T., Emmenegger Y., et al. (2015). Clock-Talk: Interactions between Central and Peripheral Circadian Oscillators in Mammals. Cold Spring Harb. Symp. Quant. Biol. 80, 223–232. doi:10.1101/sqb.2015.80.027490
Solt L. A., Kojetin D. J., Burris T. P. (2011). The REV-ERBs and RORs: Molecular Links between Circadian Rhythms and Lipid Homeostasis. Future Med. Chem. 3, 623–638. doi:10.4155/fmc.11.9
Stephan F. K. (2002). The “Other” Circadian System: Food as a Zeitgeber. J. Biol. Rhythms 17, 284–292. doi:10.1177/074873040201700402
Tahara Y., Shibata S. (2018). Entrainment of the Mouse Circadian Clock: Effects of Stress, Exercise, and Nutrition. Free Radic. Biol. Med. 119, 129–138. doi:10.1016/j.freeradbiomed.2017.12.026
Tinoco A. B., Nisembaum L. G., de Pedro N., Delgado M. J., Isorna E. (2014). Leptin Expression is Rhythmic in Brain and Liver of Goldfish (Carassius Auratus). Role of Feeding Time. Gen. Comp. Endocrinol. 204, 239–247. doi:10.1016/j.ygcen.2014.06.006
Tsang A. H., Barclay J. L., Oster H. (2014). Interactions between Endocrine and Circadian Systems. J. Mol. Endocrinol. 52, R1–R16. doi:10.1530/JME-13-0118
Vatine G., Vallone D., Appelbaum L., Mracek P., Ben-Moshe Z., Lahiri K., et al. (2009). Light Directs Zebrafish Period2 Expression via Conserved D and E Boxes. PLoS Biol. 7, e1000223. doi:10.1371/journal.pbio.1000223
Velarde E., Haque R., Iuvone P. M., Azpeleta C., Alonso-Gómez A. L., Delgado M. J. (2009). Circadian Clock Genes of Goldfish, Carassius Auratus: cDNA Cloning and Rhythmic Expression of Period and Cryptochrome Transcripts in Retina, Liver, and Gut. J. Biol. Rhythms 24, 104–113. doi:10.1177/0748730408329901
Vieira E., Merino B., Quesada I. (2015). Role of the Clock geneRev-Erbαin Metabolism and in the Endocrine Pancreas. Diabetes Obes. Metab. 17, 106–114. doi:10.1111/dom.12522
Weikum E. R., Liu X., Ortlund E. A. (2018). The Nuclear Receptor Superfamily: A Structural Perspective. Protein Sci. 27, 1876–1892. doi:10.1002/pro.3496
Yang X., Downes M., Yu R. T., Bookout A. L., He W., Straume M., et al. (2006). Nuclear Receptor Expression Links the Circadian Clock to Metabolism. Cell 126, 801–810. doi:10.1016/j.cell.2006.06.050
Yang X., Lamia K. A., Evans R. M. (2007). Nuclear Receptors, Metabolism, and the Circadian Clock. Cold Spring Harb. Symp. Quant. Biol. 72, 387–394. doi:10.1101/sqb.2007.72.058
Yin L., Wu N., Curtin J. C., Qatanani M., Szwergold N. R., Reid R. A., et al. (2007). Rev-erbα, a Heme Sensor that Coordinates Metabolic and Circadian Pathways. Science 318, 1786–1789. doi:10.1126/science.1150179
Yúfera M., Perera E., Mata-Sotres J. A., Calduch-Giner J., Martínez-Rodríguez G., Pérez-Sánchez J. (2017). The Circadian Transcriptome of Marine Fish (Sparus Aurata) Larvae Reveals Highly Synchronized Biological Processes at the Whole Organism Level. Sci. Rep. 7, 12943. doi:10.1038/s41598-017-13514-w
Keywords: circadian system, nr1c, nr1f, nr1d1, feeding-fasting cycle, light-dark cycle
Citation: Gómez-Boronat M, De Pedro N, Alonso-Gómez ÁL, Delgado MJ and Isorna E (2022) Nuclear Receptors (PPARs, REV-ERBs, RORs) and Clock Gene Rhythms in Goldfish (Carassius auratus) Are Differently Regulated in Hypothalamus and Liver. Front. Physiol. 13:903799. doi: 10.3389/fphys.2022.903799
Received: 24 March 2022; Accepted: 19 May 2022;
Published: 06 June 2022.
Edited by:
Jose Fernando Lopez-Olmeda, University of Murcia, SpainReviewed by:
Catarina Oliveira, University of Algarve, PortugalJuan Ignacio Bertucci, Spanish Institute of Oceanography (IEO), Spain
Copyright © 2022 Gómez-Boronat, De Pedro, Alonso-Gómez, Delgado and Isorna. This is an open-access article distributed under the terms of the Creative Commons Attribution License (CC BY). The use, distribution or reproduction in other forums is permitted, provided the original author(s) and the copyright owner(s) are credited and that the original publication in this journal is cited, in accordance with accepted academic practice. No use, distribution or reproduction is permitted which does not comply with these terms.
*Correspondence: Esther Isorna, ZWlzb3JuYWFAYmlvLnVjbS5lcw==
†Present address: Miguel Gómez-Boronat, Departamento de Odontología Preclínica, Facultad de Ciencias Biomédicas y de la Salud, Universidad Europea de Madrid, Madrid, Spain.