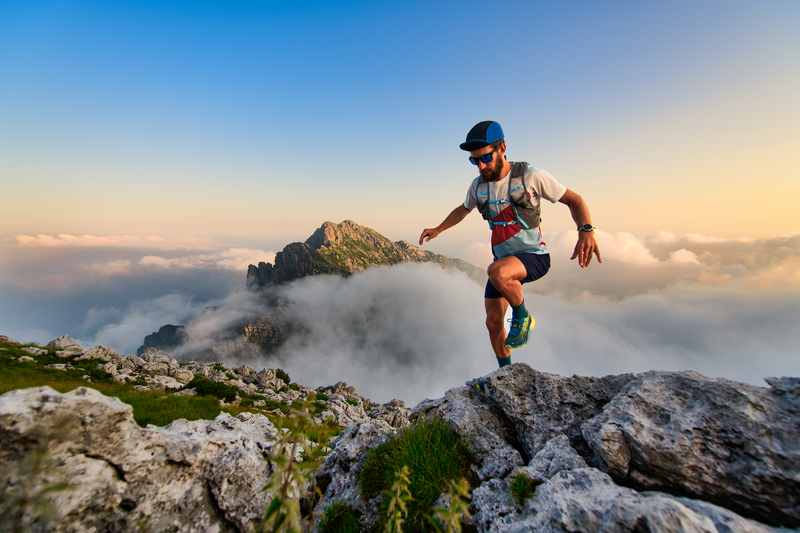
95% of researchers rate our articles as excellent or good
Learn more about the work of our research integrity team to safeguard the quality of each article we publish.
Find out more
PERSPECTIVE article
Front. Physiol. , 25 May 2022
Sec. Striated Muscle Physiology
Volume 13 - 2022 | https://doi.org/10.3389/fphys.2022.886149
Human postmortem skeletal muscles are a unique source of satellite cells for skeletal muscle regenerative studies. Presomite and somite satellite cells obtained by postmortem muscles have been established as populations of human skeletal muscle precursor cells able to proliferate and differentiate in vitro. It is extremely interesting to have access to a large amount of postmortem human skeletal muscle precursor cells, especially from craniofacial as well as limb skeletal muscles in order to evaluate their potential application not only for the fundamental understanding of muscle physiology and diseases but also for drug testing in a challenging 3D-shaping muscles like skeletal muscle microphysiological systems.
The skeletal muscle regeneration is a fundamental biological aspect based on activation of adult stem cells and satellite cells (SCs) that accompany humans throughout their entire life. Indeed, SCs have been found alive several days post mortem, and these cells are able to generate a progeny through few steps in vivo (Latil et al., 2012; Scott et al., 2013). However, scarce data exist on postmortem SCs and any of their functional features. Not to mention the craniofacial muscle stem cell biology, which only recently has started to be gathered (Cheng et al., 2021). Indeed, most data of these muscles remain at the level of tissue description, few indications about their SCs, and no data exist at the postmortem level for presomite SC features. Briefly, it is worth remembering that the skeletal muscles originate from two distinct embryonic districts: craniofacial muscles like thyrohyoid derived from pharyngeal arches. The striated muscle of each arch (sometimes termed branchiomeric) is derived from the rostral continuation of the paraxial mesoderm. The paraxial mesoderm of the head rostral to the occipital region is unsegmented. The somitomeres, which are spherical clusters of mesenchymal cells in the presomitic mesoderm, presage the segmentation of somites in the paraxial mesoderm. The trunk/limb muscles derive from the lateral plate mesoderm. In this perspective, the human postmortem SC functions, both from trunk and craniofacial derivations, deserve attention and need to be explored to understand their regenerative potential and possible applications (Feige et al., 2018).
We aimed to investigate some features of postmortem in vitro SCs, namely human muscle precursor cells (hMPCs), both obtained from somite and pharyngeal arch muscles, specifically the retainment of alive postmortem hMPCs and their ability to proliferate and differentiate along with their intracellular calcium signaling, which was never investigated before, to the best of our knowledge.
The presomite muscles (from pharyngeal arches) thyrohyoid muscles was obtained from the corpses of 40, 43, 45, and 71 years old, and the somite muscles considered were ileopsoas obtained from the same corpses. Informed consents were signed by the deceased subjects’ family members according to the Ethic Committee approval (COET n 6065-04.03.2021).
Histopathological examination of tissue samples obtained during autopsies showed no signs of pathologies that could invalidate the value of further investigations. The muscle has been sampled and immediately immersed in sterile solution containing HAM’s F10 and gentamicin. Each sampling involved the removal of tissue fragments for each muscle through a small accessory cutaneous cut. The sampling on the craniofacial muscle was carried out on the lateral margin, 2 cm from the clavicular insertion; the removal of the vastus lateral muscle will instead be carried out on the lateral margin 2 cm from its origin at the level of the greater trochanter. The weight of the muscle was about 1 g each.
Two different protocols were used to collect the muscle samples: in the first protocol, the muscle sample was put into a physiological medium containing HAM’s F10 and gentamicin and stored for 24 h at 4°C. The medium was renewed three times in order to wash the blood residues, and then the muscle sample was treated for explant formation. In the second protocol, the muscle sample was stored in liquid nitrogen in fetal bovine serum (FBS, Euroclone) + 10% of dimethyl sulfoxide (DMSO, Sigma-Aldrich). Frozen dissected muscle biopsies were thawed at 37°C and washed with PBS before the treatment for explant formation.
Satellite cells were isolated from muscle tissues using the explant procedure as previously described (Pietrangelo et al., 2011 and Pietrangelo et al., 2015). Briefly, small pieces of muscles, named explants, obtained by mincing using sterilized scissors, were put on Petri dishes with a drop of FBS and stored in an incubator at 37°C, 5% CO2, and saturated humidity. During the following 2 weeks, the SCs migrated out of the explants and started to proliferate. To be coherent with the literature, we name these cells hMPCs. After detaching with trypsin-EDTA, the cells were counted, and the population doubling level was calculated at each passage with the following equation: log10(N/n)/ln2 with N as the number of cells at the time of the passage and n as the number of cells initially plated. At the first passage, the cell population was considered at 1 population doubling level (PDL). The proliferative state was maintained by feeding the hMPCs with a growth medium (GM) containing (% vol/vol): HAM’s F10 (Euroclone), 0.1 gentamycin and 1 penicillin/streptomycin 100X (Euroclone), 20 FBS heat-inactivated (56°C, 36 min) (Hyclone), and 1 l-Glutamax 100 × (Gibco). The percentages of myogenic cells were obtained using an immunocytochemistry assay for the marker desmin and with biotinylated streptavidin-AP kits (LSAB + System-AP Universal kits; Cat. No. K0678; DAKO, DakoCytomation, Glostrup, Denmark). Cell cultures with desmin positivity of less than 70% were sorted for surface myogenic markers using CD56 (mouse monoclonal antibody (Abcam, Cambridge, UK)) by flow cytometric analysis (Di Filippo et al., 2016) in order to achieve high myogenic populations.
The differentiation was induced by feeding the hMPCs with a differentiation medium (DM) containing (% vol/vol): DMEM high glucose, 0.1 gentamycin, five heat-inactivated HS (56°C, 36 min), 10 μg/ml insulin, 100 μg/ml apo-tranferrin (Sigma), 1 sodium pyruvate 100 mM, 1 penicillin/streptomycin 100 ×, and 1 l- glutamine 100 × for 7 and 12 days. The myotubes were positive for both the primary antibody against desmin and myosin heavy chain using the MF20 anti-MHC monoclonal antibody (diluted 1:50; Developmental Studies Hybridoma Bank, University of Iowa, Iowa City, IA, United States).
The proliferating hMPCs in the range of 2–3 PDL and differentiated myotubes (7 and 12 days differentiation) were loaded with Fura2-AM (final concentration, 5 μM) for 30 min, washed by removal solution, and incubated for further 30 min at 37°C prior to the intracellular calcium concentration at the cytosolic level ([Ca2+]i) measurement, to allow intracellular Fura2-AM de-esterification. The experiments were performed at the room temperature of 21°C and sea-level O2 and CO2 partial pressure. Images were acquired using the procedures and set-up described by Pietrangelo et al. (2015).
The analyses, mean and standard deviation, and unpaired t-tests were performed using GraphPad Prism Software, version 5 (GraphPad Software, La Jolla, United States).
We isolated postmortem hMPCs from thyrohyoid and ileopsoas. Their desmin positivity was tested, and if it was less than 70%, the cells were sorted for CD56 marker. The cell yield was 5 × 103 ± 700 per mg of presomite muscles and for somite muscles, was 5 × 103 ± 970 cells. Similar results were obtained for the postmortem hMPCs from samples derived by the two procedures described before in Materials and Methods, specifically samples stored at 4°C for 24 h and samples frozen for 2 weeks in liquid nitrogen.
Previously, we have demonstrated that the hMPCs obtained from alive donors, migrated out of the explants within 1 month and reached the proliferative senescence (cells do not duplicate anymore) in about 3–4 months (Pietrangelo et al., 2009). Postmortem hMPCs, both from presomite and somite muscles, migrated out of the explant within 15 days, faster than those migrating out of the explants from muscles of living volunteers. Postmortem hMPCs showed an increased proliferation rate (Figure 1A, postmortem hMPCs in green and orange curves) compared with hMPCs derived from alive donors (Figure 1A black curves). Moreover, postmortem thyrohyoid hMPCs (Figure 1B) proliferate faster than postmortem ileopsoas hMPCs (green vs. orange curve in Figure 1).
FIGURE 1. Results on undifferentiated thyrohyoid and ileopsoas hMPCs. (A) Population doubling level (PDL) of postmortem hMPCs derived from thyrohyoid muscle obtained from 40– (orange squared symbol) and from iliopsoas muscle obtained from 71– (green triangle symbols) year-old corpses while black symbols represent the PDL of five different hMPC populations derived from vastus lateralis of alive donors in the range of 40–71 years old. (B) Picture of thyrohyoid hMPCs in Petri dishes. (C) The graph shows mean and standard deviation of [Ca2+]i measurement on undifferentiated thyrohyoid (UNDIF THY) and ileopsoas (UNDIF IL) hMPCs. They significantly differ with p ≤ 0.05. (D) Representative [Ca2+]i oscillation recorded on thyrohyoid hMPCs. The bar in panel B represents 100 μm.
We measured the [Ca2+]i in postmortem hMPCs as proliferating undifferentiated cells under resting conditions. The [Ca2+]i in thyrohyoid hMPCs was significantly less than [Ca2+]i in ileopsoas hMPCs (Figure 1C). Moreover, thyrohyoid hMPCs showed peculiar spontaneous intracellular [Ca2+] waves. The [Ca2+]i regularly oscillates with a peak followed by a resting level for a period of few minutes. The [Ca2+]i oscillation was recorded for 50 ± 15 min. These oscillatory pathways showed different frequencies. Figure 1D shows a representative trace with a frequency of about 30 [Ca2+]i peaks per hour; we also recorded slower frequencies of about 13 and 6 [Ca2+]i peaks per hour. We never recorded oscillatory [Ca2+]i in ileopsoas postmortem hMPCs.
We analyzed the differentiation process in both somite and presomite hMPCs along with their [Ca2+]i. Figure 2B shows multinucleated myotubes of thyrohyoid hMPCs stained for desmin, while panel C stained for myosin heavy chain protein expression. It can be observed that not all the hMPCs were fused into myotubes at 7 days despite being myogenic cells (panel C). Prolonging the differentiation at 10–12 days, about 90% of hMPCs formed myotubes. We measured the [Ca2+]i in myotubes (panel D) under resting conditions (Figure 2A) and found similar [Ca2+]i in presomite and somite myotubes. We stimulated the myotubes with 500 μM nicotine in order to reveal the presence and the opening of achetylcholine channels. The somite-differentiated hMPCs showed responsiveness to nicotine at 7 days while presomite myotubes showed later at 10–12 days of differentiation.
FIGURE 2. Results of differentiated postmortem hMPCs. (A) [Ca2+]i recorded as the basal level in differentiated hMPCs derived from both thyrohyoid (DIF TY) and ileopsoas (DIF IL) muscles. (B,C) Representative images of immunostaining for desmin (B) and myosin heavy chain proteins (C) on myotubes derived from postmortem thyrohyoid hMPCs. The bars represent 100 μm.
To our current understanding, no one has previously established that postmortem presomite muscles were able to release hMPCs in vitro to be cultured and differentiated. Our results demonstrated that postmortem thyrohyoid hMPCs proliferate with features similar to somite ones, and both lineages are able to come out of the explants earlier and proliferate faster than those from biopsies obtained from alive donors. Postmortem thyrohyoid hMPCs showed peculiar spontaneous [Ca2+] waves lasting tens of minutes. It is worth mentioning that embryological pharyngeal arches originate in not only neck muscles but also cardiomyocytes, which have been demonstrated to be able to give the oscillatory pattern of resting cytosolic calcium (Eisner et al., 2017; Cheng et al., 2021). This creates proactive behavior of the presomite hMPCs, a sort of pacemaking prone to contraction. Considering the large amount of calcium mobilization during oscillatory waves (peak more than 1 μM), we thought that this pattern could be a death signal, but it is worth mentioning that any significant apoptotic cell increase has been recorded on thyroyoid hMPCs.
The [Ca2+]i under resting conditions was significantly less in presomite hMPCs with respect to somite and differentiated myotubes. Following these results, the human postmortem hMPCs functions, both from somite and craniofacial muscles, need to be further explored to deeply understand their regenerative potential and possible applications.
Distinct lineages of SCs are responsible for both head and trunk/limb muscle tissue formation with specific genetic differences in fiber development (Harel et al., 2009; Tzahor, 2009). It has been demonstrated that mutant mice lines that show no development of trunk and limb muscles are still able to form embryonic and fetal muscles of the head (Rodriguez-Outeiriño et al., 2021; Rudnicki et al., 1993).
Scott et al. (2013) well described the isolation of human skeletal muscle satellite cells from postmortem somitic muscles and proposed their utility. Recently, Feige et al. (2021) established a “novel model” to evaluate the human satellite cell fate using postmortem intact human muscle myofibers with muscle stem cells within the niche microenvironment but only on the somite human psoas.
However, to our knowledge, the data we reported in this study are the first demonstration that pre-somitic SCs can be achieved from postmortem presomite muscles, established in culture, and used for studies on muscle regeneration despite the fact that craniofacial muscle regeneration has physiological peculiar properties and promising characteristics observed during aging or muscle disease. Interestingly, eye extrinsic muscles do not show signs of sarcopenia and are less affected by muscular dystrophies (McLoon et al., 2007; La Rovere et al., 2014; Cheng et al., 2021). The amount of SCs we obtained by thyrohyoid is also impressive: if we consider that 1 mg of muscle furnished about 5 × 103 cells at the first PDL, we had 1 g of muscle. This means achieving more than 100 × 106 at the first passage in vitro during culture. Considering that one of the main limitations in microphysiological systems like building muscle organoids is the requirement of large number of adult stem cells (Low et al., 2021); we suggest overcoming it by taking advantage of large-scale and homogeneous postmortem hMPC populations. Human biopsy sampling on living volunteers presents some limitations as very small muscle sampling was performed to avoid the scar tissue formation/muscle function impairment, very rare or rather impossibility to sampling presomite muscle. Postmortem presomite and somite sampling basically overcomes or postpones these limitations. Indeed, the coroner has cut a large amount of muscle for investigations, and further it is worth mentioning that our preliminary data suggest that postmortem hMPCs lifespan significantly increases, even with a certain variability, with respect to the lifespan of hMPCs populations obtained by living donors. We did not test the extent of lifespan yet, but it seems present in each hMPC population we obtained. It will be very interesting to investigate if postmortem thyrohyoid hMPCs change their Hayflick limit for duplicative senescence with respect to somite ones.
Using postmortem SCs, we can produce not only a large number of cells useful for organ-on-chip and organoid differentiation protocol but also allow having a structure mimicking nerve-dependent skeletal muscle contraction. Muscle fiber contractions are visible in about 20 days and can be maintained over a long period, thanks to the production of innervated multinucleated mature skeletal muscle fibers (Mazaleyrat et al., 2020).
Since the discovery in humans of somite cell reprogramming into pluripotent stem cells (hiPSCs), several protocols of cell lineage proliferation and differentiation have been created aimed at modeling physiological programs, starting the modern stem cell-based regenerative medicine (Ortiz-Vitali and Darabi, 2019). Matsuda et al. (2020) used iPSCs for studying the stepwise origin that recapitulates more complex features of human mesoderm development and in vitro induction of presomite mesoderm on human somitogenesis, with the interesting demonstration of good achievement on spondylocostal dysostosis. However, the skeletal muscle differentiation studies based on iPSCs have lagged behind those of other cell lineages. The protocols for generating mature presomite muscle fibers with sarcolemmal organization using iPSCs remain unexplored, and the investigation of the complexity of mature skeletal muscle is still lacking. Not to mention modeling and investigating specific interesting features of presomite skeletal muscles under dystrophic conditions, in which presomite muscles survive longer than other muscles also under very severe conditions like Duchenne muscular dystrophies.
Overall, these efforts pave the way to demonstrate the great potential of presomite iPSCs for disease modeling considering several pathologies, as well as helping identify new pathologic mechanisms involved (Dutta et al., 2017). Considering regenerative medicine, several other conditions can take advantage of the studies on presomite 2D and 3D organoids, as those for the identification of physiological or pathological mechanisms underlying the regeneration process as well as drug interference (Ostrovidov et al., 2019).
Amazing possibilities may emerge from the advancement of our perspective. First, the definition of protocols for muscle stem cells-derived organ-on-chip and organoids;. second, the subsequent disease modeling and no-patient clinical trials (Mummery et al., 2014); and third, extending the field of regenerative medicine models for organ transplants (Hoogduijn et al., 2020) with postmortem models.
In perspective, this work opens a new field of investigation on postmortem satellite cells from presomite and somite skeletal muscle finalized to specific biobanks to be used for organoid formation.
In conclusion, our perspective work is in line with the recent advances in regenerative and precision medicine and the need of understanding the fundamental mechanisms in presomite and somite SC models and their role in microphysiological systems for skeletal muscle studies (Jalal et al., 2021). Moreover, our ability to collect and manage postmortem presomite as well as somite hMPCs can significantly contribute to creating a new biobank shareable with researchers involved in physiological and pathological studies on skeletal muscle.
The raw data supporting the conclusion of this article will be made available by the authors, without undue reservation.
The studies involving human participants were reviewed and approved by the Comitato etico delle province di Chieti e Pescara.
TP, VV, CD’O, RD, and ED’A conceived the experimental plan. CD’O, RD, and PR performed autopsies. TP, CS, NP, ER, MB, ML, AT, DB, LM, and SF optimized and performed experiments. TP, CD’O, RD, and ED’A wrote the manuscript. All the authors corrected and approved the published manuscript.
The authors were supported by the local funds from their universities.
The authors declare that the research was conducted in the absence of any commercial or financial relationships that could be construed as a potential conflict of interest.
All claims expressed in this article are solely those of the authors and do not necessarily represent those of their affiliated organizations, or those of the publisher, the editors, and the reviewers. Any product that may be evaluated in this article, or claim that may be made by its manufacturer, is not guaranteed or endorsed by the publisher.
The authors want to acknowledge the familiar for their consent to muscle sampling. The authors also thank Laura Magliulo for her help at the beginning of the study.
Cheng X., Shi B., Li J. (2021). Distinct Embryonic Origin and Injury Response of Resident Stem Cells in Craniofacial Muscles. Front. Physiol. 12, 690248. doi:10.3389/fphys.2021.690248
Di Filippo E. S., Mancinelli R., Pietrangelo T., La Rovere R. M., Quattrocelli M., Sampaolesi M., et al. (2016). Myomir Dysregulation and Reactive Oxygen Species in Aged Human Satellite Cells. Biochem. Biophys. Res. Commun. 473 (2), 462–470. doi:10.1016/j.bbrc.2016.03.030
Dutta D., Heo I., Clevers H. (2017). Disease Modeling in Stem Cell-Derived 3D Organoid Systems. Trends Mol. Med. 23, 393–410. doi:10.1016/j.molmed.2017.02.007
Eisner D. A., Caldwell J. L., Kistamás K., Trafford A. W. (2017). Calcium and Excitation-Contraction Coupling in the Heart. Circ. Res. 121 (2), 181–195. doi:10.1161/CIRCRESAHA.117.310230
Feige P., Brun C. E., Ritso M., Rudnicki M. A. (2018). Orienting Muscle Stem Cells for Regeneration in Homeostasis, Aging, and Disease. Cell Stem Cell 23, 653–664. doi:10.1016/j.stem.2018.10.006
Feige P., Tsai E. C., Rudnicki M. A. (2021). Analysis of Human Satellite Cell Dynamics on Cultured Adult Skeletal Muscle Myofibers. Skelet. Muscle 11, 1. doi:10.1186/s13395-020-00256-z
Harel I., Nathan E., Tirosh-Finkel L., Zigdon H., Guimarães-Camboa N., Evans S. M., et al. (2009). Distinct Origins and Genetic Programs of Head Muscle Satellite Cells. Dev. Cell 16, 822–832. doi:10.1016/j.devcel.2009.05.007
Hoogduijn M. J., Montserrat N., Laan L. J. W., Dazzi F., Perico N., Kastrup J., et al. (2020). The Emergence of Regenerative Medicine in Organ Transplantation: 1st European Cell Therapy and Organ Regeneration Section Meeting. Transpl. Int. 33, 833–840. doi:10.1111/tri.13608
Jalal S., Dastidar S., Tedesco F. S. (2021). Advanced Models of Human Skeletal Muscle Differentiation, Development and Disease: Three-Dimensional Cultures, Organoids and beyond. Curr. Opin. Cell Biol. 73, 92–104. doi:10.1016/j.ceb.2021.06.004
La Rovere R. M. L., Quattrocelli M., Pietrangelo T., di Filippo E. S., Maccatrozzo L., Cassano M., et al. (2014). Myogenic Potential of Canine Craniofacial Satellite Cells. Front. Aging Neurosci. 6, 90. doi:10.3389/fnagi.2014.00090
Latil M., Rocheteau P., Châtre L., Sanulli S., Mémet S., Ricchetti M., et al. (2012). Skeletal Muscle Stem Cells Adopt a Dormant Cell State Post Mortem and Retain Regenerative Capacity. Nat. Commun. 3, 903. doi:10.1038/ncomms1890
Low L. A., Mummery C., Berridge B. R., Austin C. P., Tagle D. A. (2021). Organs-on-chips: into the Next Decade. Nat. Rev. Drug Discov. 20, 345–361. doi:10.1038/s41573-020-0079-3
Matsuda M., Yamanaka Y., Uemura M., Osawa M., Saito M. K., Nagahashi A., et al. (2020). Recapitulating the Human Segmentation Clock with Pluripotent Stem Cells. Nature 580, 124–129. doi:10.1038/s41586-020-2144-9
Mazaleyrat K., Badja C., Broucqsault N., Chevalier R., Laberthonnière C., Dion C., et al. (2020). Multilineage Differentiation for Formation of Innervated Skeletal Muscle Fibers from Healthy and Diseased Human Pluripotent Stem Cells. Cells 9, 1531. doi:10.3390/cells9061531
McLoon L., Thorstenson K., Solomon A., Lewis M. (2007). Myogenic Precursor Cells in Craniofacial Muscles. Oral Dis. 13, 134–140. doi:10.1111/j.1601-0825.2006.01353.x
C. Mummery, A. van de Stolpe, B. A. J. Roelen, and H. Clevers (Editors) (2014). “Chapter 13 - Human Stem Cells for Organs-On-Chips: Clinical Trials without Patients?,” in Stem Cells. 11Part of this chapter was adapted from an Organs-on-Chips meeting report, Lab on a Chip 2013, 10.1039/c3lc50248a. Second Edn (Boston: Academic Press), 343–361. doi:10.1016/B978-0-12-411551-4.00013-1.
Ortiz-Vitali J. L., Darabi R. (2019). iPSCs as a Platform for Disease Modeling, Drug Screening, and Personalized Therapy in Muscular Dystrophies. Cells 8, 20. doi:10.3390/cells8010020
Ostrovidov S., Salehi S., Costantini M., Suthiwanich K., Ebrahimi M., Sadeghian R. B., et al. (2019). 3D Bioprinting in Skeletal Muscle Tissue Engineering. Small 15, 1805530. doi:10.1002/smll.201805530
Pietrangelo T., Puglielli C., Mancinelli R., Beccafico S., Fanò G., Fulle S. (2009). Molecular Basis of the Myogenic Profile of Aged Human Skeletal Muscle Satellite Cells During Differentiation. Exp. Gerontol. 44, 523–531. doi:10.1016/j.exger.2009.05.002
Pietrangelo T., D’Amelio L., Doria C., Mancinelli R., Fulle S., Fanò G. (2011). Tiny Percutaneous Needle Biopsy: an Efficient Method for Studying Cellular and Molecular Aspects of Skeletal Muscle in Humans. Int. J. Mol. Med. 27, 361–367. doi:10.3892/ijmm.2010.582
Pietrangelo T., Di Filippo E. S., Mancinelli R., Doria C., Rotini A., Fanò-Illic G., et al. (2015). Low Intensity Exercise Training Improves Skeletal Muscle Regeneration Potential. Front. Physiol. 6 (dec), 399. doi:10.3389/fphys.2015.00399
Rodriguez-Outeiriño L., Hernandez-Torres F., Ramírez-de Acuña F., Matías-Valiente L., Sanchez-Fernandez C., Franco D., et al. (2021). Muscle Satellite Cell Heterogeneity: Does Embryonic Origin Matter? Front. Cell Dev. Biol. doi:10.3389/fcell.2021.750534
Rudnicki M. A., Schnegelsberg P. N. J., Stead R. H., Braun T., Arnold H.-H., Jaenischs R. (1993). MyoD or Myf-5 Is Required for the Formation of Skeletal Muscle. Cell 75, 1351. doi:10.1016/0092-8674(93)90621-v
Scott I. C., Tomlinson W., Walding A., Isherwood B., Dougall I. G. (2013). Large-scale Isolation of Human Skeletal Muscle Satellite Cells from Post-mortem Tissue and Development of Quantitative Assays to Evaluate Modulators of Myogenesis. J. Cachexia Sarcopenia Muscle 4, 157–169. doi:10.1007/s13539-012-0097-z
Keywords: satellite cells, skeletal muscle regeneration, postmortem, presomitic muscles, somitic muscles, embryonic origin, organoids
Citation: Pietrangelo T, Demontis R, Santangelo C, Pini N, Bonelli M, Rosato E, Roberti P, Locatelli M, Tartaglia A, Marramiero L, Verratti V, Bondi D, Fulle S, D’Aloja E and D’Ovidio C (2022) New Perspectives for Postmortem Human Satellite Cells of Different Embryological Origin. Front. Physiol. 13:886149. doi: 10.3389/fphys.2022.886149
Received: 28 February 2022; Accepted: 22 April 2022;
Published: 25 May 2022.
Edited by:
Cesare Gargioli, University of Rome Tor Vergata, ItalyReviewed by:
Alessio Reggio, University of Rome Tor Vergata, ItalyCopyright © 2022 Pietrangelo, Demontis, Santangelo, Pini, Bonelli, Rosato, Roberti, Locatelli, Tartaglia, Marramiero, Verratti, Bondi, Fulle, D’Aloja and D’Ovidio. This is an open-access article distributed under the terms of the Creative Commons Attribution License (CC BY). The use, distribution or reproduction in other forums is permitted, provided the original author(s) and the copyright owner(s) are credited and that the original publication in this journal is cited, in accordance with accepted academic practice. No use, distribution or reproduction is permitted which does not comply with these terms.
*Correspondence: Tiziana Pietrangelo, dGl6aWFuYS5waWV0cmFuZ2Vsb0B1bmljaC5pdA==
Disclaimer: All claims expressed in this article are solely those of the authors and do not necessarily represent those of their affiliated organizations, or those of the publisher, the editors and the reviewers. Any product that may be evaluated in this article or claim that may be made by its manufacturer is not guaranteed or endorsed by the publisher.
Research integrity at Frontiers
Learn more about the work of our research integrity team to safeguard the quality of each article we publish.