- Departamento de Genética Molecular, Instituto de Fisiología Celular, Universidad Nacional Autónoma de México, Ciudad Universitaria, Mexico City, Mexico
From 2.5 to 2.0 billion years ago, atmospheric oxygen concentration [O2] rose thousands of times, leading to the first mass extinction. Reactive Oxygen Species (ROS) produced by the non-catalyzed partial reduction of O2 were highly toxic eliminating many species. Survivors developed different strategies to cope with ROS toxicity. At the same time, using O2 as the final acceptor in respiratory chains increased ATP production manifold. Thus, both O2 and ROS were strong drivers of evolution, as species optimized aerobic metabolism while developing ROS-neutralizing mechanisms. The first line of defense is preventing ROS overproduction and two mechanisms were developed in parallel: 1) Physiological uncoupling systems (PUS), which increase the rate of electron fluxes in respiratory systems. 2) Avoidance of excess [O2]. However, it seems that as avoidance efficiency improved, PUSs became less efficient. PUS includes branched respiratory chains and proton sinks, which may be proton specific, the mitochondrial uncoupling proteins (UCPs) or unspecific, the mitochondrial permeability transition pore (PTP). High [O2] avoidance also involved different strategies: 1) Cell association, as in biofilms or in multi-cellularity allowed gas-permeable organisms (oxyconformers) from bacterial to arthropods to exclude O2. 2) Motility, to migrate from hypoxic niches. 3) Oxyregulator organisms: as early as in fish, and O2-impermeable epithelium excluded all gases and only exact amounts entered through specialized respiratory systems. Here we follow the parallel evolution of PUS and O2-avoidance, PUS became less critical and lost efficiency. In regard, to proton sinks, there is fewer evidence on their evolution, although UCPs have indeed drifted in function while in some species it is not clear whether PTPs exist.
Introduction
During the great oxygenation event (GOE), 2.5 to 2 billion years ago, oxygen concentration ([O2]) increased thousands of times, leading to the first massive extinction (Lane, 2002; Reinhard et al., 2016). Except for those living in anaerobic niches, surviving organisms had to adapt and thus aerobic metabolism developed: organisms harness the highly exergonic O2-reduction, increasing ATP yields up to 16 times. However, the toxicity of the highly motile free radicals of O2, the ROS had to be dealt with, and many strategies were developed (Weiss et al., 2016). Detoxifying enzymes such as superoxide dismutase, catalase and the glutathione peroxidase system eliminate ROS (Cortassa et al., 2014). These iron-containing antioxidant enzymes are present in strict anaerobes, possibly inherited from their anaerobic prokaryotic ancestors (Ślesak et al., 2016).
Another mechanism against oxygen toxicity is to prevent the non-catalyzed partial reduction of O2 through physiological uncoupling systems (PUS), which increases the rate of electrons in flow in respiratory chains (RC) lowering both cellular [O2] and free radical concentrations (Kadenbach, 2003; Guerrero-Castillo et al., 2011; Uribe-Carvajal et al., 2011; Guerrero-Castillo et al., 2012; Cabrera-Orefice et al., 2015). PUS may be intrinsic, as branched RCs or extrinsic, and as in proton sinks. Branched RCs are those that contain non-proton pumping redox enzymes, while mitochondrial proton sinks include uncoupling proteins (UCPs) and permeability transition pores (PTP) (Kadenbach, 2003; Demine et al., 2019; Zhao et al., 2019).
Initially, for many species anaerobic niches must have been the only option for survival (Müller et al., 2012). Total avoidance of O2 is observed in anaerobic organisms such as those living in animal digestive tubes (Fenchel and Finlay, 1994). However, when accidentally exposed to high [O2], anaerobic organisms may differentially express branched RCs (Rosas-Lemus et al., 2016; Jayawardhane et al., 2020). Another avoidance strategy is association into biofilms, where surface cells limit O2 diffusion, creating an anaerobic internal microenvironment (Stewart, 2003). Perhaps these structures became stable, giving birth to multicellular organisms that move, migrating to areas where [O2] is lower (Abele et al., 2007). Among pluricellular organisms, fungi and arthropods are oxyconformers, i.e., their control of O2 diffusion is partial (Martínez-Cruz et al., 2012). The most sophisticated O2 avoidance system first appeared in fish and evolved in amphibians, reptiles, birds, and mammals, which developed a gas-impermeable epithelium plus a specialized external respiratory system (gills or lungs) (Stamati et al., 2011). These are the oxyregulator organisms where [O2] reaching internal cells is 20–31 μM or four to five hundred times less that atmospheric [O2] which is 1,026 μM at sea level (Figure 1) (Rosas-Lemus et al., 2016). Interestingly, amphibians already have lungs, even when their skin is still permeable to gases and participates in O2/CO2 exchange (skin breathing) (Tattersall, 2007).
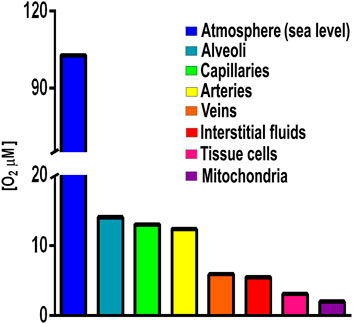
FIGURE 1. Oxygen concentrations in different compartments in an oxyregulator organism. Atmospheric [O2] 1,028 μM, lung alveoli [O2] 143 μM; capillaries [O2] 130 mM; arteries [O2] 123 μM; interstitial fluids [O2] 55 μM; tissue cells [O2] 31 μM; and mitochondria [O2] 20 µM. Values from Rosas-Lemus et al., 2016 and references therein.
During evolution from prokaryotes to unicellular eukaryotes to pluricellular organisms, the ability to exclude oxygen improved while in contrast, respiratory chain branching was progressively lost (Hsia et al., 2013). Increasing O2-avoidance efficiency rendered alternative redox enzymes superfluous and thus these were lost (McDonald et al., 2009). However, O2 overexposure accidents are more dangerous when PUS become less efficient (Rosas-Lemus et al., 2016).
The amount of O2 consumed by mitochondria is determined by energy demand rather than availability; at high [ATP], O2 pressure is higher than 0.5 mm Hg+, while addition of an uncoupler (CCCP or FCCP) increases the respiratory rate 6–10 times while lowering pO2 to less than 0.03 mm Hg+ (Gnaiger et al., 1995). When cells are treated with an uncoupler, the decrease in pO2 is significantly smaller than in isolated mitochondria, suggesting that there are intracellular diffusion barriers in the cytoplasm (Wilson, 1990). The diffusion barriers are probably more difficult to estimate in whole tissues and organisms (Slggaard-Andersen et al., 1995). In mammals, the mitochondrial pO2 at state 3 only slightly lower than at state 4, while adding an uncoupler drives pO2 levels to dangerously low levels (Rumsey et al., 1990; Guidot et al., 1995; Steinlechner-Maran et al., 1996). This illustrates the intricate interaction of diffusion gradients, metabolic states, and coupling/uncoupling states on respiratory metabolism in oxyregulator organisms. In contrast, Saccharomyces cerevisiae cells collected during log phase growth in lactate it exhibits a superoxide scavenging activity that directly correlates with oxygen tension (Gnaiger et al., 1995). Thus, mitochondria from oxyregulators are probably more sensitive to hypoxia and reoxygenation than shrimp or yeast; let alone bacteria (Martínez-Cruz et al., 2017).
Here, we analyze the parallel evolution of PUS and avoidance strategies. Special attention is given to the branched structure in RCs. It is observed that as species developed better avoidance mechanisms, branching of their respiratory chains decreased, probably due to an effort to become more efficient to produce ATP and to the decreased need to control ROS overproduction in an O2-controlled environment. Whether this decrease in resistance to sudden oxygenation applies to proton sinks is not as well defined. Nonetheless, some considerations are made at the end of the chapter.
Prokaryotes
When GOE arrived, most organisms were prokaryotes and the first eukaryotes were only beginning (Shikama and Matsuoka, 2004). All anaerobic species had to either avoid the toxic high [O2] environment or develop the ability to deplete O2 adapting their respiratory chains (RC) (Pahl and Baeuerle, 1994). RC constituents are at least substrate dehydrogenases that transferring electrons to a quinone pool and terminal reductases receiving electrons from quinones. Substrate-specific dehydrogenases transfer reducing equivalents from various donor substrates (NADH, succinate, glycerol phosphate, formate, hydrogen, pyruvate, and lactate) to a quinone pool (menaquinone, ubiquinone or dimethylmenaquinone) (Unden and Bongaerts, 1997). Then terminal reductases transfer electrons from quinol to a final electron acceptor (Brunori et al., 2005). In aerobic metabolism the terminal electron acceptor is O2 and thus reductases are termed oxidases (Cotter et al., 1990). As an additional element, some bacteria use quinol to reduce cytochrome c (Borisov and Verkhovsky, 2015).
Facultative bacteria express a highly adaptable RC allowing them to live in different environments, e.g., Escherichia coli lives in highly aerobic to anaerobic environments through the differential expression of different RC redox enzymes (Borisov and Verkhovsky, 2015). Differential expression of redox enzymes seems to protect the cell against stress, e.g. cytochrome bd-I has been reported to protect E. coli against H2O2 toxicity (Borisov et al., 2021). Staphylococcus epidermidis is a facultative anaerobe living in a broad range of [O2], including human skin, where [O2] varies from 2 to 5% (Peyssonnaux et al., 2008), ischemic/anoxic tumors, and abscesses, where [O2] is zero (Atkuri et al., 2007; Wiese et al., 2012). At different [O2], S. epidermidis RC composition varies widely (Table 1). In high O2, S. epidermidis expresses five oxido-reductases, namely glycerol-3-phosphate dehydrogenase, pyruvate dehydrogenase, ethanol dehydrogenase, and succinate dehydrogenase, as well as the cytochromes bo and aa3. Under these conditions biofilms production is minimal (Uribe-Alvarez et al., 2016). At low [O2], pyruvate dehydrogenase and ethanol dehydrogenase levels drop by 50%, while glycerol-3-phosphate dehydrogenase and succinate dehydrogenase levels vanish; among reductases, cytochrome bo increases, cytochrome aa3 disappears, and nitrate reductase activity is present. Under anaerobic conditions, quinone donors do not vary further, while cytochrome bo decreases, and nitrate reductase is the predominant terminal electron acceptor (Uribe-Alvarez et al., 2016). Again, differential redox enzyme expression seems to protect S. epidermidis against high O2 concentrations. This response is coordinated with biofilm generation (see below) (Fang et al., 2016; Pedroza-Dávila et al., 2020).
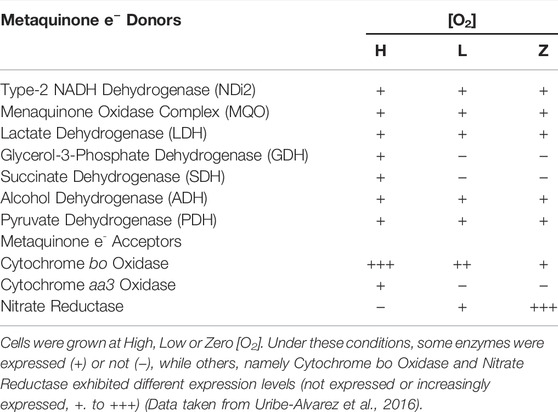
TABLE 1. Enzyme expression at different [O2] (High Low or Zero) in the S. epidermidis branched respiratory chain.
Another interesting facultative bacterium is Bacillus cereus, which during sporulation, dormancy, or germination expresses different RC activities depending on its menaquinone concentration (Escamilla et al., 1988). NADH oxidase activity is inactivated during sporulation stages III to VI, most likely due to a substantial drop in menaquinone. During the same time frame, NADH oxidase in the mother cell progressively decreases to about 50%. Menadione restores NADH-dependent respiration and cytochrome reduction in dormant spore membranes (Escamilla et al., 1988). Mycobacterium tuberculosis is an obligate aerobe with a branched respiratory chain where electrons may travel from the cytochrome bc1 complex to an aa3-type cytochrome c oxidase or may enter directly to a cytochrome bd-type quinol oxidase. Overexpression of cytochrome bd is linked to enhanced peroxide resistance, so this may be a survival strategy against the host immunological response (Lu et al., 2015).
Cytochrome cbb3, together with the bd oxidase, plays a key role in the protection of O2-sensitive nitrogenase in Azorhizobium caulinodans, a bacterium that develops a nitrogen-fixing symbiosis with plants of the genus Sesbania. Bacteria with bd-type oxidases have been found to be resistant to nitric oxide (NO), peroxynitrite, sulfide, ammonia, and cyanide. This is most likely why harmful bacteria have so much cytochrome bd. Because these enzymes are not found in eukaryotes, they are particularly appealing as prospective targets for novel antibacterial drugs (Borisov et al., 2021). One last example is Neisseria gonorrhoeae, which needs nitrite reductase, cytochrome c peroxidase and nitric oxide reductase to survive under stress and thus enhance its pathogenicity (Apicella et al., 2011; Phillips et al., 2012).
Rickettsia prowazekii (McGinn and Lamason, 2021), Wolbachia sp., and Sodalis (Attardo et al., 2020) are obligate endosymbiont bacteria that reside inside the cells of their hosts. D'Autréaux and Toledano (2007) speculate that these organisms invade the cytoplasm to live in a microaerophilic environment with O2 consuming organelles and ROS detoxifying enzymes. It is unclear if obligatory endosymbionts have a respiratory chain that can help host mitochondria deplete intracellular oxygen as even in the stationary phase, may force the host to maintain a high respiratory activity (Uribe-Alvarez et al., 2019).
Unicellular Eukaryotes
Unicellular organisms show high adaptability to a wide range of [O2] in the medium through manipulation of their own anaerobic/aerobic metabolism (Bunn and Poyton, 1996; Johnson, 2019; López-Barneo and Simon, 2020), using detoxifying enzymes (Sies, 1993; Inupakutika et al., 2016; Lyall et al., 2020) or uncoupling proteins (UCPs) (Jarmuszkiewicz et al., 2010; Busiello et al., 2015; Demine et al., 2019; Nicholls, 2021).
The ability to sense and respond to variations in [O2] is essential for survival (Cadenas, 1989). In eukaryotic cells ROS are produced in the reactions catalyzed by NAD(P)H oxidase and by some other specialized oxidases and as a byproduct of many redox reactions (Turpaev, 2002). Oxyconformer organisms (see below) have branched RCs (McDonald et al., 2009). The classical mitochondrial oxidative phosphorylation (OxPhos) components in the mitochondrial inner membrane are four respiratory complexes plus a fifth complex, the ATP synthase (Unden and Bongaerts, 1997). In eukaryotic cells RC branching is not as varied as in prokaryotes. The branches found are type-2 NADH dehydrogenases (NDH2) and alternative oxidases (AOX) (Figure 2) (Saari et al., 2019).
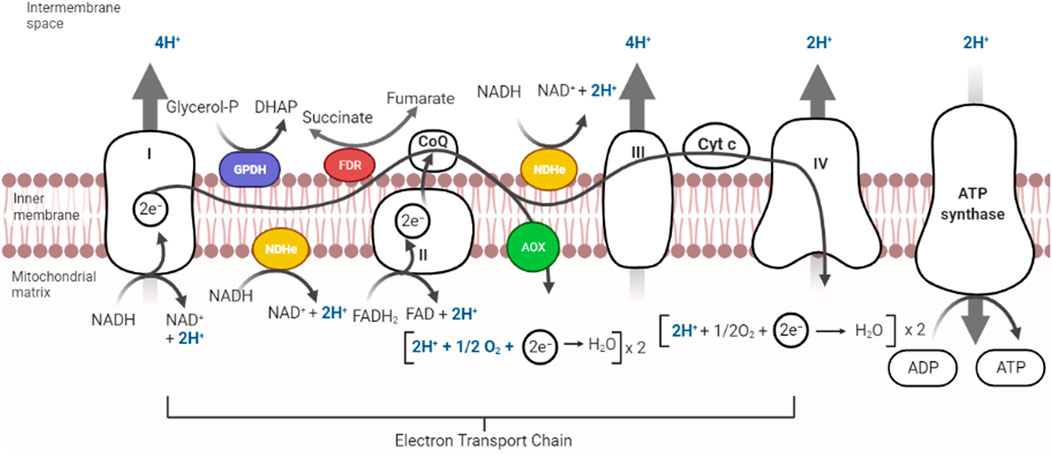
FIGURE 2. Components in mitochondrial respiratory chains. Most mitochondrial RCs are constituted by the classic complexes I, II, III, and IV. In addition, some RCs contain alternative redox enzymes donating electrons to the quinone pool such as alternative NADH dehydrogenase (in yellow), Glycerol-P-dehydrogenase (purple) or fumarate reductase (red). An alternative oxidase (AOX) oxidizes quinol reducing O2. Branched RCs skip proton pumps, decreasing proton pumping stoichiometry. Complex V uses proton gradients to produce ATP.
NDH2 reduces ubiquinone as does Complex I. However, NDH2 does not pump protons, it is not inhibited by rotenone, and it is not a transmembrane protein (Rasmusson et al., 2004; McDonald and Gospodaryov, 2019). NDH2 has been found in a variety of organisms, including plants, fungi, and yeasts. In fungi, these enzymes are found on both sides of the mitochondrial membrane, allowing them to oxidize either cytosolic or mitochondrial NAD(P)H. For example, S. cerevisiae has two external NDH2s (Nde1 and Nde2) plus one internal NDH2 (Ndi1) (Yamashita et al., 2018). In S. cerevisiae and Saccharomyces carlsbergensis Complex I is substituted by three alternative NDH2s (Helmerhorst et al., 2002). NDH2s may be in either side of the mitochondrial inner membrane, oxidizing NADH from the matrix or from the cytoplasm (Rigoulet et al., 2004).
The ultimate physiological uncoupling effect occurs when NADH dehydrogenases act in concert with AOX (Kerscher et al., 2002). AOX reduces O2 substituting the cytochrome pathway (Joseph-Horne et al., 2001). Alternative redox enzymes are induced during stress or in the stationary phase (Medenstsev et al., 1999; Medenstsev et al., 2002). At high [O2], AOX may decrease ROS production. The different O2 affinities of the cytochrome (Km = 0.1 µmol) and alternative (Km = 10–20 µmol) pathways enable COX to sustain OxPhos while AOX lowers [O2] (Gupta et al., 2015). These enzymes may also coexist with the usual complexes as in Yarrowia lipolytica, where an external NDH2 (Nde) and an AOX coexist with Complexes I to IV (Kerscher et al., 1999; Kerscher et al., 2002; Guerrero-Castillo et al., 2009). In Candida parapsilosis and Candida albicans antibiotic resistance has been linked to AOX expression (Guérin and Camougrand, 1986; Milani et al., 2001; Ruy et al., 2006). Additionally, in C. albicans the branched respiratory chain and UCP are important for decreasing ROS and modulating cell proliferation (Jarmuszkiewicz et al., 2000; Ruy et al., 2006).
In Y. lipolytica during the logarithmic growth phase, Nde interacts with complexes III–IV both of which are proton pumps (Guerrero-Castillo et al., 2009). In contrast, during the stationary growth phase, electrons are directly transferred from alternative NDH2 to AOX, thus uncoupling oxidative phosphorylation and decreasing ROS production (Guerrero-Castillo et al., 2012). Debaryomyces hansenii is a halotolerant yeast with a branched respiratory chain constituted by complexes I to IV plus an Nd2e and an AOX. In the stationary phase, a permeability transition pore opens and matrix NADH is depleted, which inactivates Complex I; then the alternative pathway becomes important for O2 depletion and prevention of ROS overproduction (Cabrera-Orefice et al., 2014). In D. hansenni grown in the presence of high osmolyte concentrations, AOX is coupled to complex I, promoting proton pumping even under conditions where the cytochrome pathway is compromised (Figure 2) (Garcia-Neto et al., 2017).
Biofilms
Biofilm formation was developed soon after the beginning of life (Westall et al., 2000). Organisms became capable of anchoring themselves near a feeding source or away from a toxic substance (Davey and O’toole, 2000; Azúa-Bustos et al., 2009; Wessel et al., 2014; Hall and Mah, 2017). Biofilms regulate exposure of individuals in the community to outside factors, where nutrients, oxygen, or molecules have a specific diffusion rate (Costerton et al., 2003; Stewart, 2003; Harrison et al., 2005). Aggregated cells were held together by secreted polysaccharides (Wingender et al., 1999). The carboxyl and phosphoryl groups in these polysaccharides led to biofilm mineralization and eventual fossilization (Westall et al., 2000). Biofilm fossils have provided morphological evidence suggesting that more than one microorganism species lived in these communities (Frances et al., 2001). Biofilms have been preserved over time as one of the most effective mechanisms of protection against many factors, in addition to varying [O2] (Figure 3A). It is currently preserved in most unicellular organisms such as bacteria, archaea, algae, some fungi, and protozoa (De la Fuente-Núñez et al., 2013).
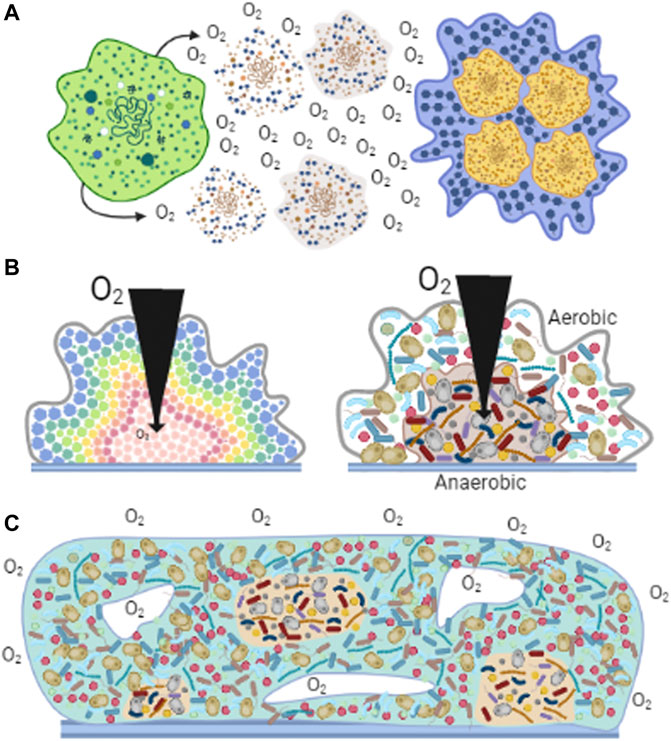
FIGURE 3. Biofilms observed in nature. Cells associate to form biofilms forming different structures. (A) Cells react to high [O2] by excreting polysaccharides that help them aggregate (Wingender et al., 1999). (B) Diversity in biofilms. In homogeneous aggregates (left panel), at different biofilm depths, cells perceive different levels of O2, nutrients and other metabolites and thus adapt their metabolism (López et al., 2010). In heterogeneous aggregates (right panel), aerobic organisms are located on the surface, using O2 before it enters deeper parts of the aggregate, enabling hypo/anaerobic organism to grow (Vilne et al., 2021). (C) Generation of microenvironments. Diverse cell species distribute according to their affinities for nutrients or [O2] (Wessel et al., 2014). The formation of channels (white spaces) allows the dynamic distribution of molecules and providing a path for waste disposal (Frances et al., 2001).
Biofilms provide favorable microenvironments enhancing the development of organisms (Wessel et al., 2014). In biofilms formed by a single species, cell metabolism may vary depending on their location in the agglomerate, resulting in communities with metabolically active and inactive cells, or cells expressing different genes (Figure 3B) (López et al., 2010). i.e., in S. epidermidis changing [O2] affects growth rate, oxygen consumption, ATP synthesis and ROS resistance by expressing a defective respiratory chain and impact in the formation of biofilm (Pedroza-Dávila et al., 2020).
Low [O2] increases the synthesis of biofilm-associated compounds, including the cell adhesion-promoting extracellular polysaccharide -1,6-linked glycosaminoglycan (Cramton et al., 1999). Thus, biofilms would allow cells to grow in hypoxic environments. Still, at high [O2], biofilms formed by Shewanella putrefaciens, are regulated by [O2] (Wu et al., 2013). S. putrefaciens has an oxygen-sensitive diguanylate cyclase that regulates the expression of an BpfA adhesin and allows biofilms to be formed at high [O2] (Wu et al., 2013). In addition to their regulation of BpfA, diguanylate cyclases modulate the abundance of cyclic di-3′,5′-guanylate, a second messenger that regulates many bacterial behaviors, such as motility and biofilm formation (Valentini and Filloux, 2016).
In biofilms formed under anaerobic or hypoxic conditions, such as in C. albicans, biofilm regulators in normoxia such as hyphae promoters and glycosyltransferases do not interfere with biofilm development in anaerobic conditions (Rossignol et al., 2009; Stichternoth and Ernst, 2009), which means that the requirements for biofilm formation in hypoxic environments are different from those present under oxygenated conditions. In the anaerobic hyperthermophiles Thermotoga maritimos, Archaeoglobus fulgidus, and Methanococcus jannaschii, biofilm formation can be induced by high pH or ultraviolet light in addition to high [O2] (Pysz et al., 2004).
Besides the mentioned mutual protection against the environment, biofilms led to greater socialization (information exchange), enhancing survival abilities in participating species (Penesyan et al., 2021). In this regard, it has been proposed that biofilms led to the formation of eukaryotic cells: the “third space hypothesis” suggests some cells in the aggregates eventually stopped working for the common good, becoming predators and then, probably through phagocytosis incorporated future mitochondria (Davey and O’toole, 2000). Another hypothesis suggests that eukaryotic cells evolved through the exchange of “hyperstructures” between multiple types of cells, forming a “metacell”, which over time became integrated, compacted, and simplified to what we now know as eukaryotic cells (Norris and Root-Bernstein, 2009; Bateman, 2020).
Different species may associate in biofilms profiting from [O2] gradients or using secondary metabolite concentration gradients to find a niche within the community (Bradshaw et al., 1996; Penesyan et al., 2021). An interesting case is a biofilm found in the hot water pipes of residential buildings in the city of Riga (Latvia), where a strictly anaerobic microorganism (Thermodesulfovibrio) and a strictly aerobic species (Phenylobacterium) coexisted (Vilne et al., 2021). In this biofilm the aerobic organism was located mostly on the outer surface, while the anaerobic organism was inside the biofilm (Fox et al., 2014). In addition, biofilms can exist within other organisms; a clear example of this is the bovine digestive system where bacteria, protozoa and yeasts are embedded in the large biofilm covering the mucosal layer of the rumen (Harrison et al., 2005).
Biofilms formed by heterogeneous organisms show some similarities with multicellular organisms (Penesyan et al., 2021). The microenvironment generated by diffusion and distribution of compounds promotes different functions for different organisms much as in multicellular organisms (Penesyan et al., 2021). Thus, cells in the circumference of the biofilm deliver nutrients to the deeper zones. In addition, surface cells may control O2 distribution to the inner cells (de Beer et al., 1994). Ducts and channels resembling the circulatory systems in multicellular organisms are also found in biofilms and likely participate in the distribution of nutrients or O2 as well as in the outflow of wastes (Frances et al., 2001). Still, in biofilms microorganisms can survive independently, an ability that was lost in the specialized cells from multicellular organisms (Figure 3C) (Penesyan et al., 2021).
Oxyconformer Pluricellular Organisms
Early in evolution, the control of O2 diffusion into pluricellular organisms was only partial, so organisms had to deal with sudden changes in [O2]. These organisms are termed oxyconformers (van Winkle and Mangum, 1975). Among these the phylum Arthropoda is the most numerous oxyconformer on the planet (Martínez-Cruz et al., 2012; Stork, 2018). Crustaceans are aquatic oxyconformers who confront a circadian cycle of water O2 levels throughout their lifetimes. To avoid overproducing ROS, crustaceans exhibit different strategies. The ghost shrimp Lepidophtalmus louisianensis and the branchiopod Artemia franciscana can survive in severely hypoxic and even anoxic settings by slowing down their respiratory and metabolic rates (Martínez-Cruz et al., 2017). When ambient oxygen levels are low, the white shrimp Litopenaeus vannamei can slow down its metabolic rate controlling mitochondrial function (Jiménez-Gutiérrez et al., 2014).
As in unicellular eukaryotes, alternative oxidase (AOX) is present in hypoxia-tolerant invertebrates from the phylum Porifera, Cnidaria, Nematoda, Annelida, Mollusca, and Echinodermata (McDonald and Gospodaryov, 2019). In these organisms AOX expression is enhanced under stress or hypoxia, e.g., in Urechis unicinctus the levels of AOX mRNA increase under stress (McDonald and Vanlerberghe 2004; McDonald et al., 2009).
AOXA and AOXB are two AOX variants found in abundance in the eastern oyster Crassostrea virginica. Under normal settings, AOXA has greater expression than AOXB, but under hypoxia, AOXB is the dominant form, and suggesting that it might be a stress-adaptation (Liu and Guo, 2017). Under anoxia, A. franciscana cysts may remain for years as a cyst and survive hatching in highly oxygenated water probably aided by AOX activity (Clegg and Conte, 1980; Rodriguez-Armenta et al., 2018). In the bivalve Arctica islandica, when OxPhos activity decreases, AOX helps maintain the mitochondrial respiratory rate probably aiding in prevention of ROS overproduction (Abele et al., 2007). In the Pacific giant oyster Crassostrea gigas, a cycle of hypoxia/re-oxygenation leads to a large increase in AOX expression (Sussarellu et al., 2012).
A mechanism complementing the differential expression of RC alternative redox enzymes, motile aquatic organisms such as L. vannamei and Artemia sp migrate, both during the day or in different stages of growth to those regions in water where they find optimal [O2] for their metabolism (Rosas-Lemus et al., 2016).
Oxyregulators
Beginning with fish, organisms were enveloped in a gas impermeable epithelium that excluded O2. At the same time, specialized organs were developed which delivered exact quantities of O2 to internal tissues. Internalized O2 was not free, but tightly bound to different proteins such as hemoglobin or myoglobin (Bonaventura and Bonaventura, 1980; Reeder and Wilson, 2005). Through this mechanism, organisms are highly efficient to avoid the effect of ambient [O2] variations (Reinhard et al., 2016; Rosas-Lemus et al., 2016; Leiva et al., 2018). These organisms are termed oxyregulators and possess an optimal O2-avoidance system (Prosser, 1955). However, this led to an unwanted consequence as physiological uncoupling mechanisms became obsolete and RC branching disappeared and thus any accidental variation within the organism was highly dangerous. In oxyregulators, RCs lost all alternative redox enzymes, optimizing OxPhos. However, cells became more susceptible to variations in [O₂]. Still, in eukaryotes other PUS, and the proton sinks. It is not clear to what extent these can prevent cell death in oxyregulators.
Extrinsic Physiological Uncoupling Systems (Proton Sinks)
In addition to RC branching, extrinsic mechanisms PUS were developed in eukaryotes. The uncoupling proteins (UCPs) and the permeability transition pore (PTP) are both immersed in the inner mitochondrial membrane (Guerrero-Castillo et al., 2011).
UCPs belong to the six-transmembrane helix family of mitochondrial transporters (Palmieri, 2014). UCPs help minimize ROS generation by partially dissipating the proton electrochemical gradient (Luévano-Martínez, 2012; Berry et al., 2018; Ji et al., 2020). In general, UCPs are needed for protection against stress, although it seems that their functions have drifted in diverse organism and these have been linked to the control of immunity as well as a variety of diseases such as sepsis, diabetes, and cancer (Dutra et al., 2018; Tian et al., 2018; Ding et al., 2019). Proton and chloride transport across lipid bilayers can be catalyzed by UCPs, suggesting that they play a role in decreasing ROS production through OxPhos uncoupling (Echtay et al., 2018; Gaudry and Jastroch, 2019).
Mitochondrial UCP activity is induced by fatty acids and inhibited by purine nucleotides (Lunetti et al., 2022). In insects and crustaceans, the distribution of UCP varies by species, tissues/organs, and developmental stage (Slocinska et al., 2011; Slocinska et al., 2012; Alves-Bezerra et al., 2014; Da-Ré et al., 2014; Mendez-Romero et al., 2020). Different roles have been proposed for mitochondrial UCPs in invertebrates, e.g., in the Drosophila melanogaster, DmUCP5 is involved in metabolic homeostasis (Sánchez-Blanco et al., 2006), while in the cold, at 15°C DmUCP4C is necessary for larvae transition to adulthood, suggesting that uncoupling respiration allows the fly to be cold-tolerant (Da-Ré et al., 2014) and DmUCP4 export aspartate from mitochondria (Lunetti et al., 2022). In the worm Caenorhabditis elegans, UCP4 confers cold tolerance (Iser et al., 2005), and transport succinate (Pfeiffer et al., 2011). In all, the main physiological role of UCPs may be protection against mitochondrial oxidative stress, as demonstrated in mitochondria from the cockroach Gromphadorhina coquereliana (Slocinska et al., 2011), the beetle Zophobas atratus (Slocinska et al., 2013), the blood-sucking bug Rhodnius prolixus (Alves-Bezerra et al., 2014) and the white shrimp Litopenaeus vannamei (Mendez-Romero et al., 2020).
The second extrinsic OxPhos uncoupling system is the permeability transition pore (PTP) (Haworth and Hunter, 1979). PTP seems to undergo different open states, a transient open state allows passage of up to 600 Da molecules (Boyman et al., 2019) while the fully open state is permeable to larger molecules up to 1,500 Da, which has been proposed for years as a mechanism leading to cell death (Akopova and Sagach, 2005; Palmeira and Rolo, 2012). However, PTP opening reversal has been reported, even in proteoliposomes containing bovine ATP synthase (Urbani et al., 2019). PTP opening reversibility, promotes transient small decreases in mitochondrial ΔΨ. Thus, frequent PTP opening/closing cycles (flickering) would result in matrix Ca2+ depletion and increased rate of electron flow that would in turn prevent ROS overproduction. Flickering has been studied mostly in heart, muscle, and astrocytes (Figure 4). In isolated heart mitochondria PTP opening of pore may last 10 min and addition of 1 mM of EGTA can still close the pore and restore ΔΨ (Crompton et al., 1987).
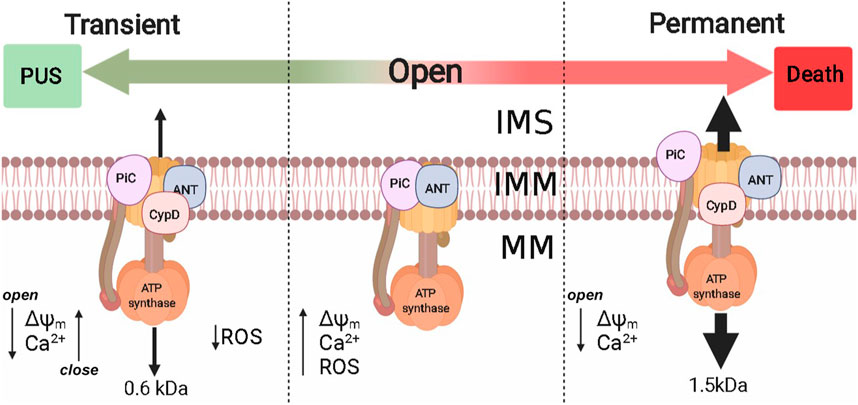
FIGURE 4. Permeability transition pore and their implications in PUS. Extrinsic PUS mechanisms were developed in eukaryotes and are present from yeast to mammals, probably to control intramitochondrial [Ca2+] and ROS production. The molecular identity of PTP is still under debate, although at least Complex V, PiC, ANT, and CypD have been proposed. PTP probably has two opening modes: 1) Transient, where PTP is permeable to molecules up to 600 Da and reversibly decreases ΔΨm [Ca2+]m, decreasing ROS production; 2) Permanent that allows molecules up to 1.5 kDa and metabolite efflux. Nonreversibility may lead to cell death. ΔΨm: mitochondrial transmembrane potential; [Ca2+]m, mitochondrial Ca2+; IMS, intermembrane space; IMM, inner mitochondrial membrane; MM, mitochondrial matrix.
In the rat heart, alternative opening/closing of PTP in a process known as preconditioning decreases mitochondrial Ca2+ and prevents ROS overproduction, which prevents tissue damage (Saotome et al., 2009). Another instance where PTP flickering may be helpful for the heart is during extremely demanding activity where O2 consumption increases up to ten times (Jafri et al., 2001). The transition of PTP opening from transitory to permanent has been linked to heart failure (Giordano and Giordano, 2005; Belosludtsev et al., 2020). In intact cardiac, striated muscle, kidney cells, and astrocytes, PTP reversibility does lead to alternative ΔΨ decrease/increase and Ca2+ release/uptake that in turn lead to decreased ROS as measured by superoxide “flashes” (Li W. et al., 2016; Lu et al., 2016; Boyman et al., 2019; Feng et al., 2019).
Unicellular eukaryotes such as yeast resist wide [O₂] variations much more efficiently than oxyregulators, most of these possess branched respiratory chains, but in addition may express proton sinks. PTP flickering has been observed in these organisms. In S. cerevisiae, the Mitochondrial Unspecific Channel (MUC) (Manon et al., 1998) may remain open for a long time and then be closed by high cytoplasmic Ca2+ during the cell cycle or when haploid yeast mate, which is an event where high amounts of ATP are required (Iida, et al., 1990a; Iida, et al., 1990b). When the S. cerevisiae MUC closes the ΔΨm increases, mitochondrial swelling is prevented and the control on O2 consumption is restored (Cabrera-Orefice et al., 2014; Gutiérrez-Aguilar et al., 2014; Morales-García et al., 2021). A special case is D. hansenii where the MUC is special in the sense that it is sensitive to monovalent cations (Cabrera-Orefice et al., 2010). Mitochondria from oxyconformers such as the branchiopod A. fransciscana and the shrimp species Lepidophthalmus louisianensis, Crangon crangon, Palaemon serratus, and L. vannamei PTP opening is not induced by calcium overloads and these organelles can store high [Ca+2]. The absence of a calcium-regulated PTP in crustaceans has led to propose that other PUS such as UCPs and branched respiratory chains are critical for survival during stress (Menze et al., 2005; Holman and Hand, 2009; Rodriguez-Armenta et al., 2021).
OxPhos Decreases [O2] and Thus Prevents ROS Overproduction
Electrons flow down RC following a redox gradient in a transmembrane sensitive process (Quinlan et al., 2013). During high metabolic activity, when ATP is rapidly consumed, proton gradients are consumed, thus enhancing the rate of electron flow through RC (Nicholls and Ferguson, 2013). The observed high rate of O2 consumption is traditionally known as known as state-3 respiration and results from a partial, reversible decrease in ΔΨm and these conditions decrease ROS production similarly to PUS activation (Guerrero-Castillo et al., 2011). In addition, ATP channeling seems to further promote OxPhos optimization and inhibition of ROS production (Korshunov et al., 1997). That is, the physical interaction of the mitochondrial adenine-nucleotide carrier (ANC) with the cytoplasmic hexokinases and/or the intermembrane space creatine-kinase constitutes a metabolon, where the ATP transported by ANC directly enters the active site of the kinase in an exchange for ADP (da-Silva et al., 2004; Meyer et al., 2006; Santiago et al., 2008). In fact, a recent study in bats, naked mole rats and mice demonstrated that hexokinase association with the adenine-nucleotide carrier optimizes OxPhos efficiency, decreasing ROS production and elongating lifespan (Vyssokikh et al., 2020). Extrapolating from these results it has been proposed that both aging, and lifespan may be controlled by exercising, i.e., by activating state 3 systematically to prevent ROS overproduction (Dey et al., 2016; Delaire et al., 2021).
ROS Detoxification Systems
When all ROS overproduction systems fail, there is still a second line of defense, namely the antioxidant systems that include the enzymes superoxide dismutase (SOD; EC 1.15.1.1), catalase (CAT; EC 1.11.1.6) and the glutathione recycling system made by glutathione peroxidase (GPx; EC 1.11.1.9), glutathione reductase (GR; EC 1.6.4.2), and glutathione S-transferase (GST; 2.5.1.18). In addition, there are molecules, that sequester ROS, such as glutathione, carotenoids, vitamin C, vitamin A, and vitamin E (Li Y. et al., 2016). ROS detoxification systems work at a steady level but are critical during events leading to massive ROS overproduction such as ischemia/reperfusion or hypoxia/reoxygenation.
Antioxidant systems exist in all kingdoms (Ślesak et al., 2016). These are critical for survival of species usually exposed to extremely variable and stressful environments. Some of these are intertidal invertebrates, hypoxia-tolerant fish, and desiccation/freeze-tolerant creatures (Sokolova, 2018). A particular case occurs in Clostridium acetobutylicum, a strict anaerobe organism that contains Fe- and Mn-SODs, heme- and Mn-catalases and in addition 1Fe or 2Fe-SORs that reduce the superoxide anion to H2O2 (Riebe et al., 2009; Morvan et al., 2021).
Final Considerations
O2 and its toxic derivatives are very strong drivers of evolution. All O2-exposed organisms are at risk of being damaged, age and eventually die because of the deleterious actions of ROS. Many systems have been designed to counteract toxicity while using the advantages of aerobic metabolism. However, the two most important mechanisms to prevent ROS toxicity evolved in opposite sense, as avoiding O2 by exclusion became optimal, branching of RC decreased and eventually disappeared. That is, as oxyregulators optimized O2-exclusion, they enhanced their ATP producing efficiency, and slowly lost stress-response ability in their RCs. It remains to be analyzed whether proton sinks those first appeared in eukaryotes have also lost efficiency or not. Substrate channeling as described above is another ROS overproduction prevention system that seems to exist only in eukaryotes. A second line of defense, which was considered only briefly here, are the ROS detoxifying system that play vital roles during crisis such as ischemia/reperfusion and anoxic periods.
Author Contributions
OM-R, CR-G, PC-T, NC-F: writing, review and editing. SU-C: Conceptualization, writing, review and editing, supervision, project administration and funding acquisition.
Funding
OM-R has a Postdoctoral fellowship from CONACYT (Consejo Nacional de Ciencia y Tecnología, Mexico) CVU 639365. CR-G is a MsSc CONACYT fellow, CVU 966402 enrolled in the Ciencias Bioquímicas Program at UNAM; PCT PhD CONACYT fellow CVU 708685 enrolled in the Ciencias Bioquímicas Program at UNAM. Partially funded by a research grant to SUC from UNAM/DGAPA/PAPIIT IN208821.
Conflict of Interest
The authors declare that the research was conducted in the absence of any commercial or financial relationships that could be construed as a potential conflict of interest.
Publisher’s Note
All claims expressed in this article are solely those of the authors and do not necessarily represent those of their affiliated organizations, or those of the publisher, the editors and the reviewers. Any product that may be evaluated in this article, or claim that may be made by its manufacturer, is not guaranteed or endorsed by the publisher.
Acknowledgments
We thank Juan Manuel Barbosa and Ivette Rosas at the computer facility at IFC, Natalia Ivanovna Copitin and Consejo Nacional de Ciencia y Tecnología (CONACyT, National Council for Research and Technology, Mexico) the scholarship to OM-R, CR-G, and PC-T.
References
Abele D., Philipp E., GonzalezPuntarulo P. S. (2007). Marine Invertebrate Mitochondria and Oxidative Stress. Front. Biosci. 12, 933–946. doi:10.2741/2115
Akopova O. V., Sagach V. F. (2005). Calcium Release from the Rat Liver Mitochondria during Collapse of the Membrane Potential. Ukr Biokhim Zh (1999) 77 (3), 68–75.
Alves-Bezerra M., Cosentino-Gomes D., Vieira L. P., Rocco-Machado N., Gondim K. C., Meyer-Fernandes J. R. (2014). Identification of Uncoupling Protein 4 from the Blood-Sucking Insect Rhodnius prolixus and its Possible Role on protection against Oxidative Stress. Insect Biochem. Mol. Biol. 50, 24–33. doi:10.1016/j.ibmb.2014.03.011
Atkuri K. R., Herzenberg L. A., Niemi A.-K., Cowan T., Herzenberg L. A. (2007). Importance of Culturing Primary Lymphocytes at Physiological Oxygen Levels. Proc. Natl. Acad. Sci. U.S.A. 104 (11), 4547–4552. doi:10.1073/pnas.0611732104
Attardo G. M., Scolari F., Malacrida A. (2020). Bacterial Symbionts of Tsetse Flies: Relationships and Functional Interactions between Tsetse Flies and Their Symbionts. Results Probl. Cel Differ. 69, 497–536. doi:10.1007/978-3-030-51849-3_19
Azúa-Bustos A., González-Silva C., Mancilla R. A., Salas L., Palma R. E., Wynne J. J., et al. (2009). Ancient Photosynthetic Eukaryote Biofilms in an Atacama Desert Coastal Cave. Microb. Ecol. 58 (3), 485–496. doi:10.1007/S00248-009-9500-5/FIGURES/7
Bateman A. (2020). Division of Labour in a Matrix, rather Than Phagocytosis or Endosymbiosis, as a Route for the Origin of Eukaryotic Cells. Biol. Direct. 15 (1), 1–33. doi:10.1186/s13062-020-00260-9
Belosludtsev K., Dubinin M., Talanov E., Starinets V., Tenkov K., Zakharova N., et al. (2020). Transport of Ca2+ and Ca2+-dependent Permeability Transition in the Liver and Heart Mitochondria of Rats with Different Tolerance to Acute Hypoxia. Biomolecules 10 (1), 114. doi:10.3390/biom10010114
Berry B. J., Trewin A. J., Amitrano A. M., KimWojtovich M. A. P., Wojtovich A. P. (2018). Use the Protonmotive Force: Mitochondrial Uncoupling and Reactive Oxygen Species. J. Mol. Biol. 430 (21), 3873–3891. doi:10.1016/j.jmb.2018.03.025
Bonaventura J., Bonaventura C. (1980). Hemocyanins Relationships in Their Structure, Function and Assembly. Am. Zool. 20 (1), 7–17. doi:10.1093/icb/20.1.7
Borisov V. B., Siletsky S. A., Nastasi M. R., Forte E. (2021). ROS Defense Systems and Terminal Oxidases in Bacteria. Antioxidants 10 (6), 839. doi:10.3390/antiox10060839
Borisov V. B., Verkhovsky M. I. (2009). Oxygen as Acceptor. EcoSal Plus 3 (2). doi:10.1128/ecosalplus.3.2.7
Boyman L., Coleman A. K., Zhao G., Wescott A. P., Joca H. C., Greiser B. M., et al. (2019). Dynamics of the Mitochondrial Permeability Transition Pore: Transient and Permanent Opening Events. Arch. Biochem. Biophys. 666, 31–39. doi:10.1016/j.abb.2019.03.016
Bradshaw D. J., Marsh P. D., Allison C., Schilling K. M. (1996). Effect of Oxygen, Inoculum Composition and Flow Rate on Development of Mixed-Culture Oral Biof Ilms. Microbiol 142 (3), 623–629. doi:10.1099/13500872-142-3-623/CITE/REFWORKS
Brunori M., Giuffre A., Sarti P. (2005). Cytochrome Oxidase, Ligands and Electrons. J. Inorg. Biochem. 99 (1), 324–336. doi:10.1016/J.JINORGBIO.2004.10.011
Bunn H. F., Poyton R. O. (1996). Oxygen Sensing and Molecular Adaptation to Hypoxia. Physiol. Rev. 76 (3), 839–885. doi:10.1152/PHYSREV.1996.76.3.839
Busiello R. A., Savarese S., Lombardi A. (2015). Mitochondrial Uncoupling Proteins and Energy Metabolism. Front. Physiol. 6 (FEB), 36. doi:10.3389/FPHYS.2015.00036
Cabrera-Orefice A., Chiquete-Félix N., Espinasa-Jaramillo J., Rosas-Lemus M., Guerrero-Castillo S., Peña A., et al. (2014). The Branched Mitochondrial Respiratory Chain from Debaryomyces Hansenii: Components and Supramolecular Organization. Biochim. Biophys. Acta (Bba) - Bioenerg. 1837 (1), 73–84. doi:10.1016/j.bbabio.2013.07.011
Cabrera-Orefice A., Guerrero-Castillo S., Luévano-Martínez L. A., PeñaUribe-Carvajal A. S., Uribe-Carvajal S. (2010). Mitochondria from the Salt-Tolerant Yeast Debaryomyces Hansenii (Halophilic Organelles?). J. Bioenerg. Biomembr. 42 (1), 11–19. doi:10.1007/s10863-009-9264-0
Cabrera-Orefice A., Ibarra-García-Padilla R., Maldonado-Guzmán R., Guerrero-Castillo S., Luévano-Martínez L. A., Pérez-Vázquez V., et al. (2015). The Saccharomyces cerevisiae Mitochondrial Unselective Channel Behaves as a Physiological Uncoupling System Regulated by Ca2+, Mg2+, Phosphate and ATP. J. Bioenerg. Biomembr. 47 (6), 477–491. doi:10.1007/s10863-015-9632-x
Cadenas E. (1989). Biochemistry of Oxygen Toxicity. Annu. Rev. Biochem. 58 (1), 79–110. doi:10.1146/annurev.bi.58.070189.000455
Clegg J. S., Conte F. P. (1980). “A Review of the Cellular and Developmental Biology of artemia,” in The Brine Shrimp artemia. Editors G. Persoone, P. Sorgeloos, O. Roels, and E. Jaspers (Wetteren: Universa Press), Vol. 2, 11–54.
Cortassa S., O'Rourke B., Aon M. A. (2014). Redox-optimized ROS Balance and the Relationship between Mitochondrial Respiration and ROS. Biochim. Biophys. Acta (Bba) - Bioenerg. 1837 (2), 287–295. doi:10.1016/j.bbabio.2013.11.007
Costerton J. W., Lewandowski Z., Caldwell D. E., Korber D. R., Lappin-Scott H. M. (1995). Microbial Biofilms. Annu. Rev. Microbiol. 49, 711–745. doi:10.1146/ANNUREV.MI.49.100195.003431
Cotter P. A., Chepuri V., Gennis R. B., Gunsalus R. P. (1990). Cytochrome O (cyoABCDE) and D (cydAB) Oxidase Gene Expression in Escherichia coli Is Regulated by Oxygen, pH, and the Fnr Gene Product. J. Bacteriol. 172 (11), 6333–6338. doi:10.1128/JB.172.11.6333-6338.1990
Cramton S. E., Gerke C., Schnell N. F., Nichols W. W., Götz F. (1999). The Intercellular Adhesion ( Ica ) Locus Is Present in Staphylococcus aureus and Is Required for Biofilm Formation. Infect. Immun. 67 (10), 5427–5433. doi:10.1128/IAI.67.10.5427-5433.1999
Crompton M., Costi A., Hayat L. (1987). Evidence for the Presence of a Reversible Ca2+-dependent Pore Activated by Oxidative Stress in Heart Mitochondria. Biochem. J. 245 (3), 915–918. doi:10.1042/bj2450915
D'Autréaux B., Toledano M. B. (2007). ROS as Signalling Molecules: Mechanisms that Generate Specificity in ROS Homeostasis. Nat. Rev. Mol. Cel Biol 8 (10), 813–824. doi:10.1038/NRM2256
Da‐Ré C., De Pittà C., Zordan M. A., Teza G., Nestola F., Zeviani M., et al. (2014). UCP4C Mediates Uncoupled Respiration in Larvae of Drosophila melanogaster. EMBO Rep. 15 (5), 586–591. doi:10.1002/embr.201337972
da-Silva W. S., Gómez-Puyou A., de Gómez-Puyou M. T., Moreno-Sanchez R., De Felice F. G., de Meis L., et al. (2004). Mitochondrial Bound Hexokinase Activity as a Preventive Antioxidant Defense. J. Biol. Chem. 279 (38), 39846–39855. doi:10.1074/jbc.M403835200
Davey M. E., O'toole G. A. (2000). Microbial Biofilms: from Ecology to Molecular Genetics. Microbiol. Mol. Biol. Rev. 64 (4), 847–867. doi:10.1128/MMBR.64.4.847-867.2000
de Beer D., Stoodley P., Roe F., Lewandowski Z. (1994). Effects of Biofilm Structures on Oxygen Distribution and Mass Transport. Biotechnol. Bioeng. 43 (11), 1131–1138. doi:10.1002/BIT.260431118
de la Fuente-Núñez C., Reffuveille F., Fernández L., Hancock R. E. (2013). Bacterial Biofilm Development as a Multicellular Adaptation: Antibiotic Resistance and New Therapeutic Strategies. Curr. Opin. Microbiol. 16 (5), 580–589. doi:10.1016/J.MIB.2013.06.013
Delaire L., Courtay A., Fauvernier M., Humblot J., Bonnefoy M. (2021). Integrating a Prevention Care Path into the Daily Life of Older Adults with Mobility Disability Risk: Introducing a Predictive Response Model to Exercise. Clin. Interv. Aging 16, 1617–1629. doi:10.2147/cia.s315112
Demine S., Renard P., Arnould T. (2019). Mitochondrial Uncoupling: a Key Controller of Biological Processes in Physiology and Diseases. Cells 8 (8), 795. doi:10.3390/CELLS8080795
Dey S., Sidor A., O'Rourke B. (2016). Compartment-specific Control of Reactive Oxygen Species Scavenging by Antioxidant Pathway Enzymes. J. Biol. Chem. 291 (21), 11185–11197. doi:10.1074/jbc.M116.726968
Ding Y., Zheng Y., Huang J., Peng W., Chen X., Kang X., et al. (2019). UCP2 Ameliorates Mitochondrial Dysfunction, Inflammation, and Oxidative Stress in Lipopolysaccharide-Induced Acute Kidney Injury. Int. Immunopharmacology 71, 336–349. doi:10.1016/j.intimp.2019.03.043
Dutra M. R. H., Feliciano R. d. S., Jacinto K. R., Gouveia T. L. F., Brigidio E., Serra A. J., et al. (2018). Protective Role of UCP2 in Oxidative Stress and Apoptosis during the Silent Phase of an Experimental Model of Epilepsy Induced by Pilocarpine. Oxidative Med. Cell Longevity 2018, 1–12. doi:10.1155/2018/6736721
Echtay K. S., Bienengraeber M., Mayinger P., Heimpel S., Winkler E., Druhmann D., et al. (2018). Uncoupling Proteins: Martin Klingenberg's Contributions for 40 Years. Arch. Biochem. Biophys. 657, 41–55. doi:10.1016/j.abb.2018.09.006
Escamilla J. E., Barquera B., Ramírez R., García-Horsman A., Del Arenal P. (1988). Role of Menaquinone in Inactivation and Activation of the Bacillus Cereus Forespore Respiratory System. J. Bacteriol. 170 (12), 5908–5912. doi:10.1128/JB.170.12.5908-5912.1988
Falsetta M. L., Steichen C. T., McEwan A. G., Cho C., Ketterer M., Shao J., et al. (2011). The Composition and Metabolic Phenotype of Neisseria Gonorrhoeae Biofilms. Front. Microbio. 2. doi:10.3389/fmicb.2011.00075
Fang F. C., Frawley E. R., Tapscott T., Vázquez-Torres A. (2016). Bacterial Stress Responses during Host Infection. Cell Host & Microbe 20 (2), 133–143. doi:10.1016/j.chom.2016.07.009
Feng S., Dang S., Han T. W., Ye W., Jin P., Cheng T., et al. (2019). Cryo-EM Studies of TMEM16F Calcium-Activated Ion Channel Suggest Features Important for Lipid Scrambling. Cel Rep. 28 (2), 567–579. e564. doi:10.1016/j.celrep.2019.06.023
Fox E. P., Cowley E. S., Nobile C. J., Hartooni N., Newman D. K., Johnson A. D. (2014). Anaerobic Bacteria Grow within Candida Albicans Biofilms and Induce Biofilm Formation in Suspension Cultures. Curr. Biol. 24 (20), 2411–2416. doi:10.1016/J.CUB.2014.08.057
Frances W., Wit M. J. D., Dann J., Gaast S. V. D., Ronde C. E. J. D., Gerneke D. (2001). Early Archean Fossil Bacteria and Biofilms in Hydrothermally-Influenced Sediments from the Barberton Greenstone belt, South Africa. Precambrian Res. 1–2 (106), 93–116. doi:10.1016/S0301-9268(00)00127-3
Garcia-Neto W., Cabrera-Orefice A., Uribe-Carvajal S., Kowaltowski A. J., Alberto Luévano-Martínez L. (2017). High Osmolarity Environments Activate the Mitochondrial Alternative Oxidase in Debaryomyces Hansenii. PLoS One 12 (1), e0169621. doi:10.1371/journal.pone.0169621
Gaudry M. J., Jastroch M. (2019). Molecular Evolution of Uncoupling Proteins and Implications for Brain Function. Neurosci. Lett. 696, 140–145. doi:10.1016/j.neulet.2018.12.027
Giordano F. J., Giordano F. J. (2005). Oxygen, Oxidative Stress, Hypoxia, and Heart Failure. J. Clin. Invest. 115 (3), 500–508. doi:10.1172/JCI200524408.500
Gnaiger E., Steinlechner-Maran R., Méndez G., Eberl T., Margreiter R. (1995). Control of Mitochondrial and Cellular Respiration by Oxygen. J. Bioenerg. Biomembr. 27 (6), 583–596. doi:10.1007/BF02111656
Guerin M., Camougrand N. (1986). The Alternative Oxidase of Candida Parapsilosis. Eur. J. Biochem. 159 (3), 519–524. doi:10.1111/j.1432-1033.1986.tb09917.x
Guerrero-Castillo S., Araiza-Olivera D., Cabrera-Orefice A., Espinasa-Jaramillo J., Gutiérrez-Aguilar M., Luévano-Martínez L. A., et al. (2011). Physiological Uncoupling of Mitochondrial Oxidative Phosphorylation. Studies in Different Yeast Species. J. Bioenerg. Biomembr. 43 (3), 323–331. doi:10.1007/s10863-011-9356-5
Guerrero-Castillo S., Cabrera-Orefice A., Vázquez-Acevedo M., González-Halphen D., Uribe-Carvajal S. (2012). During the Stationary Growth Phase, Yarrowia Lipolytica Prevents the Overproduction of Reactive Oxygen Species by Activating an Uncoupled Mitochondrial Respiratory Pathway. Biochim. Biophys. Acta (Bba) - Bioenerg. 1817 (2), 353–362. doi:10.1016/J.BBABIO.2011.11.007
Guerrero-Castillo S., Vázquez-Acevedo M., González-Halphen D., Uribe-Carvajal S. (2009). In Yarrowia Lipolytica Mitochondria, the Alternative NADH Dehydrogenase Interacts Specifically with the Cytochrome Complexes of the Classic Respiratory Pathway. Biochim. Biophys. Acta (Bba) - Bioenerg. 1787 (2), 75–85. doi:10.1016/j.bbabio.2008.10.008
Guidot D. M., Repine J. E., Kitlowski A. D., Flores S. C., Nelson S. K., Wright R. M., et al. (1995). Mitochondrial Respiration Scavenges Extramitochondrial Superoxide Anion via a Nonenzymatic Mechanism. J. Clin. Invest. 96 (2), 1131–1136. doi:10.1172/JCI118100
Gupta K. J., Neelwarne B., Mur L. A. J. (2015). “Integrating Classical and Alternative Respiratory Pathways,” in Alternative Respiratory Pathways in Higher Plants (Hoboken, NJ, USA: Wiley Blackwell), 3–19. doi:10.1002/9781118789971.ch1
Gutiérrez-Aguilar M., López-Carbajal H. M., Uribe-Alvarez C., Espinoza-Simón E., Rosas-Lemus M., Chiquete-Félix N., et al. (2014). Effects of Ubiquinone Derivatives on the Mitochondrial Unselective Channel of Saccharomyces cerevisiae. J. Bioenerg. Biomembr. 46 (6), 519–527. doi:10.1007/s10863-014-9595-3
Hall C. W., Mah T.-F. (2017). Molecular Mechanisms of Biofilm-Based Antibiotic Resistance and Tolerance in Pathogenic Bacteria. FEMS Microbiol. Rev. 41 (3), 276–301. doi:10.1093/FEMSRE/FUX010
Harrison J., Turner R., Marques L., Ceri H. (2005). Biofilms. Amer. Scientist 93 (6), 508. doi:10.1511/2005.56.977
Haworth R. A., Hunter D. R. (1979). The Ca2+-Induced Membrane Transition in Mitochondria. Arch. Biochem. Biophys. 195 (2), 460–467. doi:10.1016/0003-9861(79)90372-2
Helmerhorst E. J., Murphy M. P., Troxler R. F., Oppenheim F. G. (2002). Characterization of the Mitochondrial Respiratory Pathways in Candida Albicans. Biochim. Biophys. Acta (Bba) - Bioenerg. 1556 (1), 73–80. doi:10.1016/S0005-2728(02)00308-0
Holman J. D., Hand S. C. (2009). Metabolic Depression Is Delayed and Mitochondrial Impairment Averted during Prolonged Anoxia in the Ghost Shrimp, Lepidophthalmus Louisianensis (Schmitt, 1935). J. Exp. Mar. Biol. Ecol. 376 (2), 85–93. doi:10.1016/j.jembe.2009.06.008
Hsia C. C. W., Schmitz A., Lambertz M., Perry S. F., Maina J. N. (2013). Evolution of Air Breathing: Oxygen Homeostasis and the Transitions from Water to Land and Sky. Compr. Physiol. 3 (2), 849–915. doi:10.1002/cphy.c120003
Iida H., Sakaguchi S., Yagawa Y., Anraku Y. (1990a). Cell Cycle Control by Ca2+ in Saccharomyces cerevisiae. J. Biol. Chem. 265 (34), 21216–21222. doi:10.1016/S0021-9258(17)45348-8
Iida H., Yagawa Y., Anraku Y. (1990b). Essential Role for Induced Ca2+ Influx Followed by [Ca2+]i Rise in Maintaining Viability of Yeast Cells Late in the Mating Pheromone Response Pathway. A Study of [Ca2+]i in Single Saccharomyces cerevisiae Cells with Imaging of Fura-2. J. Biol. Chem. 265 (22), 13391–13399. doi:10.1016/S0021-9258(19)38311-5
Inupakutika M. A., Sengupta S., Devireddy A. R., Azad R. K., Mittler R. (2016). The Evolution of Reactive Oxygen Species Metabolism. Exbotj 67 (21), 5933–5943. doi:10.1093/JXB/ERW382
Iser W. B., Kim D., BachmanWolkow E. C., Wolkow C. (2005). Examination of the Requirement for UCP-4, a Putative Homolog of Mammalian Uncoupling Proteins, for Stress Tolerance and Longevity in C. elegans. Mech. Ageing Develop. 126 (10), 1090–1096. doi:10.1016/j.mad.2005.04.002
Jafri M. S., Dudycha S. J., O'Rourke B. (2001). Cardiac Energy Metabolism: Models of Cellular Respiration. Annu. Rev. Biomed. Eng. 3 (1), 57–81. doi:10.1146/annurev.bioeng.3.1.57
Jarmuszkiewicz W., Milani G., Fortes F., Schreiber A. Z., Sluse F. E., Vercesi A. E. (2000). First Evidence and Characterization of an Uncoupling Protein in Fungi Kingdom: CpUCP of Candida Parapsilosis. FEBS Lett. 467 (2), 145–149. doi:10.1016/S0014-5793(00)01138-8
Jarmuszkiewicz W., Woyda-Ploszczyca A., Antos-Krzeminska N., Sluse F. E. (2010). Mitochondrial Uncoupling Proteins in Unicellular Eukaryotes. Biochim. Biophys. Acta (Bba) - Bioenerg. 1797 (6), 792–799. doi:10.1016/j.bbabio.2009.12.005
Jayawardhane J., Cochrane D. W., Vyas P., Bykova N. v., Vanlerberghe G. C., Igamberdiev A. U. (2020). Roles for Plant Mitochondrial Alternative Oxidase under Normoxia, Hypoxia, and Reoxygenation Conditions. Front. Plant Sci. 11 (May). doi:10.3389/fpls.2020.00566
Ji L. L., Yeo D., Kang C., Zhang T. (2020). The Role of Mitochondria in Redox Signaling of Muscle Homeostasis. J. Sport Health Sci. 9, 386–393. doi:10.1016/j.jshs.2020.01.001
Jimenez-Gutierrez L. R., Uribe-Carvajal S., Sanchez-Paz A., Chimeo C., Muhlia-Almazan A. (2014). The Cytochrome C Oxidase and its Mitochondrial Function in the Whiteleg Shrimp Litopenaeus Vannamei during Hypoxia. J. Bioenerg. Biomembr. 46 (3), 189–196. doi:10.1007/s10863-013-9537-5
Johnson R. S. (2019). “Scientific Background. How Cells Sense and Adapt to Oxygen Availability,” in The Nobel Prize in Physiology or Medicine 2019.
Joseph-Horne T., Hollomon D. W., Wood P. M. (2001). Fungal Respiration: a Fusion of Standard and Alternative Components. Biochim. Biophys. Acta 1504 (2), 179–195. doi:10.1016/S0005-2728(00)00251-6
Kadenbach B. (2003). Intrinsic and Extrinsic Uncoupling of Oxidative Phosphorylation. Biochim. Biophys. Acta (Bba) - Bioenerg. 1604 (2), 77–94. doi:10.1016/S0005-2728(03)00027-6
Kerscher S., Dröse S., Zwicker K., Zickermann V., Brandt U. (2002). Yarrowia Lipolytica, a Yeast Genetic System to Study Mitochondrial Complex I. Biochim. Biophys. Acta 1555 (1-3), 83–91. doi:10.1016/S0005-2728(02)00259-1
Kerscher S. J., Okun J. G., Brandt U. (1999). A Single External Enzyme Confers Alternative NADH:ubiquinone Oxidoreductase Activity in Yarrowia Lipolytica. J. Cel Sci. 112 (14), 2347–2354. doi:10.1242/jcs.112.14.2347
Korshunov S. S., Skulachev V. P., Starkov A. A. (1997). High Protonic Potential Actuates a Mechanism of Production of Reactive Oxygen Species in Mitochondria. FEBS Letts 416 (1), 15–18. doi:10.1016/S0014-5793(97)01159-9
Leiva F. P., Garcés C., Verberk W. C. E. P., Care M., Paschke K., Gebauer P. (2018). Differences in the Respiratory Response to Temperature and Hypoxia across Four Life-Stages of the Intertidal Porcelain Crab Petrolisthes Laevigatus. Mar. Biol. 165 (9), 1–10. doi:10.1007/s00227-018-3406-z
Li W., Sun T., Liu B., Wu D., Qi W., Wang X., et al. (2016). Regulation of Mitoflash Biogenesis and Signaling by Mitochondrial Dynamics. Sci. Rep. 6. doi:10.1038/srep32933
Li Y., Wei L., Cao J., Qiu L., Jiang X., Li P., et al. (2016). Oxidative Stress, DNA Damage and Antioxidant Enzyme Activities in the pacific white Shrimp (Litopenaeus Vannamei) when Exposed to Hypoxia and Reoxygenation. Chemosphere 144, 234–240. doi:10.1016/j.chemosphere.2015.08.051
Liu M., Guo X. (2017). A Novel and Stress Adaptive Alternative Oxidase Derived from Alternative Splicing of Duplicated Exon in Oyster Crassostrea Virginica. Sci. Rep. 7, 10785. doi:10.1038/s41598-017-10976-w
Lopez D., Vlamakis H., Kolter R. (2010). Biofilms. Cold Spring Harbor Perspect. Biol. 2 (7), a000398. doi:10.1101/CSHPERSPECT.A000398
López-Barneo J., Simon M. C. (2020). Cellular Adaptation to Oxygen Deficiency beyond the Nobel Award. Nat. Commun. 11 (1), 1–3. doi:10.1038/s41467-020-14469-9
Lu P., Heineke M. H., Koul A., Andries K., Cook G. M., Lill H., et al. (2015). The Cytochrome Bd-type Quinol Oxidase Is Important for Survival of Mycobacterium Smegmatis under Peroxide and Antibiotic-Induced Stress. Sci. Rep. 5 (1), 10333. doi:10.1038/srep10333
Lu X., Kwong J. Q., Molkentin J. D., Bers D. M. (2016). Individual Cardiac Mitochondria Undergo Rare Transient Permeability Transition Pore Openings. Circ. Res. 118 (5), 834–841. doi:10.1161/CIRCRESAHA.115.308093
Luévano-Martínez L. A. (2012). Uncoupling Proteins (UCP) in Unicellular Eukaryotes: True UCPs or UCP1-like Acting Proteins? FEBS Letts 586 (7), 1073–1078. doi:10.1016/j.febslet.2012.03.009
Lunetti P., Gorgoglione R., Curcio R., Marra F., Pignataro A., Vozza A., et al. (2022). Drosophila melanogaster Uncoupling protein-4A (UCP4A) Catalyzes a Unidirectional Transport of Aspartate. Int. J. Mol. Sci. 23 (3), 1020. doi:10.3390/ijms23031020
Lyall R., Nikoloski Z., Gechev T. (2020). Comparative Analysis of ROS Network Genes in Extremophile Eukaryotes. Int. J. Mol. Sci. 21 (23), 9131. doi:10.3390/IJMS21239131
Manon S., Roucou X., Guérin M., Rigoulet M., Guérin B. (1998). Minireview: Characterization of the Yeast Mitochondria Unselective Channel: A Counterpart to the Mammalian Permeability Transition Pore? J. Bioenerg. Biomembr. 30 (5), 419–429. doi:10.1023/A:1020533928491
Martinez-Cruz O., Chimeo C., Rodriguez-Armenta C. M., Muhlia-Almazan A. (2017). Crustacean Bioenergetics: Mitochondrial Adaptive Molecular Responses to Face Environmental Challenges. J. Shellfish Res. 36 (3), 771–786. doi:10.2983/035.036.0327
Martínez-Cruz O., Sanchez-Paz J. A., Garcia-Carreño F. L., Jimenez-Gutierrez L. R., Navarrete del Toro M. A., Muhlia-Almazan A. (2012). Invertebrates Mitochondrial Function and Energetic Challenges Bioenergetics. Croatia: INTECH, 181–218.
McDonald A. E., Gospodaryov D. V. (2019). Alternative NAD(P)H Dehydrogenase and Alternative Oxidase: Proposed Physiological Roles in Animals. Mitochondrion 45, 7–17. doi:10.1016/j.mito.2018.01.009
McDonald A. E., Vanlerberghe G. C., Staples J. F. (2009). Alternative Oxidase in Animals: Unique Characteristics and Taxonomic Distribution. J. Exp. Biol. 212 (16), 2627–2634. doi:10.1242/jeb.032151
McDonald A., Vanlerberghe G. (2004). Branched Mitochondrial Electron Transport in the Animalia: Presence of Alternative Oxidase in Several Animal Phyla. IUBMB Life (International Union Biochem. Mol. Biol. Life) 56, 333–341. doi:10.1080/1521-6540400000876
McGinn J., Lamason R. L. (2021). The Enigmatic Biology of Rickettsiae: Recent Advances, Open Questions and Outlook. Pathog. Dis. 79 (4). doi:10.1093/FEMSPD/FTAB019
Medentsev A., Arinbasarova A., Golovchenko N., Akimenko V. (2002). Involvement of the Alternative Oxidase in Respiration of Mitochondria Is Controlled by the Activity of the Cytochrome Pathway. FEMS Yeast Res. 2 (4), 519–524. doi:10.1111/j.1567-1364.2002.tb00118.x10.1016/s1567-1356(02)00123-x
Medentsev A. G., Arinbasarova A. Y., Akimenko V. K. (1999). Regulation and Physiological Role of Cyanide-Resistant Oxidases in Fungi and Plants. Biochem. (Mosc)Biokhimiia 64 (11), 1230–1243.
Mendez-Romero O., Rodriguez-Armenta C., Uribe-Carvajal S., Muhlia-Almazan A. (2020). Functional Characterization of the Mitochondrial Uncoupling Proteins from the white Shrimp Litopenaeus Vannamei. Biochim. Biophys. Acta (Bba) - Bioenerg. 1861 (8), 148209. doi:10.1016/j.bbabio.2020.148209
Menze M. A., Hutchinson K., Laborde S. M., Hand S. C. (2005). Mitochondrial Permeability Transition in the crustaceanArtemia Franciscana: Absence of a Calcium-Regulated Pore in the Face of Profound Calcium Storage. Am. J. Physiology-Regulatory, Integr. Comp. Physiol. 289 (1), R68–R76. doi:10.1152/ajpregu.00844.2004
Meyer L. E., Machado L. B., Santiago A. P. S. A., da-Silva W. S., De Felice F. G., Holub O., et al. (2006). Mitochondrial Creatine Kinase Activity Prevents Reactive Oxygen Species Generation. J. Biol. Chem. 281 (49), 37361–37371. doi:10.1074/jbc.M604123200
Milani G., Jarmuszkiewicz W., Sluse-Goffart C. M., Schreiber A. Z., Vercesi A. E., Sluse F. E. (2001). Respiratory Chain Network in Mitochondria ofCandida Parapsilosis: ADP/O Appraisal of the Multiple Electron Pathways. FEBS Lett. 508 (2), 231–235. doi:10.1016/S0014-5793(01)03060-5
Morales-García L., Ricardez-García C., Castañeda-Tamez P., Chiquete-Félix N., Uribe-Carvajal S. (2021). Coupling/uncoupling Reversibility in Isolated Mitochondria from Saccharomyces cerevisiae. Life 11 (12), 1307. doi:10.3390/life11121307
Morvan C., Folgosa F., Kint N., Teixeira M., Martin‐Verstraete I. (2021). Responses of Clostridia to Oxygen: from Detoxification to Adaptive Strategies. Environ. Microbiol. 23 (8), 4112–4125. doi:10.1111/1462-2920.15665
Müller M., Mentel M., van Hellemond J. J., Henze K., Woehle C., Gould S. B., et al. (2012). Biochemistry and Evolution of Anaerobic Energy Metabolism in Eukaryotes. Microbiol. Mol. Biol. Rev. 76 (2), 444–495. doi:10.1128/MMBR.05024-11
Nicholls D. G., Ferguson S. J. (2013). Bioenergetics. Cambridge, MA, USA: Academic Press. doi:10.1016/C2010-0-64902-9
Nicholls D. G. (2021). Mitochondrial Proton Leaks and Uncoupling Proteins. Biochim. Biophys. Acta (Bba) - Bioenerg. 1862 (7), 148428. doi:10.1016/J.BBABIO.2021.148428
Norris V., Root-Bernstein R. (2009). The Eukaryotic Cell Originated in the Integration and Redistribution of Hyperstructures from Communities of Prokaryotic Cells Based on Molecular Complementarity. Int. J. Mol. Sci. 10 (6), 2611–2632. doi:10.3390/IJMS10062611
Pahl H. L., Baeuerle P. A. (1994). Oxygen and the Control of Gene Expression. BioEssays 16 (7), 497–502. doi:10.1002/BIES.950160709
Palmeira C. M., Rolo A. P. (2012). Mitochondrial Membrane Potential (ΔΨ) Fluctuations Associated with the Metabolic States of Mitochondria. Methods Mol. Biol. 810, 89–101. doi:10.1007/978-1-61779-382-0_6
Palmieri F. (2014). Mitochondrial Transporters of the SLC25 Family and Associated Diseases: a Review. J. Inherit. Metab. Dis. 37, 565–575. doi:10.1007/s10545-014-9708-5
Pedroza-Dávila U., Uribe-Alvarez C., Morales-García L., Espinoza-Simón E., Méndez-Romero O., Muhlia-Almazán A., et al. (2020). Metabolism, ATP Production and Biofilm Generation by Staphylococcus Epidermidis in Either Respiratory or Fermentative Conditions. AMB Expr. 10 (1). doi:10.1186/S13568-020-00966-Z
Penesyan A., Paulsen I. T., Kjelleberg S., Gillings M. R. (2021). Three Faces of Biofilms: a Microbial Lifestyle, a Nascent Multicellular Organism, and an Incubator for Diversity. NPJ Biofilms Microbiomes 7 (1), 1–9. doi:10.1038/s41522-021-00251-2
Peyssonnaux C., Boutin A. T., Zinkernagel A. S., Datta V., NizetJohnson V. R. S., Johnson R. S. (2008). Critical Role of HIF-1α in Keratinocyte Defense against Bacterial Infection. J. Invest. Dermatol. 128 (8), 1964–1968. doi:10.1038/jid.2008.27
Pfeiffer M., Kayzer E.-B., Yang X., Abramson E., Kenaston M. A., Lago C. U., et al. (2011). Caenorhabditis elegans UCP4 Protein Controls Complex II-Mediated Oxidative Phosphorylation through Succinate Transport. J. Biol. Chem. 286 (43), 37712–37720. doi:10.1074/jbc.M111.271452
Phillips N. J., Steichen C. T., Schilling B., Post D. M. B., Niles R. K., Bair T. B., et al. (2012). Proteomic Analysis of Neisseria Gonorrhoeae Biofilms Shows Shift to Anaerobic Respiration and Changes in Nutrient Transport and Outermembrane Proteins. PLoS ONE 7 (6), e38303. doi:10.1371/journal.pone.0038303
Prosser C. L. (1955). Physiological Variation in Animals. Biol. Rev. 30 (3), 229–261. doi:10.1111/j.1469-185X.1955.tb01208.x
Pysz M. A., Conners S. B., Montero C. I., Shockley K. R., Johnson M. R., Ward D. E., et al. (2004). Transcriptional Analysis of Biofilm Formation Processes in the Anaerobic, Hyperthermophilic Bacterium Thermotoga Maritima. Appl. Environ. Microbiol. 70 (10), 6098–6112. doi:10.1128/AEM.70.10.6098-6112.2004
Quinlan C. L., Perevoshchikova I. V., Hey-Mogensen M., Orr A. L., Brand M. D. (2013). Sites of Reactive Oxygen Species Generation by Mitochondria Oxidizing Different Substrates. Redox Biol. 1 (1), 304–312. doi:10.1016/j.redox.2013.04.005
Rasmusson A. G., Soole K. L., Elthon T. E. (2004). Alternative NAD(P)H Dehydrogenases of Plant Mitochondria. Annu. Rev. Plant Biol. 55 (1), 23–39. doi:10.1146/annurev.arplant.55.031903.141720
Reeder B. J., Wilson M. T. (2005). Hemoglobin and Myoglobin Associated Oxidative Stress: from Molecular Mechanisms to Disease States. Curr. Med. Chem. 12 (23), 2741–2751. doi:10.2174/092986705774463021
Reinhard C. T., Planavsky N. J., Olson S. L., Lyons T. W., Erwin D. H. (2016). Earth's Oxygen Cycle and the Evolution of Animal Life. Proc. Natl. Acad. Sci. U.S.A. 113 (32), 8933–8938. doi:10.1073/pnas.1521544113
Riebe O., Fischer R.-J., Wampler D. A., Kurtz D. M., Bahl H. (2009). Pathway for H2O2 and O2 Detoxification in Clostridium Acetobutylicum. Microbiol (Reading, England) 155 (Pt 1), 16–24. doi:10.1099/mic.0.022756-0
Rigoulet M., Aguilaniu H., Avéret N., Bunoust O., Camougrand N., Grandier-Vazeille X., et al. (2004). Organization and Regulation of the Cytosolic NADH Metabolism in the Yeast Saccharomyces cerevisiae. Mol. Cel. Biochem. 256 (1), 73–81. doi:10.1023/B:MCBI.0000009888.79484.fd
Rodriguez-Armenta C., Reyes-Zamora O., De la Re-Vega E., Sanchez-Paz A., Mendoza-Cano F., Mendez-Romero O., et al. (2021). Adaptive Mitochondrial Response of the Whiteleg Shrimp Litopenaeus Vannamei to Environmental Challenges and Pathogens. J. Comp. Physiol. B. 1-16. doi:10.1007/s00360-021-01369-7
Rodriguez-Armenta C., Uribe-Carvajal S., Rosas-Lemus M., Chiquete-Felix N., Huerta-Ocampo J. A., Muhlia-Almazan A. (2018). Alternative Mitochondrial Respiratory Chains from Two Crustaceans: Artemia Franciscana Nauplii and the white Shrimp, Litopenaeus Vannamei. J. Bioenerg. Biomembr. 50 (2), 143–152. doi:10.1007/s10863-018-9753-0
Rosas-Lemus M., Uribe-Alvarez C., Contreras-Zentella M., Luévano-Martínez L. A., Chiquete-Félix N., Morales-García N. L., et al. (2016). “Oxygen: from Toxic Waste to Optimal (Toxic) Fuel of Life,” in Free Radicals and Diseases (London: InTech). doi:10.5772/63667
Rossignol T., Ding C., Guida A., d'Enfert C., Higgins D. G., Butler G. (2009). Correlation between Biofilm Formation and the Hypoxic Response in Candida Parapsilosis. Eukaryot. Cel. 8 (4), 550–559. doi:10.1128/EC.00350-08
Rumsey W. L., Schlosser C., Nuutinen E. M., Robiolio M., Wilson D. F. (1990). Cellular Energetics and the Oxygen Dependence of Respiration in Cardiac Myocytes Isolated from Adult Rat. J. Biol. Chem. 265 (26), 15392–15399. doi:10.1016/S0021-9258(18)55409-0
Ruy F., Vercesi A. E., Kowaltowski A. J. (2006). Inhibition of Specific Electron Transport Pathways Leads to Oxidative Stress and Decreased Candida Albicans Proliferation. J. Bioenerg. Biomembr. 38 (2), 129–135. doi:10.1007/s10863-006-9012-7
Saari S., Garcia G. S., Bremer K., Chioda M. M., Andjelković A., Debes P. V., et al. (2019). Alternative Respiratory Chain Enzymes: Therapeutic Potential and Possible Pitfalls. Biochim. Biophys. Acta (Bba) - Mol. Basis Dis. 1865 (4), 854–866. doi:10.1016/j.bbadis.2018.10.012
Santiago A. P. S. A., Chaves E. A., OliveiraGalina M. F. A., Galina A. (2008). Reactive Oxygen Species Generation Is Modulated by Mitochondrial Kinases: Correlation with Mitochondrial Antioxidant Peroxidases in Rat Tissues. Biochimie 90 (10), 1566–1577. doi:10.1016/j.biochi.2008.06.013
Saotome M., Katoh H., Yaguchi Y., Tanaka T., Urushida T., Satoh H., et al. (2009). Transient Opening of Mitochondrial Permeability Transition Pore by Reactive Oxygen Species Protects Myocardium from Ischemia-Reperfusion Injury. Am. J. Physiology-Heart Circulatory Physiol. 296 (4), H1125–H1132. doi:10.1152/ajpheart.00436.2008
Sánchez-Blanco A., Fridell Y.-W. C., Helfand S. L. (2006). Involvement of Drosophila Uncoupling Protein 5 in Metabolism and Aging. Genetics 172 (3), 1699–1710. doi:10.1534/genetics.105.053389
Shikama K., Matsuoka A. (2004). Structure-function Relationships in Unusual Nonvertebrate Globins. Crit. Rev. Biochem. Mol. Biol. 39 (4), 217–259. doi:10.1080/10409230490514008
Sies H. (1993). Strategies of Antioxidant Defense. Eur. J. Biochem. 215 (2), 213–219. doi:10.1111/J.1432-1033.1993.TB18025.X
Ślesak I., Ślesak H., Zimak-Piekarczyk P., Rozpądek P. (2016). Enzymatic Antioxidant Systems in Early Anaerobes: Theoretical Considerations. Astrobiology 16 (5), 348–358. doi:10.1089/ast.2015.1328
Slggaard-Andersen O., Ulrich A., Gthgen I. H. (1995). Classes of Tissue Hypoxia. Acta Anaesth. Scand. 39, 137–142. doi:10.1111/j.1399-6576.1995.tb04348.x
Slocinska M., Antos-Krzeminska N., Golebiowski M., Kuczer M., Stepnowski P., Rosinski G., et al. (2013). UCP4 Expression Changes in Larval and Pupal Fat Bodies of the Beetle Zophobas Atratus under Adipokinetic Hormone Treatment. Comp. Biochem. Physiol. A: Mol. Integr. Physiol. 166 (1), 52–59. doi:10.1016/j.cbpa.2013.05.009
Slocinska M., Antos-Krzeminska N., Rosinski G., Jarmuszkiewicz W. (2011). Identification and Characterization of Uncoupling Protein 4 in Fat Body and Muscle Mitochondria from the Cockroach Gromphadorhina Cocquereliana. J. Bioenerg. Biomembr. 43 (6), 717–727. doi:10.1007/s10863-011-9385-0
Slocinska M., Antos-Krzeminska N., Rosinski G., Jarmuszkiewicz W. (2012). Molecular Identification and Functional Characterisation of Uncoupling Protein 4 in Larva and Pupa Fat Body Mitochondria from the Beetle Zophobas Atratus. Comp. Biochem. Physiol. B: Biochem. Mol. Biol. 162 (4), 126–133. doi:10.1016/j.cbpb.2012.03.002
Sokolova I. (2018). Mitochondrial Adaptations to Variable Environments and Their Role in Animals' Stress Tolerance. Integr. Comp. Biol. 58 (3), 519–531. doi:10.1093/icb/icy017
Stamati K., Mudera V., Cheema U. (2011). Evolution of Oxygen Utilization in Multicellular Organisms and Implications for Cell Signalling in Tissue Engineering. J. Tissue Eng. 2 (1), 204173141143236–12. doi:10.1177/2041731411432365
Steinlechner-Maran R., Eberl T., Kunc M., Margreiter R., Gnaiger E. (1996). Oxygen Dependence of Respiration in Coupled and Uncoupled Endothelial Cells. Am. J. Physiology-Cell Physiol. 271 (6), C2053–C2061. doi:10.1152/ajpcell.1996.271.6.C2053
Stewart P. S. (2003). Diffusion in Biofilms. J. Bacteriol. 185 (5), 1485–1491. doi:10.1128/JB.185.5.1485-1491.2003
Stichternoth C., Ernst J. F. (2009). Hypoxic Adaptation by Efg1 Regulates Biofilm Formation by Candida Albicans. Appl. Environ. Microbiol. 75 (11), 3663–3672. doi:10.1128/AEM.00098-09
Stork N. E. (2018). How many Species of Insects and Other Terrestrial Arthropods Are There on Earth? Annu. Rev. Entomol. 63, 31–45. doi:10.1146/annurev-ento-020117-043348
Sussarellu R., Fabioux C., Camacho Sanchez M., Le Goïc N., Lambert C., Soudant P., et al. (2012). Molecular and Cellular Response to Short-Term Oxygen Variations in the Pacific Oyster Crassostrea gigas. J. Exp. Mar. Biol. Ecol. 412, 87–95. doi:10.1016/j.jembe.2011.11.007
Tattersall G. (2007). “Skin Breathing in Amphibians,” in Endothelial Biomedicine: A Comprehensive Reference (Cambridge, UK: Cambridge University Press), 85–91. doi:10.1017/CBO9780511546198
Tian X. Y., Ma S., Tse G., Wong W. T., Huang Y. (2018). Uncoupling Protein 2 in Cardiovascular Health and Disease. Front. Physiol. 9, 1060. doi:10.3389/fphys.2018.01060
Turpaev K. T. (2002). Reactive Oxygen Species and Regulation of Gene Expression. Biochemistry-Moscow 67 (3), 281–292. doi:10.1023/A:1014819832003
Unden G., Bongaerts J. (1997). Alternative Respiratory Pathways of Escherichia coli: Energetics and Transcriptional Regulation in Response to Electron Acceptors. Biochim. Biophys. Acta (Bba) - Bioenerg. 1320 (3), 217–234. doi:10.1016/S0005-2728(97)00034-0
Urbani A., Giorgio V., Carrer A., Franchin C., Arrigoni G., Jiko C., et al. (2019). Purified F-ATP Synthase Forms a Ca2+-dependent High-Conductance Channel Matching the Mitochondrial Permeability Transition Pore. Nat. Commun. 10 (1), 4341. doi:10.1038/s41467-019-12331-1
Uribe‐Alvarez C., Chiquete‐Félix N., Morales‐García L., Bohórquez‐Hernández A., Delgado‐Buenrostro N. L., Vaca L., et al. (2019). Wolbachia Pipientisgrows inSaccharomyces Cerevisiaeevoking Early Death of the Host and Deregulation of Mitochondrial Metabolism. MicrobiologyOpen 8 (4), e00675. doi:10.1002/mbo3.675
Uribe-Alvarez C., Chiquete-Félix N., Contreras-Zentella M., Guerrero-Castillo S., Peña A., Uribe-Carvajal S. (2016). Staphylococcus Epidermidis: Metabolic Adaptation and Biofilm Formation in Response to Different Oxygen Concentrations. Pathog. Dis. 74 (1), ftv111. doi:10.1093/femspd/ftv111
Uribe-Carvajal S., Luévano-Martínez L. A., Guerrero-Castillo S., Cabrera-Orefice A., Corona-de-la-Peña N. A., Gutiérrez-Aguilar M. (2011). Mitochondrial Unselective Channels throughout the Eukaryotic Domain. Mitochondrion 11 (3), 382–390. doi:10.1016/j.mito.2011.02.004
Valentini M., Filloux A. (2016). Biofilms and Cyclic Di-GMP (C-Di-GMP) Signaling: Lessons from Pseudomonas aeruginosa and Other Bacteria. J. Biol. Chem. 291 (24), 12547–12555. doi:10.1074/JBC.R115.711507
van Winkle W., Mangum C. (1975). Oxyconformers and Oxyregulators: A Quantitative index. J. Exp. Mar. Biol. Ecol. 17 (2), 103–110. doi:10.1016/0022-0981(75)90025-8
Vilne B., Grantiņa-Ieviņa L., Ķibilds J., Mališevs A., Konvisers G., Makarova S., et al. (2021). Investigating Bacterial and Free-Living Protozoa Diversity in Biofilms of Hot Water Pipes of Apartment Buildings in the City of Riga (Latvia). Front. Water 3, 179. doi:10.3389/FRWA.2021.799840
Vyssokikh M. Y., Holtze S., Averina O. A., Lyamzaev K. G., Panteleeva A. A., Marey M. V., et al. (2020). Mild Depolarization of the Inner Mitochondrial Membrane Is a Crucial Component of an Anti-aging Program. Proc. Natl. Acad. Sci. U.S.A. 117 (12), 6491–6501. doi:10.1073/pnas.1916414117
Weiss M. C., Sousa F. L., Mrnjavac N., Neukirchen S., Roettger M., Nelson-Sathi S., et al. (2016). The Physiology and Habitat of the Last Universal Common Ancestor. Nat. Microbiol. 1, 16116. doi:10.1038/nmicrobiol.2016.116
Wessel A. K., Arshad T. A., Fitzpatrick M., Connell J. L., Bonnecaze R. T., Shear J. B., et al. (2014). Oxygen Limitation within a Bacterial Aggregate. MBio 5 (2), 992–1006. doi:10.1128/mBio.00992-14
Westall F., Steele A., Toporski J., Walsh M., Allen C., Guidry S., et al. (2000). Polymeric Substances and Biofilms as Biomarkers in Terrestrial Materials: Implications for Extraterrestrial Samples. J. Geophys. Res. 105 (E10), 24511–24527. doi:10.1029/2000JE001250
Wiese M., Gerlach R. G., Popp I., Matuszak J., Mahapatro M., Castiglione K., et al. (2012). Hypoxia-mediated Impairment of the Mitochondrial Respiratory Chain Inhibits the Bactericidal Activity of Macrophages. Infect. Immun. 80 (4), 1455–1466. doi:10.1128/IAI.05972-11
Wilson D. F. (1990). Contribution of Diffusion to the Oxygen Dependence of Energy Metabolism in Cells. Experientia 46 (11–12), 1160–1162. doi:10.1007/BF01936927
Wingender J., Neu T. R., Flemming H.-C. (1999). “What Are Bacterial Extracellular Polymeric Substances?” in Microbial Extracellular Polymeric Substances. Editors J. Wingender, T. R. Neu, and H. C. Flemming (Berlin, Heidelberg: Springer), 1–19. doi:10.1007/978-3-642-60147-7_1
Wu C., Cheng Y.-Y., Yin H., Song X.-N., Li W.-W., Zhou X.-X., et al. (2013). Oxygen Promotes Biofilm Formation of Shewanella Putrefaciens CN32 through a Diguanylate Cyclase and an Adhesin. Sci. Rep. 3 (1), 1–7. doi:10.1038/srep01945
Yamashita T., Inaoka D. K., Shiba T., Oohashi T., Iwata S., Yagi T., et al. (2018). Ubiquinone Binding Site of Yeast NADH Dehydrogenase Revealed by Structures Binding Novel Competitive- and Mixed-type Inhibitors. Sci. Rep. 8 (1), 2427. doi:10.1038/s41598-018-20775-6
Keywords: mitochondria, apoptosis, physiological uncoupling, ROS, oxygen avoidance, oxyregulators, oxyconformers
Citation: Mendez-Romero O, Ricardez-García C, Castañeda-Tamez P, Chiquete-Félix N and Uribe-Carvajal S (2022) Thriving in Oxygen While Preventing ROS Overproduction: No Two Systems Are Created Equal. Front. Physiol. 13:874321. doi: 10.3389/fphys.2022.874321
Received: 12 February 2022; Accepted: 11 March 2022;
Published: 04 April 2022.
Edited by:
Paolo Bernardi, University of Padua, ItalyReviewed by:
Stéphen Manon, CNRS, FranceBoris Victor Chernyak, Lomonosov Moscow State University, Russia
Copyright © 2022 Mendez-Romero, Ricardez-García, Castañeda-Tamez, Chiquete-Félix and Uribe-Carvajal. This is an open-access article distributed under the terms of the Creative Commons Attribution License (CC BY). The use, distribution or reproduction in other forums is permitted, provided the original author(s) and the copyright owner(s) are credited and that the original publication in this journal is cited, in accordance with accepted academic practice. No use, distribution or reproduction is permitted which does not comply with these terms.
*Correspondence: S. Uribe-Carvajal, suribe@ifc.unam.mx