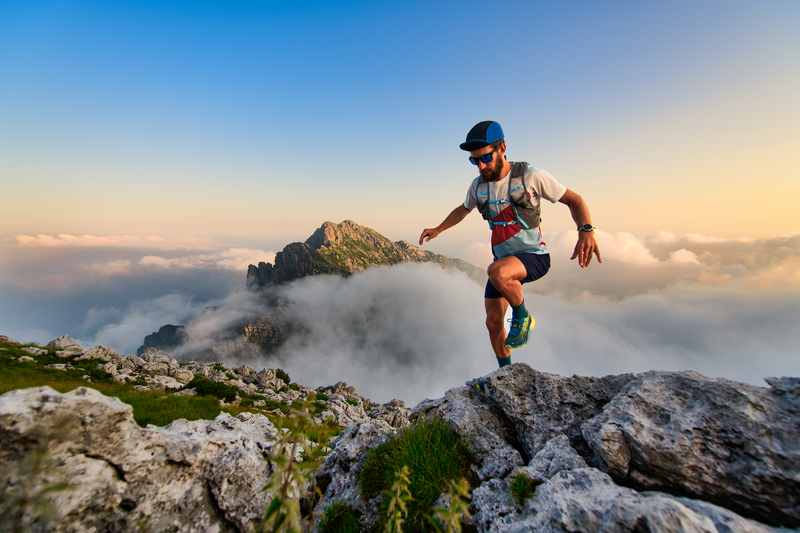
95% of researchers rate our articles as excellent or good
Learn more about the work of our research integrity team to safeguard the quality of each article we publish.
Find out more
HYPOTHESIS AND THEORY article
Front. Physiol. , 04 May 2022
Sec. Metabolic Physiology
Volume 13 - 2022 | https://doi.org/10.3389/fphys.2022.859820
This article is part of the Research Topic Mitochondrial Bioenergetics Impairments in Genetic and Metabolic Diseases View all 6 articles
Metabolic flexibility in mammals enables stressed tissues to generate additional ATP by converting large amounts of glucose into lactic acid; however, this process can cause transient local or systemic acidosis. Certain mammals are adapted to extreme environments and are capable of enhanced metabolic flexibility as a specialized adaptation to challenging habitat niches. For example, naked mole-rats (NMRs) are a fossorial and hypoxia-tolerant mammal whose metabolic responses to environmental stressors markedly differ from most other mammals. When exposed to hypoxia, NMRs exhibit robust hypometabolism but develop minimal acidosis. Furthermore, and despite a very long lifespan relative to other rodents, NMRs have a remarkably low cancer incidence. Most advanced cancers in mammals display increased production of lactic acid from glucose, irrespective of oxygen availability. This hallmark of cancer is known as the Warburg effect (WE). Most malignancies acquire this metabolic phenotype during their somatic evolution, as the WE benefits tumor growth in several ways. We propose that the peculiar metabolism of the NMR makes development of the WE inherently difficult, which might contribute to the extraordinarily low cancer rate in NMRs. Such an adaptation of NMRs to their subterranean environment may have been facilitated by modified biochemical responses with a stronger inhibition of the production of CO2 and lactic acid by a decreased extracellular pH. Since this pH-inhibition could be deeply hard-wired in their metabolic make-up, it may be difficult for malignant cells in NMRs to acquire the WE-phenotype that facilitates cancer growth in other mammals. In the present commentary, we discuss this idea and propose experimental tests of our hypothesis.
In this commentary we address the interplay between glycolysis and oxidative phosphorylation (OxPhos) in humans and most adult mammals, with a particular focus on the physiological flexibility that is enabled by extensive exchange of lactic acid. Of particular interest is the metabolism of naked mole-rats (NMRs; Heterocephalus glaber), in which experimental data and metabolic logic both suggest that lactic acidosis occurs only to a very limited extent. NMRs also have a very low incidence of cancer, a disease in which extracellular lactic acidosis in the tumor microenvironment is a common hallmark.
Based on these observations, we hypothesize that the low incidence of cancer in the NMR is related to two overlapping propositions: (1) lactic acid production by NMR tissues is strongly inhibited by extracellular acidosis, and (2) this sensitivity of NMR cells to acidosis is retained by cancerous cells after malignant transformation. As a result, the Warburg effect (WE), which is central to malignant cancer progression and underlies excessive lactic acidosis in tumors, may be strongly inhibited in this species.
Glycolysis is a ubiquitous metabolic pathway and its constituent enzymes are relatively conserved throughout evolution (Fothergill-Gilmore and Michels, 1993; Bar-Even et al., 2012). Glycolysis yields 2 ATP and 2 pyruvate molecules per glucose molecule catabolized (Bar-Even et al., 2012) (Figure 1). Oxidative phosphorylation (OxPhos) is the mitochondrial high ATP-yield pathway that uses oxygen as the terminal electron acceptor, leading to the full oxidation of pyruvate into CO2 and H2O.
FIGURE 1. Lactate shuttling allows the uncoupling of glycolysis and OxPhos at the cellular level. Simplified depiction of aerobic and anaerobic metabolism of glucose consumption and production or consumption of lactic acid. Intracellular lactate dehydrogenase (LDH) can interconvert pyruvate and lactate and the monocarboxylate transporter (MCT) can export or import lactic acid.
Under maximally stressed conditions, such as maximal exertion, the production of pyruvate is greatly increased. Irrespective of optimal mitochondrial function, sufficient oxygen delivery, and sufficient pO2 at the mitochondrial level, the rate of pyruvate production can be far greater than the ability of OxPhos to metabolize it (Gladden, 2008; Brooks, 2018). Moreover, when mitochondrial metabolism cannot keep up with increased production of pyruvate, the accumulation of pyruvate and NADH will inhibit glycolysis. To allow glycolysis to continue, it is necessary for pyruvate to be reduced into lactate (La−), with the concomitant oxidation of NADH to restore NAD+. This near equilibrium reaction is catalyzed by lactate dehydrogenase (LDH). Together with H+, La− can be exported to the extracellular space via monocarboxylate transporters (MCTs) (Wang et al., 2021). Essentially, all exported La− is later metabolized by the same or different cells via the same route in reverse, i.e., intracellular uptake mediated by MCT and conversion to pyruvate by LDH (Figure 1). Importantly, although the terms lactate and lactic acid are frequently used interchangeably, lactate is exported or imported by the MCT as H+ and La−, or lactic acid (HLa) (Halestrap, 2013). Thus, whenever the term HLa is used herein, we mean the dissociated form, because given its pKa is 3.85, virtually all HLa will be dissociated in vivo (Kellum and Elbers, 2009).
Not surprisingly, LDH and MCTs are expressed in virtually all mammalian tissues (Rabinowitz and Enerbäck, 2020) and lactate is continuously produced and consumed in varying amounts by different tissues and organs. As qualitatively indicated in Figure 2, both whole body metabolism and specific tissue metabolism can display different states depending on time and the involved tissues.
FIGURE 2. Flexible coupling of glycolysis and OxPhos in various states in mammals. Rates of net transport are indicated by the thickness of the arrows during various states as they can develop from rest to increasing stress levels and then recovery: (A) basal metabolism; (B) enhanced aerobic metabolism with matched glycolysis and OxPhos; (C) increased “anaerobic” glycolysis with increased lactic acid production; (D) increased aerobic and anaerobic metabolism as can be seen in muscle during maximal work, for example during a fight-or-flight response. “Aerobic glycolysis” is a common metabolic phenomenon that can be intermittently displayed by many tissues different from muscle to meet specific requirements. State (E) shows that during recovery, excess lactate can be oxidized by the same or other tissues.
Under basal conditions (Figure 2A) or mildly stressed conditions (Figure 2B) oxidation by OxPhos may match pyruvate output from glycolysis. When greater amounts of ATP are required beyond the mitochondrial capacity, glycolysis may outstrip OxPhos with the consequent production of large amounts of HLa (Figure 2C). Figure 2D illustrates even more pronounced metabolism wherein both OxPhos and lactic acid production are increased. This state is observed for example during strenuous muscle exercise wherein both oxygen and glucose consumption may be maximized.
A large percentage of the protons that enter the circulation will be buffered by bicarbonate and lead to increased CO2 exhalation and a decrease in bicarbonate. However, despite this buffering, the exported HLa can lead to decreases in the local or arterial pH to <6.5 and <7.0, respectively. In healthy persons, such pH levels can be observed during severe exertion but in critically ill patients they are associated with poor outcomes (Alkozai et al., 2018). Figure 2E shows how lactic acid is used as a fuel by tissue, for example during recovery after exertion.
The spatio-temporal separation of lactic acid production and consumption allows so-called lactate shuttles to function such that situations (2a) through (2e) can occur simultaneously in different tissues or sequentially in the same tissue (Gladden, 2008; Sun et al., 2017; Brooks, 2018). It is even possible for the same tissue to simultaneously produce and consume lactate (Hui et al., 2017). This gives animals and their tissues the metabolic flexibility required for many stress responses, such as the fight-or-flight reaction. Accordingly, whole-body lactate fluxes are already considerable under basal conditions (>1,000 mmol/d in humans) (Hui et al., 2017; Brooks, 2018; Rabinowitz and Enerbäck, 2020). Since only negligible amounts of lactate are taken up through food or excreted from the body, lactate is arguably the most important intermediate metabolite in mammals (Rabinowitz and Enerbäck, 2020).
Accordingly, Figures 2, 3 are schematic depictions of only net production or consumption of lactic acid. For the sake of simplicity, the figures do not reflect the considerably higher—but often balanced—amounts that are simultaneously being released and taken up, as it also occurs in rest.
FIGURE 3. Hypothesized rigid coupling of glycolysis and OxPhos in the NMR with limited lactic acid production. States (A–D) reflect the large variation in oxygen consumption seen in NMRs depending on ambient temperature and oxygen availability. During hypoxia the NMR can rapidly and dramatically reduce its metabolic rate and oxygen consumption, without resorting to anaerobic glycolysis which would result in lactic acidosis. At 3% O2 the NMRs decreased
Deep hypoxia leads to severe lactic acidosis. In humans and many mammals, a low arterial pO2 will generate both a systemic catecholamine-mediated stress response as well as stress at the cellular level resulting from decreasing ATP levels. Both usually induce an immediate increase of anaerobic glycolysis (Figure 2C) with increased HLa production. However, many biochemical and physiological processes can only transiently cope with severe acidosis, and a prolonged arterial pH < 7.0 is not compatible with life. Since clearance of an increasing HLa load (Figure 2E) under hypoxia is not possible, sustained progressive lactic acidosis will result until the patient or animal dies.
When successful recovery from hypoxia or severe exertion occurs, excess anaerobically generated HLa is cleared from the body. The HLa is either fully oxidized to CO2 (Figure 2E) or regenerated to muscle glycogen or glucose through hepatic or renal gluconeogenesis. Since both processes consume oxygen, this phase is characterized by increased oxygen consumption (
NMRs live mainly in east Africa (Jarvis and Sale, 1971), where large colonies spend virtually their whole life in extensive and sometimes poorly ventilated tunnel systems. To cope with this environment, the NMR possesses some physiological features that are strikingly different from most other adult mammals, and these features support the ability to survive severely challenging levels of hypercapnia and hypoxia. With a lower basal metabolic rate (MR) than expected for its body mass (Lovegrove, 1986), the NMR is an exception to the allometric principle which states that - after log-log transformation - basal MR correlates linearly with body mass (White and Seymour, 2003). Likewise, the NMR has lower circulating glucose levels than other similarly sized mammals (Umminger, 1975; Park et al., 2017). The NMR’s natural habitat is believed to be both hypoxic and hypercapnic in more densely-populated areas (Buffenstein et al., 2022; Pamenter, 2022). Hypercapnia can induce respiratory acidosis and hypoxia can induce metabolic (lactic) acidosis. Cellular acidosis also has significant effects on aerobic mitochondrial respiration because deviations from physiological pH (acidic or alkaline) may shift the mitochondrial H+ gradient, alter the kinetics of the various enzymes of the mitochondrial electron transport chain (ETC), impact reactive oxygen species (ROS) generation, or damage mitochondrial components, among other effects (Forman and Wilson, 1982; Holtzman et al., 1987; Selivanov et al., 2008; Santo-Domingo and Demaurex, 2012; Genders et al., 2019). Thus, unbuffered changes in pH may further impair ATP production in hypoxia and promote cell death.
The NMR tolerates ambient CO2 levels of <7.5% without hyperventilating or developing systemic acidosis, and can survive 80% CO2 for 5 h, which is lethal to mice within 5 min (Park et al., 2017). An important component of this resistance is the loss of the strong sensitivity to ambient CO2 that other mammals possess. Since above-ground CO2 is 0.04% and the end-expiratory CO2 may be ∼5%, direct or indirect sensing of arterial CO2 closely controls minute ventilation in most animals, and thus hypercapnia is a key driver of hyperventilation. But apart from this important sensing consideration where NMRs possess relatively blunted hypercapnic responses (Clayson et al., 2020), high ambient CO2 or high arterial pCO2 as such do not preclude “normal” metabolism, as demonstrated by chronically hypercapnic patients with severe chronic lung disease (Rose and Post, 2001). The usual adaptation in mammals to hypercapnia is to increase circulating bicarbonate levels to restore a normal pH. The Henderson-Hasselbalch equation relates pH, pCO2 and the bicarbonate concentration:
where c depends on temperature and units used. When pH and pCO2 are known, the bicarbonate level is determined accordingly. Subsequently, acid-base principles in mammals (Rose and Post, 2001; Kellum and Elbers, 2009), imply that this increase in bicarbonate requires an adaptive response from the kidneys or gut that leads to an increase in the strong-ion difference. As the strong ion difference is mainly determined by the difference between [Na+] and [Cl−], this response involves either enhanced absorption of sodium and/or enhanced excretion of chloride (Rose and Post, 2001; Kellum and Elbers, 2009), both leading to increased bicarbonate. When the environmental CO2 level is increased, the arterial pCO2 must be at least equal to the environmental pCO2 and when the observed pH then remains (initially) stable, bicarbonate must have increased (Park et al., 2017; Fig S1). It is not yet clear how the NMR might rapidly achieve elevated bicarbonate levels.
Hypoxia poses a more fundamental challenge than hypercapnia for the NMR (and other mammals) (Storz and McClelland, 2017). Since physiological constraints offer only limited possibilities to increase oxygen transport or extraction, oxygen consumption must usually decrease in hypoxia to maintain metabolic equilibrium (Buck and Pamenter, 2006). Whereas hypoxia-intolerant mammals typically attempt to upregulate anaerobic metabolism to maintain ATP supply when oxygen is limited, most hypoxia-tolerant species instead exhibit robust metabolic rate suppression (Seagroves et al., 2001; Hochachka and Somero, 2002). This is presumably because upregulating anaerobic metabolism is limited by finite systemic glycogen stores to fuel glycolysis, and is maladaptive due to the occurrence of progressive acidosis resulting from the accumulation of acidic metabolic end-products (primarily lactate) (Gaesser and Brooks, 1984; Gladden, 2008; Sun et al., 2017). Notably, although fructose metabolism is upregulated in NMRs during anoxia (Park et al., 2017), compared to glucose this does not offer benefits in terms of ATP-production.
Nonetheless, all species must use glycolytic throughput to at least partially compensate for a drastically reduced ability to produce ATP in severe hypoxia and thus many hypoxia-tolerant species have developed elegant strategies to ameliorate the impact of lactic acidosis arising from sustained reliance on anaerobic metabolism. For example, the production of alternative end-products reduces or prevents backlogs of metabolic pathways and cellular acidification in some species (Shoubridge and Hochachka, 1980; Fagernes et al., 2017), while others have developed robust pH-buffering capacities (Jackson 1997; Jackson, 2000; Reese et al., 2004).
Under experimental hypoxia (from 9–3% O2), NMRs rapidly decrease their
Moreover, NMRs do not develop marked systemic acidosis, which occurs in above-ground mammals during hypoxia (Hochachka and Somero, 2002). In fact, after 4 h of progressive hypoxia down to an ambient O2 of 3%, no acidosis but even a mild but significant rise in pH and only a minimal decrease of base excess is observed in most tissues (except in brain, see below) (Pamenter et al., 2019a). Under these conditions the respiratory quotient (RQ; =
On the other hand, some in vivo evidence supports reliance on glycolytic metabolism in the hypoxic NMR, at least to some degree and in certain tissues. For example, in hypoxia we observe mobilization of liver glycogen stores and increase in blood glucose levels. The increase in blood glucose levels may also partly be explained by decreased glucose consumption during hypometabolic states (Pamenter et al., 2019a). In the brain, and following acute in vivo hypoxic exposure, mild acidification is observed, lactate dehydrogenase (LDH) protein expression increases, and the expression of SREP2, a key regulator of fatty acid synthesis, decreases (Hadj-Moussa et al., 2021b), all consistent with increased glycolytic throughput in this tissue. Finally, NMRs have large cardiac glycogen stores relative to C57/BL5 mice (Faulkes et al., 2019), suggesting this organ is primed for sustained glycolytic metabolism. Indeed, it is metabolically advantageous in terms of produced ATP and H+ for the myocardium to use glycogenolysis compared to importing circulating glucose as glycogenolysis does not require the initial ATP investment that occurs when imported glucose is phosphorylated in the first step of glycolysis (Allard et al., 1997).
Thus, the degree to which NMRs rely on glycolysis in acute hypoxia is an open-ended question and likely depends on the severity and duration of hypoxic exposure. It is clear that NMRs can generate lactate (Park et al., 2017), and the moderately elevated lactate levels in brain slices are comparable between mice and NMRs (∼3 umol/g). In rats, blood lactate levels of ∼14 mmol/L have been observed after 1 h of 10% inspiratory oxygen (Alva et al., 2010). Blood lactate levels have to our knowledge not been measured in the NMR under such conditions. However, the observed normal pH of the blood combined with the absence of hyperventilation during hypoxia in NMRs (Pamenter et al., 2019a) effectively precludes the accumulation of the amount of lactic acid seen in other mammals during hypoxia. This suggests that, even if glycolysis is important in certain tissues during hypoxia, localized lactate production in a few organs does not translate to significant increases at the organismal level. Indeed, given the overall capability of NMRs to rapidly reduce
NMRs can live for decades, which is an order of a magnitude more than observed for other mammals with a similar body mass (White and Seymour, 2003; Healy et al., 2014). Despite their long lifespan, NMRs also have an extraordinarily low incidence of cancer compared to other animals (Buffenstein, 2005; Tian et al., 2013; Deweerdt, 2014; Healy et al., 2014; Taylor et al., 2017; Bredberg and Schmitz, 2019). Various hypotheses have been suggested to explain the low incidence of cancer in NMRs (Anonymous, 2013; Miyawaki et al., 2016; Bredberg and Schmitz, 2019). For example, high-molecular-mass hyaluronan, as expressed in the extracellular matrix of NMRs, has been proposed as a contact inhibitor of tumor growth (Tian et al., 2013), although a more recent study was unable to reproduce key observations underlying this hypothesis (Hadi et al., 2020). Alternatively, some authors have suggested that the positive relationship between animals’ longevity and body size may related to the relatively lower incidence of cancer in larger animals, since these animals have a lower specific MR (i.e. BMR indexed for body weight) (Dang 2015). NMRs also maintain lower basal levels of pleiotropic hormones including thyroxine, cortisol and insulin, which appears to be intimately connected to expanded lifespan (Buffenstein et al., 2001; Buffenstein and Pinto 2009). Intriguingly, others have noted that the ability of NMRs to withstand hypoxia may be related to both their very long lifespan and their low cancer incidence, for example because of better defenses against oxygen radical formation (Rodriguez et al., 2011; Hawkins et al., 2019; Munro et al., 2019).
A large majority of solid and hematological malignancies acquire the WE (Vander Heiden et al., 2009) between the initiation of abnormal growth resulting from a mutated cell and the time when a macroscopic cancer becomes clinically detectable (Warburg et al., 1927). The WE is a final common metabolic phenotype that can result from various mutations and is recognized as one of the hallmarks of cancer (Hanahan and Weinberg, 2011). The WE involves the uptake of large amounts of glucose and the export of lactic acid by tumors, irrespective of the presence of oxygen (Warburg et al., 1927). Otto Warburg claimed that these findings were caused by disruptions in mitochondrial bioenergetics (Warburg, 1956); however, this has been demonstrated not to be true and in fact many studies indicate that tumoral mitochondrial function remains intact (Borst, 1960; Racker, 1972).
The WE supports many of the tumor’s invasive purposes (Vander Heiden et al., 2009). The WE boosts biomass generation of the tumor (Vander Heiden et al., 2009) but also results in considerable release of lactic acid to the extracellular space (Horsman and Vaupel, 2016; de la Cruz-Lopéz et al., 2019; Ji et al., 2019; Boedtkjer and Pedersen, 2020). This local acidosis also assists tumor growth as it can degrade the tumor environment and is associated with increased resistance to chemo-, radio- and immuno-therapy (Roma-Rodrigues et al., 2019; Boedtkjer and Pedersen, 2020). Importantly from a clinical perspective, this large glucose uptake by cancers that display the WE is the basis of the extra-ordinary utility of the glucose analogue fluorodeoxyglucose positron emission tomography (FDG-PET) for diagnosis and follow-up of many cancers (Hustinx et al., 2002; Mandelkern and Raines, 2002; Ide and Suzuki, 2005).
It should be underscored that the so-called aerobic glycolysis, i.e., the conversion of glucose to lactic acid while abundant oxygen is available, occurs commonly in physiology, so it is not unique to the WE. Aerobic glycolysis is a common metabolic phenomenon that can be intermittently displayed by many tissues to meet specific requirements, e.g., by the muscles during a sprint (Figure 2D). The key distinction between the WE and physiological aerobic glycolysis, is that the WE is a continuous process that is not under physiological control.
Based on the principles and data discussed above, we conclude that unlike for other mammals (Figure 4, upper panel), uncoupling of glycolysis and OxPhos does not confer advantages to the NMR, which has devised a unique strategy of immediate hypometabolism when confronted with hypoxia.
FIGURE 4. Proposed metabolic difference between surface mammals and NMRs with respect to coupling of glycolysis and OxPhos. The generation of lactic acid allows tissues to more flexibly generate ATP. A consequence of this flexibility is transient regional or systemic acidosis. We hypothesize that acidosis is strongly inhibited in the NMR, and that consequently the coupling of glycolysis and OxPhos must be much stronger in NMRs, to avoid undesired pH-decreases. Accordingly, it is proposed that, compared to above-ground mammals, the NMR will display less acidosis and lower lactate levels under various stress conditions.
Therefore, we hypothesize that:
a) in the NMR (or in most NMR tissues), glycolysis and OxPhos are strongly coupled (Figure 4, lower panel) to avoid either systemic or local lactic acidosis resulting from glycolysis outrunning OxPhos. Strong inhibition of glycolysis by lowered pH would be the most obvious mechanism for the NMR to achieve this, given pH’s central role in regulating biochemical reactions.
b) since it is important that this inhibition should hold across tissues and organs, the higher pH-setpoint for inhibition of glycolysis could be deeply hardwired in the NMR’s biochemistry. Thus, even malignant cells cannot circumvent this inhibition to develop the WE.
It should be noted that the observation in NMRs of mildly elevated lactate levels without acidosis, as has been reported under normal FiO2 conditions (Holtze et al., 2020) does not contradict this hypothesis. Lactate can be produced by stressed tissues and consumed when sufficient oxygen is available, as long as acidosis is avoided. But in contrast to above-ground mammals that can physiologically develop marked hyperlactatemia and acidosis (i.e., lactate>10 mmol/L and arterial pH < 7.0), this may not occur in the NMR.
Notably, metabolic demand may overcome this limitation in certain tissues in which glycolysis may proceed to some extent (e.g., brain) but NMRs appear to possess numerous defenses against cancer progression, which may explain low incidence rates of brain cancer in this organism, even if the WE were not inhibited in this tissue.
Several clear implications of the strong inhibition of glycolysis by acidosis could be verified through comparisons of NMRs with other animals.
At the whole animal level, the most straightforward test would be to perform blood gas analyses, including lactate measurements, in NMRs under hypoxia. Although hyperlactatemia has been abundantly demonstrated in many mammalian models, this has unfortunately not been assessed in NMRs, since in vivo blood sampling is far more difficult in the NMR than in mice due to anatomical differences. Once this technical challenge is addressed, our hypothesis predicts lower lactate levels in NMRs than in mice under hypoxia and other comparable stress conditions such as heavy exertion. Alternatively, artificially induced metabolic acidosis (e.g., through oral administration of ammonium chloride) should decrease glycolysis and induce hypometabolism. Likewise, administration of lactate to NMRs in the form of HLa or as sodium lactate (NaLa) could be used to compare the ability of NMRs to clear lactate with that of other animals. Labelling with stable isotopes of important substrates may help define how metabolic fluxes differ and how they are affected by pH changes.
Alternatively, at the tissue or cellular levels, the coupling of glycolysis and OxPhos could be compared in vitro under various conditions, including under varying oxygen, glucose and lactate concentrations, and for different extracellular pH levels, all of which are relatively easy to manipulate in these ex vivo systems. It would also be instructive to determine the pH sensitivity of the enzymes of glycolysis (e.g., phosphofructokinase) in the NMR. Likewise, the general expression of LDH or MCT’s may be lower, leading to lower generation of HLa. Alternatively, post-translational changes in LDH might result in lower enzyme activity (Pamenter et al., 2019b).
Beyond these “baseline” measures, several cancer-targeted investigations could help test our hypothesis. Only a few cases of cancer in NMRs have been reported and described in detail postmortem (Taylor et al., 2017). Samples from these cancers, if obtained, could support histochemical and molecular analysis to establish if they do or do not conform to the Warburg phenotype. Alternatively, xenografts of modified NMR cells transplanted into mice have been performed (Tian et al., 2013), and these tumors could be assessed for the WE, for example with FDG-PET scanning. In the case of xenografts when increased tissue glycolysis is observed, it should be verified if host cells such as fibroblasts or the malignant cells are contributing to the WE. Cultured malignant transformed NMR cells could also be assessed to determine whether or not they have a strongly glycolytic profile as seen for many cancer cells from other animals. Likewise, the effect of the pH of the culture medium on growth and metabolism could be examined.
It is not unreasonable to assume that the NMR’s unique metabolism, which suits its underground habitat, is in some way related to its remarkable resistance to cancer. The ability of NMRs to tolerate and exercise in hypoxic environments without significant acidosis is a striking metabolic characteristic of this mammal. Thus, we hypothesize that the low cancer incidence in the NMR may be related to a hard-wired coupling of glycolysis with OxPhos leading to the inability to express the Warburg effect, a phenotype that characterizes most cancers in other mammals.
PFJ and MN wrote the first draft of the manuscript. PFJ, MN, and MP wrote sections of the manuscript. MR and MG made important intellectual contributions to the manuscript. All authors contributed to manuscript revision, read, and approved the submitted version.
The authors declare that the research was conducted in the absence of any commercial or financial relationships that could be construed as a potential conflict of interest.
All claims expressed in this article are solely those of the authors and do not necessarily represent those of their affiliated organizations, or those of the publisher, the editors and the reviewers. Any product that may be evaluated in this article, or claim that may be made by its manufacturer, is not guaranteed or endorsed by the publisher.
ATP, adenosine triphosphate; BMR, basal metabolic rate; FDG-PET, fluorodeoxyglucose positron emission tomography; HLa, lactic acid; La−, lactate; LDH, lactate dehydrogenase; MCT, monocarboxylic acid transporter; MR, metabolic rate; NADH, Nicotinamide-adenine-dinucleotide; NMR, naked mole-rat; OxPhos, oxidative phosphorylation; pCO2, pO2; RQ, respiratory quotient;
Alkozai E. M., Mahmoodi B. K., Decruyenaere J., Porte R. J., Oude Lansink-Hartgring A., Lisman T., et al. (2018). Systematic Comparison of Routine Laboratory Measurements with In-Hospital Mortality: ICU-Labome, a Large Cohort Study of Critically Ill Patients. Clin. Chem. Lab. Med. 56 (7), 1140–1151. doi:10.1515/cclm-2016-1028
Allard M. F., Henning S. L., Wambolt R. B., Granleese S. R., English D. R., Lopaschuk G. D. (1997). Glycogen Metabolism in the Aerobic Hypertrophied Rat Heart. Circulation 96 (2), 676–682. doi:10.1161/01.cir.96.2.676
Alva N., Carbonell T., Palomeque J. (2010). Hypothermic protection in an Acute Hypoxia Model in Rats: Acid-Base and Oxidant/antioxidant Profiles. Resuscitation 81 (5), 609–616. doi:10.1016/j.resuscitation.2010.01.023
A. Rodriguez K., Wywial E., I. Perez V., J. Lambert A., H. Edrey Y., N. Lewis K., et al. (2011). Walking the Oxidative Stress Tightrope: a Perspective from the Naked Mole-Rat, the Longest-Living Rodent. Cpd 17, 2290–2307. doi:10.2174/138161211797052457
Bar-Even A., Flamholz A., Noor E., Milo R. (2012). Rethinking Glycolysis: on the Biochemical Logic of Metabolic Pathways. Nat. Chem. Biol. 8 (6), 509–517. doi:10.1038/nchembio.971
Boedtkjer E., Pedersen S. F. (2020). The Acidic Tumor Microenvironment as a Driver of Cancer. Annu. Rev. Physiol. 82 (82), 103–126. doi:10.1146/annurev-physiol-021119-034627
Borst P. (1960). Preparation and Properties of Mitochondria from Ehrlich Ascites Tumor Cells. J. Biophys. Biochem. Cytol. 7, 381–383. doi:10.1083/jcb.7.2.381
Bredberg A., Schmitz B. (2019). Human Cancer, the Naked Mole Rat and Faunal Turnovers. Cancer Med. 8, 1652–1654. doi:10.1002/cam4.2011
Brooks G. A. (2018). The Science and Translation of Lactate Shuttle Theory. Cel Metab. 27 (4), 757–785. doi:10.1016/j.cmet.2018.03.008
Buck L. T., Pamenter M. E. (2006). Adaptive Responses of Vertebrate Neurons to Anoxia-Matching Supply to Demand. Respir. Physiol. Neurobiol. 154 (1-2), 226–240. doi:10.1016/j.resp.2006.03.004
Buffenstein R., Amoroso V., Andziak B., Avdieiev S., Azpurua J., Barker A. J., et al. (2022). The Naked Truth: a Comprehensive Clarification and Classification of Current 'myths' in Naked Mole-Rat Biology. Biol. Rev. Camb Philos. Soc. Feb 97 (1), 115–140. doi:10.1111/brv.12791
Buffenstein R., Pinto M. (2009). Endocrine Function in Naturally Long-Living Small Mammals. Mol. Cel Endocrinol 299, 101–111. doi:10.1016/j.mce.2008.04.021
Buffenstein R. (2005). The Naked Mole-Rat: a New Long-Living Model for Human Aging Research. Journals Gerontol. Ser. A: Biol. Sci. Med. Sci. 60, 1369–1377. doi:10.1093/gerona/60.11.1369
Buffenstein R., Woodley R., Thomadakis C., Daly T. J. M., Gray D. A. (2001). Cold-induced Changes in Thyroid Function in a Poikilothermic Mammal, the Naked Mole-Rat. Am. J. Physiology-Regulatory, Integr. Comp. Physiol. 280, R149–R155. doi:10.1152/ajpregu.2001.280.1.R149
Clayson M. S., Devereaux M. E. M., Pamenter M. E. (2020). Neurokinin-1 Receptor Activation Is Sufficient to Restore the Hypercapnic Ventilatory Response in the Substance P-Deficient Naked Mole-Rat. Am. J. Physiology-Regulatory, Integr. Comp. Physiol. 318 (4), R712–R721. doi:10.1152/ajpregu.00251.2019
Cori C. F. (1981). The Glucose-Lactic Acid Cycle and Gluconeogenesis. Curr. Top. Cel Regul 18, 377–387. doi:10.1016/b978-0-12-152818-8.50028-1
Dang C. V. (2015). A Metabolic Perspective of Peto's Paradox and Cancer. Phil. Trans. R. Soc. B 370 (1673), 20140223. doi:10.1098/rstb.2014.0223
de la Cruz-López K. G., Castro-Muñoz L. J., Reyes-Hernández D. O., García-Carrancá A., Manzo-Merino J. (2019). Lactate in the Regulation of Tumor Microenvironment and Therapeutic Approaches. Front. Oncol. 1 (9), 1143. doi:10.3389/fonc.2019.01143
Fagernes C. E., Stensløkken K.-O., Røhr Å. K., Berenbrink M., Ellefsen S., Nilsson G. E. (2017). Extreme Anoxia Tolerance in Crucian Carp and Goldfish through Neofunctionalization of Duplicated Genes Creating a New Ethanol-Producing Pyruvate Decarboxylase Pathway. Sci. Rep. 7 (1), 7884. doi:10.1038/s41598-017-07385-4
Faulkes C. G., Eykyn T. R., Aksentijevic D. (2019). Cardiac Metabolomic Profile of the Naked Mole-Rat-Glycogen to the rescue. Biol. Lett. 15, 20190710. doi:10.1098/rsbl.2019.0710
Forman N. G., Wilson D. F. (1982). Energetics and Stoichiometry of Oxidative Phosphorylation from NADH to Cytochrome C in Isolated Rat Liver Mitochondria. J. Biol. Chem. 257 (21), 12908–12915. doi:10.1016/s0021-9258(18)33601-9
Fothergill-Gilmore L. A., Michels P. A. M. (1993). Evolution of Glycolysis. Prog. Biophys. Mol. Biol. 59 (2), 105–235. doi:10.1016/0079-6107(93)90001-Z
Gaesser G. A., Brooks G. A. (1984). Metabolic Bases of Excess post-exercise Oxygen Consumption. Med. Sci. Sports Exerc. 16, 29–43. doi:10.1249/00005768-198401000-00008
Genders A. J., Martin S. D., McGee S. L., Bishop D. J. (2019). A Physiological Drop in pH Decreases Mitochondrial Respiration, and HDAC and Akt Signaling, in L6 Myocytes. Am. J. Physiology-Cell Physiol. 316, C404–C414. doi:10.1152/ajpcell.00214.2018
Gladden L. B. (2008). A "Lactatic" Perspective on Metabolism. Med. Sci. Sports Exerc. Mar. 40 (3), 477–485. doi:10.1249/MSS.0b013e31815fa580
Gu C., Jun J. C. (2018). Does Hypoxia Decrease the Metabolic Rate? Front. Endocrinol. 9, 668. doi:10.3389/fendo.2018.00668
Hadi F., Kulaberoglu Y., Lazarus K. A., Bach K., Ugur R., Beattie P., et al. (2020). Transformation of Naked Mole-Rat Cells. Nature 583, E1–E7. doi:10.1038/s41586-020-2410-x
Hadj‐Moussa H., Pamenter M. E., Storey K. B. (2021b). Hypoxic Naked Mole-Rat Brains Use microRNA to Coordinate Hypometabolic Fuels and Neuroprotective Defenses. J. Cel Physiol 236, 5080–5097. doi:10.1002/jcp.30216
Hadj-Moussa H., Chiasson S., Cheng H., Eaton L., Storey K. B., Pamenter M. E. (2021a). MicroRNA-mediated Inhibition of AMPK Coordinates Tissue-specific Downregulation of Skeletal Muscle Metabolism in Hypoxic Naked Mole-Rats. J. Exp. Biol. Aug1 224 (15), jeb242968. doi:10.1242/jeb.242968
Halestrap A. P. (2013). Monocarboxylic Acid Transport. Compr. Physiol. 3, 1611–1643. doi:10.1002/cphy.c130008
Hanahan D., Weinberg R. A. (2011). Hallmarks of Cancer: the Next Generation. Cell 144, 646–674. doi:10.1016/j.cell.2011.02.013
Hardie D. G., Ross F. A., Hawley S. A. (2012). AMPK: a Nutrient and Energy Sensor that Maintains Energy Homeostasis. Nat. Rev. Mol. Cel Biol 13 (4), 251–262. doi:10.1038/nrm3311
Hawkins L. J., Hadj-Moussa H., Nguyen V. C., Pamenter M. E., Storey K. B. (2019). Naked Mole Rats Activate Neuroprotective Proteins during Hypoxia. J. Exp. Zool. 331, 571–576. doi:10.1002/jez.2321
Healy K., Guillerme T., Finlay S., Kane A., Kelly S. B. A., McClean D., et al. (2014). Ecology and Mode-Of-Life Explain Lifespan Variation in Birds and Mammals. Proc. R. Soc. B. 281, 20140298. doi:10.1098/rspb.2014.0298
Hochachka P. W., Somero G. N. (2002). Biochemical Adaptation: Mechanisms and Process in Physiological Evolution. New York: Oxford University Press, 466.
Holtze S., Koch R., Hildebrandt T. B., Lemma A., Szafranski K., Platzer M., et al. (2020). Hematologic Adaptation to the Subterranean Environment by the Naked Mole-Rat, Heterocephalus glaber (Ctenohystrica: Heterocephalidae). J. Mammalogy 101, 1000–1009. doi:10.1093/jmammal/gyaa053
Holtzman D., Olson J. E., Nguyen H., Hsu J., Lewiston N. (1987). Brain Cellular and Mitochondrial Respiration in media of Altered pH. Metab. Brain Dis. 2, 127–137. doi:10.1007/BF00999723
Horsman M. R., Vaupel P. (2016). Pathophysiological Basis for the Formation of the Tumor Microenvironment. Front. Oncol. 6 (6), 66. doi:10.3389/fonc.2016.00066
Hui S., Ghergurovich J. M., Morscher R. J., Jang C., Teng X., Lu W., et al. (2017). Glucose Feeds the TCA Cycle via Circulating Lactate. Nature 551, 115–118. doi:10.1038/nature24057
Hustinx R., Bénard F., Alavi A. (2002). Whole-body FDG-PET Imaging in the Management of Patients with Cancer. Semin. Nucl. Med. 32, 35–46. doi:10.1053/snuc.2002.29272
Ide M., Suzuki Y. (2005). Is Whole-Body FDG-PET Valuable for Health Screening? Eur. J. Nucl. Med. Mol. Imaging 32, 339–341. doi:10.1007/s00259-005-1774-3
Ilacqua A. N., Kirby A. M., Pamenter M. E. (2017). Behavioural Responses of Naked Mole Rats to Acute Hypoxia and Anoxia. Biol. Lett. 13, 20170545. doi:10.1098/rsbl.2017.0545
Jackson D. (1997). Lactate Accumulation in the Shell of the Turtle Chrysemys picta Bellii during Anoxia at 3°C and 10°C. J. Exp. Biol. 200 (Pt 17), 2295–2300. doi:10.1242/jeb.200.17.2295
Jackson D. C. (2000). Living without Oxygen: Lessons from the Freshwater Turtle. Comp. Biochem. Physiol. A: Mol. Integr. Physiol. 125, 299–315. doi:10.1016/s1095-6433(00)00160-4
J. A. Kellum, and P. W. G. Elbers (Editors) (2009). Stewart's Textbook of Acid Base. 2nd ed. (Amsterdam: Acidbase.org).
Jarvis J. U. M., Sale J. B. (1971). Burrowing and Burrow Patterns of East African Mole‐rats Tachyoryctes, Heliophobius and Heterocephalus. J. Zoolog. 163, 451–479. doi:10.1111/j.1469-7998.1971.tb04544.x
Ji K., Mayernik L., Moin K., Sloane B. F. (2019). Acidosis and Proteolysis in the Tumor Microenvironment. Cancer Metastasis Rev. 38 (1-2), 103–112. doi:10.1007/s10555-019-09796-3
Lovegrove B. G. (19861986). The Metabolism of Social Subterranean Rodents: Adaptation to Aridity. Oecologia 69 (4), 551–555. doi:10.1007/BF00410361
Mandelkern M., Raines J. (2002). Positron Emission Tomography in Cancer Research and Treatment. Technol. Cancer Res. Treat. 1, 423–439. doi:10.1177/153303460200100603
Miyawaki S., Kawamura Y., Oiwa Y., Shimizu A., Hachiya T., Bono H., et al. (2016). Tumour Resistance in Induced Pluripotent Stem Cells Derived from Naked Mole-Rats. Nat. Commun. 7, 11471. doi:10.1038/ncomms11471
Munro D., Baldy C., Pamenter M. E., Treberg J. R. (2019). The Exceptional Longevity of the Naked Mole‐rat May Be Explained by Mitochondrial Antioxidant Defenses. Aging Cell 18, e12916. doi:10.1111/acel.12916
National Center for Biotechnology Information (2021). PubChem Pathway Summary for Pathway WP1946, Cori Cycle, Source: WikiPathways. https://pubchem.ncbi.nlm.nih.gov/pathway/WikiPathways:WP1946 (Accessed December 9, 2021).
Pamenter M. E. (2022). Adaptations to a Hypoxic Lifestyle in Naked Mole-Rats. J. Exp. Biol. Feb 15 225 (4), jeb196725. doi:10.1242/jeb.196725
Pamenter M. E., Dzal Y. A., Thompson W. A., Milsom W. K. (2019a). Do naked Mole Rats Accumulate a Metabolic Acidosis or an Oxygen Debt in Severe Hypoxia? J. Exp. Biol. 222. doi:10.1242/jeb.191197
Pamenter M. E., Lau G. Y., Richards J. G., Milsom W. K. (2018). Naked Mole Rat Brain Mitochondria Electron Transport System Flux and H+ Leak Are Reduced during Acute Hypoxia. J. Exp. Biol. 221, jeb171397. doi:10.1242/jeb.171397
Pamenter M. E., Uysal-Onganer P., Huynh K. W., Kraev I., Lange S. (2019b). Post-translational Deimination of Immunological and Metabolic Protein Markers in Plasma and Extracellular Vesicles of Naked Mole-Rat (Heterocephalus glaber). Ijms 20, 5378. doi:10.3390/ijms20215378
Park T. J., Reznick J., Peterson B. L., Blass G., Omerbašić D., Bennett N. C., et al. (2017). Fructose-driven Glycolysis Supports Anoxia Resistance in the Naked Mole-Rat. Science 356 (6335), 307–311. doi:10.1126/science.aab3896
Rabinowitz J. D., Enerbäck S. (2020). Lactate: the Ugly Duckling of Energy Metabolism. Nat. Metab. 2, 566–571. doi:10.1038/s42255-020-0243-4
Reese S. A., Ultsch G. R., Jackson D. C. (2004). Lactate Accumulation, Glycogen Depletion, and Shell Composition of Hatchling Turtles during Simulated Aquatic Hibernation. J. Exp. Biol. 207, 2889–2895. doi:10.1242/jeb.01124
Roma-Rodrigues C., Mendes R., Baptista P., Fernandes A. (2019). Targeting Tumor Microenvironment for Cancer Therapy. Ijms 20 (4), 840. doi:10.3390/ijms20040840
Rose B. D., Post T. W. (2001). Clinical Physiology of Acid-Base and Electrolyte Disorders. 5th Edition. New York: McGraw-Hill.
Santo-Domingo J., Demaurex N. (2012). The Renaissance of Mitochondrial pH. J. Gen. Physiol. 139, 415–423. doi:10.1085/jgp.201110767
Seagroves T. N., Ryan H. E., Lu H., Wouters B. G., Knapp M., Thibault P., et al. (2001). Transcription Factor HIF-1 Is a Necessary Mediator of the Pasteur Effect in Mammalian Cells. Mol. Cel Biol 21, 3436–3444. doi:10.1128/MCB.21.10.3436-3444.2001
Selivanov V. A., Zeak J. A., Roca J., Cascante M., Trucco M., Votyakova T. V. (2008). The Role of External and Matrix pH in Mitochondrial Reactive Oxygen Species Generation. J. Biol. Chem. 283, 29292–29300. doi:10.1074/jbc.M801019200
Shoubridge E. A., Hochachka P. W. (1980). Ethanol: Novel End Product of Vertebrate Anaerobic Metabolism. Science 209, 308–309. doi:10.1126/science.7384807
Storz J. F., McClelland G. B. (2017). Rewiring Metabolism under Oxygen Deprivation. Science 356 (6335), 248–249. doi:10.1126/science.aan1505
Sun S., Li H., Chen J., Qian Q. (2017). Lactic Acid: No Longer an Inert and End-Product of Glycolysis. Physiology 32, 453–463. doi:10.1152/physiol.00016.2017
Taylor K. R., Milone N. A., Rodriguez C. E. (2017). Four Cases of Spontaneous Neoplasia in the Naked Mole-Rat (Heterocephalus glaber), A Putative Cancer-Resistant Species. Gerona 72, 38–43. doi:10.1093/gerona/glw047
Tian X., Azpurua J., Hine C., Vaidya A., Myakishev-Rempel M., Ablaeva J., et al. (2013). High-molecular-mass Hyaluronan Mediates the Cancer Resistance of the Naked Mole Rat. Nature 499, 346–349. doi:10.1038/nature12234
Umminger B. L. (1975). Body Size and Whole Blood Sugar Concentrations in Mammals. Comp. Biochem. Physiol. A: Physiol. 52 (3), 455–458. doi:10.1016/s0300-9629(75)80065-x
Vander Heiden M. G., Cantley L. C., Thompson C. B. (2009). Understanding the Warburg Effect: the Metabolic Requirements of Cell Proliferation. Science 324 (5930), 1029–1033. doi:10.1126/science.1160809
Wang N., Jiang X., Zhang S., Zhu A., Yuan Y., Xu H., et al. (2021). Structural Basis of Human Monocarboxylate Transporter 1 Inhibition by Anti-cancer Drug Candidates. Cell 184 (2), 370–383. doi:10.1016/j.cell.2020.11.043
Warburg O. (1956). On the Origin of Cancer Cells. Science 123, 309–314. doi:10.1126/science.123.3191.309
Warburg O., Wind F., Negelein E. (1927). The Metabolism of Tumors in the Body. J. Gen. Physiol. 8, 519–530. doi:10.1085/jgp.8.6.519
Keywords: metabolism, Warburg effect, naked mole-rat, cancer metabolism, metabolic fuel switching, hypoxic metabolic response, hypoxic ventilatory response (HVR), thermoregulation
Citation: Freire Jorge P, Goodwin ML, Renes MH, Nijsten MW and Pamenter M (2022) Low Cancer Incidence in Naked Mole-Rats May Be Related to Their Inability to Express the Warburg Effect. Front. Physiol. 13:859820. doi: 10.3389/fphys.2022.859820
Received: 21 January 2022; Accepted: 11 April 2022;
Published: 04 May 2022.
Edited by:
Fernanda Marques da Cunha, Universidade Federal de São Paulo, BrazilReviewed by:
Giselle Zenker Justo, Universidade Federal de Sao Paulo, BrazilCopyright © 2022 Freire Jorge, Goodwin, Renes, Nijsten and Pamenter. This is an open-access article distributed under the terms of the Creative Commons Attribution License (CC BY). The use, distribution or reproduction in other forums is permitted, provided the original author(s) and the copyright owner(s) are credited and that the original publication in this journal is cited, in accordance with accepted academic practice. No use, distribution or reproduction is permitted which does not comply with these terms.
*Correspondence: Pedro Freire Jorge, cGVkcm9qZmpvcmdlQGdtYWlsLmNvbQ==
Disclaimer: All claims expressed in this article are solely those of the authors and do not necessarily represent those of their affiliated organizations, or those of the publisher, the editors and the reviewers. Any product that may be evaluated in this article or claim that may be made by its manufacturer is not guaranteed or endorsed by the publisher.
Research integrity at Frontiers
Learn more about the work of our research integrity team to safeguard the quality of each article we publish.