- 1Swire Institute of Marine Science, School of Biological Sciences, The University of Hong Kong, Hong Kong, Hong Kong SAR, China
- 2MARE—Marine and Environmental Sciences Centre, Laboratório Marítimo da Guia, Faculdade de Ciências, Universidade de Lisboa, Cascais, Portugal
- 3School of Biological Sciences, The University of Queensland, St. Lucia, QLD, Australia
Cleaning symbioses are key mutualistic interactions where cleaners remove ectoparasites and tissues from client fishes. Such interactions elicit beneficial effects on clients’ ecophysiology, with cascading effects on fish diversity and abundance. Ocean acidification (OA), resulting from increasing CO2 concentrations, can affect the behavior of cleaner fishes making them less motivated to inspect their clients. This is especially important as gnathiid fish ectoparasites are tolerant to ocean acidification. Here, we investigated how access to cleaning services, performed by the cleaner wrasse Labroides dimidiatus, affect individual client’s (damselfish, Pomacentrus amboinensis) aerobic metabolism in response to both experimental parasite infection and OA. Access to cleaning services was modulated using a long-term removal experiment where cleaner wrasses were consistently removed from patch reefs around Lizard Island (Australia) for 17 years or left undisturbed. Only damselfish with access to cleaning stations had a negative metabolic response to parasite infection (maximum metabolic rate—ṀO2Max; and both factorial and absolute aerobic scope). Moreover, after an acclimation period of 10 days to high CO2 (∼1,000 µatm CO2), the fish showed a decrease in factorial aerobic scope, being the lowest in fish without the access to cleaners. We propose that stronger positive selection for parasite tolerance might be present in reef fishes without the access to cleaners, but this might come at a cost, as readiness to deal with parasites can impact their response to other stressors, such as OA.
Introduction
Parasite infection can adversely impact fish. When a parasite feeds on host tissues and resources, it alters the host physiological states, compromising activities that require energy expenditure (Sheldon and Verhulst, 1996; Lochmiller and Deerenberg, 2000). Specifically, ectoparasites can impact the hosts’ energy budgets by affecting the metabolism (inducing higher resting metabolic rate), growth, and reproduction (Fenton and Rands, 2006; Jones and Grutter, 2008; Grutter et al., 2011). On coral reefs, dedicated cleaner fishes provide cleaning services by eating ectoparasites off the bodies of other “client” fishes (Grutter, 1999; Vaughan et al., 2017). The cleaner wrasse, Labroides dimidiatus, is the most ubiquitous species of cleaner in the Indo-Pacific region. This cleaner engages in more than 2,200 interactions with clients, eating more than 1,100 ectoparasites a day (Grutter, 1996). Analysis of cleaners’ stomach contents indicates that these cleaners mostly eat body-fluid feeding gnathiid isopod ectoparasites, removed during their cleaning interactions with clients (Grutter, 1996; Grutter, 1997). In addition to their effect on host metabolism, gnathiids can lower the blood volume of their hosts (up to 85% from young damselfish, Pomacentrus amboinensis) (Grutter et al., 2011), transmit blood-borne parasites (Hayes et al., 2011a), and in large numbers, can even kill the adult fish (Hayes et al., 2011b). Since cleaners can consume most of the daily emerged gnathiids on a reef per day, access to cleaning services represents a substantial advantage to clients, as cleaners can directly remove the parasites from client surfaces and control gnathiid populations by reducing the parasite infection rates (Grutter, 2008; Grutter et al., 2018). Therefore, it is no surprise that the presence of L. dimidiatus is associated with the increased client condition, growth and body size, and influences fish abundance, biodiversity, and juvenile recruitment (Bshary, 2003; Grutter et al., 2003; Clague et al., 2011; Ros et al., 2011; Waldie et al., 2011; Sun et al., 2015; Wagner et al., 2015).
Reef fishes can be vulnerable to ocean acidification (OA) caused by a decrease in seawater pH by the absorption of anthropogenic CO2 emissions. High CO2, and thus the associated lower pH, has been documented to impact behavior traits such as activity, homing, anxiety, learning, lateralization, and olfactory and auditory systems (reviewed by Clements and Hunt, 2015). Recent studies have also documented low or no effect of OA on fish behavior (Sundin et al., 2017; Raby et al., 2018; Clark et al., 2020), suggesting the species-specific variability in fish behavioral responses to OA. In addition, cleaning mutualisms are not immune to OA. After prolonged exposure to OA, interactions between the cleaners and clients are reduced and the cleaners lose their motivation to engage in cleaning interactions along with a disruption in interaction quality through possible neurobiological disruption (Paula et al., 2019a) and interaction-derived cognitive performance (Paula et al., 2019b). Within this context, the client fishes’ ability to reduce ectoparasite loads diminishes due to lower cleaner motivation, along with the resulting potential increase in the infection rates onto fish (Grutter et al., 2018), as reduced cleaning activity also may not control local gnathiid population densities (Sikkel et al., 2019). However, it is also possible that after the long-term lack of access to cleaning services, surviving client fishes might be physiologically adapted to deal with ectoparasite infections. Moreover, a previous study showed that gnathiid survival is not affected by laboratory acclimation to high CO2, suggesting that gnathiids can tolerate ocean acidification (Paula et al., 2020).
High CO2 has also been documented to impact the reef fish physiological traits such as escape performance (Allan et al., 2013; Allan et al., 2014), metabolism (Wisenden, 2012; Couturier et al., 2013; Rummer et al., 2013; McMahon et al., 2020), and reproduction (Miller et al., 2013; Welch and Munday, 2016). A meta-analysis of fish metabolic responses to OA revealed that there is an increase on resting metabolic rate (ṀO2Rest), but there seems to be no overall effect of OA on fish absolute aerobic scope (ṀO2Max - ṀO2Rest) (Hannan and Rummer, 2018). Metabolic rate can be measured during resting (resting metabolic rate—ṀO2Rest), giving us information on the basal metabolic costs of an organism to perform life-sustaining functions. During maximum exercise, metabolic rate measures the maximum rate of aerobic metabolism (maximum metabolic rate—ṀO2Max) an animal can reach, and it is related to the maximum rate of oxygen transport, extraction, and use (Hannan and Rummer, 2018; Hasley et al., 2018). Aerobic scope represents the animal’s capacity to increase its aerobic metabolic rate above the maintenance levels (i.e., the difference between ṀO2Max and ṀO2Rest), being used as proxy for physiological fitness (Clark et al., 2013). Aerobic scope can be presented in absolute terms (i.e., ṀO2Max - ṀO2Rest) or as factorial aerobic scope (i.e., ṀO2Max/ṀO2Rest). These measures indicate an absolute or proportional increase, respectively, in the oxygen consumption rates that an animal can reach above the baseline levels (Clark et al., 2013). Measuring and presenting these different metabolic variables in the context of environmental stress research is crucial to understand how a given stressor (such as OA) can add a cost to the basal life-sustaining functions (ṀO2Rest), impact oxygen transport (ṀO2Max), and affect physiological fitness. So far, only four studies have reported a decreased in aerobic scope (along with lower ṀO2Max; Methling et al., 2013; Tirsgaard et al., 2015; or no differences in ṀO2Max and ṀO2Rest; Hamilton et al., 2017) and of those only one used CO2 levels predicted to occur at the end of the century (along with higher ṀO2Rest; Munday et al., 2009). In contrast, 19 studies have reported no effects on aerobic measures of performance and in three studies performance increased over 12 different fish species (reviewed by Hannan and Rummer, 2018). One explanation for these conflicting results is that there is a species-specific response to high CO2.
Since the cleaners’ motivation to interact with clients drops with OA (Paula et al., 2019a), one can conceive a future scenario where client fishes must deal with the physiological impacts of OA together with the stress induced by ectoparasitism, without having access to a way to remove parasites (i.e., cleaning interactions). To understand how access to cleaning interactions can modulate fish physiological responses to parasitic infection and OA, we used the small resident ambon damselfish (Pomacentrus amboinensis) collected from a long-term cleaner removal experiment on Lizard Island (Australia) maintained since 2000 (Waldie et al., 2011; Grutter et al., 2019). In this long-term experiment, 18 isolated patch reefs have been manipulated so that half had all juvenile and adult L. dimidiatus removed around every 3 months over 17 years, whereas the other reefs were left unmanipulated as controls. This created habitats where fish either have no access to cleaning interactions and a potentially higher exposure to ectoparasites, or access to cleaning with a potentially lower parasite exposure (Grutter et al., 2018). Since this long-term experiment has been running longer than the lifespan of this damselfish [approximately 6.5 years (McCormick, 2016)], individuals from “cleaner-absent” reefs had no access to cleaners and possibly had higher ectoparasite exposure for their lifetime. Importantly, since each reef is separated by at least 5 m of sand, these territorial resident damselfish do not cross between the reefs once juveniles have settled on the reef (Binning et al., 2018). We combined this long-term experiment with a 10-days laboratory exposure to high CO2 and examined whether living on reefs with or without the access to cleaner wrasses (L. dimidiatus) influences clients’ (P. amboinensis) metabolic responses under OA (the predicted conditions of ocean acidification for 2100, ∼1,000 μatm, RCP 8.5 scenario; Bindoff et al., 2019). Moreover, we also performed an experimental parasite infection assay using the cultured gnathiid isopods. This allowed us to test the effects of CO2 treatment, cleaner presence, and parasite infection on client fish aerobic metabolism, namely resting and maximum metabolic rate, and aerobic scope (both factorial and absolute aerobic scope are presented and analyzed, providing transparency as suggested in Halsey et al., 2018).
Material and Methods
Cleaner Fish Removal Experiment
Our study was conducted using fish from eight small spatially isolated patch reefs in shallow (3–7 m deep) areas in Lagoon and Casuarina Beach of Lizard Island (14°40S, 145°28′E), Great Barrier Reef, Australia, during the austral winter (August-September 2017). These reefs are part of a larger ongoing long-term cleaner fish removal experiment initially involving 18 reefs in total (12 at Lagoon and 6 at Casuarina Beach, then reduced to 16 reefs, see Waldie et al., 2011; Sikkel et al., 2019). At the time of the study, the reefs were allocated randomly into seven removal (“cleaner-absent,” n = 7, average area ± SE: 131 ± 34 m2) and nine control reefs (“cleaner-present,” n = 9, average area ± SE: 134 ± 17 m2). Since September 2000, after the removal of the initial cleaners, these reefs were inspected at approximately 3-month intervals for the presence of cleaner wrasses. In the cleaner-absent reefs, any new cleaner recruit was removed by hand- and barrier nets, whereas on the cleaner-present reefs they were counted (see Supplementary Figure S1 for numbers of cleaners encountered from 2000 to 2017). Following the 2016/2017 mass bleaching event at Lizard Island, along with the northern portion of the Great Barrier Reef (Hughes et al., 2017), four of the nine control cleaner-present reefs lost their cleaners (Sikkel et al., 2019). Here, we only used fish from the four cleaner-present reefs that had not lost their cleaners during the bleaching event.
Fish Capture and CO2 Exposure
To investigate whether the presence of cleaner wrasses affected the clients’ physiological responses to ocean acidification and parasite infection, we collected 96 individuals (P. amboinensis; 12 per reef; SL = 4.63 ± 0.12 cm, weight = 4.78 ± 0.34 g, and mean ± SE), 48 from the cleaner-present reefs (reefs 9, 12, 15, and 16, Figure 1) and other 48 from four randomly selected cleaner-absent reefs (reefs 2, 6, 17, and 18, Figure 1). Among the initial 96 fish captured, three died during the acclimation due to unknown reasons (one in high CO2/cleaner-present; one in high CO2/cleaner-absent; and one in control CO2/cleaner-present). After 4 days of acclimation to the experimental tanks at Lizard Island Research Station, we exposed them for 10 days to one of the two treatments, control water (present-day CO2 ∼ 350 µatm CO2), or the predicted conditions of ocean acidification for 2100, following the Representative Concentration Pathway (RCP) 8.5 scenario from the Intergovernmental Panel on Climate Change (IPCC; high CO2 ∼ 1,000 µatm CO2; Bindoff et al., 2019) in a crossed factorial design (n = 24—control/cleaner-absent; n = 23—control/cleaner-present n = 23—high CO2/cleaner-absent; n = 23—high CO2/cleaner-present; see Figure 1). Initial exposure day among fish varied randomly over 12 days, as the aerobic metabolism analysis (done at day 11, after 10 complete days of exposure) took 12 days to complete. This ensured that all fish had the same exposure duration to CO2. This totaled 23 days from the exposure of the first batch of four fish to the metabolism analysis of the last batch of four fish. The exposure time is in line with several other ocean acidification studies performed with the same species (Munday et al., 2009; Heuer et al., 2016; Munday et al., 2016). The fish were maintained in one of the eight flow-through systems (4 fish per CO2 treatment) where natural seawater was pumped from the sea into three 10,000 L seawater storage tanks. From these, the seawater was supplied to a mixing tank (flux: 270 L h −1; volume: 130 L) and from these to each experimental tank (flux 12 L h −1; volume: 10 L). The fish were provided with a polyvinyl chloride (PVC) tube for refuge (4 cm diameter, 10 cm length). pCO2 control was performed indirectly by adjusting the pH to a nominal value defined by CO2SYS software using measured salinity, total alkalinity, temperature, and desired pCO2 as input variables. Levels of pHNBS were automatically adjusted (Profilux 3.1N, GHL, Germany), downregulated by direct injection of CO2 gas (BOC, Australia), and upregulated through aeration with atmospheric air in mixing tanks. Seawater temperature was maintained similar to the current reef temperature due to the flow of recently captured seawater. We used handheld equipment to complement the automatic systems to monitor and record seawater parameters, measuring seawater temperature (Hanna CheckTemp 1C, Portugal), salinity (V2 refractometer, TMC, Portugal), and pHtotal (VWR pHenomenal pH 1100H, connected to a glass electrode calibrated with TRIS-HCl and 2-aminopyridine-HCl buffers). Seawater carbonate system speciation was calculated from the total alkalinity (titration) and pH measurements. Bicarbonate and pCO2 values were calculated using CO2SYS software. Seawater parameters are summarized in ESM (Supplementary Table S1).
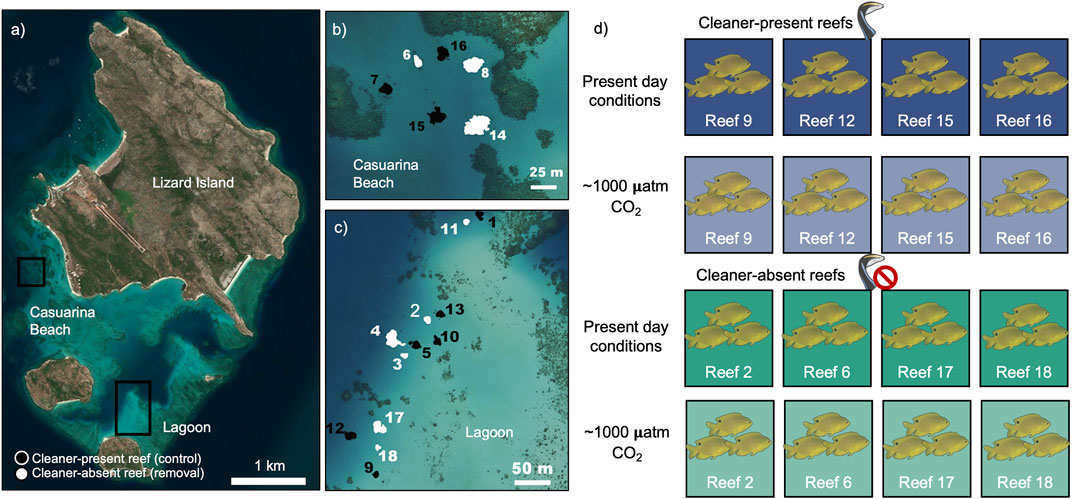
FIGURE 1. Experimental design used in this study. On the maps (A–C), white represent reefs where cleaner fish Labroides dimidiatus were removed throughout 17 years, black represent control reefs. (D) Pomacentrus amboinensis were collected from reefs 9, 12, 15, and 16 (control reefs) and reefs 2, 6, 17, and 18 (removal reefs) and acclimated to one of two conditions for 20 days: Control (∼350 µatm pCO2) or ocean acidification (High CO2, ∼1,000 µatm pCO2). Map adapted from Grutter et al. (2019).
Experimental Parasite Infection
We used controlled parasite infections. Gnathiids, namely Gnathia aureamaculosa, were obtained from a parasite culture maintained at Lizard Island Research Station since 2001 (Hutson et al., 2018; Grutter et al., 2020). Gnathiids were collected by moving a black tray around the culture tank to simulate the movement of a host, which briefly attracts the unfed (determined by presence of an unengorged gut) gnathiids to the tray and be easily captured with a pipette. After 10 days of acclimation to the CO2 treatment, individual damselfish were placed in individual containers (1 L), filled with water from the respective CO2 treatment, and randomly assigned to a parasite treatment (parasite, n = 48, or control n = 45). Six gnathiids were added to the parasite treatment containers. We counted the number of gnathiids attached to each fish at 15 min intervals for a total of 240 min (as at that time, most parasites had detached from the fish, Supplementary Figure S1). Control fish spent the same time in the treatment containers without parasites. The 10-days acclimation ensured that any recent effects of gnathiid parasites were due to this experimental parasite infection and not gnathiids obtained in the wild, as gnathiids only feed on hosts for a few minutes to hours before detaching (Grutter 2003). Following this, the fish were transferred to a hand net, which resulted in any remaining cultured gnathiid parasite being removed from their body as they readily detach when disturbed (Grutter, 1995). Then fish were subjected to an aerobic metabolism analysis described as follows.
Aerobic Metabolism
Oxygen uptake rates (ṀO2), corrected for fish mass, were determined by using an intermittent flow respirometry, according to the established respirometry methods (Clark et al., 2013; Roche et al., 2013; Rummer et al., 2016). The aerobic metabolism measures were performed between 7:00 and 19:00 h with two runs a day, where four fish were measured simultaneously in each run. The overall aerobic metabolism analysis took 12 days to complete (4 fish per run, two runs a day, eight fish per day). The fish were starved for 24 h prior testing to ensure a postabsorptive metabolic state (Niimi and Beamish, 1974). Maximum metabolic rate (ṀO2Max) was measured by chasing the fish for 3 min in a circular container (30 cm diameter and 10 cm height) with a hand net, followed by air exposure (1 min) involving holding the fish in a hand net. Immediately following air exposure, the fish were gently placed into individual respirometry chambers (volume: 668 ml, including tubing), which were submerged in a water bath (volume: 100 L) containing the respective treatment water. The water bath had a continuous flow of treatment water to ensure that CO2 and temperature were kept constant. Water mixing within each chamber was achieved with an external DC brushless pump that moved water through the chamber and around an external circuit of gas-tight tubing (flowrate: 100 ml min−1). Automated flush pumps supplied the chambers with oxygenated clean water for 3 min every 8 min, so that the O2 levels within the chambers never fell below 80% air saturation. The flush pumps were controlled via Profilux 3.1N using a programmed timing sequence. In each chamber, every 2 s, the temperature-compensated oxygen concentration (mg L−1) of the water was recorded using the flow-through cells with an integrated optical oxygen sensor (OXFTC2, PyroScience, Germany), calibrated before each run. The flow-through cells were linked to the external circuit of gas-tight tubing and connected to a Firesting Optical Oxygen Meter (PyroScience, Germany) with fiber-optic cables. ṀO2Max was measured before the first flush, for 5 min after the fish was placed in the chambers. The fish remained in chambers to recover to their resting oxygen uptake rates (resting metabolic rate, ṀO2Rest) over 6 h. ṀO2Rest is here defined as the average rate of metabolism, when an animal is in a postabsorptive state as mostly inactive (Killen et al., 2014; Metcalfe et al., 2016). ṀO2Rest was calculated using the average of the three lowest ṀO2 values obtained within 6 h (these values were normally obtained towards the end of the reading, and at least after 3 h of reading). This methodology was previously demonstrated valid for this species as these fish can recover from exercise (after chasing) within 90 min, with their oxygen uptake remaining stable for 8 h (Killen et al., 2014). Moreover, while confined in a respirometer, following recovery, the damselfish remained mostly inactive (Killen et al., 2014). Before and after each fish ṀO2 measurement, the full setup was cleaned with fresh water and the chambers walls wiped with a tissue soaked in 70% ethanol, followed by refilling with seawater; a 1 h of full respirometry cycles was then run with empty chambers to measure any background ṀO2 by bacteria and other microorganisms. Background respiration was assumed to increase linearly (from start to end of each respirometry trial) and was subtracted from each measure of ṀO2. The respirometry data was analyzed with the R software package “respR” (Harianto et al., 2019). The oxygen data was corrected for fish mass and was analyzed as mg O2·gfish−1·hour−1. Individual’s capacity to transport oxygen, relative to their baseline rate of oxygen uptake, was determined using both absolute aerobic scope (AAS) and factorial aerobic scope (FAS), which are calculated by subtracting ṀO2Rest from ṀO2Max or by the ratio of ṀO2Max to ṀO2Rest, respectively. AAS is more widely used (and stable) for when the variability in ṀO2Rest is the major concern (e.g., when animals are restlessness) and FAS is more widely used (and stable), when ṀO2Max is the main concern (e.g., when animals are averse to be active in a laboratory setting) (Halsey et al., 2018). According to the best practices suggested by Halsey et al. (2018), both AAS and FAS are presented and analyzed. Both AAS and FAS used the mass corrected data.
Statistical Analysis
All measurements were taken from distinct samples (i.e., each fish). We evaluated differences in the mean number of gnathiids attached during the experimental parasite infection, using a GLMM with Gaussian error distribution, CO2, and cleaner present as categorical fixed factors and tank and reef ID as random factors (ESM Supplementary Table S2). We analyzed the differences in size (standard length, SL) and weight using the generalized linear mixed-effect models (GLMM) with Gaussian distribution, CO2 treatment, cleaner presence, and parasite treatment as categorical fixed factors, and tank and reef ID as random. For the metabolic data (ṀO2Max, ṀO2Rest, AAS and FAS), we used a gamma error distribution as the metabolic data is right skewed, following Bolker (2008). These models used CO2 treatment, cleaner presence, and parasite treatment as categorical fixed factors, and tank replicate, reef ID and measurement time of day (morning vs. afternoon) as random factors (ESM Supplementary Tables S3,S4). The full models, with all possible interactions, were tested using the function “glmmTMB” from the package “glmmTMB” (Brooks et al., 2017) and the function “Anova” from the package “car” (Fox and Weisberg, 2011) in R, version 3.4.3 (R Core Team, 2017). Post-hoc multiple comparisons were performed using the package “emmeans” and applying Tukey corrections via the Monte Carlo approximation of the multivariate distribution (Lenth, 2020). Model assumptions and performance were validated using the package “performance” (Lüdecke et al., 2021). Data exploration used the HighstatLibV10 R library from Highland Statistics (Zuur et al., 2009; Zuur et al., 2010).
Results
The size (SL) of damselfish did not differ with treatment (parasite: χ2 = 0.855, d.f. = 1, p = 0.355; cleaner: χ2 = 0.015, d.f. = 1, p = 0.902; and CO2: χ2 = 0.026, d.f. = 1, p = 0.873) nor any interactions of treatments (parasite × CO2, × cleaner: χ2 = 0.034, d.f. = 1, p = 0.853; parasite × CO2: χ2 = 0.059, d.f. = 1, p = 0.808; parasite × cleaner: χ2 = 0.67, d.f. = 1, p = 0.413; and CO2 × cleaner: χ2 = 0.512, d.f. = 1, p = 0.474). Likewise, fish weight did not differ with treatment (parasite: χ2 = 0.832, d.f. = 1, p = 0.362; cleaner: χ2 = 0.031, d.f. = 1, p = 0.861; CO2: and χ2 = 0.603, d.f. = 1, p = 0.438) nor any interaction of treatments (parasite × CO2, × cleaner: χ2 = 1.767, d.f. = 1, p = 0.184; parasite × CO2: χ2 = 0.1029, d.f. = 1, p = 0.31; parasite × cleaner: χ2 = 1.241, d.f. = 1, p = 0.265; and CO2 × cleaner: χ2 = 0.569, d.f. = 1, p = 0.451).
During the experimental parasite infection, the damselfish were infected with an average of 1.37 ± 0.08 gnathiids (mean ± SE). This number is within the range they are infected within the wild over 12 h (Grutter et al., 2018). The average number of attached gnathiids did not differ with CO2 or cleaner treatment or the interaction between CO2 and cleaner treatment (CO2: χ2 = 0.067, d.f. = 1, p = 0.796; cleaner: χ2 = 0.031, d.f. = 1, p = 0.861; and cleaner × CO2: χ2 = 0.971, d.f. = 1, p = 0.324; Supplementary Table S2, Figure 2).
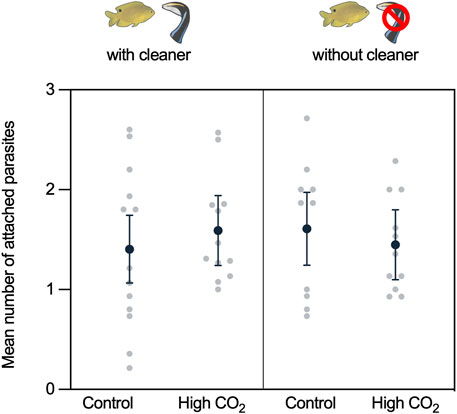
FIGURE 2. Mean number of attached gnathiids during the experimental parasite infection. Back-transformed predicted means ±95% confidence intervals (C.I.) from the model and raw data values are presented.
Resting metabolism (ṀO2Rest) of fish was not affected by CO2, cleaner, or parasite treatment (CO2: χ2 = 2.289, d.f. = 1, p = 0.130; cleaner: χ2 = 2.427, d.f. = 1, p = 0.119; and parasite: χ2 = 0.484, d.f. = 1, p = 0.487; Supplementary Table S3, Figure 3A). All interactions were not significant (Supplementary Table S3).
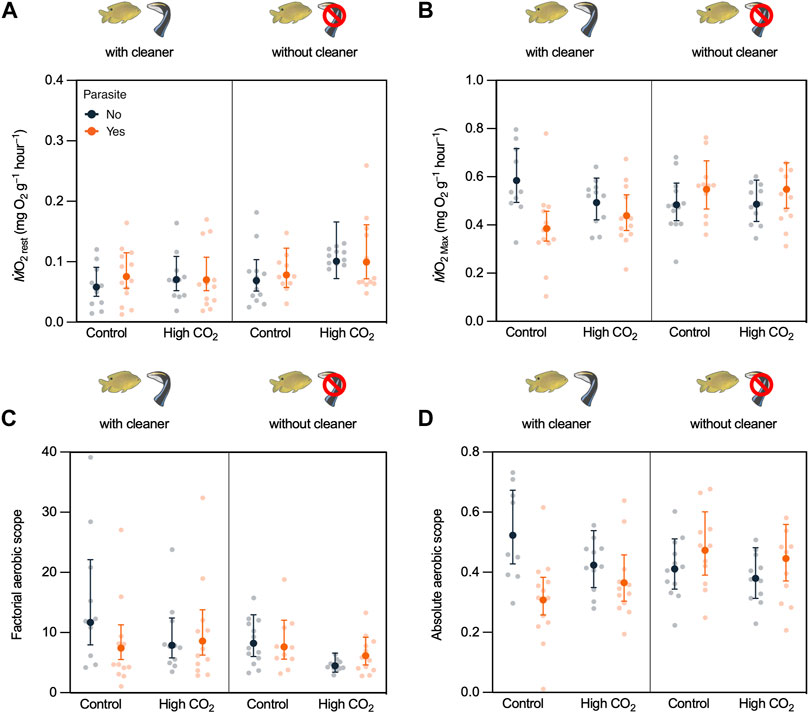
FIGURE 3. Damselfish Pomacentrus amboinensis aerobic physiology obtained through the exhaustive chase protocol according to CO2 treatment (Control vs. High CO2), cleaner wrasse removal treatment (with cleaner vs. without cleaner), and parasite infection (Yes vs. No). (A) resting metabolic rate (ṀO2Res, (B) maximum metabolic rate (ṀO2Max), (C) factorial aerobic scope (FAS), and (D) absolute aerobic scope (AAS). Back-transformed predicted means ±95% confidence intervals (C.I.) from the model and raw data values are presented.
For the maximum metabolic rate (ṀO2Max), there was a significant interaction between the cleaner presence and parasite infection (cleaner × parasite: χ2 = 11.390, d.f. = 1, p < 0.001, Supplementary Table S3, Figure 3B). ṀO2Max decreased when damselfish from the cleaner-present reefs were infected with parasites (t-ratio = -3.197; p = 0.011; Supplementary Table S4, see Table 1), but not on damselfish from the cleaner-absent reefs (t-ratio = 1.518; p = 0.432; Supplementary Table S4, see Table 1). Moreover, ṀO2Max was lower for damselfish infected with parasites from the cleaner-present reefs compared to infected damselfish from the cleaner-absent reefs (t-ratio = 3.369; p = 0.006; Supplementary Table S4, see Table 1). CO2 did not affect ṀO2Max (CO2: χ2 = 0.026, d.f. = 1, p = 0.872, Supplementary Table S3, Figure 3B, see Table 1).
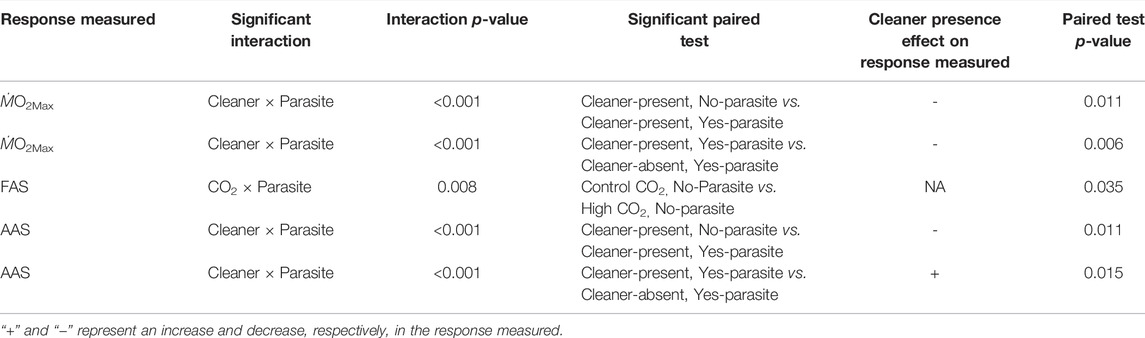
TABLE 1. Summary of results of the post-hoc multiple comparisons in relation to the effect of cleaner fish treatment (presence or absence).
Regarding the factorial aerobic scope (FAS), there was a significant interaction between CO2 and parasite infection (CO2 × parasite: χ2 = 6.9591, d.f. = 1, p = 0.008, Supplementary Table S3, Figure 3C). Post-hoc comparisons indicated that nonparasite damselfish under high CO2 had significantly lower FAS than that of nonparasite fish in control CO2 (t-ratio = -2.759; p = 0.035; Supplementary Table S4, see Table 1). Cleaner presence did not affect FAS (FAS: χ2 = 3.528, d.f. = 1, p = 0.060, Supplementary Table S3, Figure 3C, see Table 1).
Absolute aerobic scope was altered by an interaction between cleaner presence and parasite infection (cleaner × parasite: χ2 = 11.414, d.f. = 1, p < 0.001, Supplementary Tables S4, S5, Figure 3D), but high CO2 had no significant effect (CO2: χ2 = 0.580, d.f. = 1, p = 0.476, Supplementary Table S3, Figure 3D). Post-hoc comparisons revealed that parasite infection decreased AAS only in fish with access to cleaners (t-ratio = -3.191; p = 0.011; Supplementary Table S4, see Table 1) and that parasite damselfish on cleaner-absent reefs had higher AAS than parasitized damselfish from the cleaner-present reefs (t-ratio = 3.063; p = 0.015; Supplementary Table S4, see Table 1).
Discussion
Cleaner wrasses provide an essential ecological service to their clients by controlling their ectoparasite loads through direct removal of ectoparasites from their surfaces and reducing infection rates by lowering gnathiid densities (Grutter, 1996; Grutter, 1999; Grutter et al., 2018). We hypothesized that clients deprived of access to cleaning services would be physiologically adapted to deal with ectoparasite infections. In line with this hypothesis, we found that the ectoparasite infection results in a decreased maximum metabolic rate [ṀO2Max, inducing so-called limiting stress, as defined in Heuer and Grosell, 2014)] with parasitism, only in ambon damselfish from the cleaner-present reefs. As infected damselfish of cleaner-present reefs had lower ṀO2Max than infected damselfish from cleaner-absent reefs (Figure 3B), this suggests that damselfish from the cleaner-absent reefs are more adapted to tolerate ectoparasite infection. Parasite infection might be lowering ṀO2Max due to the elevated costs of activation of an immune response. In mice, activation of the immune response led to a decrease in ṀO2Max, and the selection for animals with higher ṀO2Max led to a reduction in the innate immune function (Downs et al., 2013). Even low parasite infections can have huge costs, especially for the small hosts like damselfish at settlement, as gnathiids can kill their hosts, or by consuming 85% of their blood, which can cause significant sublethal effects (Clague et al., 2011; Waldie et al., 2011). Therefore, in reefs where cleaners are absent, fish might either develop a higher tolerance to gnathiid infection (e.g. better immune response) or be exposed to strong selective pressure for a tolerance to gnathiids. Indeed, at settlement stage, gnathiid infection can increase damselfish oxygen consumption (resting metabolic rate) and decrease their critical swimming speed, making damselfish more prone to predation (Grutter et al., 2011). Previously, Grutter et al. (2011), suggested that if gnathiids affect the settlement stage damselfishes, they might ultimately affect the adult population distribution and abundance. The observed tolerance to ectoparasite infection observed in damselfishes from the cleaner-absent reef might indeed reflect this, where, due to the absence of cleaner fishes and high parasitism, only individuals that tolerate parasite infections could successfully settle and reach adulthood. Variation of tolerance to ectoparasitism in wild fish populations has been previously described in dace (Leuciscus leuciscus) (Blanchet et al., 2010). This variation could be due to an investment in limiting tissue degradation during ectoparasite feeding, such as investing in higher cell regeneration (i.e. better immune response, Råberg et al., 2009), or it could be a selection for individuals that are genetically better at dealing with infection (Klemme et al., 2020). However, the mechanisms that could provide higher parasite tolerance to damselfishes from the cleaner-absent reefs observed here remain unknown and need to be further investigated. In contrast, in reefs without cleaners, selection might favor damselfish that suppress their innate immune function, preventing a limitation on their aerobic capacity (ṀO2Max), as described in mice selected for higher ṀO2Max (Downs et al., 2013).
ṀO2Rest was not altered by parasite infection regardless of its access to cleaning or CO2. Infection with small ectoparasites in salmon also had no effects on ṀO2Rest (Hvas et al., 2017). On the other hand, studies using larger parasites, such as Anilocra spp. isopods, showed enhanced resting metabolic rate in the coral reef fish (Östlund-Nilsson et al., 2005; Binning et al., 2013). Yet, it is worth mentioning that such an increase in the resting metabolic rate was due to the destabilizing effect of the asymmetrically attached parasite rather than any physiological effect of parasitism. This indicates that parasite infection does not add a so-called loading stress (as defined in Heuer and Grosell 2014). Moreover, as found by Couturier et al., 2013, exposure to high CO2 did not affect the damselfish resting metabolic rate (ṀO2Rest), suggesting that the metabolic costs of living in a high CO2 environment, namely potentially altered acid-base balance, ion regulation, and cardiorespiratory function also do not add a loading stress for this species (Figure 3A).
When we analyzed damselfish oxygen transport capacity relative to their resting rate of oxygen uptake (i.e., aerobic scope), we observed that their response to parasite infection was dependent on access to cleaning services. Only fish from the cleaner-present reefs decreased AAS (ṀO2Max - ṀO2Rest) with parasite exposure. This suggests that fish who lack access to cleaning services have a higher physiological tolerance to parasite infection. Yet, independent of cleaner presence and only in fish without parasite infection, FAS (ṀO2Max/ṀO2Rest) decreased with CO2 (but not AAS). Here, fish under control conditions without parasite infection had the highest average FAS and CO2 significantly decreased damselfish FAS. This indicates that the combination of parasite stress and CO2 does not have an additive effect. However, by comparing FAS with both ṀO2Max and ṀO2Rest, we observed that this decrease in FAS results from different physiological processes for both the stressors. On one hand, the decrease in FAS under high CO2 could be driven by a slight (nonsignificant) increase in ṀO2Rest, suggesting that a slight increase in resting metabolic costs (i.e., increase in loading stress) under high CO2 is what is causing a lower damselfish aerobic capacity (FAS). On the other hand, the decrease in FAS (and AAS) due to parasite infection is driven by a decrease in ṀO2Max, possibly due to an increase in the damselfish immune response. The difference between FAS and AAS is related to their different sensitivities for each metabolic parameter measured. While both FAS and AAS are used to represent an animal’s capacity to increase its aerobic metabolic rate above the maintenance levels, AAS is more widely used for when the variability in ṀO2Rest is the major concern, and FAS is more widely used when ṀO2Max is the main concern (Halsey et al., 2018). Following the best practices used in the field, we present both measures. Yet, we must interpret this result with caution as the present exposure to high CO2 had no evolutionary component and was of a relatively short duration (Sunday et al., 2014). Therefore, we cannot exclude the possibility that these fishes could be able to adapt to this increase in CO2.
Experiments on species’ resilience to climate change stressors should be analyzed in an evolutionary perspective. This study starts with the premise that cleaning interactions could be altered with OA, since Paula and collaborators found significant changes in cleaning motivation after a 45 day acclimation period to high CO2 (Paula et al., 2019a). Additionally, in a follow-up study, it was observed that: i) cleaner wrasse populations have a standing variation to deal with mild increases of CO2 (i.e., 750 µatm pCO2) from a behavioral perspective and ii) only at higher acidification levels (980 µatm pCO2) was their cognitive performance severely affected (Paula et al., 2019b). However, one should note that to fully address the climate change problem, we should consider a multistressor perspective, e.g., including a temperature increase (Gunderson et al., 2016). Within this context, it is worth noting that both the cleaner and clients are particularly sensitive to extreme heatwave events (e.g., 2016 marine heatwave and associated coral bleaching), as their abundance can diminish drastically after such extreme events (Triki et al., 2018; Triki and Bshary, 2019). Gnathiid abundance also decreased at our study site during the same extreme event (2016), and to a following one in 2017. But while it quickly recovered after the first extreme event (possibly due to lower coral cover, see Paula et al., 2021), it did not do so in 2017 and remained low postbleaching (in 2018) (Sikkel et al., 2019). This overall decrease in gnathiids may have been caused by an interaction between the short-term negative impacts of thermal stress on gnathiids, as shown in laboratory studies (Shodipo et al., 2020), and a decline in host availability, causing gnathiid abundance to drop (Sikkel et al., 2019; Triki and Bshary, 2019). Since heatwave intensity and frequency is increasing (Oliver et al., 2018), client fish (e.g., P. amboinensis) population attempts of adaptation to either ocean acidification or parasite infection can quickly be erased following such extreme events, if, for example, individuals that develop tolerance to either parasite infection or OA die during such extreme events. Additionally, this possible adaptation to parasite infection (e.g., putative higher investment in higher cell regeneration) may come at a cost, as damselfish from the cleaner-absent reefs are smaller, and thus probably less fecund, since fecundity and size are correlated in these fish (Birkeland and Dayton 2005; Clague et al., 2011).
In conclusion, we demonstrated, for the first time, that cooperative cleaning interactions, fundamental ecological components of coral reef fish ecosystems, can influence the physiological fitness of a client fish species. Namely, we found that the lack of access to cleaning services leads to more physiological tolerance to parasite infection. However, independent of access to cleaners, high CO2 lowered fish fitness, although this was not exacerbated by parasite infection. This study adds a layer of complexity to the climate change-related studies, namely the importance of species interactions, that should be included to fully understand the biological impacts of climate change in the ocean of tomorrow.
Data Availability Statement
The datasets generated and analysed during this study are available in the Figshare repository, https://doi.org/10.6084/m9.figshare.13656647.
Ethics Statement
Experimental procedures were approved by the University of Queensland Animal Ethics Committee. Faculdade de Ciências da Universidade de Lisboa animal welfare body (ORBEA) and Direção-Geral de Alimentação e Veterinaria (DGAV) also approved these experiments in accordance with the requirements imposed by the Directive 2010/63/EU on the protection of animals used for scientific purposes.
Author Contributions
JP, AG, and RR designed the study. JP and TR performed the experiment and collected the data. JP, AG, and RR wrote the manuscript. All authors discussed the results and commented on the manuscript at all stages.
Funding
Portuguese national funds funded this study through FCT—Fundação para a Ciência e Tecnologia, I.P., within the projects PTDC/BIA-BMA/0080/2021 (ChangingMoods), PTDC/MAR-EST/5880/2014 (MUTUALCHANGE), the strategic project UID/MAR/04292/2020 and a PhD scholarship (SFRH/BD/111153/2015). Lizard Island Reef Research Foundation also supported this study with a Lizard Island Doctoral Fellowship to JP. This publication was funded by the European Union’s Horizon 2020 Research and Innovation Programme under grant agreement N810139: Project Portugal Twinning for Innovation and Excellence in Marine Science and Earth Observation—PORTWIMS.
Conflict of Interest
The authors declare that the research was conducted in the absence of any commercial or financial relationships that could be construed as a potential conflict of interest.
Publisher’s Note
All claims expressed in this article are solely those of the authors and do not necessarily represent those of their affiliated organizations, or those of the publisher, the editors, and the reviewers. Any product that may be evaluated in this article, or claim that may be made by its manufacturer, is not guaranteed or endorsed by the publisher.
Acknowledgments
We acknowledge Dr. Januar Harianto for his help implementing respR analysis and Lizard Island Research Station, namely the directors, Dr. Anne Hoggett AM and Dr. Lyle Vail AM, and staff, Marianne Dwyer and John Williamson, for their friendship and help, and many colleagues and volunteers that assisted in maintaining the parasite culture and the long-term cleaner removal experiment over the years. We acknowledge the traditional owners of Jiigurru (Lizard Island), the Dingaal people, on whose land the Lizard Island Research Station is situated.
Supplementary Material
The Supplementary Material for this article can be found online at: https://www.frontiersin.org/articles/10.3389/fphys.2022.859556/full#supplementary-material
References
Allan B. J. M., Domenici P., McCormick M. I., Watson S.-A., Munday P. L. (2013). Elevated CO2 Affects Predator-Prey Interactions through Altered Performance. PLoS One 8, e58520. doi:10.1371/journal.pone.0058520
Allan B. J. M., Miller G. M., Mccormick M. I., Domenici P., Munday P. L. (2014). Parental Effects Improve Escape Performance of Juvenile Reef Fish in a high-CO2world. Proc. R. Soc. B 281, 20132179–20132187. doi:10.1098/rspb.2013.2179
Bindoff N. L., Cheung W. W. L., Kairo J. G., Aristegui J., Guinder V. A., Hallberg R., et al. (2019). “Changing Ocean, Marine Ecosystems, and Dependent Communities,” in IPCC Special Report on the Ocean and Cryosphere in a Changing Climate. Editors H.-O. Pörtner, D. C. Roberts, V. Masson-Delmotte, P. Zhai, M. Tignor, E. Poloczanskaet al. (Cambridge, United Kingdom and New York, NY: Cambridge University Press), 447–587. doi:10.1017/9781009157964.007
Binning S. A., Roche D. G., Grutter A. S., Colosio S., Sun D., Miest J., et al. (2018). Cleaner Wrasse Indirectly Affect the Cognitive Performance of a Damselfish through Ectoparasite Removal. Proc. R. Soc. B 285, 20172447. doi:10.1098/rspb.2017.2447
Binning S. A., Roche D. G., Layton C. (2013). Ectoparasites Increase Swimming Costs in a Coral Reef Fish. Biol. Lett. 9, 20120927. doi:10.1098/rsbl.2012.0927
Birkeland C., Dayton P. (2005). The Importance in Fishery Management of Leaving the Big Ones. Trends Ecol. Evol. 20, 356–358. doi:10.1016/j.tree.2005.03.015
Blanchet S., Rey O., Loot G. (2010). Evidence for Host Variation in Parasite Tolerance in a Wild Fish Population. Evol. Ecol. 24, 1129–1139. doi:10.1007/s10682-010-9353-x
Bolker B. (2008). Probability and Stochastic Distributions for Ecological Modelling” in Ecological Models and Data in R. Editor B. Bolker (Princeton: Princeton University Press). doi:10.1515/9781400840908-005
Brooks M. E., Kristensen K., Benthem K. J. v., Magnusson A., Berg C. W., Nielsen A., et al. (2017). glmmTMB Balances Speed and Flexibility Among Packages for Zero-Inflated Generalized Linear Mixed Modeling. R J. 9, 378–400. doi:10.32614/rj-2017-066
Bshary R. (2003). The Cleaner Wrasse, Labroides dimidiatus, Is a Key Organism for Reef Fish Diversity at Ras Mohammed National Park, Egypt. J. Anim. Ecol. 72, 169–176. doi:10.1046/j.1365-2656.2003.00683.x
Clague G. E., Cheney K. L., Goldizen A. W., McCormick M. I., Waldie P. A., Grutter A. S. (2011). Long-term Cleaner Fish Presence Affects Growth of a Coral Reef Fish. Biol. Lett. 7, 863–865. doi:10.1098/rsbl.2011.0458
Clark T. D., Raby G. D., Roche D. G., Binning S. A., Speers-Roesch B., Jutfelt F., et al. (2020). Ocean Acidification Does Not Impair the Behaviour of Coral Reef Fishes. Nature 577, 370–375. doi:10.1038/s41586-019-1903-y
Clark T. D., Sandblom E., Jutfelt F. (2013). Aerobic Scope Measurements of Fishes in an Era of Climate Change: Respirometry, Relevance and Recommendations. J. Exp. Biol. 216, 2771–2782. doi:10.1242/jeb.084251
Clements J., Hunt H. (2015). Marine Animal Behaviour in a High CO2 Ocean. Mar. Ecol. Prog. Ser. 536, 259–279. doi:10.3354/meps11426
Couturier C. S., Stecyk J. A. W., Rummer J. L., Munday P. L., Nilsson G. E. (2013). Species-specific Effects of Near-Future CO2 on the Respiratory Performance of Two Tropical Prey Fish and Their Predator. Comp. Biochem. Physiology Part A Mol. Integr. Physiology 166, 482–489. doi:10.1016/j.cbpa.2013.07.025
Downs C. J., Brown J. L., Wone B., Donovan E. R., Hunter K., Hayes J. P. (2013). Selection for Increased Mass-independent Maximal Metabolic Rate Suppresses Innate but Not Adaptive Immune Function. Proc. R. Soc. B 280, 20122636. doi:10.1098/rspb.2012.2636
Fenton A., Rands S. A. (2006). The Impact of Parasite Manipulation and Predator Foraging Behavior on Predator-Prey Communities. Ecology 87, 2832–2841. doi:10.1890/0012-9658(2006)87[2832:tiopma]2.0.co;2
Fox J., Weisberg S. (2011). An R Companion to Applied Regression. Second. California: Sage. Available at: http://cran.r-project.org/web/packages/car/citation.html.
Grutter A. (1995). Comparison of Methods for Sampling Ectoparasites from Coral Reef Fishes. Mar. Freshw. Res. 46, 897–903. doi:10.1071/MF9950897
Grutter A. (2003). Feeding Ecology of the Fish Ectoparasite Gnathia Sp. (Crustacea: Isopoda) from the Great Barrier Reef, and its Implications for Fish Cleaning Behaviour. Mar. Ecol. Prog. Ser. 259, 295–302. doi:10.3354/meps259295
Grutter A. (1996). Parasite Removal Rates by the Cleaner Wrasse Labroides dimidiatus. Mar. Ecol. Prog. Ser. 130, 61–70. doi:10.3354/meps130061
Grutter A. S., Blomberg S. P., Box S., Bshary R., Ho O., Madin E. M. P., et al. (2019). Changes in Local Free-Living Parasite Populations in Response to Cleaner Manipulation over 12 Years. Oecologia 190, 783–797. doi:10.1007/s00442-019-04451-8
Grutter A. S., Crean A. J., Curtis L. M., Kuris A. M., Warner R. R., McCormick M. I. (2011). Indirect Effects of an Ectoparasite Reduce Successful Establishment of a Damselfish at Settlement. Funct. Ecol. 25, 586–594. doi:10.1111/j.1365-2435.2010.01798.x
Grutter A. S., De Brauwer M., Bshary R., Cheney K. L., Cribb T. H., Madin E. M. P., et al. (2018). Parasite Infestation Increases on Coral Reefs without Cleaner Fish. Coral Reefs 37, 15–24. doi:10.1007/s00338-017-1628-z
Grutter A. S., Feeney W. E., Hutson K. S., McClure E. C., Narvaez P., Smit N. J., et al. (2020). Practical Methods for Culturing Parasitic Gnathiid Isopods. Int. J. Parasitol. 50, 825–837. doi:10.1016/j.ijpara.2020.03.014
Grutter A. S. (2008). Interactions between Gnathiid Isopods, Cleaner Fish and Other Fishes on Lizard Island, Great Barrier Reef. J. Fish. Biol. 73, 2094–2109. doi:10.1111/j.1095-8649.2008.02073.x
Grutter A. S., Murphy J. M., Choat J. H. (2003). Cleaner Fish Drives Local Fish Diversity on Coral Reefs. Curr. Biol. 13, 64–67. doi:10.1016/S0960-9822(02)01393-3
Grutter A. S. (1997). Spatiotemporal Variation and Feeding Selectivity in the Diet of the Cleaner Fish Labroides dimidiatus. Copeia 1997, 346–355. doi:10.2307/1447754
Gunderson A. R., Armstrong E. J., Stillman J. H. (2016). Multiple Stressors in a Changing World: The Need for an Improved Perspective on Physiological Responses to the Dynamic Marine Environment. Annu. Rev. Mar. Sci. 8, 357–378. doi:10.1146/annurev-marine-122414-033953
Halsey L. G., Killen S. S., Clark T. D., Norin T. (2018). Exploring Key Issues of Aerobic Scope Interpretation in Ectotherms: Absolute versus Factorial. Rev. Fish. Biol. Fish. 28, 405–415. doi:10.1007/s11160-018-9516-3
Hamilton S. L., Logan C. A., Fennie H. W., Sogard S. M., Barry J. P., Makukhov A. D., et al. (2017). Species-Specific Responses of Juvenile Rockfish to Elevated pCO2: From Behavior to Genomics. PLoS One 12, e0169670. doi:10.1371/journal.pone.0169670
Hannan K. D., Rummer J. L. (2018). Aquatic Acidification: A Mechanism Underpinning Maintained Oxygen Transport and Performance in Fish Experiencing Elevated Carbon Dioxide Conditions. J. Exp. Biol. 221, jeb154559. doi:10.1242/jeb.154559
Harianto J., Carey N., Byrne M. (2019). respR-An R Package for the Manipulation and Analysis of Respirometry Data. Methods Ecol. Evol. 10, 912–920. doi:10.1111/2041-210X.13162
Hayes P. M., Smit N. J., Grutter A. S., Davies A. J. (2011b). Unexpected Response of a Captive Blackeye Thicklip, Hemigymnus melapterus (Bloch), from Lizard Island, Australia, Exposed to Juvenile Isopods Gnathia Aureamaculosa Ferreira & Smit. J. Fish. Dis. 34, 563–566. doi:10.1111/j.1365-2761.2011.01261.x
Hayes P. M., Wertheim D. F., Smit N. J., Seddon A. M., Davies A. J. (2011a). Three-dimensional Visualisation of Developmental Stages of an Apicomplexan Fish Blood Parasite in its Invertebrate Host. Parasites Vectors 4, 4–8. doi:10.1186/1756-3305-4-219
Heuer R. M., Grosell M. (2014). Physiological Impacts of Elevated Carbon Dioxide and Ocean Acidification on Fish. Am. J. Physiology-Regulatory, Integr. Comp. Physiology 307, R1061–R1084. doi:10.1152/ajpregu.00064.2014
Heuer R. M., Welch M. J., Rummer J. L., Munday P. L., Grosell M. (2016). Altered Brain Ion Gradients Following Compensation for Elevated CO2 Are Linked to Behavioural Alterations in a Coral Reef Fish. Sci. Rep. 6, 1–10. doi:10.1038/srep33216
Hughes T. P., Kerry J. T., Álvarez-Noriega M., Álvarez-Romero J. G., Anderson K. D., Baird A. H., et al. (2017). Global Warming and Recurrent Mass Bleaching of Corals. Nature 543, 373–377. doi:10.1038/nature21707
Hutson K. S., Cable J., Grutter A. S., Paziewska-Harris A., Barber I. (2018). Aquatic Parasite Cultures and Their Applications. Trends Parasitol. 34, 1082–1096. doi:10.1016/j.pt.2018.09.007
Hvas M., Karlsbakk E., Mæhle S., Wright D. W., Oppedal F. (2017). The Gill Parasite Paramoeba Perurans Compromises Aerobic Scope, Swimming Capacity and Ion Balance in Atlantic Salmon. Conserv. Physiol. 5, 1–12. doi:10.1093/conphys/cox066
Jones C. M., Grutter A. S. (2008). Reef-based Micropredators Reduce the Growth of Post-settlement Damselfish in Captivity. Coral Reefs 27, 677–684. doi:10.1007/s00338-008-0383-6
Killen S. S., Mitchell M. D., Rummer J. L., Chivers D. P., Ferrari M. C. O., Meekan M. G., et al. (2014). Aerobic Scope Predicts Dominance During Early Life in a Tropical Damselfish. Funct. Ecol. 28, 1367–1376. doi:10.1111/1365-2435.12296
Klemme I., Hyvärinen P., Karvonen A. (2020). Negative Associations between Parasite Avoidance, Resistance and Tolerance Predict Host Health in Salmonid Fish Populations. Proc. R. Soc. B 287, 20200388. doi:10.1098/rspb.2020.0388
Lenth R. (2020). Emmeans: Estimated Marginal Means, Aka Least-Squares Means. R package version 1.4.4.
Lochmiller R. L., Deerenberg C. (2000). Trade-offs in Evolutionary Immunology: Just what Is the Cost of Immunity? Oikos 88, 87–98. doi:10.1034/j.1600-0706.2000.880110.x
Lüdecke D., Ben-Shachar M., Patil I., Waggoner P., Makowski D. (2021). Performance: An R Package for Assessment, Comparison and Testing of Statistical Models. Joss 6, 3139. doi:10.21105/joss.03139
McCormick M. I. (2016). Protogyny in a Tropical Damselfish: Females Queue for Future Benefit. PeerJ 4, e2198. doi:10.7717/peerj.2198
McMahon S. J., Parsons D. M., Donelson J. M., Pether S. M. J., Munday P. L. (2020). Elevated CO2 and Heatwave Conditions Affect the Aerobic and Swimming Performance of Juvenile Australasian Snapper. Mar. Biol. 167, 1–12. doi:10.1007/s00227-019-3614-1
Metcalfe N. B., Van Leeuwen T. E., Killen S. S. (2016). Does Individual Variation in Metabolic Phenotype Predict Fish Behaviour and Performance?. J. Fish Biol. 88, 298–321. doi:10.1111/jfb.12699
Methling C., Pedersen P. B., Steffensen J. F., Skov P. V. (2013). Hypercapnia Adversely Affects Postprandial Metabolism in the European Eel (Anguilla anguilla). Aquaculture 416-417, 166–172. doi:10.1016/j.aquaculture.2013.09.014
Miller G. M., Watson S.-A., Mccormick M. I., Munday P. L. (2013). Increased CO2stimulates Reproduction in a Coral Reef Fish. Glob. Change Biol. 19, 3037–3045. doi:10.1111/gcb.12259
Munday P., Crawley N., Nilsson G. (2009). Interacting Effects of Elevated Temperature and Ocean Acidification on the Aerobic Performance of Coral Reef Fishes. Mar. Ecol. Prog. Ser. 388, 235–242. doi:10.3354/meps08137
Munday P. L., Welch M. J., Allan B. J. M., Watson S.-A., McMahon S. J., McCormick M. I. (2016). Effects of Elevated CO2on Predator Avoidance Behaviour by Reef Fishes Is Not Altered by Experimental Test Water. PeerJ 4, e2501. doi:10.7717/peerj.2501
Niimi A. J., Beamish F. W. H. (1974). Bioenergetics and Growth of Largemouth Bass (Micropterus salmoides) in Relation to Body Weight and Temperature. Can. J. Zool. 52, 447–456. doi:10.1139/z74-056
Oliver E. C. J., Donat M. G., Burrows M. T., Moore P. J., Smale D. A., Alexander L. V., et al. (2018). Longer and More Frequent Marine Heatwaves over the Past Century. Nat. Commun. 9, 1324. doi:10.1038/s41467-018-03732-9
Östlund-Nilsson S., Curtis L., Nilsson G. E., Grutter A. S. (2005). Parasitic Isopod Anilocra Apogonae, a Drag for the Cardinal Fish Cheilodipterus quinquelineatus. Mar. Ecol. Prog. Ser. 287, 209–216. doi:10.3354/meps287209
Paula J. R., Baptista M., Carvalho F., Repolho T., Bshary R., Rosa R. (2019b). The Past, Present and Future of Cleaner Fish Cognitive Performance as a Function of CO 2 Levels. Biol. Lett. 15, 20190618. doi:10.1098/rsbl.2019.0618
Paula J. R., Otjacques E., Hildebrandt C., Grutter A. S., Rosa R. (2020). Ocean Acidification Does Not Affect Fish Ectoparasite Survival. Oceans 1, 27–33. doi:10.3390/oceans1010003
Paula J. R., Repolho T., Pegado M. R., Thörnqvist P.-O., Bispo R., Winberg S., et al. (2019a). Neurobiological and Behavioural Responses of Cleaning Mutualisms to Ocean Warming and Acidification. Sci. Rep. 9, 12728. doi:10.1038/s41598-019-49086-0
Paula J. R., Sun D., Pissarra V., Narvaez P., Rosa R., Grutter A. S., et al. (2021). The Role of Corals on the Abundance of a Fish Ectoparasite in the Great Barrier Reef. Coral Reefs 40, 535–542. doi:10.1007/s00338-021-02051-8
R Core Team (2017). R: A Language and Environment for Statistical Computing. R Foundation For Statistical Computing. Available at: http://www.r-project.org.
Råberg L., Graham A. L., Read A. F. (2009). Decomposing Health: Tolerance and Resistance to Parasites in Animals. Phil. Trans. R. Soc. B 364, 37–49. doi:10.1098/rstb.2008.0184
Raby G. D., Sundin J., Jutfelt F., Cooke S. J., Clark T. D. (2018). Exposure to Elevated Carbon Dioxide Does Not Impair Short-Term Swimming Behaviour or Shelter-Seeking in a Predatory Coral-Reef Fish. J. Fish. Biol. 93, 138–142. doi:10.1111/jfb.13728
Roche D. G., Binning S. A., Bosiger Y., Johansen J. L., Rummer J. L. (2013). Finding the Best Estimates of Metabolic Rates in a Coral Reef Fish. J. Exp. Biol. 216, 2103–2110. doi:10.1242/jeb.082925
Ros A. F. H., Lusa J., Meyer M., Soares M., Oliveira R. F., Brossard M., et al. (2011). Does Access to the Bluestreak Cleaner Wrasse Labroides dimidiatus Affect Indicators of Stress and Health in Resident Reef Fishes in the Red Sea? Hormones Behav. 59, 151–158. doi:10.1016/j.yhbeh.2010.11.006
Rummer J. L., Stecyk J. A., Couturier C. S., Watson S. A., Nilsson G. E., Munday P. L. (2013). Elevated CO2 Enhances Aerobic Scope of a Coral Reef Fish. Conserv. Physiol. 1, cot023. doi:10.1093/conphys/cot023.Introduction
Rummer J. L., Binning S. A., Roche D. G., Johansen J. L. (2016). Methods Matter: Considering Locomotory Mode and Respirometry Technique when Estimating Metabolic Rates of Fishes. Conserv. Physiol. 4, cow008. doi:10.1093/conphys/cow008
Sheldon B. C., Verhulst S. (1996). Ecological Immunology: Costly Parasite Defences and Trade-Offs in Evolutionary Ecology. Trends Ecol. Evol. 11, 317–321. doi:10.1016/0169-5347(96)10039-2
Shodipo M. O., Duong B., Graba-Landry A., Grutter A. S., Sikkel P. C. (2020). Effect of Acute Seawater Temperature Increase on the Survival of a Fish Ectoparasite. Oceans 1, 215–236. doi:10.3390/oceans1040016
Sikkel P. C., Richardson M. A., Sun D., Narvaez P., Feeney W. E., Grutter A. S. (2019). Changes in Abundance of Fish-Parasitic Gnathiid Isopods Associated with Warm-Water Bleaching Events on the Northern Great Barrier Reef. Coral Reefs 38, 721–730. doi:10.1007/s00338-019-01835-3
Sun D., Cheney K. L., Werminghausen J., Meekan M. G., McCormick M. I., Cribb T. H., et al. (2015). Presence of Cleaner Wrasse Increases the Recruitment of Damselfishes to Coral Reefs. Biol. Lett. 11, 20150456. doi:10.1098/rsbl.2015.0456
Sunday J. M., Calosi P., Dupont S., Munday P. L., Stillman J. H., Reusch T. B. H. (2014). Evolution in an Acidifying Ocean. Trends Ecol. Evol. 29, 117–125. doi:10.1016/j.tree.2013.11.001
Sundin J., Amcoff M., Mateos-González F., Raby G. D., Jutfelt F., Clark T. D. (2017). Long-term Exposure to Elevated Carbon Dioxide Does Not Alter Activity Levels of a Coral Reef Fish in Response to Predator Chemical Cues. Behav. Ecol. Sociobiol. 71, 108. doi:10.1007/s00265-017-2337-x
Tirsgaard B., Moran D., Steffensen J. F. (2015). Prolonged SDA and Reduced Digestive Efficiency under Elevated CO2 May Explain Reduced Growth in Atlantic Cod (Gadus morhua). Aquat. Toxicol. 158, 171–180. doi:10.1016/j.aquatox.2014.11.009
Triki Z., Bshary R. (2019). Fluctuations in Coral Reef Fish Densities after Environmental Disturbances on the Northern Great Barrier Reef. PeerJ 7, e6720. doi:10.7717/peerj.6720
Triki Z., Wismer S., Levorato E., Bshary R. (2018). A Decrease in the Abundance and Strategic Sophistication of Cleaner Fish after Environmental Perturbations. Glob. Change Biol. 24, 481–489. doi:10.1111/gcb.13943
Vaughan D. B., Grutter A. S., Costello M. J., Hutson K. S. (2017). Cleaner Fishes and Shrimp Diversity and a Re-evaluation of Cleaning Symbioses. Fish. Fish. 18, 698–716. doi:10.1111/faf.12198
Wagner E. L. E. S., Roche D. G., Binning S. A., Wismer S., Bshary R. (2015). Temporal Comparison and Predictors of Fish Species Abundance and Richness on Undisturbed Coral Reef Patches. PeerJ 3, e1459. doi:10.7717/peerj.1459
Waldie P. A., Blomberg S. P., Cheney K. L., Goldizen A. W., Grutter A. S. (2011). Long-term Effects of the Cleaner Fish Labroides dimidiatus on Coral Reef Fish Communities. PLoS One 6, e21201. doi:10.1371/journal.pone.0021201
Welch M. J., Munday P. L. (2016). Contrasting Effects of Ocean Acidification on Reproduction in Reef Fishes. Coral Reefs 35, 485–493. doi:10.1007/s00338-015-1385-9
Wisenden B. D. (2012). Cognitive Dysfunction and Risk Assessment by Prey: Predictable Changes in Global Climate Have Unpredictable Effects. Funct. Ecol. 26, 551–552. doi:10.1111/j.1365-2435.2011.01956.x
Zuur A. F., Ieno E. N., Elphick C. S. (2010). A Protocol for Data Exploration to Avoid Common Statistical Problems. Methods Ecol. Evol. 1, 3–14. doi:10.1111/j.2041-210X.2009.00001.x
Keywords: cooperation, cleaning mutualism, ocean acidification, climate change, metabolism, adaptation
Citation: Paula JR, Repolho T, Grutter AS and Rosa R (2022) Access to Cleaning Services Alters Fish Physiology Under Parasite Infection and Ocean Acidification. Front. Physiol. 13:859556. doi: 10.3389/fphys.2022.859556
Received: 21 January 2022; Accepted: 27 April 2022;
Published: 08 June 2022.
Edited by:
Enric Gisbert, Institute of Agrifood Research and Technology (IRTA), SpainReviewed by:
Amélie Crespel, University of Turku, FinlandTrevor James Hamilton, MacEwan University, Canada
Copyright © 2022 Paula, Repolho, Grutter and Rosa. This is an open-access article distributed under the terms of the Creative Commons Attribution License (CC BY). The use, distribution or reproduction in other forums is permitted, provided the original author(s) and the copyright owner(s) are credited and that the original publication in this journal is cited, in accordance with accepted academic practice. No use, distribution or reproduction is permitted which does not comply with these terms.
*Correspondence: José Ricardo Paula, anJwYXVsYUBmYy51bC5wdA==
†ORCID: José Ricardo Paula, orcid.org/0000-0002-1729-7256; Tiago Repolho, orcid.org/0000-0002-1048-8009; Alexandra S. Grutter, orcid.org/0000-0003-1688-2821; Rui Rosa orcid.org/0000-0003-2801-5178