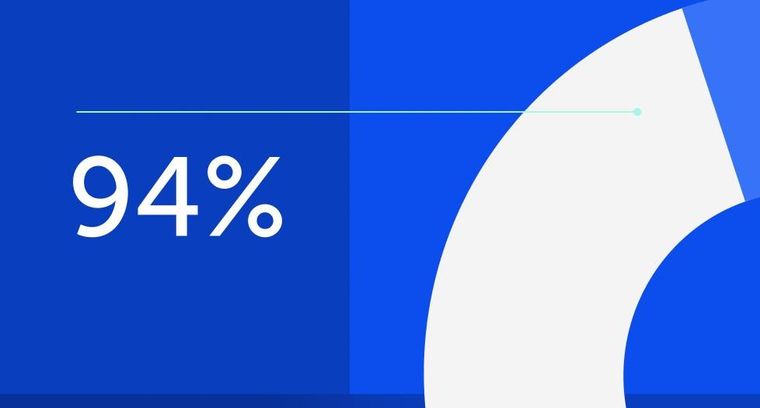
94% of researchers rate our articles as excellent or good
Learn more about the work of our research integrity team to safeguard the quality of each article we publish.
Find out more
REVIEW article
Front. Physiol., 16 March 2022
Sec. Integrative Physiology
Volume 13 - 2022 | https://doi.org/10.3389/fphys.2022.858941
This article is part of the Research TopicInsights in Integrative Physiology: 2021View all 27 articles
The paraventricular nucleus (PVN) is a highly organized structure of the hypothalamus that has a key role in regulating cardiovascular and osmotic homeostasis. Functionally, the PVN is divided into autonomic and neuroendocrine (neurosecretory) compartments, both equally important for maintaining blood pressure (BP) and body fluids in the physiological range. Neurosecretory magnocellular neurons (MCNs) of the PVN are the main source of the hormones vasopressin (VP), responsible for water conservation and hydromineral balance, and oxytocin (OT), involved in parturition and milk ejection during lactation. Further, neurosecretory parvocellular neurons (PCNs) take part in modulation of the hypothalamic–pituitary–adrenal axis and stress responses. Additionally, the PVN takes central place in autonomic adjustment of BP to environmental challenges and contributes to its variability (BPV), underpinning the PVN as an autonomic master controller of cardiovascular function. Autonomic PCNs of the PVN modulate sympathetic outflow toward heart, blood vessels and kidneys. These pre-autonomic neurons send projections to the vasomotor nucleus of rostral ventrolateral medulla and to intermediolateral column of the spinal cord, where postganglionic fibers toward target organs arise. Also, PVN PCNs synapse with NTS neurons which are the end-point of baroreceptor primary afferents, thus, enabling the PVN to modify the function of baroreflex. Neuroendocrine and autonomic parts of the PVN are segregated morphologically but they work in concert when the organism is exposed to environmental challenges via somatodendritically released VP and OT by MCNs. The purpose of this overview is to address both neuroendocrine and autonomic PVN roles in BP and BPV regulation.
Occupying a small portion of the vertebrate brain (1%), the PVN is a highly organized effector structure (Swanson, 1995; Benarroch, 2005). This hypothalamic nucleus is located bilaterally around the third ventricle (Badoer, 2001). Morphological studies of the PVN reveal different cell populations within its borders, such that the PVN can be divided into at least three magnocellular (anterior, posterior and medial subnuclei) and five parvocellular (dorsal, lateral, medial, periventricular and anterior subnucleus) compartments (Swanson and Kuypers, 1980; Sawchenko and Swanson, 1982a; Swanson and Sawchenko, 1983; Badoer, 2001; Pyner, 2009). Two functionally separate areas of the PVN, neuroendocrine and autonomic, subserve its potential to regulate BP, making the PVN a major integrative site of cardiovascular function (Swanson and Kuypers, 1980; Swanson and Sawchenko, 1983; Son et al., 2013; Sladek et al., 2015). Blood pressure, which is defined by peripheral vascular resistance and cardiac output (resultant of heart rate and stroke volume), is modified by both neuroendocrine and autonomic premotor PVN in at least three different effector pathways (Figure 1): neurosecretory magnocellular, neurosecretory parvocellular, and pre-autonomic parvocellular neural pathway (Badoer, 2001; Sladek et al., 2015).
Figure 1. Neuroendocrine and pre-autonomic paraventricular nucleus in cardiovascular regulation. MCNs synthetize VP which is transported via their axons to neurohypophysis for systemic release. Once in the circulation, VP reaches distant targets (kidneys, resistance vessels) to exert its effects. Some portion of VP is released intranuclearly, and modulates the activity of pre-autonomic PCNs. These pre-autonomic neurons have the potential to modulate the autonomic outflow toward heart, kidneys and arterioles. Additionally, VP is co-expressed with CRH alternating the reactivity of HPA axis. VP, vasopressin; CRH, corticotropin-releasing hormone; ACTH, adrenocorticotropic hormone; MCN, Magnocellular neuron; PCNs, parvocellular neuron; SPANs, spinally projecting pre-autonimic neurons; RVLM, rostral ventrolateral medulla; IML, intermediolateral nucleus.
Over 30 distinct neurotransmitters and neuromodulators have been identified to be synthesized within the PVN (Swanson and Sawchenko, 1983). Most abundantly expressed are vasopressin (VP) and oxytocin (OT), which are produced by the magnocellular neurons (MCNs) of the PVN (Swanson and Sawchenko, 1980; Sladek et al., 2015). VP is best known for its role in maintaining cardiovascular and body fluid balance, whereas OT takes part in parturition, lactation and accompanying reproductive behaviors (Gimpl and Fahrenholz, 2001; Koshimizu et al., 2006; Stoop, 2014). Additionally, VP and corticotropin-releasing hormone (CRH) expressing parvocellular neurons (PCNs) of the PVN possess a secretory capacity and mediate the central response of the hypothalamic–pituitary–adrenal (HPA) axis to stress (Swanson and Sawchenko, 1980; Sawchenko et al., 1996; Benarroch, 2005; Sladek et al., 2015). Magnocellular and parvocellular neuroendocrine neurons of the PVN initiate a downstream chain of events that dictate changes in BP.
Magnocellular neurons express VP and OT in large quantities (Dierickx, 1980). Located mostly in the medial and the lateral posterior subdivision of the PVN, MCNs send their axonal projections to neurohypophysis, from where VP and OT is released into the systemic circulation (Armstrong et al., 1980; Swanson and Sawchenko, 1983; Sladek et al., 2015). Once secreted into blood, these peptide hormones act upon distant targets (Dierickx, 1980; Gutkowska et al., 2000; Japundžić-Žigon, 2013). VP and OT exert their effects through activation of cognate receptors that are expressed both in the brain and periphery (Brinton et al., 1984; Ostrowski et al., 1992; Hirasawa et al., 1994; Adan et al., 1995; Kato et al., 1995). VP receptors (VR) and OT receptors (OTR) are a subfamily of G coupled receptors (G protein-coupled receptors—GPCRs) with high structural homology (85% homology found between V1aR and OTR; Barberis et al., 1998; Thibonnier et al., 2002; Koshimizu et al., 2012). In the periphery, VP mainly engages V1aR and V2R, while in the central nervous system VP action is mostly mediated by V1aR and far less by V1bRs (Ostrowski et al., 1992, 1994; Young et al., 2006; Roper et al., 2011; Russell and Brunton, 2017).
The strongest stimulus for the MCNs to secret VP into the bloodstream is hyperosmotic change (McKinley et al., 2004). Hyperosmolality of blood is sensed by circumventricular organs in the periventricular region of the third ventricle (anteroventral third ventricle—AV3V), which is devoid of the blood brain barrier. The circumventricular subfornical organ (SFO) and organum vasculosum laminae terminalis (OVLT) are richly vascularised, and their neurons easily sense perturbations in blood osmolality (Leng et al., 1989; Cunningham and Sawchenko, 1991; McKinley et al., 2004). From these structures, direct or indirect (via medial preoptic nucleus—MnPO) excitatory axonal projections to MCNs, stimulate the secretion of VP into circulation (Leng et al., 2001; McKinley et al., 2004). Additionally, several other stimuli trigger VP secretion: decreased blood volume and pressure which induce reduction of stretch in low-pressure receptors of venous system and high-pressure baroreceptors respectively and ANG II (Japundžić-Žigon et al., 2020).
The best known roles of peripheral VP are water conservation in kidneys and vasoconstriction (Altura and Altura, 1984; Bankir, 2001; Sladek et al., 2015). Indeed, VP is often referred to as antidiuretic hormone (ADH), due to its role in water conservation by the activation of V2Rs in the kidneys (Ostrowski et al., 1993; Gordan et al., 2015). Even small changes in VP concentration in the serum will activate renal V2Rs, with highest affinity to VP, to preserve water (Japundžić-Žigon, 2013). V2Rs are located in the basolateral membrane of the epithelial cells of the collecting ducts of the kidney. Activation of V2R is responsible for a cascade of events that involve phosphorylation of aquaporin 2 (AQP-2) and its translocation into the luminal membrane of epithelial cells, leading to reabsorption of water by the kidneys. VP also affects AQP-2 transcription rates and increases AQP-2 protein abundance (Imbert et al., 1975; Knepper, 1997; Bankir, 2001; Wilson et al., 2013; Jung and Kwon, 2016).
The vasoconstrictor effect of VP is mediated by V1aRs located on blood vessels. Although in vitro studies confirm VP as a most potent vasoconstrictor agent, relatively high concentrations of VP are necessary to elevate BP in vivo under basal physiological conditions (Altura and Altura, 1984; Johnston, 1985; Koshimizu et al., 2006) in respect to much less potent vasoconstrictors at molar level such as angiotensin II and noradrenalin. Nevertheless, vascular V1aRs are crucial for BP maintenance during hypovolemia and hypotension (Koshimizu et al., 2012). Also, in some vascular beds, such as in lung, liver and kidneys VP activates V1aR and V2R to produce nitrogen monoxide (NO) dependent vasodilatation (Liard, 1984; Hirsch et al., 1989; Russ and Walker, 1992; Aki et al., 1994; Koshimizu et al., 2006).
Circulating VP can circumvent the blood brain barrier and reach centrally located receptors (Landgraf and Neumann, 2004). The most prominent impact of peripheral VP on blood pressure is exerted at the level of area postrema (AP), where it can modify the activity of the baroreflex (Brizzee and Walker, 1990; Hasser et al., 1997). Even though the VP effects on baroreflex are controversial, the majority of studies report enhancement of baroreflex sensitivity (BRS; Brizzee and Walker, 1990; Hasser and Bishop, 1990; Hasser et al., 1997; Koshimizu et al., 2006). Further support is provided by experiments which show that lesions of the AP region disable peripheral VP to modulate baroreflex (Hasser et al., 1997). Also, VP deficient Brattleboro rats exhibit decreased BRS (Imai et al., 1983b). Pharmacological studies suggest that this effect is conveyed by V1aR (Imai et al., 1983a; Hasser and Bishop, 1990; Sampey et al., 1999). The effect of VP on BRS is complex, and when VP is released centrally during stress and exercise, it will act oppositely and reduce BRS via medullary V1aRs (Unger et al., 1986).
Afferents that originate from stretch receptors and baroreceptors are tonically active under physiological conditions and inhibit VP secretion from MCNs, while decrease in blood volume and BP leads to disinhibition and consequent release of VP (Bisset and Chowdrey, 1988). Before it reaches the PVN, the information from the baroreceptors is conveyed via the nucleus tractus solitarius (NTS) and ventrolateral medulla (VLM). Afferents arising from the NTS (A2 type neurons) and VLM (A1 cell group) that project to MCNs are primarily noradrenergic (Sawchenko and Swanson, 1982b). Putative inhibitory mechanisms involve NTS residing GABAergic neurons which silence the noradrenergic excitatory pathways directed to the magnocellular subdivision of the PVN (Jhamandas and Renaud, 1987; Bisset and Chowdrey, 1988; Leng et al., 1999).
Altogether, it seems that the minor effect of VP on BP maintenance under basal physiological conditions is due to the BRS enhancement via AP and consequent decrease in heart rate which efficiently oppose its vasoconstrictor performance on the periphery (Abboud et al., 1990; Koshimizu et al., 2006).
Oxytocin is a nonapeptide hormone best known for inducing uterine contractions during labor and milk ejection during lactation (Dale, 1906; Ott and Scott, 1910; Du Vigneaud, 1954). Apart from these primary roles, OT is involved in a large number of physiological activities, including cardiovascular control (Maier et al., 1998). OT secretion in hypothalamus is not only sex dependent, and it can be modulated by hyperosmotic stimuli, hypovolemia and ANG II (Kadekaro et al., 1992; Gimpl and Fahrenholz, 2001). Mechanisms underlying OT involvement in BP regulation are not yet fully elucidated (Gutkowska et al., 2000). OT activity usually correlates with BP decrease in many species. Experiments with RNA interference report that blocking brain OT RNA leads to an increase in BP (Petersson et al., 1996, 1997; Maier et al., 1998). One of the putative mechanisms behind OT modulation of BP lies in its engagement with electrolyte excretion in the kidneys. It is suggested that OT induced natriuresis is mediated by atrial natriuretic peptide (ANP; Verbalis et al., 1991; Conrad et al., 1993; Gutkowska et al., 2000). However, this natriuretic effect is not confirmed in humans, and it appears that it is present only in certain species, such as rats (Rasmussen et al., 2004).
Since VP and OT share very similar primary structures (only differing by 2 amino acids at positions 3 and 8), as well as their receptors, OT can bind with high affinity to V1aRs as well as OTRs (Gimpl and Fahrenholz, 2001; De Bree et al., 2003). OT receptors have a wide distribution in the body (Adan et al., 1995). As well as the reproductive system, OTRs can be found within the brain and heart (Adan et al., 1995; Gutkowska et al., 1997). OT induced peripheral vasoconstriction can be mediated by V1aRs. In vitro studies reveal that the potency of OT to contract blood vessel smooth muscle cells is much less than VP, with the exception of the umbilical artery at term (Altemus et al., 2001). When vascular tone is already increased, OT will produce NO dependent vasodilatation in some vascular beds, like in basilar arteries (Katusic et al., 1986; Russ et al., 1992; Thibonnier et al., 1999). It seems that OT is not responsible for modulating the peripheral resistance in pregnant rats, and does not play a significant role in setting the levels of BP under physiological conditions (Miller et al., 2002).
Even though experimental evidence does not support strong involvement of VP and OT in BP regulation under baseline physiological conditions, studies with VP and OT gene knock-out mice provide a contrasting insight. VP knock-out mice exhibit lower basal values of BP, while OT deficient mice demonstrate elevated BP and HR values in comparison to wild type controls (Bernatova et al., 2004; Koshimizu et al., 2006). Apart from being normotensive, VP deficient Brattleboro rats exhibit decreased BRS. However, it should be noted that the complex and unpredictable developmental compensations occurring in global knock-out mice makes the interpretation of adult phenotypes problematic (Valtin, 1982; Imai et al., 1983b; Bohus and de Wied, 1998).
Vasopressin and OT can be synthesized and released from dendrites and soma of MCNs and this can happen without cell depolarization (Scala-Guenot et al., 1987; Moos et al., 1989, 1998; Pow and Morris, 1989; Neumann et al., 1993b; Ludwig et al., 1995, 2002; Hurbin et al., 1998, 2002). VP in the extracellular space can exert both autocrine and paracrine effects (Ludwig and Stern, 2015). Intranuclear VP can activate V1aRs and V1bR expressed on MCNs, or it can spill over through the extracellular space into the cerebrospinal fluid (Landgraf and Neumann, 2004) and reach remote targets (Hurbin et al., 1998, 2002; Son et al., 2013). It has been proposed that intranuclear receptors optimize the firing rate of the entire population of MCNs to best respond to physiological demands (Son et al., 2013). Somatodendritic release depends on the quality and intensity of stimulus and it can be regulated independently from systemic release (Pow and Morris, 1989; Neumann et al., 1993a; Ota et al., 1994; Kendrick et al., 1997; Gouzènes et al., 1998; Landgraf and Neumann, 2004). It has been suggested that the autocontrol of MCN by intranuclear VP can be either inhibitory or excitatory (Inenaga and Yamashita, 1986; Gouzènes et al., 1998; Dayanithi et al., 2000; Landgraf and Neumann, 2004). Additionally, somatodendritically released VP (Figure 1) can activate surrounding silent MCNs (Moos et al., 1998), or depolarize neighboring interneurons and PCNs (Carette and Poulain, 1989; Son et al., 2013). Activation of autonomic PCNs through engagement of V1aRs, especially during hyperosmotic stimulus, is particularly important in terms of integration of neuroendocrine and autonomic regulation of blood pressure (Swanson and Sawchenko, 1980; Son et al., 2013). It has also been reported that intranuclear V1bRs participate in setting sympathetic outflow toward the kidneys (El-Werfali et al., 2015).
Stress is an attributed risk factor for cardiovascular diseases that can trigger bad clinical outcomes (Hjemdahl, 2002; Rosmond, 2005). PVN involvement in the stress response has been documented in spontaneously hypertensive rats (SHRs) and other experimental models (Benarroch, 2005). The PVN promotes several aspects of hemodynamic regulation during stress (Herman et al., 1996; Benarroch, 2005). Anterior and medial PCNs that synthesize CRH, are responsible for activating the HPA axis during exposure to stress (Rivier and Vale, 1983; Swanson et al., 1983; Sawchenko et al., 1996; Sladek et al., 2015). Apart from expressing CRH, PCNs produce VP as secretagogue. It appears that the CRH:VP ratio is dictated by the type of stressor, and is crucial for maintaining responsiveness of the HPA axis during chronic stress (Sawchenko and Swanson, 1982a; Swanson and Sawchenko, 1983; Whitnall et al., 1985; de Goeij et al., 1992; Aguilera, 1994; Sawchenko et al., 1996; Amaya et al., 2001). Both CRH and VP are axonally transported to eminentia mediana and released into the portal circulation. Borne by the portal bloodstream, they reach adenohypophysis, where they act on corticotropic cells. CRH stimulates the release of adrenocorticotropic hormone (ACTH), whereas VP potentiates its release by activating V1bRs (Benarroch, 2005). Once in the systemic circulations (Figure 1), ACTH acts on the cells of zona fasciculata in adrenal gland to release glucocorticoids (Myers et al., 2012; Sladek et al., 2015). Cortisol when in excess, has been shown to contribute to hypertension (Kelly et al., 1998). Additionally, elegant ontogenetic experiments suggest that CRH PCNs can increase BP and heart rate via axonal projections to the NTS (Wang et al., 2019), involving corticotropin-releasing hormone receptor type 2 (CRHR2), also associated with hypertension triggered by intermittent hypoxia (Wang et al., 2019).
It has been shown that forced swimming and social confrontation, stressors employed in experimental conditions, can induce somatodendritic release of VP and OT (Wotjak et al., 1996; Engelmann et al., 2001; Ebner et al., 2005), which in turn modifies the activity of the HPA axis. Intranuclear VP has an inhibitory effect on CRH PCNs and consequently reduces secretion of ACTH (Wotjak et al., 1996, 2002; Bosch et al., 2004; Ebner et al., 2005). Extracellular OT exhibits both inhibitory and excitatory influence on the activity of the HPA axis (Neumann et al., 2000; Heinrichs et al., 2002; Neumann, 2002; Landgraf and Neumann, 2004).
Apart from being locally regulated, the cardiovascular system is subject to central control by numerous relevant brain areas (Dampney, 1994). It is well established that PVN takes an important central place in such control. Numerous studies show that the PVN is implicated in the heightened sympathetic tone observed in hypertension (Allen, 2002; Dampney et al., 2018). The autonomic PVN consists of morphologically and functionally diverse cell populations with a specific topography (Stern, 2001; Dampney et al., 2018). It occupies ventromedial, lateral and dorsal (dorsal cap) subdivision of PVN (Nunn et al., 2011; Sladek et al., 2015). More precisely, these PCNs are pre-autonomic, since they control functionally different sympathetic and parasympathetic centers downstream in the medulla: NTS, dorsal motor nucleus of vagus (DMV) and rostral ventrolateral medulla (RVLM), and the spinal cord: intermediolateral nucleus (IML). Therefore, the “pre-autonomic” premotor PVN is responsible for altering the autonomic output toward the cardiovascular and renal systems (Strack et al., 1989a; Coote et al., 1998; Portillo et al., 1998; Coote, 2005; Dampney et al., 2018). Hence it is not surprising that PVN is commonly referred to as an “autonomic master controller,” a term originally introduced by Loewy (1991). There are at least 3 pathways (Figure 1) through which PVN modulates sympathetic outflow (Pyner, 2009):
The first pathway includes PVN pre-sympathetic neurons that have axonal projections that terminate on somata of motor sympathetic preganglionic neurons (SPNs) in the thoraco-lumbal IML. These neurons are usually named as spinally projecting pre-autonomic neurons (SPANs; Dampney, 1994; Badoer, 2001; Nunn et al., 2011). The IML is the final spot of central integration and origin of preganglionic fibers which regulate the activity of blood vessels, heart, kidneys and adrenal gland. Therefore, this is a particularly important target of the PVN pre-autonomic neurons, displaying its enormous potential to directly alternate neurogenic output to the cardiorenal system (Strack et al., 1989a; Dampney, 1994; Badoer, 2001).
The second pathway involves pre-sympathetic PVN neurons that exert indirect influence on sympathetic activity. These neurons terminate at the level of the motor pressor nucleus of the RVLM, responsible for setting sympathetic tone. From there, second order neurons arise and project to SPNs in the thoracic and lumbar IML and change sympathetic outflow toward the cardiovascular system and the kidneys (Dampney, 1994; Badoer, 2001). In general, PVN neurons projecting to RVLM are in greater number than PVN neurons projecting monosinaptically to IML. Therfore, RVLM is a structure embedding most of the pre-sympathetic neurons, which are projecting to IML to exert major autonomic cardiovascular control (Badoer, 2001). Some of the sympatho-excitatory effects in the PVN-RVLM pathways are glutamate mediated (Yang and Coote, 1998). Additionally, evidence has emerged, that PVN can interfere with this PVN-RVLM pathway through its glutamatergic synapses within medial subnucleus of the NTS (mNTS; Kawabe et al., 2008). Using anterograde and retrograde tracing, Kawabe working group confirmed the presence of such bilateral glutamatergic projections spanning to mNTS. They showed that unilateral PVN stimulation with N-methyl-D-aspartic acid (NMDA) leads to increase in mean arterial pressure and greater splanchic nerve activity, and that this effect is emphasized by bilateral blockade of glutamate ionotropic receptors within the mNTS. The authors suggested that PVN glutamatergic pathways directed toward NTS, stimulate inhibition of RVLM-mediated cardiovascular overactivity. Therefore, PVN stimulation with NMDA evokes both RVLM and NTS neuronal routes, but the effects are opposing (Kawabe et al., 2008). Further, same authors showed that tachycardia induced by NMDA stimulation of the PVN is a result of conjoined inhibition of vagal (via ionotropic glutamate and GABA receptors in the mNTS) and activation of sympathetic outputs (via spinal ionotropic glutamate) toward heart, without involvement of spinal VRs and OTRs (Kawabe et al., 2009).
A third pathway is represented by PVN pre-autonomic neurons that can change sympathetic tone both directly and indirectly. Around 30 % of PVN neurons innervate SPNs in the IML, but send collaterals to the RVLM, thus having a dual regulatory role (Badoer, 2001).
The majority of PVN autonomic regulatory function is conveyed by SPANs (Coote, 2007). Since the relevant portion of SPANs is implicated in cardiovascular control (Badoer, 1996; Badoer, 2001; Pyner, 2009; Nunn et al., 2011), this makes them an attractive target for new drug development.
Although their exact functions have yet to be elucidated, SPANs have been suggested to regulate blood volume (Lovick et al., 1993; Pyner and Coote, 2000), circadian variations in BP (Cui et al., 2001), stress induced cardiovascular responses (Jansen et al., 1995a) and much more (Nunn et al., 2011). For these reasons SPANs are referred to as “central command neurons” (Jansen et al., 1995a). Discovering their primary physiological function is further complicated by the fact that SPANs express a lot of different neuroactive substances/neurotransmitters. Indeed, it appears that the majority of these neurons synthesize more than one neurotransmitter (Nunn et al., 2011). The largest portion of SPANs (up to 40 %) is positive for VP and OT (usually co-expressed), as well as dynorphin (Hallbeck and Blomqvist, 1999; Xi et al., 1999; Hallbeck, 2000; Hallbeck et al., 2001). Others possess met-endorphin (up to 20%) and dopamine, met-enkephalin (up to 10 %), leu-enkephalin, somatostatin, ANG II and ANP (Sawchenko and Swanson, 1982a; Cechetto and Saper, 1988; Strack et al., 1989b; Jansen et al., 1995b).
Functional in vivo studies show that spinal levels of VP and OT increase with PVN stimulation (Pittman et al., 1984; Malpas and Coote, 1994). The presence of V1aR and OTR has been confirmed in the gray matter of the spinal cord (Desaulles et al., 1995; Sermasi et al., 1998; Pyner, 2009). Intrathecal pretreatment with V1aR antagonist in the lower thoracic region prevents the increase in renal sympathetic nerve activity and mean arterial pressure normally triggered by the stimulation of the PVN (Malpas and Coote, 1994). It cannot be excluded that some of the OT effects are conveyed via spinal V1aRs as well (Sermasi and Coote, 1994). Similar effects are also observed with OTR selective antagonists which abolish the effects of increased heart rate following the PVN stimulation (Yang et al., 2009). These experimental data highlight VP and OT as PVN SPAN transmitters. VP and OT induce cardio-acceleratory and pressor effects in lower and upper thoracic spinal cord respectively (Pyner, 2009). The majority of vasopressinergic projections implicated in cardiovascular control originate in the lateral and ventral medial subdivision of parvocellular PVN and some portion is found in dorsal parvocellular part (Riphagen and Pittman, 1989; Hallbeck and Blomqvist, 1999). Oxytocinergic SPANs are located in the lateral parvocellular region and dorsal cap (Sawchenko and Swanson, 1982a; Pyner, 2009).
The effects of dopamine as a putative SPAN neurotransmitter are controversial (Strack et al., 1989b; Nunn et al., 2011). Some studies on rats suggest its excitatory influence on SPNs, while others report an inhibitory activity (Gladwell et al., 1999; Gladwell and Coote, 1999a,b; Yang et al., 2002).
Pharmacological experiments with a glutamate antagonist, following chemical stimulation of PVN, imply glutamate as an additional excitatory neurotransmitter of SPANs (Yang et al., 2002).
Although SPANs are positive for enkephalins and ANG II, their influence on sympathetic outflow has not yet been recorded (Sawchenko and Swanson, 1982a; Cechetto and Saper, 1988; Jansen et al., 1995b; Hallbeck and Blomqvist, 1999; Hallbeck et al., 2001). Despite its abundant expression in the nervous system, the presence of inhibitory γ-aminobutyric acid (GABA) as a SPAN output neurotransmitter has not been confirmed (Macdonald and Olsen, 1994; Watkins et al., 2009).
Spinally projecting pre-autonomic neuron activity can be modulated in various ways. A lot has been discovered about the neurotransmitter content of SPANs, but the data regarding receptors expressed on SPANs and molecules their receptors bind is lacking (Nunn et al., 2011). Learning about the neurotransmitters which can modulate the activity of SPANs will open venues to novel therapeutic agents.
It is well established that the PVN is involved in increased sympathetic activity driven by osmotic stimulation, but the mechanisms behind it are poorly understood. Some studies suggest involvement of intranuclear VP released from soma and dendrites of MCNs (Son et al., 2013; Ribeiro et al., 2015). Intranuclear VP stimulates V1aRs expressed on pre-sympathetic parvocellular subdivisions (including SPANs) of the PVN. This leads to increase in sympathetic outflow toward heart, blood vessels and kidneys, followed by BP increase, suggesting this pathway as potential pathophysiological mechanism in neurogenic hypertension (Son et al., 2013; Ribeiro et al., 2015).
Under basal physiological conditions, PVN neurons are tonically inhibited by surrounding GABA neurons, keeping the spontaneously generated nerve impulses at low rate, despite the excitatory influence of glutamate (Kannan et al., 1989; Martin et al., 1991; Badoer et al., 2002; Li et al., 2006). SPANs are confirmed to be silenced by GABA (Cui et al., 2001; Li et al., 2002). This inhibition could be dependent on extrasynaptic GABA (“volume” GABA transmission), but it can also be affected by the rate that glial cells take up GABA from the extracellular space (Brickley et al., 1996; Farrant and Nusser, 2005; Park et al., 2009). GABAA α2-subunit is abundantly expressed in the PVN (Fritschy and Mohler, 1995). Blockage of GABAA receptors by the selective antagonist bicuculline leads to an increase in BP and heart rate (Martin et al., 1991; Martin and Haywood, 1993). Interfering with GABA orchestrated SPAN activity is an appealing therapeutic opportunity, since increasing GABA inhibitory influence would lead to reduction of sympathetic tone and consequently BP decrease (Nunn et al., 2011).
Although injections of ATII in the PVN change blood pressure via angiotensin II receptor type 1 (AT1R; Bains et al., 1992), and this involves the activity of SPANs, it seems that this connection is indirect (Bains and Ferguson, 1995; Li et al., 2003). AT1Rs are expressed in parvocellular division of the PVN on neurons projecting to medulla, not IML (Oldfield et al., 2001; Cato and Toney, 2005).
Spinally projecting pre-autonomic neurons, as well as PCNs which project to RVLM are barosensitive. Under basal physiological conditions, these neurons exhibit spontaneous activity, but they are inhibited by rising pressure (Dampney et al., 2018). Axons arising from caudal NTS neurons terminate on PCNs in the dorsal cap of the PVN. Putative targets of these projections are pre-sympathetic PCNs or GABA interneurons (Pyner, 2009; Dampney, 2017). Other studies do not impose an important role of SPANs in the baroreflex response (Haselton et al., 1994). Volume load is another feed-back mechanism that can modify the activity of SPANs, with PVN being a command center of low-pressure blood volume receptors located in the veno-atrial junction (Gupta et al., 1966; Lovick and Coote, 1988; Lovick et al., 1993; Deng and Kaufman, 1995). Additionally, SPANs can be modulated by some types of stressors, such as psychological stress. It has been shown that conditional fear engages around 10 % of SPANs (Carrive and Gorissen, 2008; Dampney et al., 2018). Also SPANs are affected by temperature, different humoral factors, and inputs from higher brain areas (Dampney et al., 2018).
The peripheral sympathetic nervous system controlling the cardiovascular system has rhythmic activity that creates distinct patterns of sympathetic nerve discharge (SND) in response to physiological demands and pathophysiological conditions. Using frequency analysis of SND, components of SND were identified including fast cardiac and respiratory rhythms, and slow vasomotor rhythm. It is generally believed that the brain is the source of SND. Thus, which parts of the brain are involved, and how they generate peripheral SND rhythms is still unanswered. Two main theories have been postulated, both of which raised considerable criticism. A theory of a central oscillator/pacemaker suggests that the RVLM is the main structure responsible for generating peripheral sympathetic discharge patterns via axonal projections to preganglionic neurons in IML. Recordings of intracellular neuronal activity from medullary slices, uncovered ramp like depolarization following each action potential, leading to subsequent action potential suggestive of pacemaker activity (Li and Guyenet, 1996). This observation was seriously challenged by the in vivo study in rats by Lipski et al. (1996) who observed that RVLM neurons fire irregularly, at much higher discharge rates than the ones in SND, with no evidence of gradual depolarization between individual action potentials (Lipski et al., 1996). They suggested that the regular pattern of firing of RVLM neurons seen in medullary slices, was produced by deafferentation. The work of Lipski and colleagues imposes the network hypothesis, where the activity of pre-sympathetic neurons depends on their antecedent excitatory inputs opposed by tonic inhibitory inputs (setting the level of their excitability). For detailed review refer to Malpas (1998). Geber and Barman postulated a theory of a network of brainstem neurons, whose combined action creates inherent rhythmicity entrained by the baroreflex. These include sympatho-excitatory and sympatho-inhibitory neurons distributed over a wide portion of the lower brainstem that do not have necessarily spontaneous activity (Barman and Gebber, 2000).
In addition to RVLM neurons, hypothalamic PVN SPANs provide monosynaptic inputs to preganglionic neurons in IML. Malpas and Coote (1994) were first to provide evidence in anesthetized rats that chemical stimulation of the PVN by microinjections of homocysteic acid increases the amplitude of renal SND, by recruiting more active fibers, and that this can be blocked by intrathecal injection of V1R antagonist. At the same time the frequency of renal SND, which is modulated by periodic baroreflex inputs (Ninomiya et al., 1990; Malpas and Ninomiya, 1992), remained unaffected. The first experimental evidence that PVN SPANs can incite BPV at the same stimulation frequency was demonstrated in rats by Stauss and associates (Stauss and Kregel, 1996; Stauss et al., 1997). PVN electrically stimulated at frequencies between 0.1 Hz - 0.5 Hz were found to create the same frequencies in the SND pattern and generate BPV in the low frequency (LF) band, which is abolished by α-adrenergic blockade (Japundzic et al., 1990). Stimulation frequencies above 0.5 Hz did not induce BP variations as the blood vessels behaved like cut off filters to high frequencies. Studies on isolated rat vascular smooth muscle cells showed that transmission of fast SND rhythms to BPV is limited by the sluggish, metabotropic α-adrenoceptor signaling, and not by an intrinsic inability of the cells to contract and relax at higher rates (Julien et al., 2001). Thus, SND frequencies higher than 0.5 Hz in rats induce vasoconstriction, increase peripheral resistance and the mean value of BP. It follows that the faster components of BPV, the cardiac component and the respiratory component, are non-neural, created by the perturbations of the circulation induced by the contracting heart and inspiratory movements (Japundzic et al., 1990; Japundzic-Zigon, 1998). The non-neural origin also stands for the slowest, and dominant component of BPV, the very low frequency (VLF) component, which was found to be created by inherent myogenic activity of mesenteric and renal vasculature (VanBavel et al., 1991; Janssen et al., 1995). Although non-neuronal in nature, all the components of BPV can be modulated by the activity of the nervous system and neurohormones (Japundžić-Žigon et al., 2020).
We have investigated the neurochemical contribution of the PVN to short-term BPV, and found that both VP and OT modulate short-term BPV. Using pharmacological and genetic tools in conscious rats we have shown that VP modulates BPV in a complex manner: peripherally as a hormone and centrally as neurotransmitter/modulator (Japundzic-Zigon, 2001, 2013; Japundzić-Zigon et al., 2004, 2018, 2020; Milutinović et al., 2006a,b; Stojicić et al., 2008; Milutinović-Smiljanić et al., 2013; Lozić et al., 2016; Savić et al., 2020). Using spectral analysis of BPV, we found that peripheral administration of non-peptide and selective V1aR or V2R antagonists to conscious normotensive rats under baseline physiological conditions increases BPV, suggesting a buffering role for VP in the VLF domain (Japundzic-Zigon, 2001). We postulated that the decrease of VLF-BPV by VP could be mediated either by the enhancement of the baroreflex sensitivity which normally opposes VLF-BPV, or by the modulation of vasomotion in mesenteric and renal vascular beds (Janssen et al., 1995). In SHR, the buffering capacity of VP on BPV under baseline condition is not preserved (Japundzić-Zigon et al., 2004). This could be due to pathological remodeling of the vasculature in SHR (Head, 1991; Vågnes et al., 2000) making it more sensitive to vasoconstrictors, including VP. Another possibility, even more likely is that impaired baroreflex in SHRs (Dampney, 2017) reduces its capacity to buffer VLF-BPV (Dampney, 2017). However, during hemorrhage, when VP is released in excess in blood, in support of circulation, it acts similarly in normotensive and hypertensive rat strains, and prevents the respiratory related high frequency (HF-BPV) increase, possibly as a consequence of V1aR-mediated vasoconstriction which prevents the unloading of thoracic vessels underlying HF-BPV increase.
Intracerebroventricular injection of selective V1aR, V1bR and V2R antagonists to conscious normotensive rats uncovered that VP acts also centrally to buffer VLF-BPV under baseline physiological conditions by the stimulation of V1aRs possibly in AP, accessible from both sides of the blood brain barrier. However, when VP release is stimulated by stress (Stojicić et al., 2006, 2008; Milutinović et al., 2006b), or by drugs (Milutinović et al., 2006a) and when VP is injected centrally (Milutinović et al., 2006b), an increase of the sympathetically mediated LF-BPV and of the respiration mediated HF-BPV was observed (Milutinović et al., 2006a,b). These effects of VP could involve central V1aR found in abundance in the RVLM where integration of the sympathetic outflow to vasculature occurs; and in the pre-Bötzinger area, where the breathing pattern is set. These central effects of VP could be beneficial and contribute to the lifesaving effect of VP in hemorrhagic, septic and cardiogenic shock as a results of increased tissue oxygenation and additional sympathetic activation, acting synergistically with powerful V1aR mediated peripheral vasoconstriction (Levy et al., 2018). VP can also modulate respiration indirectly (Stojicić et al., 2008) by an anxiogenic action characterized by hyperventilation that occurs possibly by the stimulation of V1bR in bed nucleus stria terminalis (Griebel et al., 2002).
Paraventricular nucleus is a recognized key integrative site of the behavioral, autonomic and endocrine response to stress, expressing V1aRs and V1bRs on somata and dendrites of MCNs and surrounding glia. Stress has been shown to induce VP and OT release in the PVN too (Nishioka et al., 1998; Landgraf and Neumann, 2004), and we have shown that VP and OT act locally, in an autocrine and paracrine manner, to modulate the neuro-cardiogenic stress response. Using adenoviral gene transfer technology we have increased the gene expression and the number of V1aRs in the PVN of Wistar rats. The V1aR rat phenotype had decreased sensitivity of the baroreflex under baseline physiological conditions which was further decreased by stress along with a marked increase of the sympathetically mediated LF-BPV and LF heart rate variability (LF-HRV). These effects could be abolished by intranuclear application of V1aR antagonist. This suggests that V1aR in the PVN can increase the sympathetic outflow to the periphery and modulate BPV and HRV during stress (Lozić et al., 2014; Japundžić-Žigon et al., 2020). In clinical practice, the increase in LF-HRV has been found to predict the occurrence of life threatening arrhythmias in susceptible populations (Huikuri and Stein, 2013). In contrast to V1aR, OTR over-expression in the PVN of Wistar rats had no effect on BPV under baseline conditions but this rat phenotype exhibited reduced baroreflex desensitization by stress and reduced LF-BP increase suggesting that OTR over-expressing rat phenotype is resilient to stress (Lozić et al., 2014; Japundžić-Žigon et al., 2020). In this context, it is important to stress that the modulation of the baroreceptor desensitization during stress by VP seems to be complex and involves more than one central structure and type of VRs (Milutinović-Smiljanić et al., 2013).
A number of clinical studies in hypertensive patients unequivocally show that enhanced BPV increases the risk for developing cardiovascular complications (Mancia and Grassi, 2000). Thus, BPV, and especially sympathetically derived LF-BPV, emerged as an independent predictor of stroke, coronary artery disease, heart and renal failure, as well as all-cause mortality (Mancia et al., 1994; Mancia and Parati, 2003; Messerli et al., 2019; Parati et al., 2020). Our group investigated expression of PVN VP and VRs in the genesis of hypertension. Borderline hypertensive rats (BHR) have a genetic predisposition for hypertension and will develop it when exposed to environmental challenges. Under baseline physiological conditions BHRs have increased expression of VP and V1bR in the PVN and consequently increased plasma VP concentrations, as a constitutive trait (Savić et al., 2020). Spectral markers of sympathetic activity toward blood vessels, LF-BP, and the heart, LF/HF-HR, are comparable to normotensive rats under baseline physiological conditions, suggesting that increased expression of V1bR and VP in BHRs is confined to magnocellular (endocrine) portion of the PVN affecting plasma VP only. However, when exposed to repeated stress and prolonged isotonic saline load, BHRs exhibited LF-BPV increase depicting sympathetic overload, and overt hypertension. In these rats no changes in VP and VR gene transcription in the PVN was noted. Moreover, systemic VP release was decreased, refuting involvement of VP in stress-induced hypertension (Savić et al., 2020).
It is well established that PVN has a paramount role in cardiovascular regulation and contributes to the severity of cardiovascular diseases. Both neuroendocrine and autonomic PVN, have a dynamic part in adjusting the circulation to physiological demands and in the modulation of short-term BPV. Thus, elucidating the tightly intertwined mechanisms underlying complexity of the PVN network in health and disease may open up new therapeutic venues.
BS, DM, and NJ-Ž: outlining paper draft, writing and refining the manuscript, and critical reading of the manuscript. All of the authors have read and approved the manuscript.
This work was supported by Serbian Ministry of Education, Science and Technological Development (no. 200110, BS, NJ-Ž), British Heart Foundation (RG/11/28714, DM; FS/12/5/29339, DM), and BBSRC (BB/J005452/1, DM).
The authors declare that the research was conducted in the absence of any commercial or financial relationships that could be construed as a potential conflict of interest.
All claims expressed in this article are solely those of the authors and do not necessarily represent those of their affiliated organizations, or those of the publisher, the editors and the reviewers. Any product that may be evaluated in this article, or claim that may be made by its manufacturer, is not guaranteed or endorsed by the publisher.
ACTH, Adrenocorticotropic hormone; ADH, Antidiuretic hormone; ANP, Atrial natriuretic peptide; AP, Area postrema; AQP-2, Aquaporin 2; AT1R, Angiotensin II receptor type 1; AV3V, Anteroventral third ventricle; BHR, Borderline hypertensive rats; BPV, BP variability; BP, Blood pressure; BRS, Baroreflex sensitivity; CRH, Corticotropin-releasing hormone; CRHR2, corticotropin-releasing hormone receptor; DMV, dorsal motor nucleus of vagus; GABA, γ; GPCRs, G protein-coupled receptors; HF, High frequency; HPA, Hypothalamo–hypophyseal axis; HRV, Heart rate variability; IML, Intermediolateral nucleus; LF, Low frequency; MCNs, Magnocellular neurons; MnPO, Medial preoptic nucleus; NO, Nitrogen oxide; NTS, Nucleus tractus solitarius; OT, Oxytocin; OTR, Oxytocin receptor; OVLT, Organum vasculosum laminae terminalis; PCNs, Parvocelular neuron; PVN, Paraventricular nucleus; RVLM, Rostral ventrolateral medulla; SFO, Circumventricular subfornical organ; SHR, Spontaneously hypertensive rats; SND, Sympathetic nerve discharge; SPANs, Spinally projecting pre-utonimic neurons; VLF, Very low frequency; VLM, Ventrolateral medulla; VP, Vasopressin; VR, Vasopressin receptor.
Abboud, F. M., Floras, J. S., Aylward, P. E., Guo, G. B., Gupta, B. N., and Schmid, P. G. (1990). Role of vasopressin in cardiovascular and blood pressure regulation. J. Vasc. Res. 27, 106–115. doi: 10.1159/000158801
Adan, R. A., Van Leeuwen, F. W., Sonnemans, M. A., Brouns, M., Hoffman, G., Verbalis, J. G., et al. (1995). Rat oxytocin receptor in brain, pituitary, mammary gland, and uterus: partial sequence and immunocytochemical localization. Endocrinology 136, 4022–4028. doi: 10.1210/endo.136.9.7649111
Aguilera, G. (1994). Regulation of pituitary ACTH secretion during chronic stress. Front. Neuroendocrinol. 15, 321–350. doi: 10.1006/frne.1994.1013
Aki, Y., Tamaki, T., Kiyomoto, H., He, H., Yoshida, H., Iwao, H., et al. (1994). Nitric oxide may participate in V2 vasopressin-receptor-mediated renal vasodilation. J. Cardiovasc. Pharmacol. 23, 331–336. doi: 10.1097/00005344-199402000-00023
Allen, A. M. (2002). Inhibition of the hypothalamic paraventricular nucleus in spontaneously hypertensive rats dramatically reduces sympathetic vasomotor tone. Hypertension 39, 275–280. doi: 10.1161/hy0202.104272
Altemus, M., Redwine, L. S., Leong, Y. M., Frye, C. A., Porges, S. W., and Carter, C. S. (2001). Responses to laboratory psychosocial stress in postpartum women. Psychosom. Med. 63, 814–821. doi: 10.1097/00006842-200109000-00015
Altura, B. M., and Altura, B. T. (1984). Actions of vasopressin, oxytocin, and synthetic analogs on vascular smooth muscle. Fed. Proc. 43, 80–86.
Amaya, F., Tanaka, M., Hayashi, S., Tanaka, Y., and Ibata, Y. (2001). Hypothalamo–pituitary–adrenal axis sensitization after chronic salt loading. Neuroendocrinology 73, 185–193. doi: 10.1159/000054635
Armstrong, W. E., Warach, S., Hatton, G. I., and McNeill, T. H. (1980). Subnuclei in the rat hypothalamic paraventricular nucleus: a cytoarchitectural, horseradish peroxidase and immunocytochemical analysis. Neuroscience 5, 1931–1958. doi: 10.1016/0306-4522(80)90040-8
Badoer, E. (1996). Cardiovascular role of parvocellular neurons in the paraventricular nucleus of the hypothalamus. Physiology 11, 43–47. doi: 10.1152/physiologyonline.1996.11.1.43
Badoer, E. (2001). Proceedings of the Australian physiological and pharmacological society symposium: the hypothalamus, hypothalamic paraventricular nucleus and cardiovascular regulation. Clin. Exp. Pharmacol. Physiol. 28, 95–99. doi: 10.1046/j.1440-1681.2001.03413.x
Badoer, E., Ng, C.-W., and De Matteo, R. (2002). Tonic sympathoinhibition arising from the hypothalamic PVN in the conscious rabbit. Brain Res. 947, 17–24. doi: 10.1016/S0006-8993(02)02901-3
Bains, J. S., and Ferguson, A. V. (1995). Paraventricular nucleus neurons projecting to the spinal cord receive excitatory input from the subfornical organ. Am. J. Phys. 268, R625–R633.
Bains, J. S., Potyok, A., and Ferguson, A. V. (1992). Angiotensin II actions in paraventricular nucleus: functional evidence for neurotransmitter role in efferents originating in subfornical organ. Brain Res. 599, 223–229. doi: 10.1016/0006-8993(92)90395-P
Bankir, L. (2001). Antidiuretic action of vasopressin: quantitative aspects and interaction between V1a and V2 receptor–mediated effects. Cardiovasc. Res. 51, 372–390. doi: 10.1016/S0008-6363(01)00328-5
Barberis, C., Mouillac, B., and Durroux, T. (1998). Structural bases of vasopressin/oxytocin receptor function. J. Endocrinol. 156, 223–229. doi: 10.1677/joe.0.1560223
Barman, S. M., and Gebber, G. L. (2000). “Rapid” rhythmic discharges of sympathetic nerves: sources, mechanisms of generation, and physiological relevance. J. Biol. Rhythm. 15, 365–379. doi: 10.1177/074873000129001468
Benarroch, E. E. (2005). Paraventricular nucleus, stress response, and cardiovascular disease. Clin. Auton. Res. 15, 254–263. doi: 10.1007/s10286-005-0290-7
Bernatova, I., Rigatto, K. V., Key, M. P., and Morris, M. (2004). Stress-induced pressor and corticosterone responses in oxytocin-deficient mice. Exp. Physiol. 89, 549–557. doi: 10.1113/expphysiol.2004.027714
Bisset, G. W., and Chowdrey, H. S. (1988). Control of release of vasopressin by neuroendocrine reflexes. Q. J. Exp. Physiol. 73, 811–872. doi: 10.1113/expphysiol.1988.sp003223
Bohus, B., and de Wied, D. (1998). The vasopressin deficient Brattleboro rats: a natural knockout model used in the search for CNS effects of vasopressin. Prog. Brain Res. 119, 555–573. doi: 10.1016/s0079-6123(08)61593-9
Bosch, O. J., Krömer, S. A., Brunton, P. J., and Neumann, I. D. (2004). Release of oxytocin in the hypothalamic paraventricular nucleus, but not central amygdala or lateral septum in lactating residents and virgin intruders during maternal defence. Neuroscience 124, 439–448. doi: 10.1016/j.neuroscience.2003.11.028
Brickley, S. G., Cull-Candy, S. G., and Farrant, M. (1996). Development of a tonic form of synaptic inhibition in rat cerebellar granule cells resulting from persistent activation of GABAA receptors. J. Physiol. 497, 753–759. doi: 10.1113/jphysiol.1996.sp021806
Brinton, R. E., Gee, K. W., Wamsley, J. K., Davis, T. P., and Yamamura, H. I. (1984). Regional distribution of putative vasopressin receptors in rat brain and pituitary by quantitative autoradiography. Proc. Natl. Acad. Sci. U. S. A. 81, 7248–7252.
Brizzee, B. L., and Walker, B. R. (1990). Vasopressinergic augmentation of cardiac baroreceptor reflex in conscious rats. Am. J. Phys. 258, R860–R868.
Carette, B., and Poulain, P. (1989). Vasopressin-sensitive neurons in the lateral paraventricular nucleus area in a guinea pig slice preparation. Brain Res. Bull. 22, 969–974. doi: 10.1016/0361-9230(89)90008-7
Carrive, P., and Gorissen, M. (2008). Premotor sympathetic neurons of conditioned fear in the rat. Eur. J. Neurosci. 28, 428–446. doi: 10.1111/j.1460-9568.2008.06351.x
Cato, M. J., and Toney, G. M. (2005). Angiotensin II excites paraventricular nucleus neurons that innervate the rostral ventrolateral medulla: an in vitro patch-clamp study in brain slices. J. Neurophysiol. 93, 403–413. doi: 10.1152/jn.01055.2003
Cechetto, D. F., and Saper, C. B. (1988). Neurochemical organization of the hypothalamic projection to the spinal cord in the rat. J. Comp. Neurol. 272, 579–604. doi: 10.1002/cne.902720410
Conrad, K. P., Gellai, M., North, W. G., and Valtin, H. (1993). Influence of oxytocin on renal hemodynamics and sodium excretion. Ann. N. Y. Acad. Sci. 689, 346–362. doi: 10.1111/j.1749-6632.1993.tb55559.x
Coote, J. H. (2005). A role for the paraventricular nucleus of the hypothalamus in the autonomic control of heart and kidney. Exp. Physiol. 90, 169–173. doi: 10.1113/expphysiol.2004.029041
Coote, J. H. (2007). Landmarks in understanding the central nervous control of the cardiovascular system. Exp. Physiol. 92, 3–18. doi: 10.1113/expphysiol.2006.035378
Coote, J. H., Yang, Z., Pyner, S., and Deering, J. (1998). Control of sympathetic outflows by the hypothalamic paraventricular nucleus. Clin. Exp. Pharmacol. Physiol. 25, 461–463. doi: 10.1111/j.1440-1681.1998.tb02235.x
Cui, L. N., Coderre, E., and Renaud, L. P. (2001). Glutamate and GABA mediate suprachiasmatic nucleus inputs to spinal-projecting paraventricular neurons. Am. J. Phys. Regul. Integr. Comp. Phys. 281, R1283–R1289. doi: 10.1152/ajpregu.2001.281.4.R1283
Cunningham, E. T., and Sawchenko, P. E. (1991). Reflex control of magnocellular vasopressin and oxytocin secretion. Trends Neurosci. 14, 406–411. doi: 10.1016/0166-2236(91)90032-P
Dale, H. H. (1906). On some physiological actions of ergot. J. Physiol. 34, 163–206. doi: 10.1113/jphysiol.1906.sp001148
Dampney, R. A. (1994). Functional organization of central pathways regulating the cardiovascular system. Physiol. Rev. 74, 323–364. doi: 10.1152/physrev.1994.74.2.323
Dampney, R. A. L. (2017). Resetting of the baroreflex control of sympathetic vasomotor activity during natural behaviors: description and conceptual model of central mechanisms. Front. Neurosci. 11:461.
Dampney, R. A., Michelini, L. C., Li, D.-P., and Pan, H.-L. (2018). Regulation of sympathetic vasomotor activity by the hypothalamic paraventricular nucleus in normotensive and hypertensive states. Am. J. Physiol. Heart Circ. Physiol. 315, H1200–H1214. doi: 10.1152/ajpheart.00216.2018
Dayanithi, G., Sabatier, N., and Widmer, H. (2000). Intracellular calcium signalling in magnocellular neurones of the rat supraoptic nucleus: understanding the autoregulatory mechanisms. Exp. Physiol. 85, 75S–84S.
De Bree, F. M., Van Der Kleij, A. A. M., Nijenhuis, M., Zalm, R., Murphy, D., and Burbach, J. P. H. (2003). The hormone domain of the vasopressin prohormone is required for the correct prohormone trafficking through the secretory pathway. J. Neuroendocrinol. 15, 1156–1163. doi: 10.1111/j.1365-2826.2003.01114.x
de Goeij, D. C. E., Jezova, D., and Tilders, F. J. H. (1992). Repeated stress enhances vasopressin synthesis in corticotropin releasing factor neurons in the paraventricular nucleus. Brain Res. 577, 165–168. doi: 10.1016/0006-8993(92)90552-K
Deng, Y., and Kaufman, S. (1995). Effect of pregnancy on activation of central pathways following atrial distension. Am. J. Phys. Regul. Integr. Comp. Phys. 269, R552–R556. doi: 10.1152/ajpregu.1995.269.3.R552
Desaulles, E., Reiter, M. K., and Feltz, P. (1995). Electrophysiological evidence for oxytocin receptors on sympathetic preganglionic neurones – an in vitro study on the neonatal rat. Brain Res. 699, 139–142. doi: 10.1016/0006-8993(95)01019-R
Dierickx, K. (1980). Immunocytochemical localization of the vertebrate cyclic nonapeptide neurohypophyseal hormones and neurophysins. Int. Rev. Cytol. 62, 119–185.
Du Vigneaud, V. (1954). Hormones of the posterior pituitary gland: oxytocin and vasopressin. Harvey Lect. 50, 1–26.
Ebner, K., Wotjak, C. T., Landgraf, R., and Engelmann, M. (2005). Neuroendocrine and behavioral response to social confrontation: residents versus intruders, active versus passive coping styles. Horm. Behav. 47, 14–21. doi: 10.1016/j.yhbeh.2004.08.002
El-Werfali, W., Toomasian, C., Maliszewska-Scislo, M., Li, C., and Rossi, N. F. (2015). Haemodynamic and renal sympathetic responses to V1b vasopressin receptor activation within the paraventricular nucleus. Exp. Physiol. 100, 553–565. doi: 10.1113/expphysiol.2014.084426
Engelmann, M., Ebner, K., Landgraf, R., Holsboer, F., and Wotjak, C. T. (2001). Emotional stress triggers intrahypothalamic but not peripheral release of oxytocin in male rats. J. Neuroendocrinol. 11, 867–872. doi: 10.1046/j.1365-2826.1999.00403.x
Farrant, M., and Nusser, Z. (2005). Variations on an inhibitory theme: phasic and tonic activation of GABAA receptors. Nat. Rev. Neurosci. 6, 215–229. doi: 10.1038/nrn1625
Fritschy, J.-M., and Mohler, H. (1995). GABAA-receptor heterogeneity in the adult rat brain: differential regional and cellular distribution of seven major subunits. J. Comp. Neurol. 359, 154–194. doi: 10.1002/cne.903590111
Gimpl, G., and Fahrenholz, F. (2001). The oxytocin receptor system: structure, function, and regulation. Physiol. Rev. 81, 629–683. doi: 10.1152/physrev.2001.81.2.629
Gladwell, S. J., and Coote, J. H. (1999a). Fast excitatory post synaptic potentials and their response to catecholaminergic antagonists in rat sympathetic preganglionic neurones in vitro. Neurosci. Lett. 268, 89–92.
Gladwell, S. J., and Coote, J. H. (1999b). Inhibitory and indirect excitatory effects of dopamine on sympathetic preganglionic neurones in the neonatal rat spinal cord in vitro. Brain Res. 818, 397–407.
Gladwell, S. J., Pyner, S., Barnes, N. M., and Coote, J. H. (1999). D(1)-like dopamine receptors on retrogradely labelled sympathoadrenal neurones in the thoracic spinal cord of the rat. Exp. Brain Res. 128, 377–382. doi: 10.1007/s002210050857
Gordan, R., Gwathmey, J. K., and Xie, L.-H. (2015). Autonomic and endocrine control of cardiovascular function. World J. Cardiol. 7, 204–214. doi: 10.4330/wjc.v7.i4.204
Gouzènes, L., Desarménien, M. G., Hussy, N., Richard, P., and Moos, F. C. (1998). Vasopressin regularizes the phasic firing pattern of rat hypothalamic magnocellular vasopressin neurons. J. Neurosci. 18, 1879–1885. doi: 10.1523/JNEUROSCI.18-05-01879.1998
Griebel, G., Simiand, J., Serradeil-Le Gal, C., Wagnon, J., Pascal, M., Scatton, B., et al. (2002). Anxiolytic- and antidepressant-like effects of the non-peptide vasopressin V1b receptor antagonist, SSR149415, suggest an innovative approach for the treatment of stress-related disorders. Proc. Natl. Acad. Sci. U. S. A. 99, 6370–6375. doi: 10.1073/pnas.092012099
Gupta, P. D., Henry, J. P., Sinclair, R., and Von Baumgarten, R. (1966). Responses of atrial and aortic baroreceptors to nonhypotensive hemorrhage and to tranfusion. Am. J. Phys. 211, 1429–1437.
Gutkowska, J., Jankowski, M., Lambert, C., Mukaddam-Daher, S., Zingg, H. H., and McCann, S. M. (1997). Oxytocin releases atrial natriuretic peptide by combining with oxytocin receptors in the heart. Proc. Natl. Acad. Sci. U.S.A. 94, 11704–11709.
Gutkowska, J., Jankowski, M., Mukaddam-Daher, S., and McCann, S. M. (2000). Oxytocin is a cardiovascular hormone. Braz. J. Med. Biol. Res. 33, 625–633. doi: 10.1590/S0100-879X2000000600003
Hallbeck, M. (2000). Dynorphin mRNA-expressing neurons in the rat paraventricular hypothalamic nucleus project to the spinal cord. Neurosci. Lett. 285, 161–164. doi: 10.1016/S0304-3940(00)01093-4
Hallbeck, M., and Blomqvist, A. (1999). Spinal cord-projecting vasopressinergic neurons in the rat paraventricular hypothalamus. J. Comp. Neurol. 411, 201–211. doi: 10.1002/(SICI)1096-9861(19990823)411:2<201::AID-CNE3>3.0.CO;2-3
Hallbeck, M., Larhammar, D., and Blomqvist, A. (2001). Neuropeptide expression in rat paraventricular hypothalamic neurons that project to the spinal cord. J. Comp. Neurol. 433, 222–238. doi: 10.1002/cne.1137
Haselton, J. R., Goering, J., and Patel, K. P. (1994). Parvocellular neurons of the paraventricular nucleus are involved in the reduction in renal nerve discharge during isotonic volume expansion. J. Auton. Nerv. Syst. 50, 1–11. doi: 10.1016/0165-1838(94)9017-1
Hasser, E. M., and Bishop, V. S. (1990). Reflex effect of vasopressin after blockade of V1 receptors in the area postrema. Circ. Res. 67, 265–271. doi: 10.1161/01.RES.67.2.265
Hasser, E. M., Bishop, V. S., and Hay, M. (1997). Interactions between vasopressin and baroreflex control of the sympathetic nervous system. Clin. Exp. Pharmacol. Physiol. 24, 102–108. doi: 10.1111/j.1440-1681.1997.tb01791.x
Head, R. J. (1991). Hypernoradrenergic innervation and vascular smooth muscle hyperplastic change. Blood Vessels 28, 173–178. doi: 10.1159/000158858
Heinrichs, M., Neumann, I., and Ehlert, U. (2002). Lactation and stress: protective effects of breast-feeding in humans. Stress 5, 195–203. doi: 10.1080/1025389021000010530
Herman, J. P. -F., Prewitt, C. M., and Cullinan, W. E. (1996). Neuronal circuit regulation of the hypothalamo–pituitary–adrenocortical stress axis. Crit. Rev. Neurobiol. 10, 371–394. doi: 10.1615/CritRevNeurobiol.v10.i3-4.50
Hirasawa, A., Hashimoto, K., and Tsujimoto, G. (1994). Distribution and developmental change of vasopressin V1A and V2 receptor mRNA in rats. Eur. J. Pharmacol. Mol. Pharmacol. 267, 71–75. doi: 10.1016/0922-4106(94)90226-7
Hirsch, A. T., Dzau, V. J., Majzoub, J. A., and Creager, M. A. (1989). Vasopressin-mediated forearm vasodilation in normal humans. Evidence for a vascular vasopressin V2 receptor. J. Clin. Invest. 84, 418–426. doi: 10.1172/JCI114182
Hjemdahl, P. (2002). Stress and the metabolic syndrome: an interesting but enigmatic association. Circulation 106, 2634–2636. doi: 10.1161/01.CIR.0000041502.43564.79
Huikuri, H. V., and Stein, P. K. (2013). Heart rate variability in risk stratification of cardiac patients. Prog. Cardiovasc. Dis. 56, 153–159. doi: 10.1016/j.pcad.2013.07.003
Hurbin, A., Boissin-Agasse, L., Orcel, H., Rabié, A., Joux, N., Desarménien, M. G., et al. (1998). The V1a and V1b, but not V2, vasopressin receptor genes are expressed in the supraoptic nucleus of the rat hypothalamus, and the transcripts are essentially colocalized in the vasopressinergic magnocellular neurons. Endocrinology 139, 4701–4707. doi: 10.1210/endo.139.11.6320
Hurbin, A., Orcel, H., Alonso, G., Moos, F., and Rabié, A. (2002). The vasopressin receptors colocalize with vasopressin in the magnocellular neurons of the rat supraoptic nucleus and are modulated by water balance. Endocrinology 143, 456–466. doi: 10.1210/endo.143.2.8643
Imai, Y., Nolan, P. L., and Johnston, C. I. (1983a). Endogenous vasopressin modulates the baroreflex sensitivity in rats. Clin. Exp. Pharmacol. Physiol. 10, 289–292. doi: 10.1111/j.1440-1681.1983.tb00199.x
Imai, Y., Nolan, P. L., and Johnston, C. I. (1983b). Restoration of suppressed baroreflex sensitivity in rats with hereditary diabetes insipidus (Brattleboro rats) by arginine-vasopressin and DDAVP. Circ. Res. 53, 140–149.
Imbert, M., Chabardes, D., Montegut, M., Clique, A., and Morel, F. (1975). Vasopressin dependent adenylate cyclase in single segments of rabbit kidney tubule. Pflugers Arch. 357, 173–186. doi: 10.1007/BF00585973
Inenaga, K., and Yamashita, H. (1986). Excitation of neurones in the rat paraventricular nucleus in vitro by vasopressin and oxytocin. J. Physiol. 370, 165–180. doi: 10.1113/jphysiol.1986.sp015928
Jansen, A. S., Nguyen, X. V., Karpitskiy, V., Mettenleiter, T. C., and Loewy, A. D. (1995a). Central command neurons of the sympathetic nervous system: basis of the fight-or-flight response. Science 270, 644–646.
Jansen, A. S. P., Wessendorf, M. W., and Loewy, A. D. (1995b). Transneuronal labeling of CNS neuropeptide and monoamine neurons after pseudorabies virus injections into the stellate ganglion. Brain Res. 683, 1–24. doi: 10.1016/0006-8993(95)00276-v
Janssen, B. J., Oosting, J., Slaaf, D. W., Persson, P. B., and Struijker-Boudier, H. A. (1995). Hemodynamic basis of oscillations in systemic arterial pressure in conscious rats. Am. J. Phys. 269, H62–H71.
Japundzic, N., Grichois, M. L., Zitoun, P., Laude, D., and Elghozi, J. L. (1990). Spectral analysis of blood pressure and heart rate in conscious rats: effects of autonomic blockers. J. Auton. Nerv. Syst. 30, 91–100.
Japundzic-Zigon, N. (1998). Physiological mechanisms in regulation of blood pressure fast frequency variations. Clin. Exp. Hypertens. 20, 359–388.
Japundzic-Zigon, N. (2001). Effects of nonpeptide V1a and V2 antagonists on blood pressure fast oscillations in conscious rats. Clin. Exp. Hypertens. 23, 277–292. doi: 10.1081/CEH-100102667
Japundžić-Žigon, N. (2013). Vasopressin and oxytocin in control of the cardiovascular system. Curr. Neuropharmacol. 11, 218–230. doi: 10.2174/1570159X11311020008
Japundzic-Zigon, N. (2013). Vasopressin and oxytocin in control of the cardiovascular system. Curr. Neuropharmacol. 11, 218–230. doi: 10.2174/1570159X11311020008
Japundžić-Žigon, N., Lozić, M., Šarenac, O., and Murphy, D. (2020). Vasopressin & oxytocin in control of the cardiovascular system: an updated review. Curr. Neuropharmacol. 18, 14–33. doi: 10.2174/1570159X17666190717150501
Japundzić-Zigon, N., Milutinović, S., and Jovanović, A. (2004). Effects of nonpeptide and selective V1 and V2 antagonists on blood pressure short-term variability in spontaneously hypertensive rats. J. Pharmacol. Sci. 95, 47–55. doi: 10.1254/jphs.95.47
Japundžić-Žigon, N., Šarenac, O., Lozić, M., Vasić, M., Tasić, T., Bajić, D., et al. (2018). Sudden death: neurogenic causes, prediction and prevention. Eur. J. Prev. Cardiol. 25, 29–39. doi: 10.1177/2047487317736827
Jhamandas, J. H., and Renaud, L. P. (1987). Neurophysiology of a central baroreceptor pathway projecting to hypothalamic vasopressin neurons. Can. J. Neurol. Sci. 14, 17–24. doi: 10.1017/S0317167100026111
Johnston, C. I. (1985). Vasopressin in circulatory control and hypertension. J. Hypertens. 3, 557–569. doi: 10.1097/00004872-198512000-00001
Julien, C., Malpas, S. C., and Stauss, H. M. (2001). Sympathetic modulation of blood pressure variability. J. Hypertens. 19, 1707–1712. doi: 10.1097/00004872-200110000-00002
Jung, H. J., and Kwon, T.-H. (2016). Molecular mechanisms regulating aquaporin-2 in kidney collecting duct. Am. J. Physiol. Ren. Physiol. 311, F1318–F1328. doi: 10.1152/ajprenal.00485.2016
Kadekaro, M., Summy-Long, J. Y., Freeman, S., Harris, J. S., Terrell, M. L., and Eisenberg, H. M. (1992). Cerebral metabolic responses and vasopressin and oxytocin secretions during progressive water deprivation in rats. Am. J. Phys. 262, R310–R317. doi: 10.1152/ajpregu.1992.262.2.R310
Kannan, H., Hayashida, Y., and Yamashita, H. (1989). Increase in sympathetic outflow by paraventricular nucleus stimulation in awake rats. Am. J. Phys. 256, R1325–R1330. doi: 10.1152/ajpregu.1989.256.6.R1325
Kato, Y., Igarashi, N., Hirasawa, A., Tsujimoto, G., and Kobayashi, M. (1995). Distribution and developmental changes in vasopressin V2 receptor mRNA in rat brain. Differentiation 59, 163–169. doi: 10.1046/j.1432-0436.1995.5930163.x
Katusic, Z. S., Shepherd, J. T., and Vanhoutte, P. M. (1986). Oxytocin causes endothelium-dependent relaxations of canine basilar arteries by activating V1-vasopressinergic receptors. J. Pharmacol. Exp. Ther. 236, 166–170.
Kawabe, T., Chitravanshi, V. C., Kawabe, K., and Sapru, H. N. (2008). Cardiovascular function of a glutamatergic projection from the hypothalamic paraventricular nucleus to the nucleus tractus solitarius in the rat. Neuroscience 153, 605–617. doi: 10.1016/j.neuroscience.2008.02.076
Kawabe, T., Chitravanshi, V. C., Nakamura, T., Kawabe, K., and Sapru, H. N. (2009). Mechanism of heart rate responses elicited by chemical stimulation of the hypothalamic paraventricular nucleus in the rat. Brain Res. 1248, 115–126. doi: 10.1016/j.brainres.2008.10.059
Kelly, J. J., Mangos, G., Williamson, P. M., and Whitworth, J. A. (1998). Cortisol and hypertension. Clin. Exp. Pharmacol. Physiol. 25, S51–S56. doi: 10.1111/j.1440-1681.1998.tb02301.x
Kendrick, K. M., Da Costa, A. P. C., Broad, K. D., Ohkura, S., Guevara, R., Lévy, F., et al. (1997). Neural control of maternal behaviour and olfactory recognition of offspring. Brain Res. Bull. 44, 383–395. doi: 10.1016/S0361-9230(97)00218-9
Knepper, M. A. (1997). Molecular physiology of urinary concentrating mechanism: regulation of aquaporin water channels by vasopressin. Am. J. Physiol. Renal Physiol. 272, F3–F12. doi: 10.1152/ajprenal.1997.272.1.F3
Koshimizu, T.-A., Nakamura, K., Egashira, N., Hiroyama, M., Nonoguchi, H., and Tanoue, A. (2012). Vasopressin V1a and V1b receptors: from molecules to physiological systems. Physiol. Rev. 92, 1813–1864. doi: 10.1152/physrev.00035.2011
Koshimizu, T.-A., Nasa, Y., Tanoue, A., Oikawa, R., Kawahara, Y., Kiyono, Y., et al. (2006). V1a vasopressin receptors maintain normal blood pressure by regulating circulating blood volume and baroreflex sensitivity. Proc. Natl. Acad. Sci. U. S. A. 103, 7807–7812. doi: 10.1073/pnas.0600875103
Landgraf, R., and Neumann, I. D. (2004). Vasopressin and oxytocin release within the brain: a dynamic concept of multiple and variable modes of neuropeptide communication. Front. Neuroendocrinol. 25, 150–176. doi: 10.1016/j.yfrne.2004.05.001
Leng, G., Blackburn, R. E., Dyball, R. E., and Russell, J. A. (1989). Role of anterior peri-third ventricular structures in the regulation of supraoptic neuronal activity and neurohypophysical hormone secretion in the rat. J. Neuroendocrinol. 1, 35–46. doi: 10.1111/j.1365-2826.1989.tb00074.x
Leng, G., Brown, C. H., Bull, P. M., Brown, D., Scullion, S., Currie, J., et al. (2001). Responses of magnocellular neurons to osmotic stimulation involves coactivation of excitatory and inhibitory input: an experimental and theoretical analysis. J. Neurosci. 21, 6967–6977. doi: 10.1523/JNEUROSCI.21-17-06967.2001
Leng, G., Brown, C. H., and Russell, J. A. (1999). Physiological pathways regulating the activity of magnocellular neurosecretory cells. Prog. Neurobiol. 57, 625–655. doi: 10.1016/S0301-0082(98)00072-0
Levy, B., Fritz, C., Tahon, E., Jacquot, A., Auchet, T., and Kimmoun, A. (2018). Vasoplegia treatments: the past, the present, and the future. Crit. Care 22:52. doi: 10.1186/s13054-018-1967-3
Li, D.-P., Chen, S.-R., and Pan, H.-L. (2002). Nitric oxide inhibits spinally projecting paraventricular neurons through potentiation of presynaptic GABA release. J. Neurophysiol. 88, 2664–2674. doi: 10.1152/jn.00540.2002
Li, D.-P., Chen, S.-R., and Pan, H.-L. (2003). Angiotensin II stimulates spinally projecting paraventricular neurons through presynaptic disinhibition. J. Neurosci. 23, 5041–5049. doi: 10.1523/JNEUROSCI.23-12-05041.2003
Li, Y. W., and Guyenet, P. G. (1996). Activation of GABAB receptors increases a potassium conductance in rat bulbospinal neurons of the C1 area. Am. J. Phys. Regul. Integr. Comp. Phys. 271, R1304–R1310. doi: 10.1152/ajpregu.1996.271.5.R1304
Li, Y.-F., Jackson, K. L., Stern, J. E., Rabeler, B., and Patel, K. P. (2006). Interaction between glutamate and GABA systems in the integration of sympathetic outflow by the paraventricular nucleus of the hypothalamus. Am. J. Physiol. Heart Circ. Physiol. 291, H2847–H2856. doi: 10.1152/ajpheart.00625.2005
Liard, J. F. (1984). Vasopressin in cardiovascular control: role of circulating vasopressin. Clin. Sci. 67, 473–481. doi: 10.1042/cs0670473
Lipski, J., Kanjhan, R., Kruszewska, B., and Rong, W. (1996). Properties of presympathetic neurones in the rostral ventrolateral medulla in the rat: an intracellular study “in vivo”. J. Physiol. 490, 729–744. doi: 10.1113/jphysiol.1996.sp021181
Lovick, T. A., and Coote, J. H. (1988). Effects of volume loading on paraventriculo-spinal neurones in the rat. J. Auton. Nerv. Syst. 25, 135–140.
Lovick, T. A., Malpas, S., and Mahony, M. T. (1993). Renal vasodilatation in response to acute volume load is attenuated following lesions of parvocellular neurones in the paraventricular nucleus in rats. J. Auton. Nerv. Syst. 43, 247–255.
Lozić, M., Greenwood, M., Šarenac, O., Martin, A., Hindmarch, C., Tasić, T., et al. (2014). Overexpression of oxytocin receptors in the hypothalamic PVN increases baroreceptor reflex sensitivity and buffers BP variability in conscious rats. Br. J. Pharmacol. 171, 4385–4398. doi: 10.1111/bph.12776
Lozić, M., Tasić, T., Martin, A., Greenwood, M., Šarenac, O., Hindmarch, C., et al. (2016). Over-expression of V1A receptors in PVN modulates autonomic cardiovascular control. Pharmacol. Res. 114, 185–195. doi: 10.1016/j.phrs.2016.10.024
Ludwig, M., Callahan, M. F., and Morris, M. (1995). Effects of tetrodotoxin on osmotically stimulated central and peripheral vasopressin and oxytocin release. Neuroendocrinology 62, 619–627.
Ludwig, M., Sabatier, N., Bull, P. M., Landgraf, R., Dayanithi, G., and Leng, G. (2002). Intracellular calcium stores regulate activity-dependent neuropeptide release from dendrites. Nature 418, 85–89. doi: 10.1038/nature00822
Ludwig, M., and Stern, J. (2015). Multiple signalling modalities mediated by dendritic exocytosis of oxytocin and vasopressin. Philos. Trans. R. Soc. Lond. Ser. B Biol. Sci. 370. doi: 10.1098/rstb.2014.0182
Macdonald, R. L., and Olsen, R. W. (1994). GABAA receptor channels. Annu. Rev. Neurosci. 17, 569–602. doi: 10.1146/annurev.ne.17.030194.003033
Maier, T., Dai, W. J., Csikós, T., Jirikowski, G. F., Unger, T., and Culman, J. (1998). Oxytocin pathways mediate the cardiovascular and behavioral responses to substance P in the rat brain. Hypertension 31, 480–486. doi: 10.1161/01.HYP.31.1.480
Malpas, S. C. (1998). The rhythmicity of sympathetic nerve activity. Prog. Neurobiol. 56, 65–96. doi: 10.1016/S0301-0082(98)00030-6
Malpas, S. C., and Coote, J. H. (1994). Role of vasopressin in sympathetic response to paraventricular nucleus stimulation in anesthetized rats. Am. J. Phys. 266, R228–R236. doi: 10.1152/ajpregu.1994.266.1.R228
Malpas, S. C., and Ninomiya, I. (1992). Effect of asphyxia on the frequency and amplitude modulation of synchronized renal nerve activity in the cat. J. Auton. Nerv. Syst. 40, 199–205.
Mancia, G., Frattola, G., Parati, C., Santuccin, C., and Ulian, L. (1994). Blood pressure variability and organ damage. J. Cardiovasc. Pharmacol. 24, S6–S11.
Mancia, G., and Grassi, G. (2000). Mechanisms and Clinical Implications of Blood Pressure Variability. J. Cardiovasc. Pharmacol. 21, S15–S19. doi: 10.1097/00005344-200000004-00003
Mancia, G., and Parati, G. (2003). The role of blood pressure variability in end-organ damage. J. Hypertens. 21, S17–S23. doi: 10.1097/00004872-200307006-00004
Martin, D. S., and Haywood, J. R. (1993). Hemodynamic responses to paraventricular nucleus disinhibition with bicuculline in conscious rats. Am. J. Phys. 265, H1727–H1733. doi: 10.1152/ajpheart.1993.265.5.H1727
Martin, D. S., Segura, T., and Haywood, J. R. (1991). Cardiovascular responses to bicuculline in the paraventricular nucleus of the rat. Hypertension 18, 48–55. doi: 10.1161/01.HYP.18.1.48
McKinley, M. J., Mathai, M. L., McAllen, R. M., McClear, R. C., Miselis, R. R., Pennington, G. L., et al. (2004). Vasopressin secretion: osmotic and hormonal regulation by the lamina terminalis. J. Neuroendocrinol. 16, 340–347. doi: 10.1111/j.0953-8194.2004.01184.x
Messerli, F. H., Hofstetter, L., Rimoldi, S. F., Rexhaj, E., and Bangalore, S.. (2019). Risk factor variability and cardiovascular outcome: JACC review topic of the week. J. Am. Coll. Cardiol. 73, 2596–2603. doi: 10.1016/j.jacc.2019.02.063
Miller, M. E., Davidge, S. T., and Mitchell, B. F. (2002). Oxytocin does not directly affect vascular tone in vessels from nonpregnant and pregnant rats. Am. J. Phys. Heart Circ. Phys. 282, H1223–H1228. doi: 10.1152/ajpheart.00774.2001
Milutinović, S., Murphy, D., and Japundžić-Žigon, N. (2006a). Central cholinergic modulation of blood pressure short-term variability. Neuropharmacology 50, 874–883. doi: 10.1016/j.neuropharm.2005.12.009
Milutinović, S., Murphy, D., and Japundžić-Žigon, N. (2006b). The role of central vasopressin receptors in the modulation of autonomic cardiovascular controls: a spectral analysis study. Am. J. Phys. Regul. Integr. Comp. Phys. 291, R1579–R1591. doi: 10.1152/ajpregu.00764.2005
Milutinović-Smiljanić, S., Šarenac, O., Lozić-Djurić, M., Murphy, D., and Japundžić-Žigon, N. (2013). Evidence for involvement of central vasopressin V1b and V2 receptors in stress-induced baroreflex desensitization. Br. J. Pharmacol. 169, 900–908. doi: 10.1111/bph.12161
Moos, F., Gouzènes, L., Brown, D., Dayanithi, G., Sabatier, N., Boissin, L., et al. (1998). New aspects of firing pattern autocontrol in oxytocin and vasopressin neurones. Adv. Exp. Med. Biol. 449, 153–162.
Moos, F., Poulain, D. A., Rodriguez, F., Guerne, Y., Vincent, J.-D., and Richard, P. (1989). Release of oxytocin within the supraoptic nucleus during the milk ejection reflex in rats. Exp. Brain Res. 76, 593–602. doi: 10.1007/BF00248916
Myers, B., McKlveen, J. M., and Herman, J. P. (2012). Neural regulation of the stress response: the many faces of feedback. Cell. Mol. Neurobiol. doi: 10.1007/s10571-012-9801-y
Neumann, I. D. (2002). Involvement of the brain oxytocin system in stress coping: interactions with the hypothalamo–pituitary–adrenal axis. Prog. Brain Res. 139, 147–162. doi: 10.1016/s0079-6123(02)39014-9
Neumann, I., Ludwig, M., Engelmann, M., Pittman, Q. J., and Landgraf, R. (1993a). Simultaneous microdialysis in blood and brain: oxytocin and vasopressin release in response to central and peripheral osmotic stimulation and suckling in the rat. Neuroendocrinology 58, 637–645.
Neumann, I., Russell, J. A., and Landgraf, R. (1993b). Oxytocin and vasopressin release within the supraoptic and paraventricular nuclei of pregnant, parturient and lactating rats: a microdialysis study. Neuroscience 53, 65–75.
Neumann, I. D., Torner, L., and Wigger, A. (2000). Brain oxytocin: differential inhibition of neuroendocrine stress responses and anxiety-related behaviour in virgin, pregnant and lactating rats. Neuroscience 95, 567–575. doi: 10.1016/s0306-4522(99)00433-9
Ninomiya, I., Akiyama, T., and Nishiura, N. (1990). Mechanism of cardiac-related synchronized cardiac sympathetic nerve activity in awake cats. Am. J. Physiol. 259, R499–R506.
Nishioka, T., Anselmo-Franci, J. A., Li, P., Callahan, M. F., and Morris, M. (1998). Stress increases oxytocin release within the hypothalamic paraventricular nucleus. Brain Res. 781, 57–61. doi: 10.1016/S0006-8993(97)01159-1
Nunn, N., Womack, M., Dart, C., and Barrett-Jolley, R. (2011). Function and pharmacology of spinally-projecting sympathetic pre-autonomic neurones in the paraventricular nucleus of the hypothalamus. Curr. Neuropharmacol. 9, 262–277. doi: 10.2174/157015911795596531
Oldfield, B. J., Davern, P. J., Giles, M. E., Allen, A. M., Badoer, E., and McKinley, M. J. (2001). Efferent neural projections of angiotensin receptor (AT1) expressing neurones in the hypothalamic paraventricular nucleus of the rat. J. Neuroendocrinol. 13, 139–146. doi: 10.1046/j.1365-2826.2001.00597.x
Ostrowski, N. L., Lolait, S. J., Bradley, D. J., O’Carroll, A. M., Brownstein, M. J., and Young, W. S. (1992). Distribution of V1a and V2 vasopressin receptor messenger ribonucleic acids in rat liver, kidney, pituitary and brain. Endocrinology 131, 533–535. doi: 10.1210/endo.131.1.1535312
Ostrowski, N. L., Lolait, S. J., and Young, W. S. (1994). Cellular localization of vasopressin V1a receptor messenger ribonucleic acid in adult male rat brain, pineal, and brain vasculature. Endocrinology 135, 1511–1528. doi: 10.1210/endo.135.4.7925112
Ostrowski, N. L., Young, W. S., Knepper, M. A., and Lolait, S. J. (1993). Expression of vasopressin V1a and V2 receptor messenger ribonucleic acid in the liver and kidney of embryonic, developing, and adult rats. Endocrinology 133, 1849–1859. doi: 10.1210/endo.133.4.8404628
Ota, M., Crofton, J. T., and Share, L. (1994). Hemorrhage-induced vasopressin release in the paraventricular nucleus measured by in vivo microdialysis. Brain Res. 658, 49–54. doi: 10.1016/S0006-8993(09)90009-9
Ott, I., and Scott, J. C. (1910). The action of infundibulin upon the mammary secretion. Exp. Biol. Med. 8, 48–49. doi: 10.3181/00379727-8-27
Parati, G., Torlasco, C., Pengo, M., Bilo, G., and Ochoa, J. E. (2020). Blood pressure variability: its relevance for cardiovascular homeostasis and cardiovascular diseases. Hypertens. Res. 43, 609–620. doi: 10.1038/s41440-020-0421-5
Park, J. B., Jo, J. Y., Zheng, H., Patel, K. P., and Stern, J. E. (2009). Regulation of tonic GABA inhibitory function, presympathetic neuronal activity and sympathetic outflow from the paraventricular nucleus by astroglial GABA transporters. J. Physiol. 587, 4645–4660. doi: 10.1113/jphysiol.2009.173435
Petersson, M., Alster, P., Lundeberg, T., and Uvnäs-Moberg, K. (1996). Oxytocin causes a long-term decrease of blood pressure in female and male rats. Physiol. Behav. 60, 1311–1315. doi: 10.1016/S0031-9384(96)00261-2
Petersson, M., Lundeberg, T., and Uvnäs-Moberg, K. (1997). Oxytocin decreases blood pressure in male but not in female spontaneously hypertensive rats. J. Auton. Nerv. Syst. 66, 15–18. doi: 10.1016/s0165-1838(97)00040-4
Pittman, Q. J., Riphagen, C. L., and Lederis, K. (1984). Release of immunoassayable neurohypophyseal peptides from rat spinal cord, in vivo. Brain Res. 300, 321–326. doi: 10.1016/0006-8993(84)90842-4
Portillo, F., Carrasco, M., and Vallo, J. J. (1998). Separate populations of neurons within the paraventricular hypothalamic nucleus of the rat project to vagal and thoracic autonomic preganglionic levels and express c-Fos protein induced by lithium chloride. J. Chem. Neuroanat. 14, 95–102. doi: 10.1016/S0891-0618(97)10022-9
Pow, D. V., and Morris, J. F. (1989). Dendrites of hypothalamic magnocellular neurons release neurohypophysial peptides by exocytosis. Neuroscience 32, 435–439. doi: 10.1016/0306-4522(89)90091-2
Pyner, S. (2009). Neurochemistry of the paraventricular nucleus of the hypothalamus: implications for cardiovascular regulation. J. Chem. Neuroanat. 38, 197–208. doi: 10.1016/j.jchemneu.2009.03.005
Pyner, S., and Coote, J. H. (2000). Identification of branching paraventricular neurons of the hypothalamus that project to the rostroventrolateral medulla and spinal cord. Neuroscience 100, 549–556. doi: 10.1016/S0306-4522(00)00283-9
Rasmussen, M. S., Simonsen, J. A., Sandgaard, N. C. F., Høilund-Carlsen, P. F., and Bie, P. (2004). Effects of oxytocin in normal man during low and high sodium diets. Acta Physiol. Scand. 181, 247–257. doi: 10.1111/j.1365-201X.2004.01286.x
Ribeiro, N., Panizza, H., do, N., Santos, K. M. D., Ferreira-Neto, H. C., and Antunes, V. R. (2015). Salt-induced sympathoexcitation involves vasopressin V1a receptor activation in the paraventricular nucleus of the hypothalamus. Am. J. Phys. Regul. Integr. Comp. Phys. 309, R1369–R1379. doi: 10.1152/ajpregu.00312.2015
Riphagen, C. L., and Pittman, Q. J. (1989). Mechanisms underlying the cardiovascular responses to intrathecal vasopressin administration in rats. Can. J. Physiol. Pharmacol. 67, 269–275. doi: 10.1139/y89-044
Rivier, C., and Vale, W. (1983). Interaction of corticotropin-releasing factor and arginine vasopressin on adrenocorticotropin secretionin vivo. Endocrinology 113, 939–942. doi: 10.1210/endo-113-3-939
Roper, J. A., O’Carroll, A.-M., Young, W. S., and Lolait, S. J. (2011). The vasopressin Avpr1b receptor: Molecular and pharmacological studies. Stress 14, 98–115. doi: 10.3109/10253890.2010.512376
Rosmond, R. (2005). Role of stress in the pathogenesis of the metabolic syndrome. Psychoneuroendocrinology 30, 1–10. doi: 10.1016/j.psyneuen.2004.05.007
Russ, R. D., Resta, T. C., and Walker, B. R. (1992). Pulmonary vasodilatory response to neurohypophyseal peptides in the rat. J. Appl. Physiol. 73, 473–478. doi: 10.1152/jappl.1992.73.2.473
Russ, R. D., and Walker, B. R. (1992). Role of nitric oxide in vasopressinergic pulmonary vasodilatation. Am. J. Phys. 262, H743–H747. doi: 10.1152/ajpheart.1992.262.3.H743
Russell, J. A., and Brunton, P. J. (2017). Oxytocin: control of secretion by the brain and central roles. Ref. Mod. Neurosci. Biobehav. Psychol. doi: 10.1016/b978-0-12-809324-5.02246-x
Sampey, D. B., Burrell, L. M., and Widdop, R. E. (1999). Vasopressin V2 receptor enhances gain of baroreflex in conscious spontaneously hypertensive rats. Am. J. Phys. Regul. Integr. Comp. Phys. 276, R872–R879. doi: 10.1152/ajpregu.1999.276.3.R872
Savić, B., Martin, A., Mecawi, A. S., Bukumirić, Z., Antunes-Rodrigues, J., Murphy, D., et al. (2020). Vasopressin and v1br gene expression is increased in the hypothalamic PVN of borderline hypertensive rats. Hypertens. Res. 43, 1165–1174. doi: 10.1038/s41440-020-0469-2
Sawchenko, P. E., Brown, E. R., Chan, R. K., Ericsson, A., Li, H. Y., Roland, B. L., et al. (1996). The paraventricular nucleus of the hypothalamus and the functional neuroanatomy of visceromotor responses to stress. Prog. Brain Res. 107, 201–222.
Sawchenko, P. E., and Swanson, L. W. (1982a). Immunohistochemical identification of neurons in the paraventricular nucleus of the hypothalamus that project to the medulla or to the spinal cord in the rat. J. Comp. Neurol. 205, 260–272. doi: 10.1002/cne.902050306
Sawchenko, P. E., and Swanson, L. W. (1982b). The organization of noradrenergic pathways from the brainstem to the paraventricular and supraoptic nuclei in the rat. Brain Res. Rev. 4, 275–325. doi: 10.1016/0165-0173(82)90010-8
Scala-Guenot, D. D., Di Scala-Guenot, D., Strosser, M. T., and Richard, P. (1987). Electrical stimulations of perifused magnocellular nuclei in vitro elicit Ca2 -dependent, tetrodotoxin-insensitive release of oxytocin and vasopressin. Neurosci. Lett. 76, 209–214. doi: 10.1016/0304-3940(87)90717-8
Sermasi, E., and Coote, J. H. (1994). Oxytocin acts at V1 receptors to excite sympathetic preganglionic neurones in neonate rat spinal cord in vitro. Brain Res. 647, 323–332. doi: 10.1016/0006-8993(94)91331-5
Sermasi, E., Howl, J., Wheatley, M., and Coote, J. H. (1998). Localisation of arginine vasopressin V 1a receptors on sympatho-adrenal preganglionic neurones. Exp. Brain Res. 119, 85–91. doi: 10.1007/s002210050322
Sladek, C. D., Michelini, L. C., Stachenfeld, N. S., Stern, J. E., and Urban, J. H. (2015). Endocrine-autonomic linkages. Compr. Physiol. 5, 1281–1323. doi: 10.1002/cphy.c140028
Son, S. J., Filosa, J. A., Potapenko, E. S., Biancardi, V. C., Zheng, H., Patel, K. P., et al. (2013). Dendritic peptide release mediates interpopulation crosstalk between neurosecretory and preautonomic networks. Neuron 78, 1036–1049. doi: 10.1016/j.neuron.2013.04.025
Stauss, H. M., and Kregel, K. C. (1996). Frequency response characteristic of sympathetic-mediated vasomotor waves in conscious rats. Am. J. Phys. 271, H1416–H1422. doi: 10.1152/ajpheart.1996.271.4.H1416
Stauss, H. M., Persson, P. B., Johnson, A. K., and Kregel, K. C. (1997). Frequency-response characteristics of autonomic nervous system function in conscious rats. Am. J. Phys. 273, H786–H795.
Stern, J. E. (2001). Electrophysiological and morphological properties of pre-autonomic neurones in the rat hypothalamic paraventricular nucleus. J. Physiol. 537, 161–177. doi: 10.1111/j.1469-7793.2001.0161k.x
Stojicić, S., Milutinović, S., Sarenac, O., Zivković, S., and Japundzić-Zigon, N. (2006). Central vasopressin V1a and V1b receptors modulate the cardiovascular response to air-jet stress in conscious rats. Biomed. Tech. 51, 268–271. doi: 10.1515/BMT.2006.053
Stojicić, S., Milutinović-Smiljanić, S., Sarenac, O., Milosavljević, S., Paton, J. F. R., Murphy, D., et al. (2008). Blockade of central vasopressin receptors reduces the cardiovascular response to acute stress in freely moving rats. Neuropharmacology 54, 824–836. doi: 10.1016/j.neuropharm.2007.12.013
Stoop, R. (2014). Neuromodulation by oxytocin and vasopressin in the central nervous system as a basis for their rapid behavioral effects. Curr. Opin. Neurobiol. 29, 187–193. doi: 10.1016/j.conb.2014.09.012
Strack, A. M., Sawyer, W. B., Hughes, J. H., Platt, K. B., and Loewy, A. D. (1989a). A general pattern of CNS innervation of the sympathetic outflow demonstrated by transneuronal pseudorabies viral infections. Brain Res. 491, 156–162. doi: 10.1016/0006-8993(89)90098-x
Strack, A. M., Sawyer, W. B., Platt, K. B., and Loewy, A. D. (1989b). CNS cell groups regulating the sympathetic outflow to adrenal gland as revealed by transneuronal cell body labelling with pseudorabies virus. Brain Res. 491, 274–296. doi: 10.1016/0006-8993(89)90063-2
Swanson, L. W. (1995). Mapping the human brain: past, present, and future. Trends Neurosci. 18, 471–474. doi: 10.1016/0166-2236(95)92766-J
Swanson, L. W., and Kuypers, H. G. (1980). The paraventricular nucleus of the hypothalamus: cytoarchitectonic subdivisions and organization of projections to the pituitary, dorsal vagal complex, and spinal cord as demonstrated by retrograde fluorescence double-labeling methods. J. Comp. Neurol. 194, 555–570. doi: 10.1002/cne.901940306
Swanson, L. W., and Sawchenko, P. E. (1980). Paraventricular nucleus: a site for the integration of neuroendocrine and autonomic mechanisms. Neuroendocrinology 31, 410–417. doi: 10.1159/000123111
Swanson, L. W., and Sawchenko, P. E. (1983). Hypothalamic integration: organization of the paraventricular and supraoptic nuclei. Annu. Rev. Neurosci. 6, 269–324. doi: 10.1146/annurev.ne.06.030183.001413
Swanson, L. W., Sawchenko, P. E., Rivier, J., and Vale, W. W. (1983). Organization of ovine corticotropin-releasing factor immunoreactive cells and fibers in the rat brain: an immunohistochemical study. Neuroendocrinology 36, 165–186. doi: 10.1159/000123454
Thibonnier, M., Coles, P., Thibonnier, A., and Shoham, M. (2002). Molecular pharmacology and modeling of vasopressin receptors. Prog. Brain Res. 139, 179–196. doi: 10.1016/s0079-6123(02)39016-2
Thibonnier, M., Conarty, D. M., Preston, J. A., Plesnicher, C. L., Dweik, R. A., and Erzurum, S. C. (1999). Human vascular endothelial cells express oxytocin receptors. Endocrinology 140, 1301–1309. doi: 10.1210/endo.140.3.6546
Unger, T., Rohmeiss, P., Demmert, G., Ganten, D., Lang, R. E., and Luft, F. C. (1986). Differential modulation of the baroreceptor reflex by brain and plasma vasopressin Hypertension. 140, 1301–1309. doi: 10.1161/01.hyp.8.6_pt_2.ii157
Vågnes, O., Feng, J. J., Iversen, B. M., and Arendshorst, W. J. (2000). Upregulation of V(1) receptors in renal resistance vessels of rats developing genetic hypertension. Am. J. Physiol. Ren. Physiol. 278, F940–F948. doi: 10.1152/ajprenal.2000.278.6.F940
Valtin, H. (1982). The discovery of the Brattleboro rat, recommended nomenclature, and the question of proper controls. Ann. N. Y. Acad. Sci. 394, 1–9. doi: 10.1111/j.1749-6632.1982.tb37405.x
VanBavel, E., Giezeman, M. J., Mooij, T., and Spaan, J. A. (1991). Influence of pressure alterations on tone and vasomotion of isolated mesenteric small arteries of the rat. J. Physiol. 436, 371–383. doi: 10.1113/jphysiol.1991.sp018555
Verbalis, J. G., Mangione, M. P., and Stricker, E. M. (1991). Oxytocin produces natriuresis in rats at physiological plasma concentrations. Endocrinology 128, 1317–1322. doi: 10.1210/endo-128-3-1317
Wang, L. A., Nguyen, D. H., and Mifflin, S. W. (2019). Corticotropin-releasing hormone projections from the paraventricular nucleus of the hypothalamus to the nucleus of the solitary tract increase blood pressure. J. Neurophysiol. 121, 602–608. doi: 10.1152/jn.00623.2018
Watkins, N. D., Cork, S. C., and Pyner, S. (2009). An immunohistochemical investigation of the relationship between neuronal nitric oxide synthase, GABA and presympathetic paraventricular neurons in the hypothalamus. Neuroscience 159, 1079–1088. doi: 10.1016/j.neuroscience.2009.01.012
Whitnall, M. H., Mezey, Ė., and Gainer, H. (1985). Co-localization of corticotropin-releasing factor and vasopressin in median eminence neurosecretory vesicles. Nature 317, 248–250. doi: 10.1038/317248a0
Wilson, J. L. L., Miranda, C. A., and Knepper, M. A. (2013). Vasopressin and the regulation of aquaporin-2. Clin. Exp. Nephrol. 17, 751–764. doi: 10.1007/s10157-013-0789-5
Wotjak, C. T., Kubota, M., Liebsch, G., Montkowski, A., Holsboer, F., Neumann, I., et al. (1996). Release of vasopressin within the rat paraventricular nucleus in response to emotional stress: a novel mechanism of regulating adrenocorticotropic hormone secretion? J. Neurosci. 16, 7725–7732. doi: 10.1523/JNEUROSCI.16-23-07725.1996
Wotjak, C. T., Ludwig, M., Ebner, K., Russell, J. A., Singewald, N., Landgraf, R., et al. (2002). Vasopressin from hypothalamic magnocellular neurons has opposite actions at the adenohypophysis and in the supraoptic nucleus on ACTH secretion. Eur. J. Neurosci. 16, 477–485. doi: 10.1046/j.1460-9568.2002.02101.x
Xi, D., Kusano, K., and Gainer, H. (1999). Quantitative analysis of oxytocin and vasopressin messenger ribonucleic acids in single magnocellular neurons isolated from supraoptic nucleus of rat hypothalamus. Endocrinology 140, 4677–4682. doi: 10.1210/endo.140.10.7054
Yang, Z., and Coote, J. H. (1998). Influence of the hypothalamic paraventricular nucleus on cardiovascular neurones in the rostral ventrolateral medulla of the rat. J. Physiol. 513, 521–530.
Yang, Z., Han, D., and Coote, J. H. (2009). Cardiac sympatho-excitatory action of PVN-spinal oxytocin neurones. Auton. Neurosci. 147, 80–85. doi: 10.1016/j.autneu.2009.01.013
Yang, Z., Wheatley, M., and Coote, J. H. (2002). Neuropeptides, amines and amino acids as mediators of the sympathetic effects of paraventricular nucleus activation in the rat. Exp. Physiol. 87, 663–674. doi: 10.1113/eph8702439
Keywords: blood pressure, blood pressure variability, PVN, vasopressin, oxytocin, baroreflex
Citation: Savić B, Murphy D and Japundžić-Žigon N (2022) The Paraventricular Nucleus of the Hypothalamus in Control of Blood Pressure and Blood Pressure Variability. Front. Physiol. 13:858941. doi: 10.3389/fphys.2022.858941
Received: 20 January 2022; Accepted: 15 February 2022;
Published: 16 March 2022.
Edited by:
Geoffrey A. Head, Baker Heart and Diabetes Institute, AustraliaReviewed by:
Stephanie Tjen-A-Looi, University of California, Irvine, United StatesCopyright © 2022 Savić, Murphy and Japundžić-Žigon. This is an open-access article distributed under the terms of the Creative Commons Attribution License (CC BY). The use, distribution or reproduction in other forums is permitted, provided the original author(s) and the copyright owner(s) are credited and that the original publication in this journal is cited, in accordance with accepted academic practice. No use, distribution or reproduction is permitted which does not comply with these terms.
*Correspondence: Nina Japundžić-Žigon, bmluYS56aWdvbkBtZWQuYmcuYWMucnM=
Disclaimer: All claims expressed in this article are solely those of the authors and do not necessarily represent those of their affiliated organizations, or those of the publisher, the editors and the reviewers. Any product that may be evaluated in this article or claim that may be made by its manufacturer is not guaranteed or endorsed by the publisher.
Research integrity at Frontiers
Learn more about the work of our research integrity team to safeguard the quality of each article we publish.