- 1Provincial-Level Key Laboratory for Molecular Medicine of Major Diseases and the Prevention and Treatment with Traditional Chinese Medicine Research in Gansu Colleges and University, Gansu University of Chinese Medicine, Lanzhou, China
- 2College of Basic Medicine, Gansu University of Chinese Medicine, Lanzhou, China
- 3Key Laboratory for Transfer of Dunhuang Medicine at the Provincial and Ministerial Level, Gansu University of Chinese Medicine, Lanzhou, China
- 4College of Integrated Traditional Chinese and Western Medicine, Gansu University of Chinese Medicine, Lanzhou, China
- 5State Key Laboratory of Animal Nutrition, College of Animal Science and Technology, China Agricultural University, Beijing, China
- 6College of Public Health, Gansu University of Chinese Medicine, Lanzhou, China
Nociception refers to the process of encoding and processing noxious stimuli, which allow animals to detect and avoid potentially harmful stimuli. Several types of stimuli can trigger nociceptive sensory transduction, including thermal, noxious chemicals, and harsh mechanical stimulation that depend on the corresponding nociceptors. In view of the high evolutionary conservation of the mechanisms that govern nociception from Drosophila melanogaster to mammals, investigation in the fruit fly Drosophila help us understand how the sensory nervous system works and what happen in nociception. Here, we present an overview of currently identified conserved genetics of nociception, the nociceptive sensory neurons responsible for detecting noxious stimuli, and various assays for evaluating different nociception. Finally, we cover development of anti-pain drug using fly model. These comparisons illustrate the value of using Drosophila as model for uncovering nociception mechanisms, which are essential for identifying new treatment goals and developing novel analgesics that are applicable to human health.
Introduction
Pain is an “unpleasant sensory and emotional experience associated with actual or potential tissue damage”—as defined by the International Association for the Study of Pain1, it is an indispensable and rich sensory experience which can help people promote the healing and improvement of the injured or diseased parts of the body (Young et al., 2012). Pain can be classified into nociception, inflammatory and pathological pain according to the pathological mechanism (Costigan et al., 2009; Woolf, 2010; Haanpaa et al., 2011), and acute, chronic, and occasional pain according to the duration (Turk, 2001; Glare et al., 2019). Pain is a subjective experience, and nociception is an objective neural process that encodes and processes harmful stimuli. It is an evolutionary conservative mechanism that reminds organisms of potential tissue damage and is vital to survival (Neely et al., 2010; Khuong and Neely, 2013). For living organisms, the rapid response to harmful stimuli and the ability to react and avoid them (pain/nociception) is crucial (Milinkeviciute et al., 2012).
Most of the research objects on nociception are model animals, such as monkey (Lee et al., 2007), mice (Luo et al., 2019), zebrafish (Malafoglia et al., 2013), Drosophila melanogaster (Leung et al., 2013), C. elegans (Nkambeu et al., 2020) and so on. As a bridge between disease research and human beings, model animals play an important role in modern medical research. Extensive modeling has been performed in mammals, however, these models are expensive, and have ethical implications. In contrast, Drosophila has its unique advantages as a model animal for pain research, small size and relatively short lifecycle allows it could be produced in large numbers and easy to work with. Most importantly, fruit flies show a high degree of homology with humans at the organ and gene level, with flies sharing functional counterparts for most organ systems (Fortini et al., 2000; Chien et al., 2002). It has been estimated that 75% of human disease genes have conserved homologs in Drosophila, making this fly a model organism of great potential (Bier, 2005). Using fruit flies as human disease model would avoid the ethical controversy. At present flies has been used extensively as a model for human disease already, for example, to study cancer, Alzheimer’s disease, nociception, obesity and diabetes and so on (Milinkeviciute et al., 2012; Enomoto et al., 2018; Tsuda and Lim, 2018; Warr et al., 2018).
As previously mentioned, there are conserved physiological mechanisms underlying the nociceptive system between human and flies (Sneddon, 2018). The nociceptors in the primary afferent nerve fibers are stimulated by thermal, mechanical and chemical stimulation, converted into electrical signals, and then transmitted to the central nervous system such as the spinal cord, and finally felt the pain (Julius, 2013; Bourne et al., 2014; Dai, 2016; Sneddon, 2018; St, 2018). These nerve fibers quickly transmit the perceived harmful information to the central nervous system through action potentials. In this process, ion channels play a vital role. These ion channels are specifically expressed in the above-mentioned nerve fibers (Julius, 2013; Dai, 2016; Yam et al., 2018). TRP, Piezo and other ion channels have been identified as key pain receptors (Hwang and Oh, 2007; Volkers et al., 2015). Among these channels, TRPV1, TRPA1, Piezo1 and Piezo2 are expressed in nociceptors (Liedtke, 2007; Flood et al., 2013; Volkers et al., 2015; Himmel and Cox, 2017; Boonen et al., 2021). They serve as detectors and sensors for cold, heat, chemical and mechanical stimuli in nociceptors. These conserved genes in Drosophila well prove the potential of flies as a nociceptive model animal. The purpose of this review is to present the aggregate findings of the pain-related genes in order to discuss the possibilities for Drosophila as model animal in nociception research, and provide a comprehensive evaluation for future human nociception studies.
The Role of Pain-Related Genes in Regulating Nociception/Pain
Transient Receptor Potential Channels
Transient receptor potential (TRP) channels are a large family of ion channels, and most of them are conserved from Drosophila to humans. It has more than 50 subtypes, divided into 7 subfamilies according to their amino acid sequence homology, which includes vanilloid (TRPV1-6), canonical or classic (TRPC1-7), melastatin (TRPM1-8), non-mechanoreceptor potential C (NOMP-like, TRPN1), long TRP ankyrin (TRPA1), polycystins (TRPP1-5) and mucolipins (TRPML1-3) (Clapham et al., 2001; Nilius et al., 2005, 2012; Wu et al., 2010; Li, 2017; Bamps et al., 2021). TRP channels allow an inward cation current to regulate cell function, and have a variety of activation modes by mechanical, thermal and chemical stimuli (Nilius et al., 2007; Nilius and Owsianik, 2011). Therefore, the TRP family has a variety of physiological functions, including vision, hearing, taste, thermosensation and response to different environmental stimuli (Gees et al., 2012). The TRP channels are probably best known for its role in nociceptive perception, it is the largest group of noxious ion channels involved in pain. Among the sub-families, TRPA1, TRPV1, and TRPM2 have been shown to be related to nociception (Table 1; Flood et al., 2013; Himmel and Cox, 2017; Maiese, 2017; Logashina et al., 2019).
TRPA1 is a channel with non-selective permeability to calcium, sodium and potassium, while its permeability to calcium is higher than that of other TRPs (Kwan and Corey, 2009). It acts as a sensor for cell damage signals and is involved in inflammation and immune response (Bandell et al., 2004; Kwan et al., 2006; Kwan and Corey, 2009; Neely et al., 2011; Zygmunt and Hogestatt, 2014; Laursen et al., 2015; Viana, 2016). Mostly, TRPA1 is crucial in mediating long-term hypersensitivity to thermal, cold, chemical, and mechanical stimuli detected in nociceptive, inflammatory, and neuropathic pain models (Story et al., 2003; Corey et al., 2004; Kwan et al., 2006; Karashima et al., 2009; Del et al., 2010). TRPA1 has been proposed to function as a temperature-insensitive detrimental heat sensor and a detrimental cold sensor (Laursen et al., 2015). TRPA1 promotes excitatory effects of bradykinin through the PLC/calcium signaling pathway (Bandell et al., 2004), so it is an important downstream target for inducing pain receptor hypersensitivity (Bautista et al., 2006). In inflammatory pain, the role of TRPA1 channels is two sides. On the one hand, pro-inflammatory factors activate nociceptors through TRPA1. On the other hand, TRPA1 stimulation is usually related to the release of pro-inflammatory neuropeptides (Nassini et al., 2014).
TRPV1 is a cation permeable channel and shows important influence in feeling nociceptive stimuli and producing pain in primary afferent nociceptors (Immke and Gavva, 2006). As an ion channel, it can be activated by specific activators, such as vanillin, capsaicin, sorbamide, etc., (Gees et al., 2012; Julius, 2013; Dai, 2016; Li, 2017; Hung and Tan, 2018). TRPV1 is now considered to be a molecular integration factor of pain stimuli, and drug target. In animals, the sensitization and activation of peripheral nociceptors can cause TRPV1 to transmit nociceptive signals to the central nervous system, thereby producing unpleasant and painful sensations, warning the body of potential harmful threats (Hung and Tan, 2018). TRPV1 not only plays a vital role in nociception, but also leads to the generation of action potentials during inflammation, which in turn leads to the generation of pathological pain, such as thermal hyperalgesia, spontaneous pain and mechanical hypersensitivity (Caterina et al., 2000; Ma and Quirion, 2007). TRPV1 knockout mice have a significant reduction in thermal hypersensitivity after tissue injury, which clearly proves that TRPV1 is involved in the development of inflammatory pain (Caterina et al., 2000; Davis et al., 2000). TRPM2 as a calcium ion-permeable non-selective cation channel is expressed in the peripheral nervous system and immune system, which is activated by oxidative stress, moderate temperature and intracellular adenosine diphosphate ribose (ADPR) in various types of cells (Kolisek et al., 2005; Togashi et al., 2006). TRPM2 is of great importance in the pathogenesis of inflammation and neuropathic pain (Eisfeld and Luckhoff, 2007; Faouzi and Penner, 2014). A study showed that in carrageenan-induced inflammatory pain and sciatic nerve injury-induced neuropathic pain models, TRPM2 knockout mice have alleviated mechanical hyperalgesia and thermal hyperalgesia (Di Meglio et al., 2004).
The first evidence for the existence of TRP channels were found in Drosophila flies. Cosens and Manning used electroretinogram (ERG) measurements to analyze a spontaneous mutant in Drosophila that exhibited a temporary rather than a continuous response under long-term bright light (Cosens and Manning, 1969). It is firstly named a “type A” mutation. Later, it was found that this mutant had defects in light transmission, and had a representative name: “transient receptor potential” or Trp (Minke et al., 1975). TRP channels are diverse in structure and can modulate transduction of thermal, mechanical, and chemical stimuli and also can regulate cell growth, cell differentiation, and vascular physiology in flies (Pazienza et al., 2014; Maiese, 2017). The Drosophila genome contains genes encoding 13 TRP channels, and encodes four TRPA homologs: dTRPA1, painless, pyrexia, and water witch (Fowler and Montell, 2013). TRPA channels have been the most widely studied for their roles in temperature-sensing behavior in flies (Goodman, 2003; Xu et al., 2006; Neely et al., 2011; Bellemer, 2015) and also play important roles in chemical and mechanical sensing (Mandel et al., 2018; Boonen et al., 2021). TRPA1 has been implicated as a mammal noxious cold receptor, which is activated by extremely cold temperatures (below < 15°C) (Kwan and Corey, 2009). However, the TRPA1 homologs dTRPA1, painless, pyrexia in flies have no function in regulation of cold avoidance. The temperature-sensitive diversity of TRPA1 channels in flies and mammals makes researchers more cautious when dissecting the role of TRPA1 in thermal stimulation and screening anti-pain drug using D. melanogaster.
dTRPA1 was first identified as a heat-activated channel in flies (Rosenzweig et al., 2005; Kwon et al., 2008). It is 32% identical and 54% similar to its mammalian orthology by amino acid identity, and is activated in response to high temperature, reactive chemicals and downstream of intracellular signaling pathways (Neely et al., 2011; Bellemer, 2015; Boonen et al., 2021). The dTRPA1 channel is expressed in the multiple groups of central neurons and several classes of peripheral sensory neurons (Hamada et al., 2008; Kang et al., 2010; Kim et al., 2010). dTRPA1 participates not only in the thermal pain of adults, but also in the thermal pain of larvae (Neely et al., 2011; Luo et al., 2017). Control adults flies respond very quickly to harmful heat at 46°C, but dTRPA1 mutants respond slowly to harmful heat, and their thermal pain ability was significantly reduced (Neely et al., 2011). Fly larvae trigger noxious rolling behavior when the temperature is below 40°C, and the frequency of this behavior increases rapidly as the temperature rises until 33°C. The above-mentioned nociceptive behaviors all depend on the dTRPA1 channel, the activity of which responds to the rate of temperature change (Luo et al., 2017). In addition to participating in the response to harmful temperature stimuli, dTRPA1 has also been detected in chemical stimuli (Tracey et al., 2003; Im and Galko, 2012). Boonen et al. (2021) found that wild-type flies avoid citronellal and menthol in olfactory tests, while dTRPA1 mutant flies have reduced this behavior. dTRPA1 channel mediates chemical avoidance in gustatory receptor neurons (GRNs), in which know down of dTRPA1 in GRNs significantly reduced the aversive response to aristolochic acid (Kim et al., 2010).
Painless as a member of the TRPA family channel was discovered and identified as an important gene for thermal and mechanical nociception in Drosophila flies (Tracey et al., 2003; Xu et al., 2006). It is expressed in the larval peripheral nervous system (Tracey et al., 2003), and various regions of the adult brain, such as mushroom body, a region important for learning and memory (Busto et al., 2010) elliposoid body of the central complex (Sakai et al., 2012, 2014); olfactory projection neurons in antennal lobes (Wang et al., 2011); and the pars intercerebralis including insulin-producing cells (Sakai et al., 2012, 2014). Painless is a molecular sensor for noxious thermal stimuli in larvae and adult flies (Goodman, 2003). Studies have confirmed that wild-type larvae exhibit typical “rolling behavior” within 1 s of being contacted by the heated probe above 40°C, and this activity is absent in the painless mutant (Goodman, 2003). When the heating temperature exceeds 38°C, the firing of the multidendritic sensory neurons increases, but this increase is not seen in the painless mutant (Xu et al., 2006; Sokabe and Tominaga, 2009). In the hot plate assay, painless mutant adults exhibited a behavioral defect and could not jump quickly to escape from a hot plate, which can be rescued by a transformed painless gene, indicating that painless is required for thermal nociception in adult flies (Xu et al., 2006). Painless requires Ca2+ as a co-agonist for heat-evoked activation. Painless failed to respond to heat in the absence of intracellular and extracellular Ca2+ (Liu et al., 2003). Painless is also required for chemical and mechanical nociception (Tracey et al., 2003; Al-Anzi et al., 2006; Mandel et al., 2018). Mandel et al. (2018) found that allyl isothiocyanate (AITC) remarkably reduce the proboscis extension reflex frequencies in wild-type genotypes but did not in painless mutant, and AITC evoked calcium changes in painless expressing neurons. Expression of painless was also detected in mechanically-sensitive Johnston’s organ, while painless could be activated by mechanical stimuli (Tracey et al., 2003). Additionally, painless is involved in a variety of neural processes in flies including negative geotaxis (Sun et al., 2009), larval social behavior (Xu et al., 2008) and sexual receptivity of virgin females (Sakai et al., 2014).
Pyrexia (pyx) gene is a heat-sensitive TRPA channel and protects flies from high temperature stress (Lee et al., 2005; Xu et al., 2006; Hamada et al., 2008). It is ubiquitously expressed along the dendrites of a subset of peripheral nervous system neurons and is more permeable to K+ than to Na+ (Lee et al., 2005). 60% of pyx null flies were paralyzed within 3 min after exposure to 40°C, while applying the same stimulation to wild-type fruit flies, the number of paralysis was only 9% (Lee et al., 2005). pyx is also responsible for the response of temperature-sensitive anterior cell (AC) brain neurons, which regulate the temperature preference behavior of adult flies (Hamada et al., 2008; Tang et al., 2013). pyx is involved in temperature synchronization of circadian clocks, in which pyx mutants fail to synchronize their behavior to temperature cycles between “night” and “day” (Wolfgang et al., 2013).
Piezo Channel
Mechanical transduction is the process of converting mechanical force into biological signals, which plays a key role in various physiological processes of animals (Volkers et al., 2015). It is through the mechanically sensitive cation channel converts the mechanical stimulus received by the animal body into various activities, and plays an important role in the regulation of touch, hearing and blood pressure (Bagriantsev et al., 2014). Piezo channel is a type of mechanical-sensitive ion channel, which is necessary for cells to respond to mechanical stimuli (Coste et al., 2010). In vertebrates, Piezo channel proteins mainly include Piezo1 and Piezo2 proteins, which are encoded by the genes Piezo1/FAM38A and Piezo2/FAM38B, respectively (Coste et al., 2010, 2012; Bagriantsev et al., 2014). Piezo1 channels are characterized by slower kinetics, and can react to more persistent activation (Lewis et al., 2017). After silencing Piezo1 expression in chondrocytes, the number of chondrocytes responding to mechanical stimulation decreased, while activating Piezo1 significantly promotes mechanical response in chondrocytes (Servin-Vences et al., 2017). Piezo2 as a faster kinetics are more specified for detection of transient mechanical forces (Ranade et al., 2014; Woo et al., 2015; Szczot et al., 2018). Recent studies have shown that Piezo2 is essential for mediating abnormal tactile pain in mice (Murthy et al., 2018; Szczot et al., 2018), and it has been confirmed that Piezo2 is also necessary for humans to feel gentle touch, proprioception, and abnormal tactile pain (Szczot et al., 2018).
Only one single Piezo protein was found in lower organisms, such as nematodes and fruit flies (Hamada et al., 2008). In the Drosophila melanogaster, there is only one copy of the force-gated ion channel, DmPiezo, a Ca2+ permeable non-selective cation channel, similar to its mammalian homolog (Coste et al., 2012). DmPiezo is 24% identical to mammalian piezos, with sequence conservation throughout the length of the proteins (Coste et al., 2012). Studies have shown that the expression of DmPiezo is detected in all types of sensory neurons and some non-neural tissues of flies, including multimodal nociceptors of larvae. Among these neurons, Dmpiezo has a special contribution to mechanical pain (Kim et al., 2012). The researchers found that Dmpiezo expression in human cells induces mechanically activated currents, similar to its mammalian counterpart (Coste et al., 2012). In Dmpiezo knockout larvae, the behavioral response to harmful mechanical stimuli is severely reduced, while the response to another harmful stimulus or touch is not affected. Knockdown of Dmpiezo in sensory neurons that mediate nociception is sufficient to weaken the response to harmful mechanical stimuli. Dmpiezo and Pickpocket (ppk) are involved in the parallel pathways of ppk-positive cells, while their absence results in mechanical nociception elimination (Kim et al., 2012). Loss of DmPiezo renders class IV sensory neurons unresponsive to harsh touch (Kim et al., 2012) and makes mechanosensitive visceral neurons, which sit in the fly’s brain and innervate the gut, mechanoinsensitive (Wang et al., 2020). DmPiezo also regulate axon regeneration in flies (Song et al., 2019), in which DmPiezo activation during axon regeneration induces local Ca2+ transients at the growth cone, leading to activation of nitric oxide synthase and the downstream cGMP kinase foraging or PKG to restrict axon regrowth, while loss of DmPiezo increases axon regeneration of sensory neurons. A second Drosophila piezo family member, piezo-like (pzl; CG45783) shares similarity with that of Dmpiezo and its mammalian homologs Piezo1 and Piezo2 (Hu et al., 2019). Pzl gene expressed in larval chordotonal neurons is required for locomotion of Drosophila larvae. The pzl mutant showed severe defects in crawling pattern and body gesture control, which could be rescued by expressing human or mouse Piezo1, suggesting a conserved role the Piezo-family proteins in locomotion (Hu et al., 2019).
DEG/ENaC Family Channels
Acid-sensitive ion channels (ASICs) are a group of proton-gated ion channels that belong to the degenerin/epithelial sodium channel (DED/ENaC) family. The channel can be activated when the extracellular pH drops below 7.0, or with aprotic ligands at physiological pH levels. The activation of ASICs mainly triggers Na+ influx (Waldmann et al., 1997; Yu et al., 2010). ASIC was found to be a major player in human pain caused by acid (Luo et al., 2017). Increasing evidence further indicates that ASIC3 is a molecular determinant of pain-related tissue acidosis in rodent models. Members of the DEG/ENaC family also play a role in nociception, and have been shown to be essential mechanical transduction molecules in Drosophila flies (Luo et al., 2019). Pickpocket1 (Ppk1) encodes an ion channel subunit of the DEG/ENaC family and is responsible for mechanical nociception responses in flies (Adams et al., 1998; Zhong et al., 2010). It is widely expressed in nociceptive and class IV multidendritic neurons (Zhong et al., 2010). Another ion channel subunits balboa (also known as ppk26) is highly enriched in nociceptive neurons and could bind to PPK to regulate mechanical nociception behaviors in Drosophila larvae (Guo et al., 2014; Mauthner et al., 2014). Ppk26 mutant showed severe behavioral defects in a mechanical nociception behavioral test but responded to noxious heat stimuli compared to wild-type larvae (Guo et al., 2014). Ppk1 and ppk26 have the same signaling pathway to regulate mechanical nociception, and they do not have functional response to in thermal stimulus (Gorczyca et al., 2014). Ppk30 as a member of the Drosophila Ppk family is detected by class IV multidendritic neurons, and has a role in mechanosensation, but not in thermosensation (Jang et al., 2019).
Drosophila Models of Nociception/Pain
Drosophila fly has high homology with human disease genes (75%), reproduces rapidly on its own, and the cost of establishing and maintaining a sufficient number of drosophila is much lower than that of the same number of vertebral model animals. These advantages make fly become a tool for studying the conservative genetics of pain (Tracey et al., 2003; Bier, 2005). The fly nociceptors are similar to vertebrates in morphology and function, and they have unique naked nerve endings. The end of the nerve dendrites of Drosophila cover the entire epidermis without overlapping, allowing them to quickly perceive tissue damage. This characteristic proves the potential of Drosophila as a model animal for noxious research (Grueber et al., 2003, 2007; Hwang and Oh, 2007).
Nociceptive Sensory Neurons in Flies
Nociception refers to the sensation of harmful stimuli that can cause tissue damage. Nociceptive sensory neurons in Drosophila have one axon and one or several dendrites each (Hehlert et al., 2021). Fly larvae have two main peripheral sensory neurons located below the barrier epidermis: type I and type II, according to dendrite number and anatomy (Kernan, 2007). The type I neurons are related to bristle type and chordotonal sensory organs and have a single ciliated dendrites (Hwang et al., 2007). The type I neurons are more involved in mechanical sensory functions, such as light touch (Kernan et al., 1994). The type II neurons have many dendritic extensions that project to nearly every epidermal cell of the larval barrier epidermis, thus the type II neurons are also called multidendritic (md) sensory neurons or dendritic arborization (DA) (Grueber et al., 2002). They are structurally similar to mammalian nociceptors (Gao et al., 1999; Grueber et al., 2002). Larvae with gene-silenced md neurons are completely insensitive to harmful stimuli and cannot produce noxious responses. This underlying evidence suggests that md sensory neurons function as nociceptors (Williams and Truman, 2005; Grueber et al., 2007). The TRP channel mentioned above is necessary for nociception, and it has been confirmed that it is expressed in md neurons, which further shows that the status of md neurons in nociception is crucial (Tracey et al., 2003; Rosenzweig et al., 2005). Morphological studies on type II neurons show that these neurons are not a unified cell population. On the contrary, at least four subtypes have been identified (Grueber et al., 2002).
According to the complexity of dendrites and other morphological characteristics, these neurons are named class I-IV neurons that tile the larval body wall (Merritt and Whitington, 1995; Schrader and Merritt, 2000; Grueber et al., 2007). The dendrites of class I neurons are the simplest, while class IV neurons are the most complex (Grueber et al., 2002). Class I and Class II dendritic domains are relatively sparse and compact, while Class III and Class IV neurons have more complex branching patterns, wider coverage, and no branch overlap (Grueber et al., 2002, 2003). Class I neurons project to the motor nerve stacks of the ventral dorsal ganglia and are thought to provide feedback to the motor neurons. However, class II, class III, and class IV neurons all project to the ventral nerve pile, and by analogy with other insects, they are predicted to have somatosensory functions (Hwang et al., 2007; Yoshino et al., 2017; Burgos et al., 2018). Class I neurons are important for coordinating the appropriate timing of peristaltic locomotion (Cheng et al., 2010). Class II and Class III are both related to light contact reactions, of which type III takes the leading role (Tsubouchi et al., 2012; Yan et al., 2013). Class III neurons also mediate the mechanical nociception and cold nociception (Tsubouchi et al., 2012; Yan et al., 2013; Turner et al., 2016). Class IV neurons appear like mammalian nociceptors morphologically (Tracey et al., 2003) and have polymodal sensitivity to a variety of sensory stimuli (Ohyama et al., 2013). Ablation or silencing class IV neurons significantly eliminates larval responses to noxious stimuli, while activation of class IV neurons is sufficient to stimulate corkscrew-like rolling behavior that is similar as larvae receive noxious stimuli (Tracey et al., 2003; Hwang et al., 2012; Ohyama et al., 2013). These neurons in the peripheral nervous system are responsible for perception of multiple nociceptive modalities, including mechanical force, harmful heat, low-wavelength light, and chemical stimuli, through distinct receptors (Tracey et al., 2003; Kang et al., 2010; Xiang et al., 2010; Hwang et al., 2012; Ohyama et al., 2013). Diverse ion channel are expressed in class IV neurons to evoke depolarization in response to corresponding noxious stimuli (Tracey et al., 2003; Lee et al., 2007; Zhong et al., 2010; Hwang et al., 2012; Kim et al., 2012).
Much of the current research on pain in Drosophila flies has focused on nociception, which is similar to acute pain in mammals. When flies suffer from noxious stimuli, multiple pathways are activated in md neurons. This includes the dTRPA1 (Mandel et al., 2018), painless (Tracey et al., 2003) and Pyrexia (Lee et al., 2005) that sense thermal pain; the DmPiezo (Song et al., 2019), painless (Tracey et al., 2003) and Pickpocket families (Zhong et al., 2010) that sense mechanical pain. After acute pain perception occurs, it is often accompanied by prolonged allodynia and hyperalgesia. Multiple pathways related to allodynia and hyperalgesia are also found in md neurons. Hedgehog (Hh) signaling is involved in allodynia and hyperalgesia when Drosophila larvae are exposed to UV light (Babcock et al., 2011). Meanwhile, Hh signaling acts in parallel with tumor necrosis factor (TNF) signaling to mediate allodynia (Babcock et al., 2009), and several TRP channels described above mediate allodynia and hyperalgesia downstream of these pathways. Painless is required for the development of Hh- or TNF-induced thermal hyperalgesia, whereas dTRPA1 is required for Hh-induced thermal hyperalgesia (Babcock et al., 2011). The BMP pathway is also expressed in md neurons during allodynia and hyperalgesia, and it is located downstream of the Hh signaling pathway (Honjo and Tracey, 2018). Decapentaplegic (Dpp, mammalian bone morphogenetic protein 2/4 ortholog) and its downstream signaling pathways in Drosophila md neurons have also been shown to be required to induce allodynia (Gjelsvik et al., 2018). The above studies show that when pain occurs, related pain signaling pathways in Drosophila md neurons are co-expressed to participate in acute nociception and subsequent chronic pain (allodynia and hyperalgesia).
Different Stimulation of Acute Nociception
Currently, the method of nociceptive research using Drosophila fly as a model animal focuses on thermal, cold, chemical and mechanical stimulation of acute nociception.
Regarding the experimental example of thermal nociception, fly larvae and adults have different methods. One of the most classic experimental examples is to collect fly larvae and place them in a petri dish, touch it with a soldering iron heated to 46°C, then the wild-type larvae would make a rolling response in a very short time (Tracey et al., 2003; Petersen et al., 2018; Figure 1A). TRPA1 mutants or painless mutants will exhibit a markedly slow response to temperature. The above experimental model has opened the door to the study of nociception or pain in Drosophila (Tracey et al., 2003; Manev and Dimitrijevic, 2004; Babcock et al., 2009; Aldrich et al., 2010; Coste et al., 2010). Another way to study the heat damage of larvae is to pour water on a petri dish filled with agar to form a water film so that the larvae can roll freely, put the larvae in the petri dish, and then put the petri dish on the heating plate. Record the length of time for the rolling response of larvae at different temperatures (Oswald et al., 2011; Figure 1B). Flies cannot be exposed to low temperatures for a long time, and their behavior prefers warmer temperatures, but the mechanism by which they perceive and avoid cold stimuli has not been studied until recently. The method of measuring the cold nociception of Drosophila larvae is to place the cold probe at a 45° angle to the back of the larva, and apply enough pressure downward to make the surface of the larva slightly concave while allowing it to move forward or backward (Turner et al., 2017). Keep the cold probe still for 10 s until the cold-induced response of the Drosophila larvae is observed, which is the response latency period (Figure 1C). The response latency period is simply the reaction time to cold stimuli. The shorter the latency period, the more sensitive the flies to cold stimuli. The response latency period of wild-type flies is shorter than that of mutant flies’ in the revised manuscript.
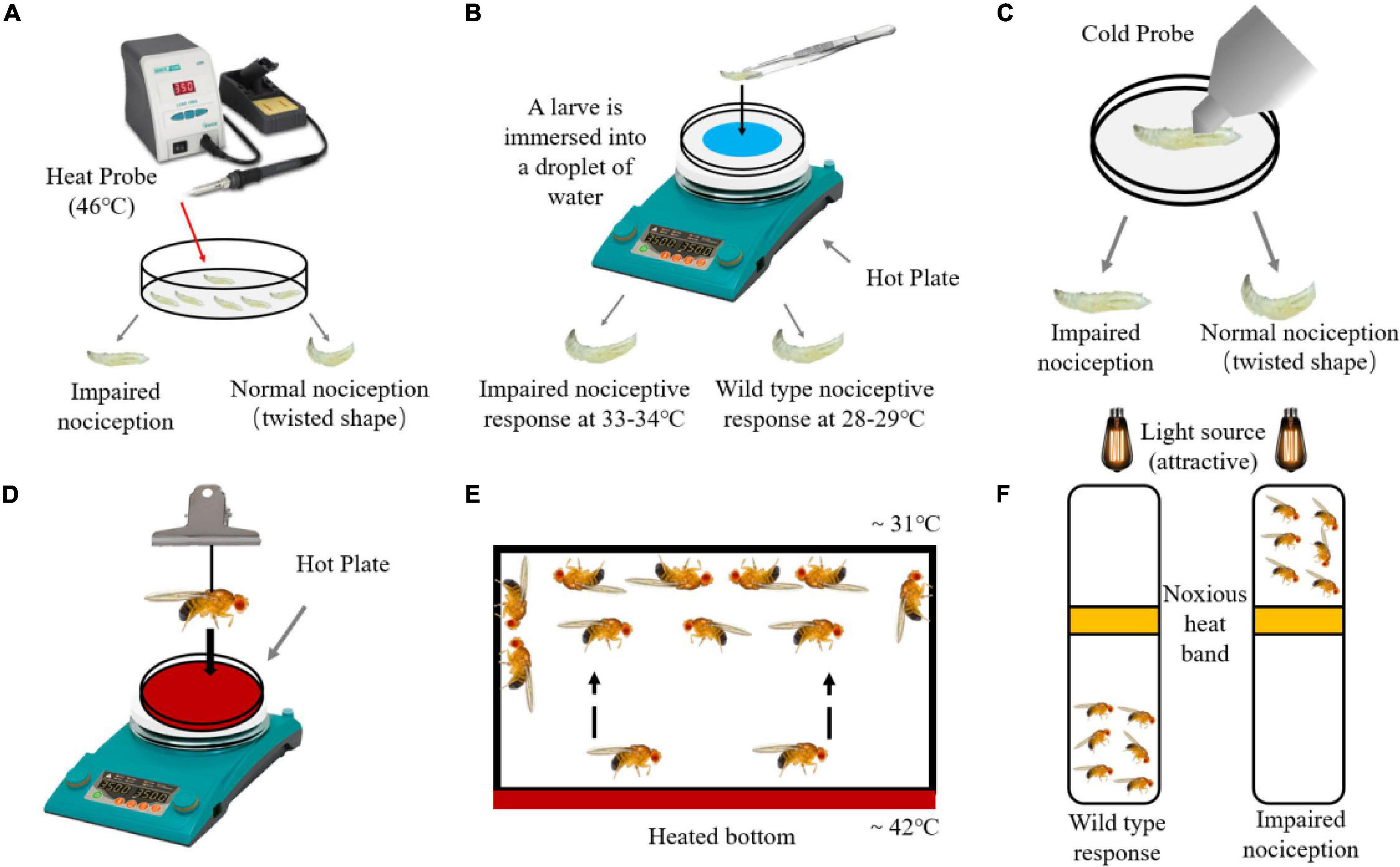
Figure 1. Detection method of harmful temperature nociception in Drosophila. (A) When using a 46°C heat probe to contact Drosophila larvae, the rolling reaction time of wild-type larvae earlier than the larvae with impaired nociception. (B) Drosophila larvae with impaired nociception and wild-type larvae can cause rolling reaction when placed in water at 33–34 and 28–29°C respectively. (C) When using a cold probe to contact larvae, the rolling reaction time of wild-type larvae earlier than the larvae with impaired nociception. (D) After keeping the adult flies on a hot plate at 47°C, record the incubation period of the Drosophila to produce a jumping response. (E) Place the adult flies in a heating device, and wild-type flies will concentrate on the upper part of the device with a suitable temperature. (F) The optical drive heat avoidance test uses a heated aluminum ring as a harmful barrier between the adult Drosophila and the light source (attractive).
The detection of typhoid fever on adult Drosophila is time-consuming and laborious. Firstly, researchers developed a method to model the “jumping” reflections that flies exhibit when exposed to noxious heat (Figure 1D), in which flies is suspended on an electric heating plate (47°C) using a nylon rope, then is dropped to get in touch with heating plate, and waiting time for flies to jump is recorded (Xu et al., 2006). Secondly, the adult flies are placed in an incubator with a heating function at the bottom, and the bottom of the box is heated to 46°C (Figure 1E). Wild-type flies will avoid that surface and rest at the upper part of 31°C (Neely et al., 2010). The group should be used as the nociception detection unit, and it is required to be simple and effective. Researchers can use this device to identify genes related to thermal nociception. The third method is to combine the light preference response of adult flies with harmful heat avoidance (Figure 1F). Flies are placed in a vertical transparent device, and a heating aluminum ring and a lamp are placed on the middle and top of the device. Wild-type flies with normal receptors are not attracted by light, while flies with knockdown of painless are attracted by light and pass through the heated aluminum ring (Benzer, 1967; Aldrich et al., 2010).
The way to study the chemical stimulation of fruit flies is to add nociceptor activators, such as capsaicin, menthol, allicin, isothiocyanate, etc., to food, which cause flies to resist food (Al-Anzi et al., 2006; Kim et al., 2010; Li et al., 2020; Figure 2A). Briefly, third-instar larvae are placed in a petri dish, use a pipette to add the above-mentioned chemical stimulus solution under and around the flies, and record the incubation period of the fruit flies (the time between the addition and the tumbling behavior) (Lopez-Bellido et al., 2019). As the concentration of the solution increases, the incubation period will become shorter and shorter. Another way to determine chemical stimulation is to test food choice, flies can make choice between control food and food with chemical irritants at the same time, and the chemical irritants can be increased in dose (Figure 2B). The control food is marked with red dye, and the food with chemical stimulus is marked with blue dye. The abdomen of wild-type flies will show a single red color, while the abdomen of mutant flies will show three colors, red, blue, and purple (two groups of food eat at the same time) (Al-Anzi et al., 2006). A method similar to the above method is to use the Drosophila’s proboscis extension response (PER) as an indicator of whether flies eat (Figure 2C). PER is judged based on the reaction of the nose of hungry flies when they eat normal food. Adding chemical stimulants to food will reduce the PER score of wild-type flies (Al-Anzi et al., 2006; Kang et al., 2010).
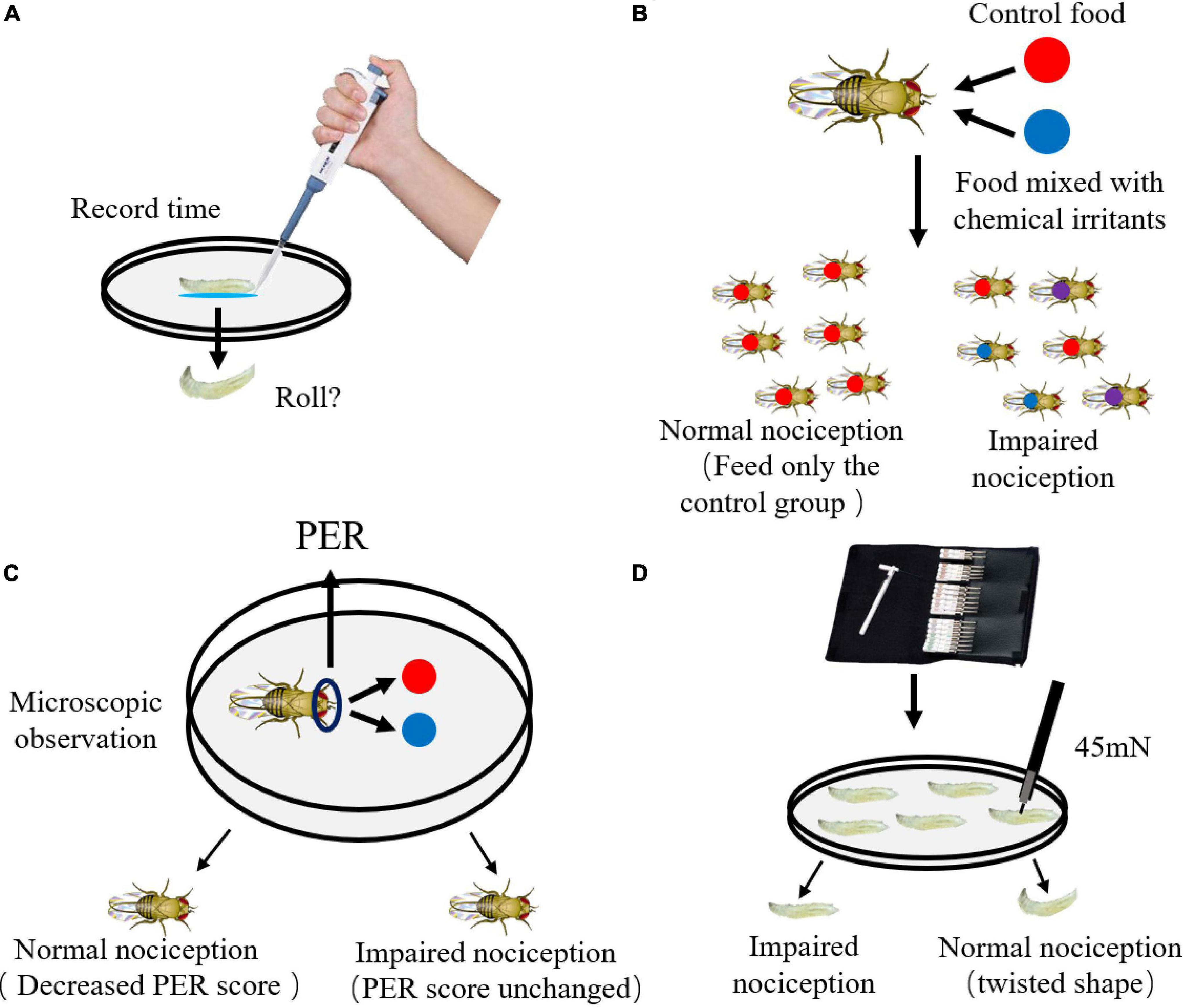
Figure 2. Detection method of chemical and mechanical nociception in Drosophila. (A) Use a pipette to place the chemical stimulus around the body of the larvae and record the incubation period. (B) Adult flies with impaired nociception eats indiscriminately, while wild-type flies eat only control food. (C) Provide two kinds of food and record the PER score of adult flies. (D) The von Frey fiber was calibrated and used for the determination of mechanical nociception: wild-type adult flies will have a rolling response when the force exceeds 45 mN, and the adult flies with impaired nociception have a greater rolling force.
The noxious rolling response of fruit flies to harmful mechanical damage is produced by stimulating von Frey fibers in a petri dish (Figure 2D; Tracey et al., 2003). The mechanical stimulation is provided by the calibrated von Frey fiber, the larvae are less active, and the noxious response is easy to evaluate, so this method is not easy to be applied to the adult mechanical damage study. First, pour clean water into a petri dish with agar so that the animals can crawl and perform rolling behaviors freely. The larvae will pause their normal feeding behavior when touched. Normal larvae elicit a rigid rolling response when subjected to a force of 45 mN von Frey fibers (Tracey et al., 2003; Hoyer et al., 2018), and painless mutant larvae appeared only spiral coiling until the stimulation increased to 100 mN (Tracey et al., 2003). This method has been improved recently. The von Frey fiber is replaced with a custom-made metal Nitinol (Nitinol) wire probe that detects mechanical damage (Hoyer et al., 2018).
Optogenetics is a powerful tool that enables spatiotemporal control of neuronal activity and circuits in behaving animals. Optogenetic nociception assay is widely used in Drosophila fly larvae (Hwang et al., 2007; Honjo et al., 2012; Dannhauser et al., 2020). The optogenetic technique with ChR2::YFP is developed and used to demonstrate the md neurons are nociceptive sensory neurons whose activation is sufficient to trigger larval nocifensive escape locomotion (Hwang et al., 2007; Honjo et al., 2012). Briefly, virgin female flies of the GAL4 driver strain that target md neurons are crossed to male flies of the UAS-ChR2::YFP strain. The larval progeny are allowed to develop and feed on the yeast paste (either atr+ or atr-) for 4 days. For behavioral analysis, the larvae are transferred to plastic Petri dishes and then stimulated with blue light (460–500 nm). Blue light pulses are manually controlled and lasted for several seconds. Nocifensive roll and nocifensive escape locomotion are videotaped and analyzed. This model can be used to dissect the molecular mechanisms that sensitize responses of nociceptors and nociception behaviors (Honjo and Tracey, 2018).
Chronic Pain Perception in Flies
The above mentioned methods are mainly used to study acute nociception. Acute nociception is often caused by noxious stimuli, which usually protect the animal body from possible harm (Bell, 2018). Chronic pain results from maladaptive changes to this nociceptive system and persists even after the healing process is complete (Voscopoulos and Lema, 2010). Much of what is currently involved in the study of chronic pain in Drosophila flies is caused by nerve damage and inflammation following noxious stimuli, which can lead to hyperalgesia (increased sensitivity to noxious stimuli) and allodynia (perceives innocuous stimuli as noxious) (Hamoudi et al., 2018; Khuong et al., 2019).
The chronic pain perception has been explored in larvae for several years. The researchers used ultraviolet (UV) light to induce tissue damage in fruit fly larvae, and then used thermal probes to demonstrate that the tissue-damaged fruit fly larvae developed allodynia and hyperalgesia (Figure 3A; Babcock et al., 2009). Briefly, the 3rd instar larvae are anesthetized with diethyl ether. Anesthetized larvae are then placed dorsal side up on a microscope slide using two-sided tape and subjected to (mJ/cm2) of UV irradiation. After UV exposure, larvae are gently rinsed and placed in a vial containing fly food for 24 h at 25°C. Then larvae are stimulated using a thermal probe. The temperature of thermal probe is set to 41°C to measure for allodynia, and 45°C to detect normal nociception. Withdrawal latency is recorded. After exposure to UV light, injured larvae exhibit heightened behavioral responses to both sub-noxious and noxious stimuli, which suggest that this model serves to effectively investigate both allodynia and hyperalgesia (Babcock et al., 2009; McParland et al., 2021). Using this model, the Hedgehog (Hh), Bone Morphogenetic Protein (BMP), Tumor Necrosis Factor alpha (TNF-α), and Tackykinin (Tk) signaling pathway are found to regulate nociceptive sensitization in response to injury in flies (Babcock et al., 2009; Im et al., 2015; McParland et al., 2021).
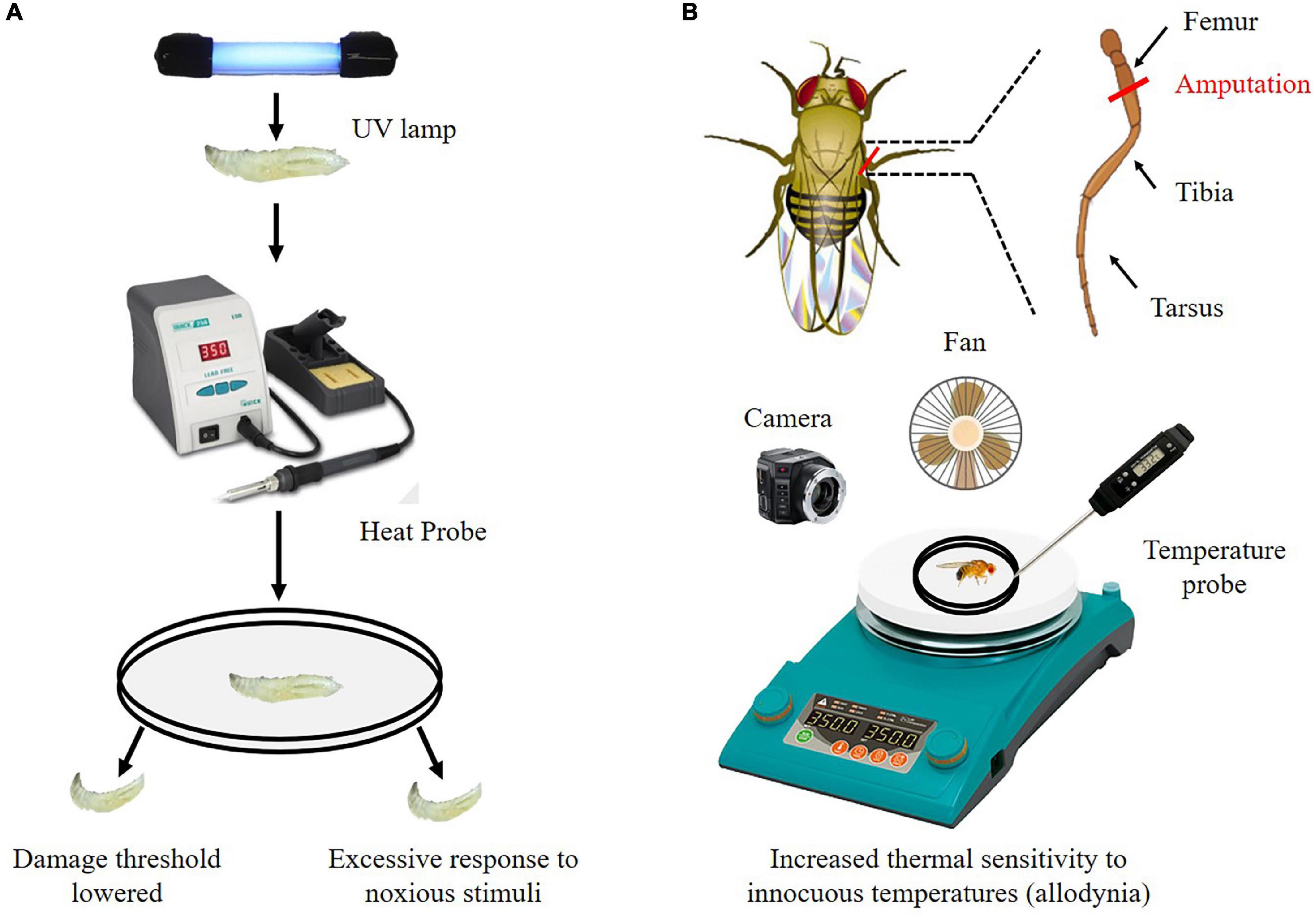
Figure 3. Detection method of chronic pain perception in flies. (A) Drosophila larvae have a reduced nociceptive temperature threshold and overreact to noxious temperature stimuli after exposure to UV light. (B) After amputation, adult flies were subjected to thermal stimulation. Adult flies have increased thermal sensitivity to innocuous temperatures (allodynia).
A novel adult fly model is developed for a chronic pain analysis process that adult flies show an increase response to a normally threshold temperature (allodynia) after they underwent a leg amputation surgery (Khuong et al., 2019; Massingham et al., 2021). Briefly, the right middle leg of adult fly is amputated at the femur segment using vannas scissors. After amputation, adult flies are fed individually in the vial containing fly food for 7 days. Then, flies are allowed to acclimate to the test chamber on a heating plate. The temperature of the heating plate is raised from 25 to 50°C over 3 min. A video recording camera positioned above the apparatus is used to record observations of flies. Jumping behavior and speed of movement are calculated according to the recorded videos (Figure 3B). This method allows for individualized analysis of allodynia and hyperalgesia.
In general, the current methods used to study the nociception of Drosophila flies are simple and easy to operate, and the equipment is extremely accessible. This makes it easier for researchers to investigate the genetics of acute and chronic pain in human using these tools and assays.
Development of Anti-Pain Drug Using Drosophila Models
Drosophila melanogaster are typically used for genetic studies but they also could be employed for drug discovery process (Lee and Min, 2019). The advantages of D. melanogaster qualified for drugs screening include the low cost of maintenance, the high reproductive capacity of propagation, and the rapidity of screening studies in the fly compared with traditional rat-based models. It places a high value on investigating new analgesics, especially, with evaluated conserved pain genes, responses and nature of nociception in parallel to human (Manev et al., 2003). Drugs can be delivered to the fruit fly by the following ways such as presented as a vapor (e.g., ethanol and cocaine) (McClung and Hirsh, 1998); either in the food or in the form of a filter paper from sucrose/drug-saturated (Nichols et al., 2002); drug can also be injected or dropped directly onto the exposed nerve cord of flies, of which have been decapitated (Torres and Horowitz, 1998); drugs injected into the abdomen where it quickly diffuses throughout the whole organism can also be available for a valid alternative (Dzitoyeva et al., 2003). In addition, the ability to perform high-throughput screening in flies through random mutation or targeted RNAi-mediated knockdown can further facilitate the identification of new drugs or drug targets (Bell et al., 2009).
Thus, the Drosophila fly model for screening putative analgesics appears to be beneficial for the discovery of new drugs. Currently, more and more researchers use fruit flies for pharmacological pain research. Discussions of pain in animals inevitably lead to anthropomorphic references. From a practical standpoint, the animal’s response to noxious stimuli and the ability of drug therapy to attenuate this response are important aspects of pain research. Excitation of gamma-aminobutyric acid B (GABAB) receptors by injecting agonist 3-aminopropyl-(methyl) phosphinic acid (3-APMPA) significantly prolong latency to heat response in adult flies, and the threshold for heat avoidance enhanced as the injected 3-APMPA concentration increase (Dzitoyeva et al., 2003; Manev and Dimitrijevic, 2004). The peptide Tv1 from Terebra variegata has an antinociceptive effect in adult flies, in which injection of Tv1 significantly reduces fly sensitivity to noxious heat (Eriksson et al., 2018). Three analogs of anesthetics (enflurane, isoflurane, and desflurane) can act at a same target as halothane, and decrease the sensitivity to avoid heat in flies that exposed to the heating induced by an intense beam of light (Campbell and Nash, 1994). Paclitaxel as a common chemotherapeutics against cancer can lead to chronic nociception. Consistently, paclitaxel exposure on the fruit fly larval nociception system result in a robust and dose-dependent increase in aversive escape response during a noxious thermal stimulus (Hamoudi et al., 2018). Paclitaxel has also been reported to be toxic in somatic cells, and causes loss of axons in peripheral nerves in Drosophila flies (Cunha et al., 2001).
Concluding Thoughts
As briefly addressed above, there have been several published work in which the fly have been displayed key features that an alternate option biology and physiology, even functional pain genes are well conserved from the fly to humans. The fruit fly applied for pain genomics and pharmacogenomics are devoted in the validation of primary small molecule, the research of the target discovery and the selection of high-throughput screening. However, many factors may participate in pain processes including change of extracellular microenvironment and break of balance in extracellular matrix metabolism, which are never discussed in flies. Pain-like emotions generated by motivational mechanisms are impossible to answer conclusively in flies. As for studies of painkillers in fly, the pharmacological action, the side effects and the best drug-delivery way have not been discussed as to whether they work as well in humans. Although the status of Drosophila as a pain research model is still somewhat different from that of mammals, its potential as a pain research model is being further explored, and its entry into the field of pain research may help reduce the pressure on mammals in vivo.
Author Contributions
JH, BL, SH, YZ, and KL: writing. MX and YL: manuscript editing. All authors contributed to the article and approved the submitted version.
Funding
This work received financial support from the National Natural Science Foundation of China (Nos. 82104562 and 82004228), “Double First Class” Scientific Research Key Project in Gansu (No. GSSYLXM-05), and Gansu Planning Projects on Science and Technology (No. 20JR10RA332).
Conflict of Interest
The authors declare that the research was conducted in the absence of any commercial or financial relationships that could be construed as a potential conflict of interest.
Publisher’s Note
All claims expressed in this article are solely those of the authors and do not necessarily represent those of their affiliated organizations, or those of the publisher, the editors and the reviewers. Any product that may be evaluated in this article, or claim that may be made by its manufacturer, is not guaranteed or endorsed by the publisher.
Footnotes
References
Adams, C. M., Anderson, M. G., Motto, D. G., Price, M. P., Johnson, W. A., and Welsh, M. J. (1998). Ripped pocket and pickpocket, novel Drosophila DEG/ENaC subunits expressed in early development and in mechanosensory neurons. J. Cell Biol. 140, 143–152. doi: 10.1083/jcb.140.1.143
Al-Anzi, B., Tracey, W. J., and Benzer, S. (2006). Response of Drosophila to wasabi is mediated by painless, the fly homolog of mammalian TRPA1/ANKTM1. Curr. Biol. 16, 1034–1040. doi: 10.1016/j.cub.2006.04.002
Aldrich, B. T., Kasuya, J., Faron, M., Ishimoto, H., and Kitamoto, T. (2010). The amnesiac gene is involved in the regulation of thermal nociception in Drosophila melanogaster. J. Neurogenet. 24, 33–41. doi: 10.3109/01677060903419751
Babcock, D. T., Landry, C., and Galko, M. J. (2009). Cytokine signaling mediates UV-induced nociceptive sensitization in Drosophila larvae. Curr. Biol. 19, 799–806. doi: 10.1016/j.cub.2009.03.062
Babcock, D. T., Shi, S., Jo, J., Shaw, M., Gutstein, H. B., and Galko, M. J. (2011). Hedgehog signaling regulates nociceptive sensitization. Curr. Biol. 21, 1525–1533. doi: 10.1016/j.cub.2011.08.020
Bagriantsev, S. N., Gracheva, E. O., and Gallagher, P. G. (2014). Piezo proteins: regulators of mechanosensation and other cellular processes. J. Biol. Chem. 289, 31673–31681. doi: 10.1074/jbc.R114.612697
Bamps, D., Vriens, J., de Hoon, J., and Voets, T. (2021). TRP channel cooperation for nociception: therapeutic opportunities. Annu. Rev. Pharmacol. Toxicol. 61, 655–677. doi: 10.1146/annurev-pharmtox-010919-023238
Bandell, M., Story, G. M., Hwang, S. W., Viswanath, V., Eid, S. R., Petrus, M. J., et al. (2004). Noxious cold ion channel TRPA1 is activated by pungent compounds and bradykinin. Neuron 41, 849–857. doi: 10.1016/s0896-6273(04)00150-3
Bautista, D. M., Jordt, S. E., Nikai, T., Tsuruda, P. R., Read, A. J., Poblete, J., et al. (2006). TRPA1 mediates the inflammatory actions of environmental irritants and proalgesic agents. Cell 124, 1269–1282. doi: 10.1016/j.cell.2006.02.023
Bell, A. (2018). The neurobiology of acute pain. Vet. J. 237, 55–62. doi: 10.1016/j.tvjl.2018.05.004
Bell, A. J., McBride, S. M. J., and Dockendorff, T. C. (2009). Flies as the ointment Drosophila modeling to enhance drug discovery. Fly 3, 39–49. doi: 10.4161/fly.3.1.7774
Bellemer, A. (2015). Thermotaxis, circadian rhythms, and TRP channels in Drosophila. Temperature (Austin) 2, 227–243. doi: 10.1080/23328940.2015.1004972
Benzer, S. (1967). Behavioral mutants of Drosophila isolated by countercurrent distribution. Proc. Natl. Acad. Sci. U.S.A. 58, 1112–1119. doi: 10.1073/pnas.58.3.1112
Bier, E. (2005). Drosophila, the golden bug, emerges as a tool for human genetics. Nat. Rev. Genet. 6, 9–23. doi: 10.1038/nrg1503
Boonen, B., Startek, J. B., Milici, A., Lopez-Requena, A., Beelen, M., Callaerts, P., et al. (2021). Activation of Drosophila melanogaster TRPA1 Isoforms by citronellal and menthol. Int. J. Mol. Sci. 22:10997. doi: 10.3390/ijms222010997
Bourne, S., Machado, A. G., and Nagel, S. J. (2014). Basic anatomy and physiology of pain pathways. Neurosurg. Clin. N. Am. 25, 629–638. doi: 10.1016/j.nec.2014.06.001
Burgos, A., Honjo, K., Ohyama, T., Qian, C. S., Shin, G. J., Gohl, D. M., et al. (2018). Nociceptive interneurons control modular motor pathways to promote escape behavior in Drosophila. eLife 7:e26016. doi: 10.7554/eLife.26016
Busto, G. U., Cervantes-Sandoval, I., and Davis, R. L. (2010). Olfactory learning in Drosophila. Physiology (Bethesda) 25, 338–346. doi: 10.1152/physiol.00026.2010
Campbell, D. B., and Nash, H. A. (1994). Use of Drosophila mutants to distinguish among volatile general anesthetics. Proc. Natl. Acad. Sci. U.S.A. 91, 2135–2139. doi: 10.1073/pnas.91.6.2135
Caterina, M. J., Leffler, A., Malmberg, A. B., Martin, W. J., Trafton, J., Petersen-Zeitz, K. R., et al. (2000). Impaired nociception and pain sensation in mice lacking the capsaicin receptor. Science 288, 306–313. doi: 10.1126/science.288.5464.306
Cheng, L. E., Song, W., Looger, L. L., Jan, L. Y., and Jan, Y. N. (2010). The role of the TRP channel NompC in Drosophila larval and adult locomotion. Neuron 67, 373–380. doi: 10.1016/j.neuron.2010.07.004
Chien, S., Reiter, L. T., Bier, E., and Gribskov, M. (2002). Homophila: human disease gene cognates in Drosophila. Nucleic Acids Res. 30, 149–151. doi: 10.1093/nar/30.1.149
Clapham, D. E., Runnels, L. W., and Strubing, C. (2001). The TRP ion channel family. Nat. Rev. Neurosci. 2, 387–396. doi: 10.1038/35077544
Corey, D. P., Garcia-Anoveros, J., Holt, J. R., Kwan, K. Y., Lin, S. Y., Vollrath, M. A., et al. (2004). TRPA1 is a candidate for the mechanosensitive transduction channel of vertebrate hair cells. Nature 432, 723–730. doi: 10.1038/nature03066
Cosens, D. J., and Manning, A. (1969). Abnormal electroretinogram from a Drosophila mutant. Nature 224, 285–287. doi: 10.1038/224285a0
Coste, B., Mathur, J., Schmidt, M., Earley, T. J., Ranade, S., Petrus, M. J., et al. (2010). Piezo1 and Piezo2 are essential components of distinct mechanically activated cation channels. Science 330, 55–60. doi: 10.1126/science.1193270
Coste, B., Xiao, B., Santos, J. S., Syeda, R., Grandl, J., Spencer, K. S., et al. (2012). Piezo proteins are pore-forming subunits of mechanically activated channels. Nature 483, 176–181. doi: 10.1038/nature10812
Costigan, M., Scholz, J., and Woolf, C. J. (2009). Neuropathic pain: a maladaptive response of the nervous system to damage. Annu. Rev. Neurosci. 32, 1–32. doi: 10.1146/annurev.neuro.051508.135531
Cunha, K. S., Reguly, M. L., Graf, U., and de Andrade, H. (2001). Taxanes: the genetic toxicity of paclitaxel and docetaxel in somatic cells of Drosophila melanogaster. Mutagenesis 16, 79–84. doi: 10.1093/mutage/16.1.79
Dannhauser, S., Lux, T. J., Hu, C., Selcho, M., Chen, J. T., Ehmann, N., et al. (2020). Antinociceptive modulation by the adhesion GPCR CIRL promotes mechanosensory signal discrimination. eLife 9:e56738. doi: 10.7554/eLife.56738
Davis, J. B., Gray, J., Gunthorpe, M. J., Hatcher, J. P., Davey, P. T., Overend, P., et al. (2000). Vanilloid receptor-1 is essential for inflammatory thermal hyperalgesia. Nature 405, 183–187. doi: 10.1038/35012076
Del, C. D., Murphy, S., Heiry, M., Barrett, L. B., Earley, T. J., Cook, C. A., et al. (2010). TRPA1 contributes to cold hypersensitivity. J. Neurosci. 30, 15165–15174. doi: 10.1523/JNEUROSCI.2580-10.2010
Di Meglio, S., Tramontano, F., Cimmino, G., Jones, R., and Quesada, P. (2004). Dual role for poly(ADP-ribose)polymerase-1 and -2 and poly(ADP-ribose)glycohydrolase as DNA-repair and pro-apoptotic factors in rat germinal cells exposed to nitric oxide donors. Biochim. Biophys. Acta 1692, 35–44. doi: 10.1016/j.bbamcr.2004.04.002
Dzitoyeva, S., Dimitrijevic, N., and Manev, H. (2003). Gamma-aminobutyric acid B receptor 1 mediates behavior-impairing actions of alcohol in Drosophila: adult RNA interference and pharmacological evidence. Proc. Natl. Acad. Sci. U.S.A. 100, 5485–5490. doi: 10.1073/pnas.0830111100
Eisfeld, J., and Luckhoff, A. (2007). Trpm2. Handb. Exp. Pharmacol. 179, 237–252. doi: 10.1007/978-3-540-34891-7_14
Enomoto, M., Siow, C., and Igaki, T. (2018). Drosophila as a cancer model. Adv. Exp. Med. Biol. 1076, 173–194. doi: 10.1007/978-981-13-0529-0_10
Eriksson, A., Anand, P., Gorson, J., Grijuc, C., Hadelia, E., Stewart, J. C., et al. (2018). Using Drosophila behavioral assays to characterize terebrid venom-peptide bioactivity. Sci. Rep. 8:15276. doi: 10.1038/s41598-018-33215-2
Faouzi, M., and Penner, R. (2014). Trpm2. Handb. Exp. Pharmacol. 222, 403–426. doi: 10.1007/978-3-642-54215-2_16
Flood, T. F., Gorczyca, M., White, B. H., Ito, K., and Yoshihara, M. (2013). A large-scale behavioral screen to identify neurons controlling motor programs in the Drosophila brain. G3 (Bethesda) 3, 1629–1637. doi: 10.1534/g3.113.006205
Fortini, M. E., Skupski, M. P., Boguski, M. S., and Hariharan, I. K. (2000). A survey of human disease gene counterparts in the Drosophila genome. J. Cell Biol. 150, F23–F30. doi: 10.1083/jcb.150.2.f23
Fowler, M. A., and Montell, C. (2013). Drosophila TRP channels and animal behavior. Life Sci. 92, 394–403. doi: 10.1016/j.lfs.2012.07.029
Gao, F. B., Brenman, J. E., Jan, L. Y., and Jan, Y. N. (1999). Genes regulating dendritic outgrowth, branching, and routing in Drosophila. Genes Dev. 13, 2549–2561. doi: 10.1101/gad.13.19.2549
Gees, M., Owsianik, G., Nilius, B., and Voets, T. (2012). TRP channels. Compr. Physiol. 2, 563–608. doi: 10.1002/cphy.c110026
Gjelsvik, K. J., Follansbee, T. L., and Ganter, G. K. (2018). Bone Morphogenetic Protein Glass Bottom Boat (BMP5/6/7/8) and its receptor Wishful Thinking (BMPRII) are required for injury-induced allodynia in Drosophila. Mol. Pain 14:2070360817. doi: 10.1177/1744806918802703
Glare, P., Aubrey, K. R., and Myles, P. S. (2019). Transition from acute to chronic pain after surgery. Lancet 393, 1537–1546. doi: 10.1016/S0140-6736(19)30352-6
Goodman, M. B. (2003). Sensation is painless. Trends Neurosci. 26, 643–645. doi: 10.1016/j.tins.2003.09.013
Gorczyca, D. A., Younger, S., Meltzer, S., Kim, S. E., Cheng, L., Song, W., et al. (2014). Identification of ppk26, a DEG/ENaC channel functioning with ppk1 in a mutually dependent manner to guide locomotion behavior in Drosophila. Cell Rep. 9, 1446–1458. doi: 10.1016/j.celrep.2014.10.034
Grueber, W. B., Jan, L. Y., and Jan, Y. N. (2002). Tiling of the Drosophila epidermis by multidendritic sensory neurons. Development 129, 2867–2878. doi: 10.1242/dev.129.12.2867
Grueber, W. B., Ye, B., Moore, A. W., Jan, L. Y., and Jan, Y. N. (2003). Dendrites of distinct classes of Drosophila sensory neurons show different capacities for homotypic repulsion. Curr. Biol. 13, 618–626. doi: 10.1016/s0960-9822(03)00207-0
Grueber, W. B., Ye, B., Yang, C. H., Younger, S., Borden, K., Jan, L. Y., et al. (2007). Projections of Drosophila multidendritic neurons in the central nervous system: links with peripheral dendrite morphology. Development 134, 55–64. doi: 10.1242/dev.02666
Guo, Y., Wang, Y., Wang, Q., and Wang, Z. (2014). The role of PPK26 in Drosophila larval mechanical nociception. Cell Rep. 9, 1183–1190. doi: 10.1016/j.celrep.2014.10.020
Haanpaa, M., Attal, N., Backonja, M., Baron, R., Bennett, M., Bouhassira, D., et al. (2011). NeuPSIG guidelines on neuropathic pain assessment. Pain 152, 14–27. doi: 10.1016/j.pain.2010.07.031
Hamada, F. N., Rosenzweig, M., Kang, K., Pulver, S. R., Ghezzi, A., Jegla, T. J., et al. (2008). An internal thermal sensor controlling temperature preference in Drosophila. Nature 454, 217–220. doi: 10.1038/nature07001
Hamoudi, Z., Khuong, T. M., Cole, T., and Neely, G. G. (2018). A fruit fly model for studying paclitaxel-induced peripheral neuropathy and hyperalgesia. F1000Res 7:99. doi: 10.12688/f1000research.13581.2
Hehlert, P., Zhang, W., and Gopfert, M. C. (2021). Drosophila mechanosensory transduction. Trends Neurosci. 44, 323–335. doi: 10.1016/j.tins.2020.11.001
Himmel, N. J., and Cox, D. N. (2017). Sensing the cold: TRP channels in thermal nociception. Channels (Austin) 11, 370–372. doi: 10.1080/19336950.2017.1336401
Honjo, K., Hwang, R. Y., and Tracey, W. J. (2012). Optogenetic manipulation of neural circuits and behavior in Drosophila larvae. Nat. Protoc. 7, 1470–1478. doi: 10.1038/nprot.2012.079
Honjo, K., and Tracey, W. J. (2018). BMP signaling downstream of the Highwire E3 ligase sensitizes nociceptors. PLoS Genet. 14:e1007464. doi: 10.1371/journal.pgen.1007464
Hoyer, N., Petersen, M., Tenedini, F., and Soba, P. (2018). Assaying mechanonociceptive behavior in Drosophila larvae. Bio Protoc. 8, e2736. doi: 10.21769/BioProtoc.2736
Hu, Y., Wang, Z., Liu, T., and Zhang, W. (2019). Piezo-like gene regulates locomotion in Drosophila larvae. Cell Rep. 26, 1369–1377. doi: 10.1016/j.celrep.2019.01.055
Hung, C. Y., and Tan, C. H. (2018). TRP channels in nociception and pathological pain. Adv. Exp. Med. Biol. 1099, 13–27. doi: 10.1007/978-981-13-1756-9_2
Hwang, R. Y., Stearns, N. A., and Tracey, W. D. (2012). The ankyrin repeat domain of the TRPA protein painless is important for thermal nociception but not mechanical nociception. PLoS One 7:e30090. doi: 10.1371/journal.pone.0030090
Hwang, R. Y., Zhong, L., Xu, Y., Johnson, T., Zhang, F., Deisseroth, K., et al. (2007). Nociceptive neurons protect Drosophila larvae from parasitoid wasps. Curr. Biol. 17, 2105–2116. doi: 10.1016/j.cub.2007.11.029
Hwang, S. W., and Oh, U. (2007). Current concepts of nociception: nociceptive molecular sensors in sensory neurons. Curr. Opin. Anaesthesiol. 20, 427–434. doi: 10.1097/ACO.0b013e3282eff91c
Im, S. H., Takle, K., Jo, J., Babcock, D. T., Ma, Z., Xiang, Y., et al. (2015). Tachykinin acts upstream of autocrine Hedgehog signaling during nociceptive sensitization in Drosophila. eLife 4:e10735. doi: 10.7554/eLife.10735
Im, S. H., and Galko, M. J. (2012). Pokes, sunburn, and hot sauce: Drosophila as an emerging model for the biology of nociception. Dev. Dyn. 241, 16–26. doi: 10.1002/dvdy.22737
Immke, D. C., and Gavva, N. R. (2006). The TRPV1 receptor and nociception. Semin. Cell Dev. Biol. 17, 582–591. doi: 10.1016/j.semcdb.2006.09.004
Jang, W., Lee, S., Choi, S. I., Chae, H. S., Han, J., Jo, H., et al. (2019). Impairment of proprioceptive movement and mechanical nociception in Drosophila melanogaster larvae lacking Ppk30, a Drosophila member of the Degenerin/Epithelial Sodium Channel family. Genes Brain Behav. 18:e12545. doi: 10.1111/gbb.12545
Julius, D. (2013). TRP channels and pain. Annu. Rev. Cell Dev. Biol. 29, 355–384. doi: 10.1146/annurev-cellbio-101011-155833
Kang, K., Pulver, S. R., Panzano, V. C., Chang, E. C., Griffith, L. C., Theobald, D. L., et al. (2010). Analysis of Drosophila TRPA1 reveals an ancient origin for human chemical nociception. Nature 464, 597–600. doi: 10.1038/nature08848
Karashima, Y., Talavera, K., Everaerts, W., Janssens, A., Kwan, K. Y., Vennekens, R., et al. (2009). TRPA1 acts as a cold sensor in vitro and in vivo. Proc. Natl. Acad. Sci. U.S.A. 106, 1273–1278. doi: 10.1073/pnas.0808487106
Kernan, M., Cowan, D., and Zuker, C. (1994). Genetic dissection of mechanosensory transduction: mechanoreception-defective mutations of Drosophila. Neuron 12, 1195–1206. doi: 10.1016/0896-6273(94)90437-5
Kernan, M. J. (2007). Mechanotransduction and auditory transduction in Drosophila. Pflugers Arch. 454, 703–720. doi: 10.1007/s00424-007-0263-x
Khuong, T. M., Wang, Q. P., Manion, J., Oyston, L. J., Lau, M. T., Towler, H., et al. (2019). Nerve injury drives a heightened state of vigilance and neuropathic sensitization in Drosophila. Sci. Adv. 5:w4099. doi: 10.1126/sciadv.aaw4099
Khuong, T. M., and Neely, G. G. (2013). Conserved systems and functional genomic assessment of nociception. FEBS J. 280, 5298–5306. doi: 10.1111/febs.12464
Kim, S. E., Coste, B., Chadha, A., Cook, B., and Patapoutian, A. (2012). The role of Drosophila Piezo in mechanical nociception. Nature 483, 209–212. doi: 10.1038/nature10801
Kim, S. H., Lee, Y., Akitake, B., Woodward, O. M., Guggino, W. B., and Montell, C. (2010). Drosophila TRPA1 channel mediates chemical avoidance in gustatory receptor neurons. Proc. Natl. Acad. Sci. U.S.A. 107, 8440–8445. doi: 10.1073/pnas.1001425107
Kolisek, M., Beck, A., Fleig, A., and Penner, R. (2005). Cyclic ADP-ribose and hydrogen peroxide synergize with ADP-ribose in the activation of TRPM2 channels. Mol. Cell 18, 61–69. doi: 10.1016/j.molcel.2005.02.033
Kwan, K. Y., Allchorne, A. J., Vollrath, M. A., Christensen, A. P., Zhang, D. S., Woolf, C. J., et al. (2006). TRPA1 contributes to cold, mechanical, and chemical nociception but is not essential for hair-cell transduction. Neuron 50, 277–289. doi: 10.1016/j.neuron.2006.03.042
Kwan, K. Y., and Corey, D. P. (2009). Burning cold: involvement of TRPA1 in noxious cold sensation. J. Gen. Physiol. 133, 251–256. doi: 10.1085/jgp.200810146
Kwon, Y., Shim, H. S., Wang, X., and Montell, C. (2008). Control of thermotactic behavior via coupling of a TRP channel to a phospholipase C signaling cascade. Nat. Neurosci. 11, 871–873. doi: 10.1038/nn.2170
Laursen, W. J., Anderson, E. O., Hoffstaetter, L. J., Bagriantsev, S. N., and Gracheva, E. O. (2015). Species-specific temperature sensitivity of TRPA1. Temperature (Austin) 2, 214–226. doi: 10.1080/23328940.2014.1000702
Lee, H., Naughton, N. N., Woods, J. H., and Ko, M. C. (2007). Effects of butorphanol on morphine-induced itch and analgesia in primates. Anesthesiology 107, 478–485. doi: 10.1097/01.anes.0000278876.20263.a7
Lee, S., and Min, K. (2019). Drosophila melanogaster as a model system in the study of pharmacological interventions in aging. Transl. Med. Aging 3, 98–103. doi: 10.1016/j.tma.2019.09.004
Lee, Y., Lee, Y., Lee, J., Bang, S., Hyun, S., Kang, J., et al. (2005). Pyrexia is a new thermal transient receptor potential channel endowing tolerance to high temperatures in Drosophila melanogaster. Nat. Genet. 37, 305–310. doi: 10.1038/ng1513
Leung, C., Wilson, Y., Khuong, T. M., and Neely, G. G. (2013). Fruit flies as a powerful model to drive or validate pain genomics efforts. Pharmacogenomics 14, 1879–1887. doi: 10.2217/pgs.13.196
Lewis, A. H., Cui, A. F., McDonald, M. F., and Grandl, J. (2017). Transduction of repetitive mechanical stimuli by piezo1 and piezo2 ion channels. Cell Rep. 19, 2572–2585. doi: 10.1016/j.celrep.2017.05.079
Li, H. (2017). TRP channel classification. Adv. Exp. Med. Biol. 976, 1–8. doi: 10.1007/978-94-024-1088-4_1
Li, Y., Bai, P., Wei, L., Kang, R., Chen, L., Zhang, M., et al. (2020). Capsaicin functions as Drosophila ovipositional repellent and causes intestinal dysplasia. Sci. Rep. 10:9963. doi: 10.1038/s41598-020-66900-2
Liedtke, W. (2007). TRPV channels’ role in osmotransduction and mechanotransduction. Handb. Exp. Pharmacol. 179, 473–487. doi: 10.1007/978-3-540-34891-7_28
Liu, L., Yermolaieva, O., Johnson, W. A., Abboud, F. M., and Welsh, M. J. (2003). Identification and function of thermosensory neurons in Drosophila larvae. Nat. Neurosci. 6, 267–273. doi: 10.1038/nn1009
Logashina, Y. A., Korolkova, Y. V., Kozlov, S. A., and Andreev, Y. A. (2019). TRPA1 channel as a regulator of neurogenic inflammation and pain: structure, function, role in pathophysiology, and therapeutic potential of ligands. Biochemistry (Mosc) 84, 101–118. doi: 10.1134/S0006297919020020
Lopez-Bellido, R., Himmel, N. J., Gutstein, H. B., Cox, D. N., and Galko, M. J. (2019). An assay for chemical nociception in Drosophila larvae. Philos. Trans. R. Soc. Lond. B Biol. Sci. 374:20190282. doi: 10.1098/rstb.2019.0282
Luo, J., Shen, W. L., and Montell, C. (2017). TRPA1 mediates sensation of the rate of temperature change in Drosophila larvae. Nat. Neurosci. 20, 34–41. doi: 10.1038/nn.4416
Luo, X., Huh, Y., Bang, S., He, Q., Zhang, L., Matsuda, M., et al. (2019). Macrophage toll-like receptor 9 contributes to Chemotherapy-Induced neuropathic pain in male mice. J. Neurosci. 39, 6848–6864. doi: 10.1523/JNEUROSCI.3257-18.2019
Ma, W., and Quirion, R. (2007). Inflammatory mediators modulating the transient receptor potential vanilloid 1 receptor: therapeutic targets to treat inflammatory and neuropathic pain. Expert Opin. Ther. Targets 11, 307–320. doi: 10.1517/14728222.11.3.307
Maiese, K. (2017). Warming up to new possibilities with the capsaicin receptor TRPV1: MTOR, AMPK, and erythropoietin. Curr. Neurovasc. Res. 14, 184–189. doi: 10.2174/1567202614666170313105337
Malafoglia, V., Bryant, B., Raffaeli, W., Giordano, A., and Bellipanni, G. (2013). The zebrafish as a model for nociception studies. J. Cell Physiol. 228, 1956–1966. doi: 10.1002/jcp.24379
Mandel, S. J., Shoaf, M. L., Braco, J. T., Silver, W. L., and Johnson, E. C. (2018). Behavioral aversion to AITC requires both painless and dTRPA1 in drosophila. Front. Neural Circuits 12:45. doi: 10.3389/fncir.2018.00045
Manev, H., Dimitrijevic, N., and Dzitoyeva, S. (2003). Techniques: fruit flies as models for neuropharmacological research. Trends Pharmacol. Sci. 24, 41–43. doi: 10.1016/S0165-6147(02)00004-4
Manev, H., and Dimitrijevic, N. (2004). Drosophila model for in vivo pharmacological analgesia research. Eur. J. Pharmacol. 491, 207–208. doi: 10.1016/j.ejphar.2004.03.030
Massingham, J. N., Baron, O., and Neely, G. G. (2021). Evaluating baseline and sensitised heat nociception in adult Drosophila. Bio Protoc. 11:e4079. doi: 10.21769/BioProtoc.4079
Mauthner, S. E., Hwang, R. Y., Lewis, A. H., Xiao, Q., Tsubouchi, A., Wang, Y., et al. (2014). Balboa binds to pickpocket in vivo and is required for mechanical nociception in Drosophila larvae. Curr. Biol. 24, 2920–2925. doi: 10.1016/j.cub.2014.10.038
McClung, C., and Hirsh, J. (1998). Stereotypic behavioral responses to free-base cocaine and the development of behavioral sensitization in Drosophila. Curr. Biol. 8, 109–112. doi: 10.1016/s0960-9822(98)70041-7
McParland, A., Moulton, J., Brann, C., Hale, C., Otis, Y., and Ganter, G. (2021). The brinker repressor system regulates injury-induced nociceptive sensitization in Drosophila melanogaster. Mol. Pain 17:794238681. doi: 10.1177/17448069211037401
Merritt, D. J., and Whitington, P. M. (1995). Central projections of sensory neurons in the Drosophila embryo correlate with sensory modality, soma position, and proneural gene function. J. Neurosci. 15, 1755–1767. doi: 10.1523/JNEUROSCI.15-03-01755.1995
Milinkeviciute, G., Gentile, C., and Neely, G. G. (2012). Drosophila as a tool for studying the conserved genetics of pain. Clin Genet 82, 359–366. doi: 10.1111/j.1399-0004.2012.01941.x
Minke, B., Wu, C., and Pak, W. L. (1975). Induction of photoreceptor voltage noise in the dark in Drosophila mutant. Nature 258, 84–87. doi: 10.1038/258084a0
Murthy, S. E., Loud, M. C., Daou, I., Marshall, K. L., Schwaller, F., Kühnemund, J., et al. (2018). The mechanosensitive ion channel Piezo2 mediates sensitivity to mechanical pain in mice. Sci. Transl. Med. 10:eaat9897. doi: 10.1126/scitranslmed.aat9897
Nassini, R., Materazzi, S., Benemei, S., and Geppetti, P. (2014). The TRPA1 channel in inflammatory and neuropathic pain and migraine. Rev. Physiol. Biochem. Pharmacol. 167, 1–43. doi: 10.1007/112_2014_18
Neely, G. G., Hess, A., Costigan, M., Keene, A. C., Goulas, S., Langeslag, M., et al. (2010). A genome-wide Drosophila screen for heat nociception identifies alpha2delta3 as an evolutionarily conserved pain gene. Cell 143, 628–638. doi: 10.1016/j.cell.2010.09.047
Neely, G. G., Keene, A. C., Duchek, P., Chang, E. C., Wang, Q. P., Aksoy, Y. A., et al. (2011). TrpA1 regulates thermal nociception in Drosophila. PLoS One 6:e24343. doi: 10.1371/journal.pone.0024343
Nichols, C. D., Ronesi, J., Pratt, W., and Sanders-Bush, E. (2002). Hallucinogens and Drosophila: linking serotonin receptor activation to behavior. Neuroscience 115, 979–984. doi: 10.1016/s0306-4522(02)00354-8
Nilius, B., Appendino, G., and Owsianik, G. (2012). The transient receptor potential channel TRPA1: from gene to pathophysiology. Pflugers Arch. 464, 425–458. doi: 10.1007/s00424-012-1158-z
Nilius, B., Owsianik, G., Voets, T., and Peters, J. A. (2007). Transient receptor potential cation channels in disease. Physiol. Rev. 87, 165–217. doi: 10.1152/physrev.00021.2006
Nilius, B., Voets, T., and Peters, J. (2005). TRP channels in disease. Sci. Stke 2005:e8. doi: 10.1126/stke.2952005re8
Nilius, B., and Owsianik, G. (2011). The transient receptor potential family of ion channels. Genome Biol. 12:218. doi: 10.1186/gb-2011-12-3-218
Nkambeu, B., Salem, J. B., and Beaudry, F. (2020). Capsaicin and its analogues impede nocifensive response of Caenorhabditis elegans to noxious heat. Neurochem. Res. 45, 1851–1859. doi: 10.1007/s11064-020-03049-4
Ohyama, T., Jovanic, T., Denisov, G., Dang, T. C., Hoffmann, D., Kerr, R. A., et al. (2013). High-throughput analysis of stimulus-evoked behaviors in Drosophila larva reveals multiple modality-specific escape strategies. PLoS One 8:e71706. doi: 10.1371/journal.pone.0071706
Oswald, M., Rymarczyk, B., Chatters, A., and Sweeney, S. T. (2011). A novel thermosensitive escape behavior in Drosophila larvae. Fly (Austin) 5, 304–306. doi: 10.4161/fly.5.4.17810
Pazienza, V., Pomara, C., Cappello, F., Calogero, R., Carrara, M., Mazzoccoli, G., et al. (2014). The TRPA1 channel is a cardiac target of mIGF-1/SIRT1 signaling. Am. J. Physiol. Heart Circ. Physiol. 307, H939–H944. doi: 10.1152/ajpheart.00150.2014
Petersen, M., Tenedini, F., Hoyer, N., Kutschera, F., and Soba, P. (2018). Assaying thermo-nociceptive behavior in Drosophila larvae. Biol. Protoc. 8:e2737. doi: 10.21769/BioProtoc.2737
Ranade, S. S., Woo, S. H., Dubin, A. E., Moshourab, R. A., Wetzel, C., Petrus, M., et al. (2014). Piezo2 is the major transducer of mechanical forces for touch sensation in mice. Nature 516, 121–125. doi: 10.1038/nature13980
Rosenzweig, M., Brennan, K. M., Tayler, T. D., Phelps, P. O., Patapoutian, A., and Garrity, P. A. (2005). The Drosophila ortholog of vertebrate TRPA1 regulates thermotaxis. Genes Dev. 19, 419–424. doi: 10.1101/gad.1278205
Sakai, T., Sato, S., Ishimoto, H., and Kitamoto, T. (2012). Significance of the centrally expressed TRP channel painless in Drosophila courtship memory. Learn. Mem. 20, 34–40. doi: 10.1101/lm.029041.112
Sakai, T., Watanabe, K., Ohashi, H., Sato, S., Inami, S., Shimada, N., et al. (2014). Insulin-producing cells regulate the sexual receptivity through the painless TRP channel in Drosophila virgin females. PLoS One 9:e88175. doi: 10.1371/journal.pone.0088175
Schrader, S., and Merritt, D. J. (2000). Central projections of Drosophila sensory neurons in the transition from embryo to larva. J. Comp. Neurol. 425, 34–44. doi: 10.1002/1096-9861(20000911)425:1<34::aid-cne4>3.0.co;2-g
Servin-Vences, M. R., Moroni, M., Lewin, G. R., and Poole, K. (2017). Direct measurement of TRPV4 and PIEZO1 activity reveals multiple mechanotransduction pathways in chondrocytes. eLife 6:e21074. doi: 10.7554/eLife.21074
Sneddon, L. U. (2018). Comparative physiology of nociception and pain. Physiology (Bethesda) 33, 63–73. doi: 10.1152/physiol.00022.2017
Sokabe, T., and Tominaga, M. (2009). A temperature-sensitive TRP ion channel, Painless, functions as a noxious heat sensor in fruit flies. Commun. Integr. Biol. 2, 170–173. doi: 10.4161/cib.7708
Song, Y., Li, D., Farrelly, O., Miles, L., Li, F., Kim, S. E., et al. (2019). The mechanosensitive ion channel piezo inhibits axon regeneration. Neuron 102, 373–389. doi: 10.1016/j.neuron.2019.01.050
St, J. S. E. (2018). Advances in understanding nociception and neuropathic pain. J. Neurol. 265, 231–238. doi: 10.1007/s00415-017-8641-6
Story, G. M., Peier, A. M., Reeve, A. J., Eid, S. R., Mosbacher, J., Hricik, T. R., et al. (2003). ANKTM1, a TRP-like channel expressed in nociceptive neurons, is activated by cold temperatures. Cell 112, 819–829. doi: 10.1016/s0092-8674(03)00158-2
Sun, Y., Liu, L., Ben-Shahar, Y., Jacobs, J. S., Eberl, D. F., and Welsh, M. J. (2009). TRPA channels distinguish gravity sensing from hearing in Johnston’s organ. Proc. Natl. Acad. Sci. U.S.A. 106, 13606–13611. doi: 10.1073/pnas.0906377106
Szczot, M., Liljencrantz, J., Ghitani, N., Barik, A., Lam, R., Thompson, J. H., et al. (2018). PIEZO2 mediates injury-induced tactile pain in mice and humans. Sci. Transl. Med. 10:eaat9892. doi: 10.1126/scitranslmed.aat9892
Tang, X., Platt, M. D., Lagnese, C. M., Leslie, J. R., and Hamada, F. N. (2013). Temperature integration at the AC thermosensory neurons in Drosophila. J. Neurosci. 33, 894–901. doi: 10.1523/JNEUROSCI.1894-12.2013
Togashi, K., Hara, Y., Tominaga, T., Higashi, T., Konishi, Y., Mori, Y., et al. (2006). TRPM2 activation by cyclic ADP-ribose at body temperature is involved in insulin secretion. EMBO J. 25, 1804–1815. doi: 10.1038/sj.emboj.7601083
Torres, G., and Horowitz, J. M. (1998). Activating properties of cocaine and cocaethylene in a behavioral preparation of Drosophila melanogaster. Synapse 29, 148–161. doi: 10.1002/(SICI)1098-2396(199806)29:2<148::AID-SYN6>3.0.CO;2-7
Tracey, W. J., Wilson, R. I., Laurent, G., and Benzer, S. (2003). Painless, a Drosophila gene essential for nociception. Cell 113, 261–273. doi: 10.1016/s0092-8674(03)00272-1
Tsubouchi, A., Caldwell, J. C., and Tracey, W. D. (2012). Dendritic filopodia, Ripped Pocket, NOMPC, and NMDARs contribute to the sense of touch in Drosophila larvae. Curr. Biol. 22, 2124–2134. doi: 10.1016/j.cub.2012.09.019
Tsuda, L., and Lim, Y. M. (2018). Alzheimer’s disease model system using drosophila. Adv. Exp. Med. Biol. 1076, 25–40. doi: 10.1007/978-981-13-0529-0_3
Turk, D. C. (2001). Management of pain: best of times, worst of times? Clin. J. Pain 17, 107–109. doi: 10.1097/00002508-200106000-00001
Turner, H. N., Armengol, K., Patel, A. A., Himmel, N. J., Sullivan, L., Iyer, S. C., et al. (2016). The TRP channels pkd2, NompC, and trpm act in Cold-Sensing neurons to mediate unique aversive behaviors to noxious cold in Drosophila. Curr. Biol. 26, 3116–3128. doi: 10.1016/j.cub.2016.09.038
Turner, H. N., Landry, C., and Galko, M. J. (2017). Novel assay for cold nociception in Drosophila larvae. J. Vis. Exp. 3:55568. doi: 10.3791/55568
Viana, F. (2016). TRPA1 channels: molecular sentinels of cellular stress and tissue damage. J. Physiol. 594, 4151–4169. doi: 10.1113/JP270935
Volkers, L., Mechioukhi, Y., and Coste, B. (2015). Piezo channels: from structure to function. Pflugers Arch. 467, 95–99. doi: 10.1007/s00424-014-1578-z
Voscopoulos, C., and Lema, M. (2010). When does acute pain become chronic? Br. J. Anaesth. 105(Suppl 1), i69–i85. doi: 10.1093/bja/aeq323
Waldmann, R., Champigny, G., Bassilana, F., Heurteaux, C., and Lazdunski, M. (1997). A proton-gated cation channel involved in acid-sensing. Nature 386, 173–177. doi: 10.1038/386173a0
Wang, K., Guo, Y., Wang, F., and Wang, Z. (2011). Drosophila TRPA channel painless inhibits male-male courtship behavior through modulating olfactory sensation. PLoS One 6:e25890. doi: 10.1371/journal.pone.0025890
Wang, P., Jia, Y., Liu, T., Jan, Y. N., and Zhang, W. (2020). Visceral mechano-sensing neurons control Drosophila feeding by using piezo as a sensor. Neuron 108, 640–650. doi: 10.1016/j.neuron.2020.08.017
Warr, C. G., Shaw, K. H., Azim, A., Piper, M., and Parsons, L. M. (2018). Using mouse and Drosophila models to investigate the mechanistic links between diet, obesity, type II diabetes, and cancer. Int. J. Mol. Sci. 19:4110. doi: 10.3390/ijms19124110
Williams, D. W., and Truman, J. W. (2005). Cellular mechanisms of dendrite pruning in Drosophila: insights from in vivo time-lapse of remodeling dendritic arborizing sensory neurons. Development 132, 3631–3642. doi: 10.1242/dev.01928
Wolfgang, W., Simoni, A., Gentile, C., and Stanewsky, R. (2013). The Pyrexia transient receptor potential channel mediates circadian clock synchronization to low temperature cycles in Drosophila melanogaster. Proc. Biol. Sci. 280:20130959. doi: 10.1098/rspb.2013.0959
Woo, S. H., Lukacs, V., de Nooij, J. C., Zaytseva, D., Criddle, C. R., Francisco, A., et al. (2015). Piezo2 is the principal mechanotransduction channel for proprioception. Nat. Neurosci. 18, 1756–1762. doi: 10.1038/nn.4162
Woolf, C. J. (2010). What is this thing called pain? J. Clin. Invest. 120, 3742–3744. doi: 10.1172/JCI45178
Wu, L. J., Sweet, T. B., and Clapham, D. E. (2010). International Union of Basic and Clinical Pharmacology. LXXVI. Current progress in the mammalian TRP ion channel family. Pharmacol. Rev. 62, 381–404. doi: 10.1124/pr.110.002725
Xiang, Y., Yuan, Q., Vogt, N., Looger, L. L., Jan, L. Y., and Jan, Y. N. (2010). Light-avoidance-mediating photoreceptors tile the Drosophila larval body wall. Nature 468, 921–926. doi: 10.1038/nature09576
Xu, J., Sornborger, A. T., Lee, J. K., and Shen, P. (2008). Drosophila TRPA channel modulates sugar-stimulated neural excitation, avoidance and social response. Nat. Neurosci. 11, 676–682. doi: 10.1038/nn.2119
Xu, S. Y., Cang, C. L., Liu, X. F., Peng, Y. Q., Ye, Y. Z., Zhao, Z. Q., et al. (2006). Thermal nociception in adult Drosophila: behavioral characterization and the role of the painless gene. Genes Brain Behav. 5, 602–613. doi: 10.1111/j.1601-183X.2006.00213.x
Yam, M. F., Loh, Y. C., Tan, C. S., Khadijah, A. S., Abdul, M. N., and Basir, R. (2018). General pathways of pain sensation and the major neurotransmitters involved in pain regulation. Int. J. Mol. Sci. 19:2164. doi: 10.3390/ijms19082164
Yan, Z., Zhang, W., He, Y., Gorczyca, D., Xiang, Y., Cheng, L. E., et al. (2013). Drosophila NOMPC is a mechanotransduction channel subunit for gentle-touch sensation. Nature 493, 221–225. doi: 10.1038/nature11685
Yoshino, J., Morikawa, R. K., Hasegawa, E., and Emoto, K. (2017). Neural circuitry that evokes escape behavior upon activation of nociceptive sensory neurons in drosophila larvae. Curr. Biol. 27, 2499–2504. doi: 10.1016/j.cub.2017.06.068
Young, E. E., Lariviere, W. R., and Belfer, I. (2012). Genetic basis of pain variability: recent advances. J. Med. Genet. 49, 1–9. doi: 10.1136/jmedgenet-2011-100386
Yu, Y., Chen, Z., Li, W. G., Cao, H., Feng, E. G., Yu, F., et al. (2010). A nonproton ligand sensor in the acid-sensing ion channel. Neuron 68, 61–72. doi: 10.1016/j.neuron.2010.09.001
Zhong, L., Hwang, R. Y., and Tracey, W. D. (2010). Pickpocket is a DEG/ENaC protein required for mechanical nociception in Drosophila larvae. Curr. Biol. 20, 429–434. doi: 10.1016/j.cub.2009.12.057
Keywords: nociception, conserved genetics, nociceptive sensory neurons, behavioral assay, Drosophila melanogaster
Citation: He J, Li B, Han S, Zhang Y, Liu K, Yi S, Liu Y and Xiu M (2022) Drosophila as a Model to Study the Mechanism of Nociception. Front. Physiol. 13:854124. doi: 10.3389/fphys.2022.854124
Received: 13 January 2022; Accepted: 28 February 2022;
Published: 28 March 2022.
Edited by:
Susumu Ohya, Nagoya City University, JapanReviewed by:
Yong Fang Zhu, McMaster University, CanadaFrancisco J. Taberner, Institute of Neurosciences (CSIC), Spain
Copyright © 2022 He, Li, Han, Zhang, Liu, Yi, Liu and Xiu. This is an open-access article distributed under the terms of the Creative Commons Attribution License (CC BY). The use, distribution or reproduction in other forums is permitted, provided the original author(s) and the copyright owner(s) are credited and that the original publication in this journal is cited, in accordance with accepted academic practice. No use, distribution or reproduction is permitted which does not comply with these terms.
*Correspondence: Yongqi Liu, bGl1eW9uZ3FpNzNAMTYzLmNvbQ==; Minghui Xiu, eGl1bWluZ2h1aTg3QDE2My5jb20=
†These authors have contributed equally to this work