- 1Department of Physiology and Biophysics, Virginia Commonwealth University, Richmond, VA, United States
- 2Research and Development, Hemanext Inc., Lexington, MA, United States
- 3Medical Affairs, Hemanext Inc., Lexington, MA, United States
The ability to store red blood cells (RBCs) and other components for extended periods of time has expanded the availability and use of transfusion as a life-saving therapy. However, conventional RBC storage has a limited window of effective preservation and is accompanied by the progressive accumulation of a series of biochemical and morphological modifications, collectively referred to as “storage lesions.” These lesions have been associated with negative clinical outcomes (i.e., postoperative complications as well as reduced short-term and long-term survival) in patients transfused with conventionally stored blood with older and deteriorated transfused red cells. Hence, there is an increased unmet need for improved RBC storage. Hypoxic storage of blood entails the removal of large amounts of oxygen to low levels prior to refrigeration and maintenance of hypoxic levels through the entirety of storage. As opposed to conventionally stored blood, hypoxic storage can lead to a reduction of oxidative damage to slow storage lesion development and create a storage condition expected to result in enhanced efficacy of stored RBCs without an effect on oxygen exchange in the lung. Hypoxic blood transfusions appear to offer minimal safety concerns, even in patients with hypoxemia. This review describes the physiology of hypoxically stored blood, how it differs from conventionally stored blood, and its use in potential clinical application, such as massively transfused and critically ill patients with oxygenation/ventilation impairments.
Introduction
Blood transfusions are the most common hospital procedures in the United States, with more than 11 million red blood cell (RBC) units transfused every year (Jones et al., 2019; D’Alessandro et al., 2020). The ability to store RBCs and other components for extended periods of time has dramatically expanded the availability and use of transfusion as a life-saving therapy (D’Alessandro et al., 2020). In conventional blood transfusion, storage of RBCs in blood banks allows for preservation of RBC units under refrigerated conditions and additive solutions for up to 42 days in most countries (D’Alessandro et al., 2020). As soon as whole blood is collected from a donor, red blood cells begin to degrade, and transfusion of stored RBCs, particularly those at the end of the approved shelf life, have been implicated in adverse clinical outcomes (Flegel et al., 2014). Storage is accompanied by the progressive accumulation of a series of biochemical and morphological modifications, collectively referred to as the “storage lesion” (Yoshida et al., 2019). Storage lesions have been associated with adverse events and increased mortality after transfusion, increasing the need for improved RBC storage protocols (Yoshida et al., 2019). Hypoxic storage of blood, where the oxygen content of RBC units is reduced to low levels prior to refrigeration and maintained at hypoxic levels through the entirety of storage, has been proposed as a method of blood storage to slow storage lesion development by reducing oxidative stress. Patients who receive hypoxic blood may be critically ill and may have significant oxygenation/ventilation impairments. Some may even require mechanical ventilatory support. In this review article, we will discuss the theoretical implication of using hypoxically stored blood in patients with oxygenation/ventilation impairments.
Conventionally Processed and Stored Blood
Typically, RBC units prepared from donors are characterized by fractional O2 saturation of hemoglobin (SO2) ranging from 30–68% (49 ± 19) with corresponding PO2 of about 70 mmHg (Dumont et al., 2016; Yoshida et al., 2021). Leukocytes and plasma usually are removed before RBC storage under refrigerated conditions (≈2–6°C). When RBCs are separated from plasma, the packed RBCs are stored in a solution formulated to achieve post-transfusion survival of at least 75% in healthy autologous donors, with less than 1% pre-transfusion hemolysis while maintaining adequate levels of adenosine triphosphate (ATP). The storage bag is made of an oxygen-permeable Polyvinyl Chloride (PVC) film and placed in room air (PO2 ≈155 mmHg; PCO2 ≈0 mmHg), gradually gaining oxygen during storage estimated to reach SO2 of 59–94% at day 42 (Yoshida et al., 2021).
Pertinent Changes in Red Blood Cell Physiology During Storage
One of the most notable changes during RBC storage is the rapid fall of 2,3 bisphosphoglycerate or 2,3-disphosphoglycerate (2,3-DPG), resulting in higher oxygen affinity to hemoglobin and thus impaired off-loading to body tissues (Benesch and Benesch, 1967; MacDonald, 1977). Levels of 2,3-DPG have been shown to fall quickly during current methods of storage of RBCs, becoming nearly undetectable within 1–3 weeks. In RBCs, ATP is produced by anaerobic glycolysis, the end-product of which is lactic acid. During hypothermic storage, ATP initially rises but then falls below pre-storage levels; the magnitude of the decrease is dependent on the composition of the storage solution and pH of the RBC suspension (Tomaiuolo, 2014). The fall in ATP is associated with alterations in RBCs that lead to a loss of deformability, among other changes (Haradin et al., 1969). Oxygen is the substrate for reactions causing oxidative damage and thus the major driver of storage lesion development. Oxidation of hemoglobin to methemoglobin initiates a cascade of reactions that damage RBCs and produce potentially harmful lipid oxidation products. Loss of RBC integrity during storage results in intravascular and extravascular hemolysis after transfusion, releasing hemoglobin and iron from hemoglobin as the body attempts to clear the cellular debris from its circulatory system. These by-products of oxidative stress can have an inflammatory effect on recipients. Therefore, the residual and rising levels of oxygen through the PVC bag within the storage environment can have a deleterious effect on preservation of normal RBC function (Yoshida and Shevkoplyas, 2010; Yoshida et al., 2017, 2019).
Clinical Implications of Storage Lesions
Deterioration of RBCs has been associated with numerous transfusion-related adverse events; however, the direct link between the storage lesion and transfusion side-effects is not completely understood. Using storage age as a surrogate for accumulated storage lesion, several large-scale randomized controlled trials (RCTs) failed to associate negative primary outcomes to transfusion of moderately aged RBCs (14–21 days, or standard practice) compared to “fresh” (aged 7–10 days) RBCs. These RCTs primary outcomes excluded evaluation of transfusing “old” (aged 35–42 days) RBCs or effects of high-volume transfusion. However, multiple reports mainly based on retrospective investigations have suggested a linkage to hyper-coagulability, inflammation, impaired perfusion, immuno-modulation, organ dysfunction, and mortality (Jones et al., 2019; Yoshida et al., 2019). Negative clinical outcomes have been reported in cardiac surgery when using “older” blood (Sanders et al., 2011). In patients undergoing cardiac surgery, transfusion of red cells stored for more than 2 weeks was associated with a significantly increased risk of postoperative complications as well as reduced short-term and long-term survival (Koch et al., 2008). Transfusion with stored allogeneic RBCs, but not autologous salvaged RBCs, is associated with a decrease in RBC cell membrane deformability that is dose-dependent and may persist beyond 3 postoperative days (Salaria et al., 2014). Multiple organ failure in surgical, pediatric ICU, and trauma ICU patients is associated with advanced age of transfused red cells (Zallen et al., 1999; Spinella et al., 2009; Karam et al., 2010). Failure to improve O2 utilization has been attributed to decreased 2,3-DPG and decreased cardiac index associated with increased blood viscosity (Shah et al., 1982). In addition, aged, transfused RBCs have been correlated with increased odds of postoperative delirium in patients undergoing cardiac surgery (Brown et al., 2014).
Hypoxically Processed and Stored Blood
The variability of oxygen levels in donated blood and increasing oxygen concentration due to the permeability of current storage bags ensures that conventionally stored blood will be subject to accumulating damage and thus potentially prone to all the consequences of compromised flow and oxygenation characteristics following transfusion. The removal of large amounts of oxygen can lead to reduction of oxidative damage and create a storage condition which will be expected to lead to enhanced efficacy of the stored product (Figure 1; Yoshida and Shevkoplyas, 2010). The oxygen reduction process also lowers PCO2 below 10 mmHg (hypocapnia), raises pH, and enhances 2,3-DPG production (Dumont et al., 2016).
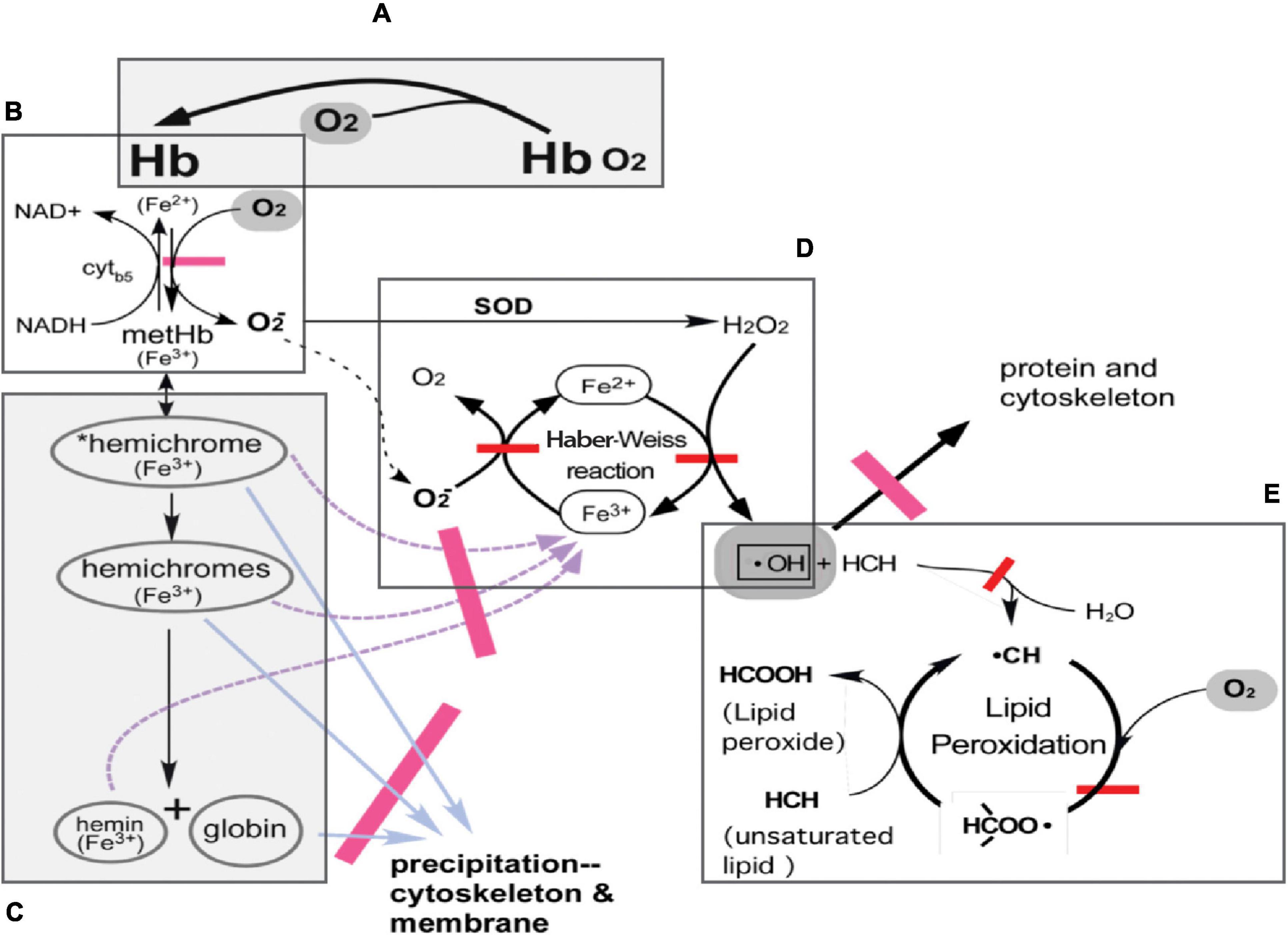
Figure 1. Hemoglobin and pathways of oxidative damage in RBC. (A) Normal function of Hb-reversible binding of O2 to reduced (ferrous) hemes in Hb. (B) Auto-oxidation of oxyHb to methemoglobin (metHb; ferric) with production of superoxide anion. In a steady state, 1–2% of Hb exists as metHb in the circulation; metHb is readily reduced back to ferrous Hb by NADH-linked cytochrome b5 metHb reductase. (C) Denaturation of metHb. MetHb denatures first to *reversible hemichromes, in which conformational distortions are minor and can still be reversed. *Reversible hemichromes further denature to “irreversible hemichromes,” which subsequently dissociate to globins and the heme moiety. (D) The Haber-Weiss reaction produces hydroxyl radicals, •OH. Superoxide anions generated in the production of metHb are converted into H2O2 by superoxide dismutase. Hydroxyl radicals are produced with H2O2 and ferrous iron from denatured metHb products functioning as Fenton reagents. Ferric iron is reduced by superoxide anions. Hydroxyl radicals oxidize and cross-link RBC proteins in their vicinity. (E) Lipid peroxidation cycle. Hydroxyl radicals in the membrane attack unsaturated lipids to form lipid radicals, then combine with molecular oxygen to form lipid peroxyl radicals, which in turn attack unsaturated lipid to complete the cycle. Adapted from Yoshida and Shevkoplyas (2010).
Hypoxic/hypocapnic blood has been shown to increase ATP (Hess, 2014) and 2,3 DPG levels up to 42 days of storage compared to conventional RBCs, and is characterized by a higher P50, indicative of better oxygen off-loading (Whitley et al., 2018, 2020). Furthermore, it has been reported that anaerobic blood storage is capable of considerably decreasing the amount of non-deformable cells present in the overall population of relatively well-preserved RBCs (Burns et al., 2016). A theoretical concern is the effect of transfusion of hypoxic blood on the pulmonary system in critically ill patients and those receiving massive transfusions.
Oxygen Exchange in the Lung
One key difference between conventionally stored blood and hypoxically stored blood is that PO2 and SO2 in transfused hypoxic blood will be significantly below the mean values of conventional RBCs (D’Alessandro et al., 2020; Whitley et al., 2020). A question of interest pertains to the potential impact one would expect hypoxically stored blood to have on oxygen uptake in the lung. For a normal, healthy lung oxygen and carbon dioxide exchange are perfusion-limited, meaning that the exchange of both these gases is complete by the time RBCs have traversed about one-third of the gas exchange region containing the pulmonary capillaries and the nearby alveoli (Pittman, 2011).
Thus, structurally, the lung has a built-in safety margin of a factor of 3 (i.e., 1/0.33) for exchange of the oxygen that enters from the systemic venous blood flowing to the lung from the pulmonary artery. The PvO2 is normally ∼40 mmHg, compared to alveolar PO2 of ∼100 mmHg, giving an initial PO2 difference of 60 mmHg driving oxygen uptake by the RBCs (Powers and Dhamoon, 2021, Aug 20). In a worst-case scenario for the entry of only deoxygenated transfused RBCs into the gas exchange region of the lung, the PO2 difference between the entering anoxic RBCs and alveolar PO2 would be increased to 100 mmHg, almost a factor of 2.
The initial rate of oxygen uptake is also proportional to this PO2 difference, so that a similar degree of equilibration occurs when blood has traversed about 40% of the gas exchange contact region and the margin of safety for gas exchange would be reduced a small amount from 3 (1/0.33) to 2.5 (1/0.4) (Powers and Dhamoon, 2021, Aug 20). One would thus expect that oxygen uptake in the normal, healthy lung would not be impaired. The question regarding what would be expected under the circumstances of impaired pulmonary gas exchange might depend upon the exact nature of the impairment.
Quantitative Approach to Oxygen Exchange in a Healthy Lung
In terms of oxygen exchange in a healthy lung, there are two issues to consider: the rate of equilibration of oxygen in the incoming blood with that of the alveoli, and the rate of uptake of oxygen by the incoming blood. These are two distinct, but related, aspects of pulmonary oxygen exchange that should be dealt with. The rate of equilibration of oxygen in the incoming blood with that of the alveoli tells how rapidly the PO2 in the blood flowing through pulmonary capillaries rises to alveolar PO2 (PAO2). (West, 2003) The healthy lung has a “safety margin” of about a factor of 3, in that the incoming systemic venous blood is equilibrated with the PAO2 by the time the blood has traversed one-third of the gas exchange region (West, 2003). Under resting conditions at normal cardiac output, blood only picks up oxygen from about one-third of the gas exchange region; the other two-thirds do not contribute to oxygen uptake under normal conditions. The PO2 with which the blood exits the lung is important because this sets the arterial PO2 of blood that then circulates through the various organs of the systemic circulation. It is normally about 100 mmHg, and normal oxygen exchange in the periphery is designed for this condition (West, 2003).
Severely Impaired Oxygen Exchange: Causes of Hypoxemia
The major causes of hypoxemia are typically classified as hypoventilation, diffusion impairment, shunt, and ventilation-perfusion inequality (Slonim and Hamilton, 1987; West, 2003). Hypoventilation and diffusion impairment are typically treated with elevated inspired oxygen (i.e., oxygen therapy) and use of blood transfusions, even hypoxic, should not be cause for concern. The existence of a shunt (either anatomic or physiologic, as in severe ventilation-perfusion mismatch) is a very serious matter, and transfusion of any blood products must be weighed against the risk of volume overload. Ventilation-perfusion inequality is the most common cause of hypoxemia and is associated with lung disorders such as chronic obstructive pulmonary disease (COPD), interstitial lung disease, and vascular disorders such as pulmonary embolism (West, 2003). It is challenging to model the consequences of the different causes of hypoxemia, except for the case of a shunt, which might occur, for instance, in the case of a gunshot wound to the chest or a traumatic automobile accident which causes chest injury. A shunt refers to the situation in which a portion of the pulmonary blood flow bypasses the gas exchange region (i.e., a direct pathway between pulmonary arterial and pulmonary venous blood), so that this blood does not participate in gas exchange.
The resulting systemic arterial blood is a flow-weighted mixture of blood that has equilibrated with the alveolar gas (weighting factor is 1 – shunt fraction or 1-FS) plus systemic venous blood (weighting factor is shunt fraction, FS) (West, 2003):
[O2]a = (1-FS) [O2]ec + FS [O2]v, [O2]a is arterial oxygen content, [O2]ec is end pulmonary capillary oxygen content, [O2]v is venous oxygen content, and FS is the fraction of pulmonary blood flow or cardiac output that flows through the shunt (i.e., bypasses the gas exchange region).
While breathing 100% oxygen, if a shunt is present, arterial PO2 will not rise much, whereas a normal lung will produce an arterial PO2 of ∼600 mmHg (West, 2003).
Hypoxic Blood Infusion on Venous Oxygen Tension
Prior to infusion of hypoxic blood into a peripheral vein, it is important to consider the potential effect on oxygenation of mixed venous blood flowing from the right ventricle into the pulmonary circulation and the outcome of mixing two volumes of blood, which have different hematocrits, oxygenation states (PO2 and SO2), and P50. Based on typical blood parameters of a human subject/patient, dissolved oxygen does not need to be accounted for, as it comprises only ∼2% of the total oxygen in blood, compared with the much larger amount of oxygen bound to hemoglobin in the red blood cells (RBCs). (Holland, 1997) A minor correction can be applied to results/predictions. Potential limitations are the simple assumptions about distribution of blood in the vascular network. Flowing blood vs. mixing two non-flowing samples involves a flow of hypoxic blood into flowing venous blood in a large vein rather than mixing two static blood samples in an imaginary container, and the calculation can be slightly modified to account for flowing fluids. The rate of mixing of blood, equilibration time, and estimates of the effect of mixing in fluid streams can be made; equilibration times for oxygen exchange in blood are well known and are approximately fractions of a second (Holland, 1997).
When static blood volumes (V1 and V2) are mixed, net diffusion occurs from an area of higher PO2 (RBCs in V1) to a region of lower PO2 (RBCs in V2). Net movement of oxygen will continue until the PO2 in all the RBCs of the mixture is the same, PmixfO2. Thus, P1iO2 will fall to PmixfO2 and P2iO2 will rise to PmixfO2 until PO2 in the mixture is uniform, creating an equilibrium state for oxygen among three phases: plasma, RBCs originally in V1, and RBCs originally in V2 (Pittman, 2016).
The amount of oxygen, AO2, in a volume, V, is equal to V [O2], where [O2] is the oxygen content of the bound oxygen, given by SO2 [Hb] CHb; SO2 is the fractional oxygen saturation of the RBC hemoglobin, [Hb] is the concentration of hemoglobin in the volume, and CHb is the oxygen-binding capacity of hemoglobin (Pittman, 2016).
Expressing [Hb] in terms of hematocrit, H, and RBC hemoglobin concentration, [Hb]RBC, it is as follows:
The total amount of oxygen in the system before mixing is AtotalO2 and is given by:
The total amount of oxygen in the new volume, V1 + V2, after mixing and attainment of equilibrium is:
AtotalO2f = (V1 + V2) [O2]mixf, where f denotes the final state and [O2]mix refers to the final oxygen content of the mixture.
Following blood in volumes V1 and V2 being mixed, oxygen will diffuse from the RBCs in V1 (higher initial PO2) to the RBCs in V2 (lower initial PO2) until the PO2 in all the RBCs of the mixture is the same, PmixfO2. Thus, P1iO2 will fall to PmixfO2 and P2iO2 will rise to PmixfO2. The total amount of oxygen in the system remains constant:
The redistribution of oxygen between the two populations of RBCs in V1 and V2 to the final mixture can be expressed as the differential of both sides of the previous equation (Pittman, 2016):
Once the mixture reaches equilibrium, the only things that change in the RBCs originally associated with V1 and V2 are their oxygen contents, [O2]1 and [O2]2, and they change in the opposite directions (Pittman, 2016):
Expressing [O2] as SO2 H [Hb]RBC CHb and noting that only SO2 will change yields:
Because SO2 is related to PO2 through the oxygen dissociation curve for the equilibrium binding of oxygen to hemoglobin, the change in SO2 is related to the corresponding change in PO2 by dSO2 = (dSO2/dPO2) dPO2, where dSO2/dPO2 is the slope of the oxygen dissociation curve, β’. To a good approximation, β’ ≈ 0.5/P50 (Pittman, 2016).
We can now rewrite the previous equation in terms of practical quantities for the two blood volumes as:
This now allows us to express the respective changes in PO2 from the initial values in the RBCs in V1 and V2 as:
where R = {V2H2P150/V1H1P250}. Since P1O2 falls from P1iO2 to PmixfO2 and P2O2 rises from P2iO2 to PmixfO2, the changes in P1O2 and P2O2 are:
How is the final PO2 after blood mixing (i.e., mixed venous blood flowing through the pulmonary artery to the lungs) related to the two initial PO2 values (i.e., peripheral venous blood and hypoxic blood infusion)? Let V1 be identified as the peripheral venous blood and V2 be identified as the hypoxic blood infusion. Substituting dP1O2 and dP2O2 from above yields (Pittman, 2016):
Solving for PmixfO2 in terms of the other factors yields:
The dimensionless ratio R contains the primary factors that will determine by how much an infusion of “low-PO2” hypoxic stored blood will lower peripheral venous PO2. The value of R is typically very small, and PmixfO2 is a weighted average of the initial PO2s in V1 and V2, which involves R:
The dominant factor in R will usually be V2/V1, the hypoxic blood infusion rate divided by the cardiac output (Pittman, 2016). Typically, the respective hematocrits of the hypoxic stored blood and the subject’s blood will be similar, as well as the respective P50s. The effect of the hypoxic blood infusion on mixed venous PO2 can be estimated more accurately by considering all the factors for hypoxically stored blood and the subject’s blood that appear in the ratio R.
Previous findings on critically ill patients receiving transfusions of conventionally stored blood did not raise any serious concerns about significant adverse effects; thus, it appears unlikely that any such issues would arise for the RBCs processed and stored under hypoxic conditions (Walsh et al., 2004; Smith et al., 2005).
Expected Impact of a Transfusion of Hypoxically Processed and Stored Red Blood Cells
A “massive transfusion” classically refers to the situation in which greater than 10 units of blood are transfused within 24 h. Infusing RBCs at a rate of 1.2 L/min is 25% of the blood volume per minute, which appears to be an extreme rate of infusion. Since normal cardiac output is also about 5 L/min, with an infusion rate of 1.2 L/min (∼25% of the cardiac output), a patient would need to have lost a large volume of blood to justify this high rate of infusion and remain with uncontrolled bleeding at the time of the transfusion (Rodman and Voelkel, 1997; Gladwin et al., 1999). Once blood volume is restored to near normal, the transfusion rate would be lowered or stopped. With the slightly reduced PO2 equilibration for hypoxically stored RBCs and the oxygen exchange “safety factor” of about 3 for the unaffected part of the lung, it is highly unlikely that RBCs exiting the gas exchange region would not be fully equilibrated with the alveolar gas, or that harm would result from infusing RBCs with a low oxygenation state. For the case of sickle cell disease, it is also unlikely that an infusion containing RBCs with a low oxygenation state would be a cause for concern of producing hypoxic pulmonary vasoconstriction (HPV), as the primary stimulus for HPV is low alveolar PO2 rather than low blood PO2 (Rodman and Voelkel, 1997; Gladwin et al., 1999). For the case of traumatic injury involving a shunt, transfusing hypoxic RBCs should not produce any different results from infusing fully oxygenated RBCs. Pathologic circumstances that might involve lung injuries combined with co-existing pulmonary diseases remain to be elucidated, and further analyses may be needed to assess an isovolemic exchange transfusion in lung-impaired patients (Rodman and Voelkel, 1997; Gladwin et al., 1999).
Conclusion
From this review of pulmonary physiology and oxygen transport in the lung, hypoxic blood transfusions appear to offer minimal safety concerns. Due to the intrinsic structural safety margin, oxygen uptake in the normal, healthy lung should not be impaired. Even in patients who receive massive transfusion or critically ill patients administered hypoxic blood who are characterized by a high shunt fraction, RBCs exiting the gas exchange region should be fully equilibrated with the alveolar gas. The outcomes of these assumptions need to be demonstrated clinically in the groups described as well as in more severely lung-impaired patients who are characterized by a combination of pathophysiologic states.
Author Contributions
RP was responsible for the formal analysis, methodology, writing the original draft, and reviewing and editing the manuscript. TY was responsible for the conceptualization, funding acquisition, and reviewing and editing the manuscript. LO was responsible for the conceptualization and reviewing and editing the manuscript. All authors contributed to the final version of the article and approved it for publication.
Funding
The work and open access publication fees were funded by Hemanext, Inc. The work in part was funded by NHLBI: 2R44HL132172.
Conflict of Interest
RP reports consulting fees from Hemanext, Inc. TY reports employment with and stock and stock options in Hemanext, Inc., and grants from the National Institute of Health, NHLBI. LO reports employment with and stock in Hemanext, Inc.
Publisher’s Note
All claims expressed in this article are solely those of the authors and do not necessarily represent those of their affiliated organizations, or those of the publisher, the editors and the reviewers. Any product that may be evaluated in this article, or claim that may be made by its manufacturer, is not guaranteed or endorsed by the publisher.
Acknowledgments
Medical writing assistance was provided by Phillip Giannopoulos of SciStrategy Communications and funded by Hemanext, Inc.
References
Benesch, R., and Benesch, R. E. (1967). The effect of organic phosphates from the human erythrocyte on the allosteric properties of hemoglobin. Biochem. Biophys. Res. Commun. 26, 162–167. doi: 10.1016/0006-291x(67)90228-8
Brown, C. H. IV, Grega, M., Selnes, O. A., McKhann, G. M., Shah, A. S., LaFlam, A., et al. (2014). Length of red cell unit storage and risk for delirium after cardiac surgery. Anesth. Analg. 119, 242–250. doi: 10.1213/ane.0000000000000134
Burns, J. M., Yoshida, T., Dumont, L. J., Yang, X., Piety, N. Z., and Shevkoplyas, S. S. (2016). Deterioration of red blood cell mechanical properties is reduced in anaerobic storage. Blood Transfus 14, 80–88. doi: 10.2450/2015.0241-15
Dumont, L. J., D’Alessandro, A., Szczepiorkowski, Z. M., and Yoshida, T. (2016). CO2 -dependent metabolic modulation in red blood cells stored under anaerobic conditions. Transfusion 56, 392–403. doi: 10.1111/trf.13364
D’Alessandro, A., Yoshida, T., Nestheide, S., Nemkov, T., Stocker, S., Stefanoni, D., et al. (2020). Hypoxic storage of red blood cells improves metabolism and post-transfusion recovery. Transfusion 60, 786–798. doi: 10.1111/trf.15730
Flegel, W. A., Natanson, C., and Klein, H. G. (2014). Does prolonged storage of red blood cells cause harm? Br. J. Haematol. 165, 3–16. doi: 10.1111/bjh.12747
Gladwin, M. T., Schechter, A. N., Shelhamer, J. H., and Ognibene, F. P. (1999). The acute chest syndrome in sickle cell disease. Possible role of nitric oxide in its pathophysiology and treatment. Am. J. Respir. Crit. Care Med. 159, 1368–1376. doi: 10.1164/ajrccm.159.5.9810094
Haradin, A. R., Weed, R. I., and Reed, C. F. (1969). Changes in physical properties of stored erythrocytes relationship to survival in vivo. Transfusion 9, 229–237. doi: 10.1111/j.1537-2995.1969.tb04929.x
Hess, J. R. (2014). Measures of stored red blood cell quality. Vox Sang. 107, 1–9. doi: 10.1111/vox.12130
Holland, R. A. B. (1997). “Kinetics of oxygen and carbon dioxide reactions” in The Lung: Scientific Foundations. 2nd Edn. eds R. G. Crystal, J. B. West, E. R. Weibel, and P. J. Barnes (Philadelphia: Lippincott – Raven Publishers). 1634–1655.
Jones, A. R., Patel, R. P., Marques, M. B., Donnelly, J. P., Griffin, R. L., Pittet, J. F., et al. (2019). Older blood is associated with increased mortality and adverse events in massively transfused trauma patients: secondary analysis of the PROPPR trial. Ann. Emerg. Med. 73, 650–661. doi: 10.1016/j.annemergmed.2018.09.033
Karam, O., Tucci, M., Bateman, S. T., Ducruet, T., Spinella, P. C., Randolph, A. G., et al. (2010). Association between length of storage of red blood cell units and outcome of critically ill children: a prospective observational study. Crit. Care 14:R57. doi: 10.1186/cc8953
Koch, C. G., Li, L., Sessler, D. I., Figueroa, P., Hoeltge, G. A., Mihaljevic, T., et al. (2008). Duration of red-cell storage and complications after cardiac surgery. N. Engl. J. Med. 358, 1229–1239. doi: 10.1056/NEJMoa070403
MacDonald, R. (1977). Red cell 2,3-diphosphoglycerate and oxygen affinity. Anaesthesia 32, 544–553. doi: 10.1111/j.1365-2044.1977.tb10002.x
Pittman, R. N. (2011). Regulation of Tissue Oxygenation. San Rafael: Morgan & Claypool Life Sciences.
Pittman, R. N. (2016). Regulation of Tissue Oxygenation, Second Edition. San Rafael: Morgan & Claypool Life Sciences.
Powers, K. A., and Dhamoon, A. S. (2021). Physiology, Pulmonary Ventilation and Perfusion. Treasure Island: StatPearls Publishing).
Rodman, D. M., and Voelkel, N. F. (1997). “Regulation of vascular tone” in The Lung: Scientific Foundations. 2nd Edn. eds R. G. Crystal, J. B. West, E. R. Weibel, and P. J. Barnes (Philadelphia: Lippincott – Raven Publishers). 1473–1492.
Salaria, O. N., Barodka, V. M., Hogue, C. W., Berkowitz, D. E., Ness, P. M., Wasey, J. O., et al. (2014). Impaired red blood cell deformability after transfusion of stored allogeneic blood but not autologous salvaged blood in cardiac surgery patients. Anesth. Analg. 118, 1179–1187. doi: 10.1213/ane.0000000000000227
Sanders, J., Patel, S., Cooper, J., Berryman, J., Farrar, D., Mythen, M., et al. (2011). Red blood cell storage is associated with length of stay and renal complications after cardiac surgery. Transfusion 51, 2286–2294. doi: 10.1111/j.1537-2995.2011.03170.x
Shah, D. M., Gottlieb, M. E., Rahm, R. L., Stratton, H. H., Barie, P. S., Paloski, W. H., et al. (1982). Failure of red blood cell transfusion to increase oxygen transport or mixed venous PO2 in injured patients. J. Trauma 22, 741–746. doi: 10.1097/00005373-198209000-00004
Smith, M. J., Stiefel, M. F., Magge, S., Frangos, S., Bloom, S., Gracias, V., et al. (2005). Packed red blood cell transfusion increases local cerebral oxygenation. Crit. Care Med. 33, 1104–1108. doi: 10.1097/01.ccm.0000162685.60609.49
Spinella, P. C., Carroll, C. L., Staff, I., Gross, R., Mc Quay, J., Keibel, L., et al. (2009). Duration of red blood cell storage is associated with increased incidence of deep vein thrombosis and in hospital mortality in patients with traumatic injuries. Crit. Care 13, R151. doi: 10.1186/cc8050
Tomaiuolo, G. (2014). Biomechanical properties of red blood cells in health and disease towards microfluidics. Biomicrofluidics 8:051501. doi: 10.1063/1.4895755
Walsh, T. S., McArdle, F., McLellan, S. A., Maciver, C., Maginnis, M., Prescott, R. J., et al. (2004). Does the storage time of transfused red blood cells influence regional or global indexes of tissue oxygenation in anemic critically ill patients? Crit. Care Med. 32, 364–371. doi: 10.1097/01.Ccm.0000108878.23703.E0
West, J. B. (2003). Pulmonary Pathophysiology - The Essentials, Sixth Edition. Philadelphia: Lippincott Williams & Wilkins.
Whitley, P., Wellington, M., Howard, P., Sawyer, S., Hopersberger, L., Dioguardi, M., et al. (2020). Improved Quality of Red Blood Cells Processed and Stored for 42 Days in the Hemanext Oxygen Reduction System after X-Ray Irradiation at Day 14 or Day 21. Transfusion 60, 184A–185A.
Whitley, P., Wellington, M., Sawyer, S., Hanley, S., West, F., Dioguardi, M., et al. (2018). 2,3 Diphosphoglycerate content and oxygen affinity of hemanext red blood cells stored under oxygen reduced conditions. Vox Sang. 113(Suppl. 1):163.
Yoshida, T., Blair, A., D’Alessandro, A., Nemkov, T., Dioguardi, M., Silliman, C. C., et al. (2017). Enhancing uniformity and overall quality of red cell concentrate with anaerobic storage. Blood Transfus 15, 172–181. doi: 10.2450/2017.0325-16
Yoshida, T., McMahon, E., Croxon, H., Dunham, A., Gaccione, P., Abbasi, B., et al. (2021). The oxygen saturation of red blood cell concentrates: the basis for a novel index of red cell oxidative stress. Transfusion 62, 183–193. doi: 10.1111/trf.16715
Yoshida, T., Prudent, M., and D’Alessandro, A. (2019). Red blood cell storage lesion: causes and potential clinical consequences. Blood Transfus 17, 27–52. doi: 10.2450/2019.0217-18
Yoshida, T., and Shevkoplyas, S. S. (2010). Anaerobic storage of red blood cells. Blood Transfus 8, 220–236. doi: 10.2450/2010.0022-10
Keywords: hypoxemia, hypoxic blood infusion, oxidative damage, oxygen exchange, red blood cells (RBCs), RBC storage lesion, transfusion
Citation: Pittman RN, Yoshida T and Omert LA (2022) Effect of Hypoxic Blood Infusion on Pulmonary Physiology. Front. Physiol. 13:842510. doi: 10.3389/fphys.2022.842510
Received: 23 December 2021; Accepted: 28 January 2022;
Published: 04 March 2022.
Edited by:
Paola Bianchi, IRCCS Ca’ Granda Foundation Maggiore Policlinico Hospital, ItalyReviewed by:
Paolo Rebulla, Foundation IRCCS Ca’ Granda Ospedale Maggiore Policlinico, ItalyPedro Cabrales, University of California, San Diego, United States
Copyright © 2022 Pittman, Yoshida and Omert. This is an open-access article distributed under the terms of the Creative Commons Attribution License (CC BY). The use, distribution or reproduction in other forums is permitted, provided the original author(s) and the copyright owner(s) are credited and that the original publication in this journal is cited, in accordance with accepted academic practice. No use, distribution or reproduction is permitted which does not comply with these terms.
*Correspondence: Roland N. Pittman, cm9sYW5kLnBpdHRtYW5AdmN1aGVhbHRoLm9yZw==