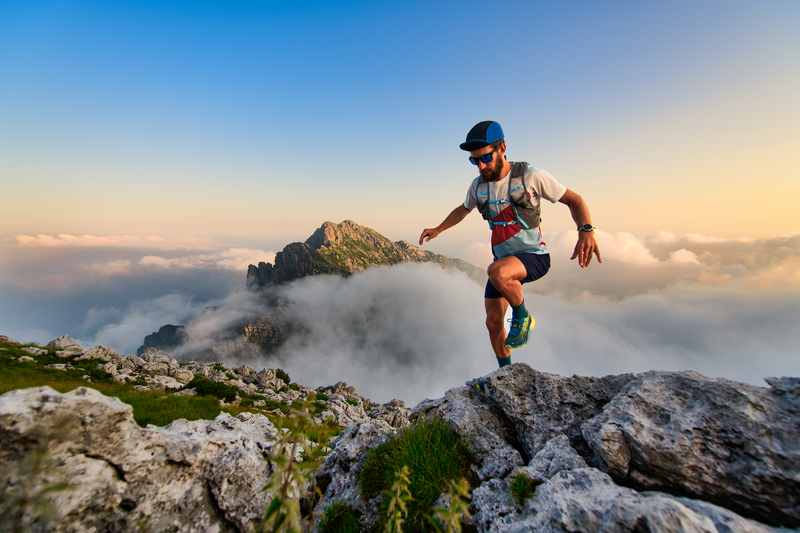
95% of researchers rate our articles as excellent or good
Learn more about the work of our research integrity team to safeguard the quality of each article we publish.
Find out more
ORIGINAL RESEARCH article
Front. Physiol. , 17 March 2022
Sec. Integrative Physiology
Volume 13 - 2022 | https://doi.org/10.3389/fphys.2022.841828
This article is part of the Research Topic Physiological and Pathological Responses to Hypoxia and High Altitude, Volume II View all 22 articles
Obstructive sleep apnea (OSA), a sleep breathing disorder featured by chronic intermittent hypoxia (CIH), is associate with pulmonary hypertension. Rats exposed to CIH develop lung vascular remodeling and pulmonary hypertension, which paralleled the upregulation of stromal interaction molecule (STIM)-activated TRPC-ORAI Ca2+ channels (STOC) in the lung, suggesting that STOC participate in the pulmonary vascular alterations. Accordingly, to evaluate the role played by STOC in pulmonary hypertension we studied whether the STOC blocker 2-aminoethoxydiphenyl borate (2-APB) may prevent the vascular remodeling and the pulmonary hypertension induced by CIH in a rat model of OSA. We assessed the effects of 2-APB on right ventricular systolic pressure (RVSP), pulmonary vascular remodeling, α-actin and proliferation marker Ki-67 levels in pulmonary arterial smooth muscle cells (PASMC), mRNA levels of STOC subunits, and systemic and pulmonary oxidative stress (TBARS) in male Sprague-Dawley (200 g) rats exposed to CIH (5% O2, 12 times/h for 8h) for 28 days. At 14 days of CIH, osmotic pumps containing 2-APB (10 mg/kg/day) or its vehicle were implanted and rats were kept for 2 more weeks in CIH. Exposure to CIH for 28 days raised RVSP > 35 mm Hg, increased the medial layer thickness and the levels of α-actin and Ki-67 in PASMC, and increased the gene expression of TRPC1, TRPC4, TRPC6 and ORAI1 subunits. Treatment with 2-APB prevented the raise in RVSP and the increment of the medial layer thickness, as well as the increased levels of α-actin and Ki-67 in PASMC, and the increased gene expression of STOC subunits. In addition, 2-APB did not reduced the lung and systemic oxidative stress, suggesting that the effects of 2-APB on vascular remodeling and pulmonary hypertension are independent on the reduction of the oxidative stress. Thus, our results supported that STIM-activated TRPC-ORAI Ca2+ channels contributes to the lung vascular remodeling and pulmonary hypertension induced by CIH.
Obstructive sleep apnea (OSA) characterized by cyclic episodes of intermittent hypoxia is a health problem that affect a large worldwide adult population (Somers et al., 2008; Dempsey et al., 2010; Floras, 2018). Obstructive sleep apnea is associated with somnolence, sleep fragmentation and cognitive dysfunction, but it is also considered as an independent risk factor for systemic diurnal hypertension (Somers et al., 2008; Dempsey et al., 2010; Iturriaga et al., 2021). Furthermore, OSA is associated with mild pulmonary hypertension with an incidence ranging from 20-50% (Sajkov and McEvoy, 2009; Floras, 2018). Chronic intermittent hypoxia (CIH) is the main factor for develop systemic hypertension in OSA patients (Somers et al., 2008; Dempsey et al., 2010) and animal exposed to CIH (Del Rio et al., 2010, 2014; Iturriaga et al., 2014, 2021; Iturriaga, 2018; Harki et al., 2021). In addition, CIH produces vascular pulmonary remodeling and increases right ventricular systolic pressure (RVSP) in rodents (Nisbet et al., 2009; Jin et al., 2014, 2016; Iturriaga and Castillo-Galán, 2019; Castillo-Galán et al., 2020). Recently, we found that CIH progressively increased the right ventricular systolic pressure (RVSP) over 35 mm Hg in the rat, enhanced the vasoconstrictor response of small pulmonary arteries to KCl and endothelin-1 (ET-1), increased the medial layer thickness, and increased the right-ventricular wall thickness and the right-ventricular end systolic volume related to the control rats (Castillo-Galán et al., 2020). However, even though the link between OSA and pulmonary hypertension is well recognized, the underlying pathogenic mechanisms are not completely known. The pathological pulmonary vascular alterations induced by CIH has been attributed to Ca2+–dependent enhanced contractility and proliferation of pulmonary arterial smooth muscle cells (PASMC) induced by the activation of hypoxic inducible factors (HIFs) and oxidative stress (Nisbet et al., 2009: Jin et al., 2014, 2016; Iturriaga and Castillo-Galán, 2019). Thus, it is likely that the activation of HIFs and reactive oxygen species (ROS)-dependent signaling pathways may increase intracellular PASMC calcium levels contributing to the development of the CIH-induced enhanced vasoconstriction, cell proliferation and pulmonary hypertension (Waypa et al., 2002; Sylvester et al., 2012).
Among channels and transporters involved in Ca2+ intracellular homeostasis, it has been proposed that store operated calcium entry contributes to enhance the PASMC contractility in sustained hypoxia (Kuhr et al., 2012; Wang et al., 2012, 2017; Reyes et al., 2018). The binding of endothelin-1 (ET-1) or serotonin (5-HT) to their receptors coupled to G-protein evokes the release of the second messenger IP3, which in turn activates intracellular calcium channels (IP3R) depleting the Ca2+ stores of the sarcoplasmic reticulum (Zhang et al., 2003; Wang et al., 2012). STOC are activated by a depletion of Ca2+ stores in the sarcoplasmic reticulum, which allow the entry of calcium from the extracellular space. A growing body of evidence supports the contribution of STOC in the contraction of pulmonary arteries in response to acute and sustained hypoxia (Weigand et al., 2005; Lu et al., 2008, 2009; Ng et al., 2008; Guibert et al., 2011). Furthermore, STOC-mediated calcium entry participates in pathological pulmonary vascular remodeling process, such as hypercontractility, proliferation and differentiation of PASMC (Golovina et al., 2001; Hou et al., 2013; Reyes et al., 2018). From a structural point of view, STOC are composed by homo or heterotetramer pore-forming subunits from the TRPC family (TRPC 1 to 7), or to the ORAI family (ORAI 1 to 3) (Reyes et al., 2018). On the other hand, an auxiliary subunit of the family of stromal interaction molecules [stromal interaction molecule (STIM) 1 and 2] acts as a calcium sensor activating pore-forming subunits in response to calcium depletion from sarcoplasmic reticulum deposits (Fuchs et al., 2010; Johnstone et al., 2010; Wray and Burdyga, 2010; Yang et al., 2010). Indeed, a growing body of evidence suggests that STIM-activated TRPC-ORAI Ca2+ channels (STOC) play a key role in PASMC remodeling and hypercontractility, which led to pulmonary hypertension in animals exposed to sustained hypoxia (Weigand et al., 2005; Ng et al., 2008; Castillo-Galán et al., 2016, 2022)
Recently, we found that CIH produced the upregulation of mRNA and protein levels of TRPC1, TRPC4, TRPC6, ORAI1, and STIM1 subunits in the rat lung tissue, which paralleled the vascular remodeling and pulmonary hypertension (Castillo-Galán et al., 2020), suggesting that STOC participate in the vascular alterations induced by CIH. Accordingly, to assess the contribution of STOC on the CIH-induced pulmonary vascular alterations, we study the effects of the STOC non-selective inhibitor 2-aminoethyldiphenylborinate (2-APB) (Bootman et al., 2002; Smith et al., 2015; Castillo-Galán et al., 2016, 2022; Jain et al., 2021) on the development of pulmonary hypertension and vascular remodeling induced by CIH in a well stablished preclinical model of OSA (Del Rio et al., 2010, 2012, 2014; Iturriaga et al., 2014, Iturriaga and Castillo-Galán, 2019). We also determined the effects of 2-APB on systemic and lung oxidative stress induced by CIH, because 2-APB ameliorates the oxidative stress in a heart ischemia-reperfusion model in mice (Morihara et al., 2017) and rat (Shen et al., 2021).
The experimental protocols were approved by the Scientific Ethical Committee for the Animal and Environment Care from the Pontificia Universidad Católica de Chile, Santiago, Chile (ID:180803006), and were performed according to the National Institutes of Health Guide (NIH, United States) for the care and use of animals. The experiments were performed in 19 male Sprague-Dawley rats (∼ 200 g) from the Center from Innovation in Biomedical Experimental Models (CIBEM) of the Pontificia Universidad Católica de Chile. Rats were fed with a standard diet and access to food and water ad libitum and the room temperature was maintained between 23 and 25°C. Rats were exposed to CIH (5% O2 for 20 s, followed by 290 s of normoxia, 12 time for 8 h/day, from 9:00 AM. to 5:00 PM. for 28 days. The O2 levels in the chambers were regulated with a computerized system, which open and close solenoid valves that control the entry of N2 and its removal by a fan (Del Rio et al., 2010, 2012, Castillo-Galán et al., 2020).
Rats were randomized divided into 3 groups (n = 6–7). At 14 days of CIH, rats were anesthetized with isoflurane 2% in 100% O2, and mini osmotic-pumps (2ML4, Alzet Scientific Products, MD, United States) were implanted subcutaneously in the back. Pumps were loaded with 2-APB (Sigma-Aldrich, United States) to produce a continuous release of 10 mg/kg/day or its vehicle (Dimethyl sulfoxide: ETOH: Saline 1: 5: 4). After surgery, both groups were exposed to CIH for 14 more days, and the results were compared with the control group of rats exposed to normoxia.
At the end of 28 days of CIH, rats were anesthetized with urethane/α-chloralose (40/800 mg/kg, i.p.), tracheostomized and artificially ventilated with a RoVent Jr ventilator (Kent Scientific, Torrington, CT, United States). A thoracotomy was performed to expose the heart, and the right ventricular systolic pressure (RVSP) was recorded with a heparinized catheter inserted into the right ventricle connected to a Statham P23 transducer (Hato Rey, Puerto Rico, United States). The heart rate was derived from the pressure signal recorded with a PowerLab 16 channels acquisition system (ADInstruments, Castle Hill, Australia) (Castillo-Galán et al., 2020).
Frozen left lung samples (∼100 mg) were homogenized in TRIzol (Thermo Fisher Scientific, Waltham, MA, United States). The RNA was resuspended in nuclease-free water (20 μl) to later determination of its concentration and purity by spectrophotometry, being the 260/280 ratio of all samples in the range of 1.9–2.0. The RNA was stored at −80°C until later use. Synthesis to cDNA was performed using the cDNA Synthesis Kit (ABM, Richmond, Canada). The gene expression of TRPC1, TRPC4, TRPC6, ORAI1, ORAI2 and 18S was determined by real-time PCR (qPCR) in a StepOne Plus equipment (Applied Biosystems, Foster City, CA, United States), using a KicqStart kit (Sigma-Aldrich, United States) with the primers sequence used by Castillo-Galán et al. (2020). Relative gene expression was calculated with the 2–ΔΔCt method using 18S gene.
The malondialdehyde (MDA) concentration was measured using a specific enzyme immunoassay thiobarbituric acid reactive substances (TBARS) kit (Cayman Chemical, Ann Arbor, MI, United States) Briefly, 25 mg of fresh lung tissue were homogenized in RIPA Buffer Concentrate (Cayman Chemical, Ann Arbor, MI United States) and centrifuged at 1600 x g for 10 min at 4°C. The supernatant were collected for analysis. Blood samples were collected from the left ventricle using a syringe with heparin. Blood samples were centrifuged at 1000 x g for 10 min at 4°C and the fresh plasma (supernatant) were use for analysis.
Samples of ∼1 cm of the external portion of the medium lobe from the left lung containing distal pulmonary arterioles were collected. The lung tissue was fixed by immersion in Paraformaldehyde 4%, included in paraffin blocks, and cut in 3–5 μm slides. For the morphological analysis, the slides were deparaffinized, dehydrated and stained with Van Gieson. Using a trinocular microscope (Olympus BX41, Japan) with a digital camera (Jenoptik Progres C3), 10–15 images of 150–300 μm internal diameter pulmonary arteries were acquired per lung from each animal. The area of the muscle layer and the artery lumen were measured using the Image Pro6.3 Software (Sigma-imaging, United Kingdom). The percentage of muscle layer was determined using the following formula: (muscle area - lumen area)/muscle area * 100, according to Castillo-Galán et al. (2020).
Immunohistochemical detection of α-actin and Ki-67 (α-actin-r and KI-67-ir) was performed with the smooth muscle α-actin antibody (Clone RM253, Sigma-Aldrich, Misuri, United States, dilution: 1:400) and the Ki-67 antibody (Clone MIB-1, Dako, Glostrup, Denmark, dilution 1:100). For all procedures, the antigen retrieval was performed at 100°C with citrate buffer at pH 6.0. Primary antibody incubations were performed overnight at 4°C in a wet chamber. The labeling was developed using a HRP-diaminobenzidine kit (Envision, Dako, CA, United States). Lung tissue imagen were digitally acquired at 40×. The α-actin-ir in the muscle layer of small pulmonary arteries was measured as pixel count per area. The percentage of KI-67-ir positive nuclei in the medial layer of PMASC was calculated as the percentage of positive KI-67 nuclei (Castillo-Galán et al., 2016).
In histological sections of lung stained with Van Gieson, 6 images were captured with a magnification of 10×. Subsequently, the vascular luminal surface (luminal area/total area) and the vascular density (number of arteries/total area) were determined in arteries of 150–300 μm of internal diameter.
Data were expressed as mean ± standard standard desviation (SD). One-way or two way ANOVA followed by a Newman-Keuls post-test was used for the analysis. Results were analyzed and plotted in GraphPad Prism 8 software (GraphPad Software Inc., San Diego, CA, United States). For all comparisons, differences were considered statistically significant when P < 0.05.
All groups showed a similar gain of weight during the experiments (P < 0.001, two-way ANOVA, between initial and final weight in each group). However, we did not find differences in the initial and final weight between the groups (P = 0.063, two way-ANOVA) (Table 1).
Following 28 days to CIH, the mean RVSP of the vehicle-treated CIH rats (Vehicle + 28d-CIH) was significantly higher related to the control rats (36.5 ± 3.2 mmHg vs. 19.4 ± 5.5 mmHg, P = 0.0001 for Vehicle + 28d-CIH vs. Control rats). Remarkably, 2-APB applied by osmotic pumps from day 14 of CIH (2-APB + 28d-CIH), prevented the increase of RVSP (24.7 ± 5.0 mmHg vs. 36.5 ± 3.2 mmHg, P = 0.004 for 2-APB + 28d-CIH vs. Vehicle + 28d-CIH, respectively, see Figure 1A). The heart rate was similar among all groups (Figure 1B).
Figure 1. Effect of 2-APB on right ventricular systolic pressure and heart rate in rats exposed to chronic intermittent hypoxia. 2-APB was infused from 14 days of CIH exposure with osmotic pumps at a rate of 10 mg/kg/day. (A) Right ventricular systolic pressure (RVSP) (ANOVA P = 0.0002). (B) Heart rate (HR) (ANOVA P = 0.41) in control (empty circles, n = 5), Vehicle + 28d-CIH (black circles, n = 5), and 2-APB + 28d-CIH rats (blue circles, n = 7). Mean ± SD. †††P < 0.001 vs. all groups, ***P < 0.001 vs. Vehicle + 28d-CIH. One way ANOVA-followed by Newman Keuls tests.
Exposure to CIH for 28 days increased the thickness of the medial layer of pulmonary arteries (150–300 μm internal diameter) in the vehicle CIH-treated animals related to the control rats (67.4 ± 7.3% vs. 45.2 ± 2.5%, for Vehicle + 28d-CIH and control rats, respectively, P < 0.0001). In addition, 2-APB treatment prevented the increase of the medial layer thickness to 47.8 ± 3.5% related to its Vehicle (P < 0.0001) (Figure 2A). 2-APB treatment also prevented the increase of α-actin-ir in the medial layer of pulmonary arteries induced by CIH (7.38 ± 1.5 pixels/μm2, 14.33 ± 1.6 pixels/μm2 and 8.83 ± 0.6 pixels/μm2, for Control group, Vehicle + 28d-CIH and 2-APB + 28d-CIH, respectively. P < 0.0001) (Figure 2B). Similarly, the percentage of Ki-67 positive cells in the pulmonary medial layer of vehicle-treated rats exposed to CIH was higher in CIH-treated rats than the control rats (6.3 ± 2.7% vs. 1.5 ± 0.8% for Vehicle + 28d-CIH vs. Control group, respectively. P = 0.0003). 2-APB treatment prevented the increased of PASMC proliferation marker Ki-67 (6.3 ± 2.7% vs. 3.9 ± 1.1% Vehicle + 28d-CIH vs. 2APB-28d-CIH, respectively. P = 0.0051) (Figure 2C).
Figure 2. Effect of 2-APB on pulmonary vascular remodeling induced by chronic intermittent hypoxia. (A) Medial layer (ANOVA P < 0.0001). (B) α-actin immunoreactivity in the medial layer (ANOVA P < 0.0001). (C) Ki-67 positive cells in PASMC (ANOVA P = 0.0009) of control (empty circles, n = 6), Vehicle + 28d-CIH (black circles, n = 6), and 2-APB + 28d-CIH rats (blue circles, n = 7). The % of medial layer was measured as (muscle area - lumen area)/(muscle area * 100). Scale bar = 100 μm. †††P < 0.001 vs. all groups, **P < 0.01 vs. Vehicle + 28d-CIH ***P < 0.001 vs. Vehicle + 28d-CIH, One way ANOVA-followed by Newman Keuls test.
Exposure to CIH for 28 days increased the number of small pulmonary arteries of 150–300 μm of internal diameter compared to the control rats (12.7 ± 2.3 arteries/mm2 vs. 7.2 ± 1.9 arteries/mm2, for Vehicle + 28-CIH vs. control rats, respectively. P = 0.0005). Treatment with 2-APB normalized the number of arteries (7.9 ± 2.5 arteries/mm2 in 2APB + 28-CIH, P = 0,001) (Figure 3A). In addition, CIH decreased the vascular luminal surface in the Vehicle-treated animals exposed to CIH as compared to the control group. Nevertheless, 2-APB prevented this decrease despite that rats were exposed to CIH, reaching similar values compared to control group (1497.0 ± 190.9 μm2/mm2, 797 ± 117.3 μm2/mm2 and 1632 ± 222.8 μm2/mm2, for control, Vehicle + 28d-CIH and 2-APB + 28d-CIH, respectively. P < 0.0001) (Figure 3B).
Figure 3. Effect of 2-APB on vascular density and luminal surface in lungs of rats subjected to chronic intermittent hypoxia. Left panel, Reconstruction of 6 lung parenchyma slices stained with Van Gieson. (A) Vascular density (ANOVA, P = 0.0007). (B) Luminal vascular surface (ANOVA, P < 0.0001) in controls (empty circles, n = 6), Vehicle + 28d-CIH (black circles, n = 6), and 2-APB + 28d-CIH rats (blue circles, n = 7). Scale bar = 100 μm. Mean ± SD. ††P < 0.01 vs. all groups, †††P < 0.001 vs. all groups, **P < 0.01 vs. Vehicle + 28d-CIH, ***P < 0.001 vs. Vehicle + 28d-CIH. One way ANOVA-followed by Newman Keuls tests.
Exposure to 28 days to CIH increase both systemic and pulmonary MDA concentration in Vehicle-treated rats related to control animals, but 2-APB treatment did not prevent the increase in both the systemic and pulmonary MDA concentration (Figure 4).
Figure 4. Effect of 2-APB on pulmonary and systemic MDA concentration (TBARS) following chronic intermittent hypoxia. (A) MDA lung concentration (ANOVA, P = 0.0002). (B) Systemic plasma concentration (ANOVA, P < 0.0001) in control (empty circles, n = 6), exposed to Vehicle + 28d-CIH (black circles, n = 6), and 2-APB + 28d-CIH rats (blue circles, n = 7). Mean ± SD. ‡‡P < 0.01 vs. Control, ‡‡‡P < 0.001 vs. Control. One way ANOVA-Newman followed by Keuls tests.
CIH for 28 days increased the relative pulmonary gene expression of TRPC1, TRPC4, TRPC6, and ORAI 1, but not ORAI 2 as compared to control animals (Figure 5). 2-APB-treated rats did not shown an increase in the relative pulmonary gene expression of TRPC 1, TRPC 4 and TRPC6 induced by CIH, being these values similar to control animals (Figures 5A–C). On the contrary, the treatment with 2-APB induced an increase in the relative pulmonary gene expression of ORAI1, which was greater than the one found in the control animals and Vehicle-28d CIH-treated rats (Figure 5D). The expression of ORAI2 remained unchanged among the groups (Figure 5E).
Figure 5. Effect of 2-APB on the relative gene expression of STOC following chronic intermittent hypoxia. (A) relative lung gene expression of TRPC1 (ANOVA, P = 0.0001). (B) TRPC4 (ANOVA, P = 0.0017). (C) TRPC6 (ANOVA, P = 0.0003). (D) ORAI1 (ANOVA, P = 0.0005), and (E) ORAI2 (ANOVA, P = 0.9612) in control (empty circles, n = 6), Vehicle + 28d-CIH (black circles, n = 6), and 2-APB + 28d-CIH rats (blue circles, n = 6). Values expressed as 2–ΔΔCT related to the control. Mean ± SD. ††P < 0.01 vs. all groups, †††P < 0.001 vs. all groups **P < 0.01 vs. Vehicle + 28d-CIH, ***P < 0.001 vs. Vehicle + 28d-CIH, One way ANOVA-followed by Newman Keuls tests.
We studied the effects of 2-APB, a drug with inhibitory actions on STOC (Bootman et al., 2002, Castillo-Galán et al., 2016, 2022), in a rat model of CIH-induced pulmonary hypertension (Del Rio et al., 2010, 2012, 2014; Iturriaga et al., 2014; Castillo-Galán et al., 2020). Consistently with previous studies, rats exposed to CIH for 28 days, develop vascular remodeling characterized by an increased thickness of the medial layer in pulmonary arteries and an elevated right ventricular systolic pressure (Nisbet et al., 2009; Jin et al., 2014, 2016; Iturriaga and Castillo-Galán, 2019; Castillo-Galán et al., 2020). The main findings of this study are that 2-APB treatment prevented the raise of RVSP, the pulmonary vascular remodeling characterized by an increased medial layer thickness, the increased α-actin-it and Ki-67 immunoreactive levels, and the lumen vascular surface reduction. In addition, we found that 2-APB did not reduce the lung and plasma oxidative stress, suggesting that the effects of 2-APB on vascular remodeling and pulmonary hypertension are independent of the oxidative stress action. Thus, present results strongly suggest that STOC contribute to the pulmonary hypertension and vascular remodeling in a pre-clinical model of OSA.
Present results showing that 2-APB infusion prevented the increase of RVSP induced by CIH, agree and extended previous studies, which found that 2-APB treatment attenuated the pulmonary vascular remodeling, the increased RVSP and the Fulton index in mice exposed for 4 weeks to sustained hypoxia, as compared to hypoxic animals treated with Vehicle (Smith et al., 2015). Similarly, the daily infusion of 2-APB reduced the vascular remodeling and pulmonary hypertension induced by pregestational hypoxia in newborn lambs in high altitude (Castillo-Galán et al., 2016). Interestingly, our results showed that 2-APB prevented the over-expression of TRPC subunits 1, 4 and 6 induced by CIH in the lung tissue. On the other hand, CIH activates the ET-1 – IP3R pathway in PASMC, releasing Ca2+ from the reticulum, which in turn activate STOC (Wang et al., 2012; Castillo-Galán et al., 2020) and raise the intracellular Ca2+ concentration (Reyes et al., 2018). In addition, Ca2+ activates or stabilized transcription factors that upregulated STOC subunits such as HIFs, NFAT, AP-1, NF-KB and the Platelet Derived Growth Factor (Shibasaki et al., 1996; Timmerman et al., 1996; Dolmetsch et al., 1997; Chen et al., 1998; Salnikow et al., 2002; Mottet et al., 2003; Ogawa et al., 2012; Jiang et al., 2014; Domínguez-Rodríguez et al., 2015; Wang et al., 2015, 2017). Taken together, the evidence suggests that 2-APB would induce a decrease in intracellular calcium (Parrau et al., 2013), which may inhibit the upregulation of TRPC1, TRPC4 and TRPC6. However, more studies are required to determine if all of these factors that regulated STOC changed in the lung tissue following CIH.
In this study, 2-APB was administered with osmotic pumps at a delivery rate of 10 mg/kg per day, which is similar to the daily dose range used by Smith et al. (2015), Castillo-Galán et al. (2016, 2022) and Jain et al. (2021) to block STOC in vivo. Indeed, Smith et al. (2015) treated chronic hypoxic mice with 2-APB (1 mg/kg/day i.p.) to reduce pulmonary hypertension and right ventricular enlargement. Castillo-Galán et al. (2016, 2022), administered 2-APB (10 mg/kg/day i.v.) to neonatal lambs with a partial or full gestation in high altitude to ameliorate the pulmonary hypertension and vascular remodeling, and Jain et al. (2021) administrated 2-APB (1 mg/kg per day i.p.) to attenuate pulmonary hypertension in hypoxic mice. We did not measure the plasma concentrations of 2-APB after its administration, but Parrau et al. (2013) estimated that a single dose of 2-APB (8 mg/kg i.v.) would reach a concentration in the extracellular space in the range of 10–100 μM, assuming a distribution volume of 40% of the body weight. The same order of 2-APB concentration blocks STOC in isolated pulmonary arteries from hypoxic lambs (Parrau et al., 2013) and in HEK293 cells transfected with Orai/Stim or TRPC subunits (Lievremont et al., 2005; Peinelt et al., 2008).
Since 2-APB is a non-selective STOC blocker, we cannot preclude effects of 2-APB at the IP3R level. This make it uncertain to determine to what extent the effects of 2-APB on pulmonary vascular remodeling and hypertension are due to its effects on any of these targets. The participation of IP3R on the response of pulmonary arteries to hypoxia is controversial and depend on the species and type of vessel studied. While in rat distal pulmonary artery PASMC, both Ca2+ release from intracellular stores and Ca2+ entry from the extracellular space induced by hypoxia are abolished by IP3R blockade with xestonpongin-C (Wang et al., 2012), in canine pulmonary artery PASMC, Ca2+ entry mediated by STOC in response to hypoxia is unaffected by IP3R blockade (Ng et al., 2008). On the other hand, in rat main pulmonary arteries, both cytosolic Ca2+ increase and contraction in response to hypoxia are biphasic, with a rapid transient fast response followed by a slowly developing and sustained response. In these arteries, the contraction in response to hypoxia develop without changes in IP3 content (Jin et al., 1993), Indeed, it has been proposed that STOC account for the sustained Ca2+ increase in response to hypoxia (Sylvester et al., 2012). Moreover, in isolated pulmonary arteries of newborn lambs, the relaxation elicited by SKF-96365, a specific STOC blocker without effect on IP3R is similar to the relaxation induced by 2-APB, and both of them increased in pulmonary arteries from hypoxic lambs (Parrau et al., 2013). Taken together these observations suggest that 2-APB may act on IP3R and STOC, but the final effect is an indirect or a direct reduction of Ca2+ entry mediated by STOC in pulmonary PASMC from animals exposed to hypoxia. Considering that 2-APB is not a selective STOC blocker, new drugs with a selective effects on ORAI1/STIM1 interaction are needed to evaluate the role of the STOC in OSA patients with pulmonary hypertension (Reyes et al., 2018; Waldron et al., 2019).
Chronic intermittent hypoxia induces oxidative stress at the pulmonary and systemic level, which may affect calcium homeostasis and contribute to the lung vascular remodeling (Del Rio et al., 2010, 2012; Jin et al., 2014, 2016; Iturriaga and Castillo-Galán, 2019; Castillo-Galán et al., 2020). Antioxidants such as procyanidin and melatonin reduced the elevated RVSP and the vascular remodeling induced by CIH (Jin et al., 2014, 2016). The activation of TRPC1 and TRPC6 by the transcription factor BMP4 in rat PASMC depended on the increased ROS levels mediated by NOX4 (Jiang et al., 2014). In addition, it has been proposed that the increase in ROS levels induced by hypoxia, activates the ryanodine receptors (RyR), which in turn induces the release of the reticular Ca2+, activating STIM1, and allowing the interaction with ORAI in the plasma membrane and subsequent activation of the STOC (Suresh and Shimoda, 2016). These results suggest that the effects of antioxidants may be related with the inactivation of STOC and subsequently decrease in intracellular calcium. Although an antioxidant action of 2-APB has been shown in ischemia/reperfusion damage in mice and rat cardiomyocytes (Morihara et al., 2017; Shen et al., 2021), our results showed that 2-APB did not prevent the systemic and lung oxidative stress, suggesting that the effects of 2-APB on PASMC in the lung are not related by a reduction in oxidative stress. Thus, it is likely that the effects of 2-APB were related to its inhibitory action on the increased intracellular Ca2+ concentrations induced by STOC (Bootman et al., 2002). Thus, the treatment of hypoxic pulmonary hypertensive rats with 2-APB, has a strong effect preventing the increase of RVSP and the pulmonary vascular remodeling without any significant action on the oxidative stress markers.
Present results indicated that 2-APB treatment to rats exposed to CIH prevented the increased RVPS and the pulmonary vascular remodeling, without effects on both systemic and pulmonary oxidative stress markers, suggest that STOC are and attractive therapeutic target to prevent pulmonary hypertension in OSA. In summary, present results showed that 2-APB strongly ameliorated the pulmonary vascular alterations induced by CIH, indicates that the STOC inhibition may potentially be used to treat the pulmonary hypertension induced by OSA.
The raw data supporting the conclusions of this article will be made available by the authors, without undue reservation.
The animal study was reviewed and approved by Scientific Ethical Committee for the Animal and Environment Care Pontificia Universidad Católica de Chile, Santiago, Chile (ID:180803006).
SC-G and BR performed the experiments. SC-G and RI contributed to the conception and design of research, analyzed the data, interpreted the results, and drafted the manuscript. All authors revised the manuscript and approved final version of the manuscript.
This work was supported by grant FONDECYT 1211443 from the Agencia Nacional de Investigación y Desarrollo. ANID, Chile and project Puente 010-2022, VRI-PUC.
The authors declare that the research was conducted in the absence of any commercial or financial relationships that could be construed as a potential conflict of interest.
All claims expressed in this article are solely those of the authors and do not necessarily represent those of their affiliated organizations, or those of the publisher, the editors and the reviewers. Any product that may be evaluated in this article, or claim that may be made by its manufacturer, is not guaranteed or endorsed by the publisher.
Bootman, M. D., Collins, T. J., Mackenzie, L., Roderick, H. L., Berridge, M. J., and Peppiatt, C. M. (2002). 2-aminoethoxydiphenyl borate (2-APB) is a reliable blocker of store-operated Ca2+ entry but an inconsistent inhibitor of InsP3-induced Ca2+ release. FASEB J. 10, 1145–1150. doi: 10.1096/fj.02-0037rev
Castillo-Galán, S., Arenas, G. A., Reyes, R. V., Krause, B. J., and Iturriaga, R. (2020). Stim- activated TRPC-ORAI channels in pulmonary hypertension induced by chronic intermittent hypoxia. Pulm. Circ. 10, 13–22. doi: 10.1177/2045894020941484
Castillo-Galán, S., Parrau, D., Hernández, I., Quezada, S., Díaz, M., Ebensperger, G., et al. (2022). The action of 2-aminoethyldiphenyl borinate on the pulmonary arterial hypertension and remodeling of high-altitude hypoxemic lambs. Front. Physiol. 12:765281. doi: 10.3389/fphys.2021.765281
Castillo-Galán, S., Quezada, S., Moraga, F. A., Ebensperger, G., Herrera, E. A., Beñaldo, F., et al. (2016). 2-aminoethyldiphenylborinate modifies the pulmonary circulation in pulmonary hypertensive newborn lambs with partial gestation at high altitude. Am. J. Physiol. Lung. Cell Mol. Physiol. 311, L788–L799. doi: 10.1152/ajplung.00230.2016
Chen, C. Y., Del Gatto-Konczak, F., Wu, Z., and Karin, M. (1998). Stabilization of interleukin-2 mRNA by the c-Jun NH2-terminal kinase pathway. Science 280, 1945–1949.
Del Rio, R., Moya, E. A., and Iturriaga, R. (2010). Carotid body and cardiorespiratory alterations in intermittent hypoxia: the oxidative link. Eur. Respir. J. 36, 143–150. doi: 10.1183/09031936.00158109
Del Rio, R., Moya, E. A., and Iturriaga, R. (2014). Carotid body potentiation during chronic intermittent hypoxia: implication for hypertension. Front. Physiol. 5:434. doi: 10.3389/fphys.2014.00434
Del Rio, R., Moya, E. A., Parga, M. J., Madrid, C., and Iturriaga, R. (2012). Carotid body inflammation and cardiorespiratory alterations in intermittent hypoxia. Eur. Respir. J. 39, 1492–1500. doi: 10.1183/09031936.00141511
Dempsey, J. A., Veasey, S. C., Morgan, B. R., and O’Donnell, C. P. (2010). Pathophysiology of sleep apnea. Physiol. Rev. 90, 47–112. doi: 10.1152/physrev.00043.2008
Dolmetsch, R. E., Lewis, R. S., Goodnow, C. C., and Healy, J. I. (1997). Differential activation of transcription factors induced by Ca2+ response amplitude and duration. Nature 386, 855–858.
Domínguez-Rodríguez, A., Ruiz-Hurtado, G., Sabourin, J., Gómez, A. M., Alvarez, J. L., and Benitah, J. P. (2015). Proarrhythmic effect of sustained EPAC activation on TRPC3/4 in rat ventricular cardiomyocytes. J. Mol. Cell. Cardiol. 87, 74–78.
Floras, J. S. (2018). Sleep apnea and cardiovascular disease. Circ Res. 122, 1741–1764. doi: 10.1161/CIRCRESAHA.118.310783
Fuchs, B., Dietrich, A., Gudermann, T., Kalwa, H., Grimminger, F., and Weissmann, N. (2010). The role of classical transient receptor potential channels in the regulation of hypoxic pulmonary vasoconstriction. Adv Exp Med Biol. 661, 187–200. doi: 10.1007/978-1-60761-500-2_12
Golovina, V. A., Platoshyn, O., Bailey, C. L., Wang, J., Limsuwan, A., Sweeney, M., et al. (2001). Upregulated TRP and enhanced capacitative Ca(2+) entry in human pulmonary artery myocytes during proliferation. Am. J. Physiol. Heart Circ. Physiol. 280, H746–H755. doi: 10.1152/ajpheart.2001.280.2.H746
Guibert, C., Ducret, T., and Savineau, J. P. (2011). Expression and physiological roles of TRP channels in smooth muscle cells. Adv. Exp. Med. Biol. 704, 687–706. doi: 10.1007/978-94-007-0265-3_36
Harki, O., Boete, Q., Pépin, J. L., Arnaud, C., Belaidi, E., Faury, G., et al. (2021). Intermittent hypoxia-related alterations in vascular structure and function: a systematic review and meta-analysis of rodent data. Eur. Respir. J. 19:2100866. doi: 10.1183/13993003.00866-2021
Hou, X., Chen, J., Luo, Y., Liu, F., Xu, G., and Gao, Y. (2013). Silencing of STIM1 attenuates hypoxia-induced PASMCs proliferation via inhibition of the SOC/Ca2+/NFAT pathway. Respir. Res. 14:2. doi: 10.1186/1465-9921-14-2
Iturriaga, R. (2018). Translating carotid body function into clinical medicine. J. Physiol. 596, 3067–3077. doi: 10.1113/JP275335
Iturriaga, R., Alcayaga, J., Chapleau, M. W., and Somers, V. K. (2021). Carotid body chemoreceptors: physiology, pathology, and implications for health and disease. Physiol Rev. 101, 1177–1235. doi: 10.1152/physrev.00039.2019
Iturriaga, R., and Castillo-Galán, S. (2019). Potential contribution of carotid body-induced sympathetic and renin-angiotensin system overflow to pulmonary hypertension in intermittent hypoxia. Curr. Hypertens. Rep. 21:89. doi: 10.1007/s11906-019-0995-y
Iturriaga, R., Andrade, D. C., and Del Rio, R. (2014). Enhanced carotid body chemosensory activity and the cardiovascular alterations induced by intermittent hypoxia. Front. Physiol. 5:468. doi: 10.3389/fphys.2014.00468
Jain, P., Lai, N., Xiong, M., Chen, J., Babicheva, A., Zhao, T., et al. (2021). TRPC6, a therapeutic target for pulmonary hypertension. Am. J. Physiol. Lung Cell Mol. Physiol. 321, L1161–L1182. doi: 10.1152/ajplung.00159.2021
Jiang, Q., Fu, X., Tian, L., Chen, Y., Yang, K., Chen, X., et al. (2014). NOX4 mediates BMP4-induced upregulation of TRPC1 and 6 protein expressions in distal pulmonary arterial smooth muscle cells. PLoS One 9:e107135. doi: 10.1371/journal.pone.0107135
Jin, H., Liu, M., Zhang, X., Pan, J., Han, J., Wang, Y., et al. (2016). Grape seed procyanidin extract attenuates hypoxic pulmonary hypertension by inhibiting oxidative stress and pulmonary arterial smooth muscle cells proliferation. J. Nutr. Biochem. 36, 81–88. doi: 10.1016/j.jnutbio.2016.07.006
Jin, H., Wang, Y., Zhou, L., Liu, L., Zhang, P., Deng, W., et al. (2014). Melatonin attenuates hypoxic pulmonary hypertension by inhibiting the inflammation and the proliferation of pulmonary arterial smooth muscle cells. J. Pineal. Res. 57, 442–450. doi: 10.1111/jpi.12184
Jin, N., Packer, C. S., English, D., and Rhoades, R. A. (1993). Inositol trisphosphate is involved in norepinephrine-but not in hypoxia-induced pulmonary arterial contraction. Am. J. Physiol. 264, L160–L164. doi: 10.1152/ajplung.1993.264.2.L160
Johnstone, L. S., Graham, S. J., and Dziadek, M. A. (2010). STIM proteins: integrators of signalling pathways in development, differentiation and disease. J. Cell. Mol. Med. 14, 1890–1903. doi: 10.1111/j.1582-4934.2010.01097.x
Kuhr, F. K., Smith, K. A., Song, M. Y., Levitan, I., and Yuan, J. X. (2012). New mechanisms of pulmonary arterial hypertension: role of Ca2 + signaling. Am. J. Physiol. Heart Circ. Physiol. 302, H1546–H1562. doi: 10.1152/ajpheart.00944.2011
Lievremont, J. P., Bird, G. S., and Putney, J. W. (2005). Mechanism of inhibition of TRPC cation channels by 2- aminoethoxydiphenylborane. Mol. Pharmacol. 68, 758–762. doi: 10.1124/mol.105.012856
Lu, W., Wang, J., Peng, G., Shimoda, L. A., and Sylvester, J. T. (2009). Knockdown of stromal interaction molecule 1 attenuates store-operated Ca2+ entry and Ca2+ responses to acute hypoxia in pulmonary arterial smooth muscle. Am. J. Physiol. Lung Cell Mol. Physiol. 297, L17–L25. doi: 10.1152/ajplung.00063.2009
Lu, W., Wang, J., Shimoda, L. A., and Sylvester, J. T. (2008). Differences in STIM1 and TRPC expression in proximal and distal pulmonary arterial smooth muscle are associated with differences in Ca2+ responses to hypoxia. Am. J. Physiol. Lung Cell Mol. Physiol. 295, L104–L113. doi: 10.1152/ajplung.00058.200
Morihara, H., Obana, M., Tanaka, S., Kawakatsu, I., Tsuchiyama, D., Mori, S., et al. (2017). 2-aminoethoxydiphenyl borate provides an anti-oxidative effect and mediates cardioprotection during ischemia reperfusion in mice. PLoS One 12:e0189948. doi: 10.1371/journal.pone.0189948
Mottet, D., Michel, G., Renard, P., Ninane, N., Raes, M., and Michiels, C. (2003). Role of ERK and calcium in the hypoxia-induced activation of HIF-1. J. Cell. Physiol. 194, 30–44. doi: 10.1002/jcp.10176
Ng, L. C., Kyle, B. D., Lennox, A. R., Shen, X. M., Hatton, W. J., and Hume, J. R. (2008). Cell culture alters Ca2+ entry pathways activated by store-depletion or hypoxia in canine pulmonary arterial smooth muscle cells. Br. J. Pharmacol. 152, 101–111. doi: 10.1152/ajpcell.00258.2007
Nisbet, R. E., Graves, A. S., Kleinhenz, D. J., Rupnow, H. L., Reed, A. L., Fan, T. H., et al. (2009). The role of NADPH oxidase in chronic intermittent hypoxia-induced pulmonary hypertension in mice. Am. J. Respir. Cell Mol. Biol. 40, 601–609. doi: 10.1165/2008-0145OC
Ogawa, A., Firth, A. L., Smith, K. A., Maliakal, M. V., and Yuan, J. X. (2012). PDGF enhances store-operated Ca2+ entry by upregulating STIM1/Orai1 via activation of Akt/mTOR in human pulmonary arterial smooth muscle cells. Am. J. Physiol. Cell Physiol. 302, C405–C411. doi: 10.1152/ajpcell.00337.2011
Parrau, D., Ebensperger, G., Herrera, E. A., Moraga, F., Riquelme, R. A., Ulloa, C. E., et al. (2013). Store-operated channels in the pulmonary circulation of high- and low-altitude neonatal lambs. Am. J. Physiol. Lung Cell Mol. Physiol. 304, L540–L548. doi: 10.1152/ajplung.00024.2012
Peinelt, C., Lis, A., Beck, A., Fleig, A., and Penner, R. (2008). 2-Aminoethoxydiphenyl borate directly facilitates and indirectly inhibits STIM1-dependent gating of CRAC channels. J. Physiol. 586, 3061–3073. doi: 10.1113/jphysiol.2008.151365
Reyes, R. V., Castillo-Galán, S., Hernandez, I., Herrera, E. A., Ebensperger, G., and Llanos, A. J. (2018). Revisiting the Role of TRP, Orai, and ASIC channels in the pulmonary arterial response to hypoxia. Front. Physiol. 9:486. doi: 10.3389/fphys.2018.00486
Sajkov, D., and McEvoy, R. D. (2009). Obstructive sleep apnea and pulmonary hypertension. Prog. Cardiovasc. Dis. 51, 363–370. doi: 10.1016/j.pcad.2008.06.001
Salnikow, K., Kluz, T., Costa, M., Piquemal, D., Demidenko, Z. N., Xie, K., et al. (2002). The regulation of hypoxic genes by calcium involves c-Jun/AP-1, which cooperates with hypoxia-inducible factor 1 in response to hypoxia. Mol. Cell. Biol. 22, 1734–1741. doi: 10.1128/MCB.22.6.1734-1741
Shen, Y. C., Shen, Y. J., Lee, W. S., Chen, M. Y., Tu, W. C., and Yang, K. T. (2021). Two benzene rings with a boron atom comprise the core structure of 2-APB responsible for the anti-oxidative and protective effect on the ischemia/reperfusion-induced rat heart Injury. Antioxidants 10:1667. doi: 10.3390/antiox10111667
Shibasaki, F., Price, E. R., Milan, D., and McKeon, F. (1996). Role of kinases and the phosphatase calcineurin in the nuclear shuttling of transcription factor NF-AT4. Nature 382, 370–373. doi: 10.1038/382370a0
Smith, K. A., Voiriot, G., Tang, H., Fraidenburg, D. R., Song, S., Yamamura, H., et al. (2015). Notch activation of Ca(2+) signaling in the development of hypoxic pulmonary vasoconstriction and pulmonary hypertension. Am. J. Respir. Cell Mol. Biol. 53, 355–367. doi: 10.1165/rcmb.2014-0235OC
Somers, V. K., White, D. P., Amin, R., Abraham, W. T., Costa, F., Culebras, A., et al. (2008). Sleep apnea and cardiovascular disease: an American heart association/American college of cardiology foundation scientific statement from the american heart association council for high blood pressure research professional education committee, council on clinical cardiology, stroke council, and council on cardiovascular nursing. In collaboration with the national heart, lung, and blood institute national center on sleep disorders research (National Institutes of Health). Circulation 118, 1080–1111. doi: 10.1161/CIRCULATIONAHA.107.189375
Suresh, K., and Shimoda, L. A. (2016). Lung circulation. Compr. Physiol. 6, 897–943. doi: 10.1002/cphy.c140049
Sylvester, J. T., Shimoda, L. A., Aaronson, P. I., and Ward, J. P. (2012). Hypoxic pulmonary vasoconstriction. Physiol. Rev. 92, 367–520. doi: 10.1152/physrev.00041.2010
Timmerman, L. A., Clipstone, N. A., Ho, S. N., Northrop, J. P., and Crabtree, G. R. (1996). Rapid shuttling of NF-AT in discrimination of Ca2+ signals and immunosuppression. Nature 383, 837–840. doi: 10.1038/383837a0
Waldron, R. T., Chen, Y., Pham, H., Go, A., Su, H. Y., Hu, C., et al. (2019). The Orai Ca2+ channel inhibitor CM4620 targets both parenchymal and immune cells to reduce inflammation in experimental acute pancreatitis. J. Physiol. 597, 3085–3105. doi: 10.1113/JP277856
Wang, J., Fu, X., Yang, K., Jiang, Q., Chen, Y., Jia, J., et al. (2015). Hypoxia inducible factor-1-dependent up-regulation of BMP4 mediates hypoxia-induced increase of TRPC expression in PASMCs. Cardiovasc. Res. 107, 108–118. doi: 10.1093/cvr/cvv122
Wang, J., Shimoda, L. A., and Sylvester, J. T. (2012). Ca2+ responses of pulmonary arterial myocytes to acute hypoxia require release from ryanodine and inositol trisphosphate receptors in sarcoplasmic reticulum. Am. J. Physiol. Lung Cell. Mol. Physiol. 303, 161–168. doi: 10.1152/ajplung.00348.2011
Wang, J., Xu, C., Zheng, Q., Yang, K., Lai, N., Wang, T., et al. (2017). Orai1, 2, 3 and STIM1 promote store-operated calcium entry in pulmonary arterial smooth muscle cells. Cell. Death. Discov. 3:17074. doi: 10.1038/cddiscovery.2017.74
Waypa, G. B., Marks, J. D., Mack, M. M., Boriboun, C., Mungai, P. T., and Schumacker, P. T. (2002). Mitochondrial reactive oxygen species trigger calcium increases during hypoxia in pulmonary arterial myocytes. Circ Res. 91, 719–726. doi: 10.1161/01.res.0000036751.04896.f1
Weigand, L., Foxson, J., Wang, J., Shimoda, L. A., and Sylvester, J. T. (2005). Inhibition of hypoxic pulmonary vasoconstriction by antagonists of store-operated Ca2+ and nonselective cation channels. Am. J. Physiol. Lung Cell Mol. Physiol. 289, L5–L13. doi: 10.1152/ajplung.00044.2005
Wray, S., and Burdyga, T. (2010). Sarcoplasmic reticulum function in smooth muscle. Physiol. Rev. 90, 113–178. doi: 10.1152/physrev.00018.2008
Yang, X. R., Lin, M. J., and Sham, J. S. (2010). Physiological functions of transient receptor potential channels in pulmonary arterial smooth muscle cells. Adv. Exp. Med. Biol. 661, 109–122. doi: 10.1007/978-1-60761-500-2_7
Keywords: 2-APB, STIM-activated TRPC-ORAI channels, chronic intermittent hypoxia, pulmonary hypertension, pulmonary vascular remodeling, hypoxia, obstructive sleep apnea
Citation: Castillo-Galán S, Riquelme B and Iturriaga R (2022) Crucial Role of Stromal Interaction Molecule-Activated TRPC-ORAI Channels in Vascular Remodeling and Pulmonary Hypertension Induced by Intermittent Hypoxia. Front. Physiol. 13:841828. doi: 10.3389/fphys.2022.841828
Received: 22 December 2021; Accepted: 09 February 2022;
Published: 17 March 2022.
Edited by:
Eduardo Colombari, Universidade Estadual Paulista, BrazilReviewed by:
Silvia V. Conde, New University of Lisbon, PortugalCopyright © 2022 Castillo-Galán, Riquelme and Iturriaga. This is an open-access article distributed under the terms of the Creative Commons Attribution License (CC BY). The use, distribution or reproduction in other forums is permitted, provided the original author(s) and the copyright owner(s) are credited and that the original publication in this journal is cited, in accordance with accepted academic practice. No use, distribution or reproduction is permitted which does not comply with these terms.
*Correspondence: Rodrigo Iturriaga, cml0dXJyaWFnYUBiaW8ucHVjLmNs
†These authors have contributed equally to this work
Disclaimer: All claims expressed in this article are solely those of the authors and do not necessarily represent those of their affiliated organizations, or those of the publisher, the editors and the reviewers. Any product that may be evaluated in this article or claim that may be made by its manufacturer is not guaranteed or endorsed by the publisher.
Research integrity at Frontiers
Learn more about the work of our research integrity team to safeguard the quality of each article we publish.