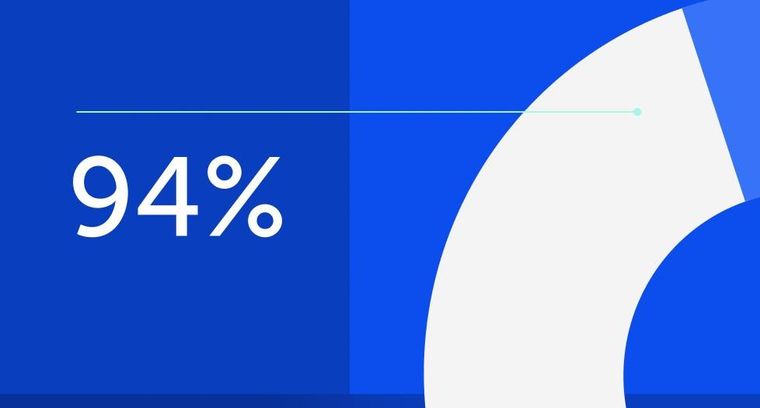
94% of researchers rate our articles as excellent or good
Learn more about the work of our research integrity team to safeguard the quality of each article we publish.
Find out more
REVIEW article
Front. Physiol., 08 March 2022
Sec. Integrative Physiology
Volume 13 - 2022 | https://doi.org/10.3389/fphys.2022.839437
Ligand-gated ion channels are an ionotropic receptor subtype characterized by the binding of an extracellular ligand, followed by the transient passage of ions through a transmembrane pore. Ligand-gated ion channels are commonly subcategorized into three superfamilies: purinoreceptors, glutamate receptors, and Cys-loop receptors. This classification is based on the differing topographical morphology of the receptors, which in turn confers functional differences. Ligand-gated ion channels have a diverse spatial and temporal expression which implicate them in key cellular processes. Given that the transcellular electrochemical gradient is finely tuned in eukaryotic cells, any disruption in this homeostasis can contribute to aberrancies, including altering the activity of pro-tumorigenic molecular pathways, such as the MAPK/ERK, RAS, and mTOR pathways. Ligand-gated ion channels therefore serve as a potential targetable system for cancer therapeutics. In this review, we analyze the role that each of the three ligand-gated ion channel superfamilies has concerning tumor proliferation and as a target for the treatment of cancer symptomatology.
Ionotropic receptors have a variety of triggering mechanisms, including ligand-gated, mechanosensitive, and chemosensitive. The ligand-gated ionotropic channels are highly expressed in a wide variety of tissues and have been successfully targeted to modulate their function since the 1950s (Bianchi and Botzolakis, 2010).
Ligand-gated ionotropic channel subtypes are commonly classified into one of three superfamilies: ATP-gated or purinoreceptors, glutamate-gated receptors, and Cys-loop receptors (Collingridge et al., 2009). These superfamily classifications are derived from the differing transmembrane domains of each superfamily, which in turn confer functional differences (Table 1). The ATP-gated or purinoreceptors, glutamate-gated receptors, and Cys-loop receptors are trimeric, tetrameric, and pentameric assemblies, respectively (Figure 1).
Figure 1. Ligand-gated ion channel superfamily morphology. Shown is a near top-down view displaying preferred ligand and ion selectivity for P2-type ionotropic purinoreceptors (A), glutamate-gated ionotropic receptors (B), and Cys-loop receptors (C). A schematic of the transmembrane domain of each of the three superfamilies is shown with their ligand-interacting domain displayed in orange. Highlighted is the cys-cys loop in the Cys-loop superfamily. Created with BioRender.com.
Ligand-gated ionotropic receptors are critical to maintaining a tightly controlled intracellular electrochemical balance in eukaryotic cells. As such, their disruption can lead to inappropriate activation of cellular pathways, including induction of a pro-tumorigenic phenotype. Here, we have taken representative examples from the three ligand-gated ionotropic channel superfamilies to illustrate how they may promote tumor growth and associated tumor symptomatology. Then, we detail the therapeutic potential to focally target the ligand-gated ionotropic channels and thus associated pathways that they contribute to regulating. In discussion of Cys-loop receptors, we highlight how modulating this receptor subtype to perturb an intracellular electrochemical balance in cancer cells can be a successful cancer-specific treatment approach in combination with other therapeutics and/or treatment modalities.
While adenosine 5’-trisphosphate (ATP) provides intracellular energy to fuel key reactions i.e., phosphorylation, active transport, and motility (Bonora et al., 2012), it also functions as a key signaling molecule (Burnstock, 1972). This signaling is mediated through the action of both metabotropic and ionotropic purinoreceptors. The P1 receptor subclass of purinoreceptors is sensitive to adenosine and displays G-protein coupled receptor (GPCR) activity, while the P2 receptor subclass of purinoreceptors is responsive to adenine and uridine (Campos-Contreras et al., 2020). The P2 subclass is further subclassified into the metabotropic P2Y purinergic receptors (P2YRs), which includes eight mammalian subtypes, and the ionotropic P2X purinergic receptors (P2XRs) with seven mammalian subtypes (Lustig et al., 1993; Brake et al., 1994; North, 2002).
P2X purinergic receptors are widely expressed, including in the brain, peripheral vasculature, lungs, heart, kidney, and immune system with many tissues expressing multiple P2XR subtypes (Huang et al., 2021). P2XRs have been implicated in a variety of intracellular processes ranging from smooth muscle contraction, neurotransmission, macrophage activation, cell proliferation, and death (Burnstock et al., 2010). P2XRs form homo-trimeric (P2X1, P2X2, P2X3, P2X4, P2X5, P2X6, and P2X7) or hetero-trimeric (P2X2/3 and P2X1/5) transmembrane channels, and bind up to three ATP molecules at the interface between adjacent subunits with an EC50 on the order of 60 μM (Zhong et al., 1998; Stelmashenko et al., 2012). There is evidence that fewer than three ATP molecules may be sufficient to mediate the opening of the purinergic transmembrane pore (Bean, 1990; Stelmashenko et al., 2012). Upon binding of the ATP ligand, the channel preferentially allows passage of sodium, potassium, and calcium (North, 2016). The P2XR mediated signaling is abrogated by ectonucleotidases, which catalyze ATP breakdown (Novak, 2003).
Purinoreceptors are important to tumor pathogenesis given that ATP is so ubiquitous in the tumor microenvironment (TME) and critical to driving cell proliferation. The concentration of ATP in the TME is approximately 100–500 μM, while 10–100 μM in normal tissues and blood (Hu et al., 2019; De Marchi et al., 2020; Mimoto et al., 2020). The mechanism underlying ATP release in the TME is multifarious as it may be actively secreted, passively released upon cell death, or generated de novo extracellularly (Di Virgilio et al., 2018a). Regardless, the dysregulation of extracellular ATP acts in a paracrine or autocrine fashion via purinoreceptors to alter the functionality of numerous nearby cell subtypes.
In combination with the changes in extracellular ATP concentration, a variety of P2XRs are upregulated in disparate cancer types, including glioblastoma (GBM), hepatocarcinoma, hepatobiliary carcinoma, breast cancer, multiple myeloma, prostate cancer, and renal cancer (Farrell et al., 2010; Di Virgilio et al., 2018a; Khalid et al., 2018; Asif et al., 2019; Huo and Chen, 2019). The specific tumor type appears to define whether upregulation of P2X may be protective or prohibitive against a tumorigenic phenotype. The mechanism of action of P2XRs in cancer proliferation and growth is multifold. Interestingly, P2XR activation lies on a spectrum as physiological ATP concentrations can promote proliferation and metastasis, whereas excessive ligand binding can induce apoptosis (Virgilio, 2012).
Another effect of P2XRs on cancer pathophysiology is tumor migration and invasiveness. One of the hallmarks of late-stage and metastatic tumors is their ability to spread. This confers a significant disease burden to the tumors as infiltration into non-native tissue can be a common cause of mortality. Therefore, if P2XRs are involved in this process, it is a promising therapeutic avenue to “antagonize” the receptor to diminish tumor motility. In particular, P2XRs have been implicated in tumor epithelial-to-mesenchymal transition (EMT). The EMT confers a morphology to tumor cells that allow for extracellular proliferation and distant metastasis (Thiery, 2002).
Purinergic receptor activation can also secondarily modify tumor proliferation through its activity in the immune system. Firstly, P2XR subtypes are widely expressed in a variety of immune cell subtypes, ranging from macrophages to lymphocytes (He et al., 2017). In human T-cells, ATP release was shown to increase IL-2 expression and p38 MAPK activation (Loomis et al., 2003). IL-2 is a key pro-proliferative cytokine that induces the proliferation of B- and T-lymphocytes. Therefore, increased expression of IL-2 would support an immunogenic response. This conclusion is supported by evidence that “antagonism” of P2X signaling led to T-cell anergy, or functional inactivation (Schenk et al., 2008). The following subsections details the various research on P2XR subclasses and their relationship to cancer pathogenesis.
One of the key processes necessary for tumor cell survival is a high rate of metabolic activity to outcompete surrounding phenotypically “normal” cells. This is especially highlighted by the Warburg effect in relation to glutamate metabolism (discussed below). Previous data has shown that extracellular ATP acting at the P2X1R, along with P2X7R and P2YRs, is a trigger for intracellular energy metabolism, enhancing oxidative phosphorylation and subsequent ATP production (Adinolfi et al., 2005; Ledderose et al., 2016; Vultaggio-Poma et al., 2020). The mechanism by which P2XRs are linked to ATP production is an increase in intracellular calcium following purinergic receptor activation which is known to increase mitochondrial metabolic machinery (Jouaville et al., 1999).
P2X2R has not been well researched in regard to cancer pathogenesis but will be discussed further in regard to cancer symptomatology (Kaan et al., 2010).
While P2X7R has been the most extensively researched P2XR subtype in cancer, there have been recent advances implicating P2X3R and P2X4R as well. High P2X3R expression is associated with poor recurrence-free survival in human hepatocellular carcinoma (Maynard et al., 2015). The proposed mechanism is that ATP-mediated activation of P2X3R leads to JNK signaling activation which is sufficient to promote cell cycle progression (Maynard et al., 2015).
Regarding P2X4R, the mechanism of action in GBM is activation of P2X4R leading to increased brain-derived neurotrophic factor (BDNF)/Trk receptor tyrosine kinase (TrkB) signaling which work in concert to upregulate activating transcription factor 4 (ATF4), a key promoter of cancer cell survival in hypoxic environments (Trang et al., 2009; Singleton and Harris, 2012; Huo and Chen, 2019). Huo and Chen (2019) showed that knockdown of P2X4R leads to diminished proliferation and growth in the rat C6 glioma model via downregulation of the BDNF/TrkB/ATF4 pathway.
Epidermal growth factor (EGF)-induced EMT in human breast cancer cells revealed an upregulation of P2X5R mRNA, resulting in an increase in ATP-mediated Ca2+ signaling (Azimi et al., 2016). However, P2X5R was simultaneously downregulated in these same cell lines when exposed to hypoxic environments (Azimi et al., 2016). This further highlights the dynamic and heterogenous nature of cancer cells in which a single receptor subtype can have different mechanisms in different microenvironments.
In human renal cell carcinoma (RCC) cell lines, P2X6R was found to be significantly upregulated compared to HK2 negative control cells (Gong et al., 2019). Upon ATP activation, P2X6R-mediated Ca2+ entry induced activation of p-ERK1/2-MMP9 axis to promote RCC metastasis and invasion (Gong et al., 2019). The activation of calcium-mediated ERK1/2 pathway is a unifying factor with the P2X5R and P2X7R putative mechanisms in cancer proliferation. This necessitates further research on a combinatorial therapeutic approach blocking multiple channels’ activity by targeting the ultimate downstream targets. Moreover, further research needs to be performed on the P2X6R subclass on whether its expression is altered in hypoxic environments like the P2X5R.
Within the P2XR subclass, the P2X7R subtype is perhaps the most well studied in regard to cancer pathogenesis (Di Virgilio et al., 2021; Hreich et al., 2021; Matyśniak et al., 2021). Regarding proliferation, it has been shown that the P2X7R subtype initiates an intracellular phosphorylation cascade resulting in the activation of the PI3K/Akt and ERK1/2 pathways, key regulators of cancer survival (Amoroso et al., 2015; Salahuddin et al., 2021). To prove this point, Qiu et al. (2014) demonstrated in human prostatic carcinoma cell lines that knockdown of P2X7R led to diminished PI3K/Akt and ERK1/2 pathway activation in response to exogenous ATP; this, in turn, decreased tumor proliferation and growth. The PI3K/Akt pathway has a reciprocal effect on P2X7Rs by increasing the transcription of the P2rx7 gene through the action of specificity protein 1 (Sp1) (Gómez-Villafuertes et al., 2015). This potentially serves as a positive feedback loop in cancer proliferation in which there is a continuous loop of increased extracellular ATP concentration leading to increase purinergic receptor activation followed by PI3K/Akt pathway activation. The activation of PI3K/Akt also suggests that P2X7R may help maintain stem cell-like populations in tumors (Rabelo et al., 2021). It has also been shown in human embryonic kidney cells that P2X7R-expressing tumors were characterized by an increased expression of the transcription factor NFATc1 which conferred increased proliferative capability (Adinolfi et al., 2012). The theme that persists even in purinergic signaling is that different receptor subtypes can have differing effects on different tumors.
P2X7R knockdown has been shown to reduce the expression of Snail, E-cadherin, Claudin-1, interleukin (IL)-8, and MMP-3 which are all EMT-related genes (Qiu et al., 2014). Another study showed that direct activation of P2X7R led to an upregulation of all forms of mature cysteine cathepsins which are proteases dedicated to the breakdown of the extracellular matrix (Jelassi et al., 2011). This in turn conferred increased invasiveness in human-derived breast cancer cells. Interestingly, the pro-tumorigenic activity of P2X7R in tamoxifen-resistant breast cancer cells was found to be linked with migration and metastasis independent of matrix metalloproteinase (MMP) signaling and EMT factors. The P2X7R-mediated migration in tamoxifen-resistant breast and melanoma cancer cells was found to be linked with miRNA-containing small extracellular vesicles (sEV) (Park et al., 2019; Pegoraro et al., 2021). Again, it is highly likely that the differential activation of P2XR subtypes leads to the activation of different intracellular pathways which all converge to increase tumor motility and proliferation.
Another interesting role of purinoreceptors in immunomodulation is the activation of metalloproteases, ADAM10 and ADAM17, by P2X7Rs which leads to the shedding of CD62L, CD27, and IL-6R (Gu et al., 1998; Di Virgilio et al., 2018b). CD62L, also known as L-selectin, is critical for circulating lymphocytes to recognize endothelial cells in a target tissue, allowing for lymphocytes to enter secondary lymphoid organs (Yang et al., 2011). CD27 is a member of the TNF receptor superfamily and upon binding of its ligand, CD70, is thought to play a key role in B-cell activation and the differentiation and clonal expansion of T-cells (Hendriks et al., 2000; Grassi and De Ponte Conti, 2021). The combination of these cleavages implicates purinergic receptor activation in an increased immune response to cancer cells (Cai et al., 2021). The combination of pro-proliferative and migratory effects of purinergic signaling and the pro-immunomodulatory response makes purinergic signaling a particularly interesting drug target (Figure 2).
Figure 2. P2-type ionotropic purinoreceptors in cancer growth, metastasis, and immunomodulation. (A) Shown are the anti-tumor, pro-immunomodulatory effects of P2XR activity on immune cells. The effect on T-cells of P2X7R activity leads to cleavage of CD27 and CD62L, which help in T-cell clonal expansion and honing to target tissue, respectively. (B) Subcellular pathways upregulated by purinergic signaling may lead to increased cell division and tumor growth, as illustrated. (C) P2X signaling upregulates molecules that favor metastasis, a key prognostic factor into the severity of tumor disease burden. Created with BioRender.com.
As with our discussion of other ligand-gated ionotropic receptors (below), we turn to recent developments in P2XR “antagonism” for tumor therapy. While purinergic signaling is a more recent target for chemotherapeutics, the targeting of purine/purinergic incorporation into rapidly dividing cancer cells has been a highly utilized cancer therapeutic mechanism. For example, the 5-Fluorouracil (5-FU) prodrug, capecitabine, both incorporate incorrect fluoronucleotides into RNA and DNA while simultaneously inhibiting the action of thymidylate synthase (Longley et al., 2003). By this mechanism of action, rapidly dividing cancer cells are preferentially killed. Returning to purinergic signaling, much of the literature surrounding P2XR “antagonism” concerns abrogation of the P2X7R subtype. In a rat model of C6 glioma, pharmacological inhibition of P2X7R by brilliant blue G (BBG) inhibited tumor growth (Ryu et al., 2011). Mohammed et al. showed that the use of the P2X7R antagonists, A438079 and AZ10606120, reduced caspase-3, caspase-1, p21, and Cdc25c in a mouse model of pancreatic cancer which resulted in decreased cancer proliferation (Mohammed et al., 2017; Zhang et al., 2021). Similarly, B16 melanoma cells and B16 melanoma-bearing mice when treated with oxidized ATP (purinergic receptor inhibitor) displayed decreased tumor cell proliferation (Hattori et al., 2012). However, one must also weigh the anti-immune effects of P2X antagonism when considering these anti-tumorigenic effects.
While having anti-tumorigenic potential, purinergic therapeutics also may be used in symptom management. Different types of pain are associated with P2XR activation. For example, the P2X3R subtype expressed on sensory neurons has been associated with the transmission of neuropathic pain (Bele and Fabbretti, 2015). Similarly, inhibition of the P2X4R subtype in spinal cord microglia through intrathecal injection of bone marrow stromal cells attenuated neuropathic pain in a murine model (Ulmann et al., 2008; Teng et al., 2019). The mechanism of this nociception is thought to be activation of P2X4R on microglia by afferent neurons leading to MAPK signaling, which in turn leads to the synthesis and excretion of brain-derived neurotrophic factor (BDNF) (Ulmann et al., 2008; Trang et al., 2009).
A specific subtype of cancer-related pain is cancer-induced bone pain (CIBP) which represents a significant burden on the quality of life. CIBP is the product of metastasis of non-bone tumors to the bone resulting in debilitating pain. Interestingly, antagonism of the P2X2/3R by minodronic acid, a third-generation bisphosphonate, resulted in an analgesic effect in a murine model of CIBP as measured by nociceptive behaviors (Kakimoto et al., 2008).
Like other ligand-gated ionotropic channel superfamilies, the glutamate family of receptors has metabotropic, metabotropic glutamate receptor (mGluR), and ionotropic, ionotropic glutamate receptor (iGluR), subfamilies. The three major receptors in the iGluR subfamily are the N-methyl-D-aspartate (NMDA), alpha-amino-3-hydroxyl-5-methyl-4-isoxazole-propionate (AMPA), and kainite receptors, so named for their endogenous agonists (Table 1; Purves et al., 2001). Ionotropic glutamatergic signaling has been well studied for its role in the CNS as the source of rapid depolarizing signaling originating from excitatory neurons. Upon binding of ligand, all three channels allow for the selective conductance of K+ and Na+ ions—with small amounts of Ca2+ in the case of the NMDA channel. Interestingly, the NMDA channel’s pore is blocked by a Mg2+ ion at hyperpolarized states but is removed when depolarized. Moreover, NMDA requires the co-activator glycine for its full conductance to be achieved. With the influx of Ca2+, NMDA channels can activate other intracellular signaling cascades, implicating NMDA channels in a variety of longer-term synaptic processes such as learning, memory, and neuroplasticity (Blanke and VanDongen, 2009). The slower activation of NMDA receptors has led to the conclusion that they are not involved in the generation of action potentials (Yi et al., 2020). On the other hand, due to the lack of a co-activator or pore blocker, the AMPA and kainate receptors have been shown to produce very fast excitatory post-synaptic currents (EPSCs). The ligand-binding domains are highly conserved across iGluR subfamilies and are classically referred to as S1 and S2 (Stern-Bach et al., 1994).
The structure of iGluRs consists of a tetrameric transmembrane domain surrounding a central pore (Figure 1). The NMDARs are constructed from several possible subunits including GluN1, GluN2A, GluN2B, GluN2C, GluN2D, GluN3A, and GluN3B (Kew and Kemp, 2005; Mayer, 2005; Bettler et al., 2019). The NMDAR consists of two requisite GluN1 subunits with any remaining subtype comprising the final two subunits of the tetramer (Paoletti et al., 2013). The GluN1 subunit determines calcium conductance, whereas the GluN2 and GluN3 subunits determine the pharmacological and electrophysiological properties of the channel. The AMPAR arises from a combination of GluA1, GluA2, GluA3, and GluA4 subunits. Kainate receptors are assembled from GluK1, GluK2, GluK3, GluK4, and GluK5 subunits that can form either a homotetramer of GluK1-3 subunits or a heterotetramer of GluK1-5 subunits (Stepulak et al., 2014; Bettler et al., 2019). Again, alternative splicing allows for another layer of heterogeneity upon this pre-existing channel subunit complexity.
Glutamate receptors are physiologically expressed in a more limited range of tissues, compared to the other superfamilies discussed in this review. Glutamate receptor tissue expression is observed primarily in the brain, spinal cord, retina, and peripheral nervous system (Traynelis et al., 2010). On the other hand, iGluRs are expressed in disparate tumor subtypes, being upregulated in CNS tumors, colorectal, hepatocellular, gastric, thyroid, larynx, melanoma, osteosarcoma, and blood neoplasms (Ganor et al., 2009; Stepulak et al., 2009; Li et al., 2012; Stepulak et al., 2014; Gong et al., 2017). Given the numerous linkages with various iGluRs in cancer, this represents a potentially highly targetable system to inhibit cancer growth (Rzeski et al., 2001). The endogenous ligand, glutamate, is synthesized by the deamination of glutamine (Schousboe et al., 2014; Yi et al., 2020). Glutamate is a key intermediary in a variety of anabolic reactions with the possibility of creating gamma-aminobutyric acid (GABA), suggesting an interplay between the two signaling pathways in cancer (see below). The additional deamination of glutamate can be used in the synthesis of purines and pyrimidines and the carbon skeleton can be incorporated into ATP. Similarly, the carbon skeleton of glutamate can be used to make other amino acids such as ornithine, arginine, and proline. Of particular relevance to this review is that glutamate is also a precursor to glutathione which is a reaction oxygen species (ROS) scavenger. As will be discussed further, this allows for cancer cells to survive oxidatively stressful environments. The dual-activity as both a key anabolic intermediate and a signaling molecule gives glutamate a critical role in tumor pathogenesis.
Particularly unique to the discussion of the glutamatergic superfamily is the fact that the ligand, glutamate, is not only implicated as an extracellular messenger but also as a key intracellular modulator of tumor proliferation. Otto Warburg noticed that cancer cells take up glucose at almost a tenfold rate compared to normal cells and shunt glucose through the anaerobic pathway to produce lactate (e.g., the Warburg effect) (Koppenol et al., 2011; Liberti and Locasale, 2016). As glucose is frequently shunted to glutamate, it is natural that increased glutamate levels in various cancers correlate to poorer prognoses. For example, in prostate cancer, glutamate concentrations directly correlated with advanced Gleason scores, a clinical prostate staging system (Koochekpour et al., 2012). Researchers are also analyzing whether glutamate levels can be used as a diagnostic approach in breast cancers due to a similar logic as in pancreatic cancers (Budczies et al., 2015). The tumor microenvironment generates high concentrations of ROS. Basal levels of ROS lead to cancer proliferation, whereas high levels of ROS induce a cytotoxic response (LeBoeuf et al., 2020). Cancer cells co-opt the elevated levels of glutamate to produce glutathione which can act as a “sponge” of ROS to maintain the optimal range of ROS concentration, allowing for cancer growth (Hayes et al., 2020).
Returning to the mechanism of glutamate binding to its receptor, the variety of glutamatergic signaling in cancer impacts multiple intracellular pathways. One well-studied association is the activation of NMDA receptors leading to activation of the mTOR pathway, which is classically associated with cell proliferation; the mechanism by which glutamate binding to NMDA activates mTOR is still debated with the two prevailing hypotheses being a Ca2+ mediated activation of p-ERK or via inhibition of membrane cationic amino acid transporters (Huang et al., 2007; Tang et al., 2015). Interestingly, the NMDA-mediated activation of the p-ERK pathway gives a potential connection to the P2XRs which operate on the same pathway. NMDA receptors are important regulators of pancreatic cancers and pharmacological blockade of NMDA receptors, especially the GluN2B sub-unit, was found to have significant growth inhibitory effects on human pancreatic tumor cells in the orthotopic xenograft mice model (North et al., 2017). Furthermore, NMDA receptors have been linked to activation of ERK signaling and cAMP-response element-binding protein (CREB) in human non-small cell lung cancer (NSCLC) cells. The non-competitive NMDA receptor antagonist MK-801 (dizocilpine) was reported to reduce phosphorylated ERK1/2 levels in a concentration-dependent manner (Stepulak et al., 2005). Further, treatment of NSCLC cells with the NMDA receptor antagonist MK-801 was shown to decrease phosphorylation of CREB, which is involved in regulating transcription of various cell cycle genes including p21 (Stepulak et al., 2005). Functional NMDA receptors were also found to be expressed in patient-derived human small cell lung cancer (SCLC) cell lines NCI-H345, DMS-53, NCI-H146, and NCI-H82 as well as in SCLC tissue sections (North et al., 2010). In vivo, MK-801 was shown to inhibit the growth of tumor xenografts of H345 SCLC cells (North et al., 2010). Finally, research on the repositioning of the Alzheimer’s drug memantine (an NMDAR antagonist) showed inhibition of cell cycle progression of prostate cancer cells (Albayrak et al., 2018). The mechanism of action was through upregulation of the Bax-dependent apoptotic pathways.
Regarding AMPAR, Ishiuchi et al. (2007) showed that glutamate released by glioblastoma cells acts in a paracrine or autocrine fashion to activate AMPAR which in turn led to the influx of Ca2+ allowing for the phosphorylation of Akt at Ser-473. The activation of Akt supports glioblastoma proliferation and growth and has been well studied in relation to the tumor suppressor PTEN (Choe et al., 2003). Not only is glutamate released by glioma cells, but there exists neuron-to-glioma synaptic transmission in which neuron-mediated glutamate release and subsequent binding to AMPAR on glioma cells led to increased proliferation in vitro (Venkatesh et al., 2019). In a mouse model of pediatric glioma, AMPAR blockade through perampanel, a non-competitive antagonist of AMPAR, led to a 50% decrease in glioma growth in perampanel-treated mice compared to vehicle control (Venkatesh et al., 2019). In pancreatic cancer, activation of AMPAR led to increased MAPK signaling and K-Ras signaling which promoted invasion and migration (Herner et al., 2011). RNAi suppression of glutamatergic signaling suppressed the observed AMPAR effects on pancreatic cancer cell migration. Similarly, antagonism of AMPA signaling in human lung adenocarcinoma cells led to a restoration of the tumor suppressor p21 and p53 with a concomitant halt in lung cancer growth (Stepulak et al., 2007).
Lastly, the kainate receptor also has been linked to the breast cancer epithelial-to-mesenchymal transition (EMT). Firstly, Xiao et al. (2019) found these kainate receptors to be significantly upregulated in human-derived breast cancer cells. The mechanism by which kainate receptors were shown to increase the EMT transition was through the upregulation of SPDEF/CDH1 signaling (Xiao et al., 2019). As mentioned previously, the EMT transition is critical to tumor proliferation and migration.
Seizures are one of the most common presenting features of CNS tumors. It is thought that the release of glutamate from the tumor microenvironment (TME) via a cystine-glutamate exchanger (SLC7A11, xCT) contributes to this clinical presentation (Sørensen et al., 2018). It has been shown that high levels of glutamate induce seizures through its excitatory effect (Buckingham et al., 2011). Perampanel, a non-competitive AMPAR antagonist, has already been established to treat patients with seizures (Lange et al., 2021). Therefore, it can be easily translated to cancer-induced seizures with the potential added benefit of inhibiting tumor progression through the aforementioned mechanisms. Aside from directly antagonizing the iGluRs, SLC7A11/xCT can be antagonized through sulfasalazine (cystine-glutamate exchanger antagonist) which reduces extracellular glutamate release, thereby decreasing seizure incidence (Figure 3; Verbruggen et al., 2021). An additional benefit of this therapeutic modality is that there is potential for a synergistic anti-tumor effect, treating the cause and symptoms simultaneously. Highlighting this point, SLC7A11 antagonism caused synthetic lethality in KRAS-mutant lung adenocarcinoma (Hu et al., 2020).
Figure 3. Glutamatergic signaling in central nervous system (CNS) tumor-induced seizures. Many CNS tumors present with seizures due to mass effect or increased extracellular glutamate release mediated by the SLC7A11 channel, a cystine-glutamate exchanger. The excess glutamate in the extracellular fluid binds to AMPA receptors autologously, leading to Ca2+ influx which may activate pro-tumorigenic pathways. Alternatively, the excess glutamate may act in a paracrine fashion, leading to nearby neuronal hyperexcitability, which manifests clinically as a seizure. Antagonists of both the SLC7A11 channel and the AMPAR can modulate this pathological state. Created with BioRender.com.
Glutamatergic signaling has also been shown to play a critical role in the peripheral sensitization and mechanical hypersensitivity associated with CIBP. Zhu et al. (2020) showed that glutamate injection-induced neuronal excitation in dorsal root ganglia (DRG) is similar to that shown to be induced by CIBP. In a breast cancer model of CIBP, sulfasalazine reduced nociceptive perception and delayed onset of behavioral pain signs (Ungard et al., 2014; Ellingson and Vanderah, 2020; Zhu et al., 2020). Furthermore, in a rat model of CIBP, direct inhibition of NMDAR in the spinal cord led to nociceptive relief (Dai et al., 2017). The proposed mechanism of action was through reduced phosphorylation of PKCγ and ERK1/2 in the spinal cord (Dai et al., 2017).
Cys-loop receptors are so named for the presence of a disulfide bridge in a loop of its extracellular domain (Figure 1; Miller and Smart, 2010). Canonical Cys-loop receptors include nicotinic acetylcholine (nACh), 5-hydroxytryptamine receptor (5-HT3/serotonin), the gamma-aminobutyric acid Type-A receptor (GABAAR), and glycine receptor. The ionic conductance of the Cys-loop receptors varies between the subtypes. For example, nicotinic acetylcholine and serotonin (5-HT3) receptors are cationic-selective channels that selectively depolarize the cell. On the other hand, GABAARs and glycine channels are anionic-selective which serve to hyperpolarize developmentally mature cells.
All Cys-loop receptors share a similar superstructure: an extracellular domain for ligand binding; a transmembrane domain for ion passage; and an intracellular domain for the potential regulation of channel conductance (Thompson et al., 2010). The transmembrane portion of the receptor consists of a pentameric topology/structure with a central pore for ion passage upon binding of the ligand (Figure 1). We focus on the GABAAR due to its proposed role in cell proliferation which highlights it as a potential pro-cancerous ligand-gated ionotropic channel (Takehara et al., 2007; Young and Bordey, 2009; Bhattacharya et al., 2021).
The endogenous GABAAR ligand, GABA, is a product of the decarboxylation of glutamic acid catalyzed by glutamic acid decarboxylase (GAD65, GAD67) (Olsen and DeLorey, 1999). Transcript levels of GAD are thus frequently used as a proxy for the identification of GABAergic neurons. The action of GABA is abrogated by its metabolism by GABA transaminase (Olsen and DeLorey, 1999; Young and Bordey, 2009). GABA is thought to regulate cell dynamics through at least two receptor subtypes—the GABAAR and the GABABR, which is a metabotropic G-protein coupled receptor (GPCR).
Even within the GABAAR subtype there exists significant compositional heterogeneity as 19 receptor subtype genes have been identified in humans, coding for six α, three β, three γ, three ρ, one δ, one ε, one π, and one θ-subunit (Sigel and Steinmann, 2012; Ghit et al., 2021). The most common adult human assembly is of a hetero-pentamer consisting of two α, two β, and one γ-subunit encoded by GABR genes GABRA, GABRB, and GABRG, respectively. The ligand (GABA) binding site is at the interface between α- and β-subunits (Figure 4; Olsen, 2018; Bhattacharya et al., 2021). Normally, a GABAAR allows for the passage of chloride anions intracellularly through the transmembrane pentameric pore following the binding of GABA. This in turn leads to a hyperpolarization of the mitochondrial transmembrane, which is characteristically associated with neuro-suppressive effects in the central nervous system (CNS). However, critical to this discussion is the “GABA switch.” During the post-natal period, neurons change the intracellular concentration of chloride anions [Cl–] due to the expression of certain channels. In particular, the Na+-K+-2Cl– (NKCC1, SLC12A2) transporter is highly expressed in immature neurons and is responsible for the high intracellular [Cl–]. The delayed expression of the K+-Cl– cotransporter (KCC2) is thought to be responsible for the reduction in intracellular [Cl–] (Kandler and Friauf, 1995). As a product of an increased extracellular to the intracellular ratio of chloride anion, GABAARs act to hyperpolarize rather than depolarize the cell following the GABA switch (Ganguly et al., 2001). Interestingly, GABAAR in cancer cells behaves similarly to GABAARs in immature, developing neurons as assessed in part by patch-clamp electrophysiology (Labrakakis et al., 1998; Sengupta et al., 2014; Kallay et al., 2019). Given that cell depolarization is commonly associated with proliferative pathways linked to malignant transformation, this detail is key to the hypothesized role of GABAARs in cancer.
Figure 4. How type-A GABA receptors may function as an electrochemical vulnerability to cancer cells. (A) In an embryonic cell, the GABAA receptor upon binding of GABA moves chloride anions out of the cell and is depolarizing (top), while in a mature neuron, GABAA receptors move chloride anions into the cell upon binding of GABA and is hyperpolarizing (bottom). (B) In cancer cells, the direction of ion flow is out of the cell, like that of the embryonic receptor. (C) Anion flow is enhanced upon binding of a benzodiazepine, a positive allosteric modulator of the GABAA receptor. Within minutes of benzodiazepine binding to the GABAA receptor, the significant efflux of chloride anions depolarizes the mitochondrial transmembrane as well causes their fission. In addition, there is enhanced expression of the oncogene TP53. (D) The intrinsic (mitochondrial) apoptotic pathway is activated, including an enhanced expression and localization of the BCL2 Associated Agonist of Cell Death (BAD) protein. There are also significant morphological changes in the cancer cells, including DNA duplication, as illustrated. Created with BioRender.com.
Gamma-aminobutyric acid type-A receptor is not functionally distinct to the CNS. GABAAR subunits are expressed throughout the body, including in the lungs, pancreas, kidney, intestine, prostate, and skin (Napoleone et al., 1990; Gilon et al., 1991; Borboni et al., 1994; Jin et al., 2008; Takano et al., 2014) where they have been shown to contribute to a diverse range of functions, including modulating proliferation, regulating secretory function, and vascular control (Ong and Kerr, 1990; Erlitzki et al., 2000; Watanabe et al., 2002; Bansal et al., 2011).
GABAAR subunits expression is enhanced in both CNS and systemic cancers, including pediatric and adult brain tumors (Cho et al., 2011) and gastric, pancreatic, ovarian, and breast cancers (Hujber et al., 2018; Jiang et al., 2020). In addition, GABAARs are not only significantly upregulated in medulloblastoma, a pediatric brain cancer, and melanoma but also are a functional GABA-responsive receptor (Sengupta et al., 2014; Pomeranz Krummel et al., 2021). The putative pathway of GABA in the proliferation of these cancers has yet to be fully elucidated, but one prevailing theory is that the lack of a GABA switch with subsequent retention of a depolarizing phenotype in many cancers allows for pro-tumorigenic pathways to be upregulated. In support of this theory is research done on pancreatic cancer cells which showed that enhancing GABAAR activity with an agonist-induced a Ca2+ influx, resulting in subsequent MAPK/ERK pathway activation (Takehara et al., 2007). This MAPK/ERK activation was reduced by the administration of a calcium chelator, further implicating calcium in this pathway. Aside from inducing proliferation, GABAergic signaling and metabolism may aid tumor metastases to survive in the brain microenvironment. Neman et al. (2014) showed that breast-to-brain metastatic cells displayed increased GABA metabolism and a strong association between HER2 and reelin, a protein expressed by GABAergic neurons, implicating this phenotype with control over cell motility and cytoskeletal shape. These studies imply that a hyperactivation of GABAAR may be partially responsible for a tumorigenic phenotype.
Conversely, other research has shown that GABA agonism may inhibit cancer proliferation and growth which complicates the therapeutic landscape given the discrepancy with the aforementioned literature. GABA signaling has been implicated in the maintenance of embryonic (ESC) and neural stem cells (NSC) in the stem cell niche, preventing proliferation (Wang et al., 2008). Paradoxically, this mechanism is found to be a hyperpolarizing effect of GABAAR despite occurring in stem cells, which presumably have not undergone a GABA-switch (Wang et al., 2008). GABAAR is thought to activate S-phase checkpoint regulatory kinases such as PIKK which in turn phosphorylate the histone H2AX, correlated with decreased proliferation in ESC and NSC cells (Andäng et al., 2008). This mechanism may provide an explanation as to why in human hepatocellular carcinoma, prostate cancer, and some brain tumors the downregulation of GABAAR allows for increased tumor proliferation (Young and Bordey, 2009).
Given GABA’s documented role in proliferation and stem cell maintenance, is the GABAergic system a possible target for anti-tumor activity? GABAARs are already a highly targeted neurotransmitter receptor subtype for the treatment of anxiety, insomnia, and epilepsy. Although benzodiazepines have received “bad press” as of late, due to their addictive potential (Oransky, 2005). An epidemiological study conducted by Kleinerman et al. revealed that patients on diazepam fared better, e.g., exhibited smaller invasive tumors and less lymph node involvement, than patients not taking the anxiolytic (Kleinerman et al., 1984). This retrospective study inspired our lab to explore the mechanism of benzodiazepine action in cancer cells. Benzodiazepine treatment in medulloblastoma and melanoma cells and respective murine models for these cancers impairs viability via mitochondrial depolarization and the intrinsic (mitochondrial) apoptotic pathway (Figure 4; Sengupta et al., 2014; Jonas et al., 2016; Kallay et al., 2019; Pomeranz Krummel et al., 2021). This anti-proliferative effect of a positive allosteric modulator, a benzodiazepine, is consistent with the earlier discussion of GABAAR and the maintenance of the stem cell niche. Given that benzodiazepines are often used as an anxiolytic during radiation treatment for a multitude of tumors, having this as a potential anti-cancer therapeutic in the “clinicians’ toolbox” would be valuable.
Importantly, benzodiazepines not only alone can impair the viability of cancer cells but also appear capable of sensitizing the cancer cells to radiation, chemotherapeutic, and an immune checkpoint inhibitor. If benzodiazepines can reduce the dose necessary to achieve the efficacy of these treatments, this may represent an approach to improve quality of life or reduce patient co-morbidities, because of these treatments. Underlying the basis for such sensitization by modulating the GABAAR may be due to an interaction of the receptor with an intracellular protein called GABARAP or GABA Type-A Receptor-Associated Protein (Pérez-Hernández et al., 2019). GABARAP has been reported to be an important contributor to the autophagosomes mediating autophagy, the process by which lysosomes degrade and recycle damaged organelles as a means for cells to control proliferation and the formation of radical inorganic species (Liu et al., 2016). This provides a putative mechanism linking the activation of GABAAR to the autophagy pathway.
Another potential pathway by which GABAergic signaling can influence tumor cell death is by modulating cells of the immune system. GABAARs appear to have roles in many immune cell subtypes, including T-cells, dendritic cells, macrophages, and neutrophils (Jin et al., 2013; Bhattacharya et al., 2021). Moreover, several immune cells have the capability of synthesizing and secreting GABA, including T-cells, macrophages, and dendritic cells (Bhat et al., 2010; Dionisio et al., 2011).
Treatment of cancer comes with a host of constitutional symptoms that significantly impact the quality of life of patients, including anxiety, insomnia, bone pain, and chemotherapy-induced nausea or vomiting (Triozzi et al., 1988). Anxiety may arise from the overall prospect of the disease itself or context-specific situations, such as radiotherapy. Benzodiazepines are commonly used as an anxiolytic during events such as radiation therapy. Bone pain can present as a consequence of a cancer metastasis. Nausea and vomiting are common side effects of chemotherapeutics. Regardless of the origin of symptoms, benzodiazepines and other GABAAR modulators are useful therapeutics, as they simultaneously treat all of the above symptoms.
Work in a rat model of CIBP found that GABA was downregulated in CIBP rats in combination with the upregulation of GABA transporter type-1 (GAT-1) in spinal cord astrocytes (Ge et al., 2019). Interestingly, administration of exogenous GAD and NO-711 (a GAT-1 specific-inhibitor) significantly reversed CIBP-induced mechanical allodynia (Ge et al., 2019). This suggests that the upregulation of GABAergic signaling has the potential to be an analgesic in CIBP. Moreover, given the effects that GABA agonism has been shown to have on tumor regression by Sengupta et al. (2014) this suggests a dual benefit to GABA treatment for cancer—addressing the symptoms and root cause simultaneously. This treatment must also be contextualized in light of the research by Neman et al. (2014) and Takehara et al. (2007) which implicated GABA signaling in tumor proliferation. Likely tissue-specific factors will determine the efficacy of GABA agonism or antagonism therapy.
Members of the three ligand-gated ionotropic channel superfamilies are implicated in both the progression and symptomatology of a variety of tumors. Alterations in purinergic, glutamatergic, and Cys-loop signaling have been shown to increase tumor proliferation via induction of intracellular signaling pathways. Some preliminary research has been conducted in terms of both “antagonism” and “agonism” of these receptor subtypes and a resulting decrease in proliferation and/or migration of tumor cells both in vitro and in vivo. Aside from the effects on tumor proliferation, antagonism of these receptor subtypes can also be helpful in the management of cancer-associated symptoms, such as cancer-induced bone pain and seizures. Given the multifaceted nature of ligand-gated ionotropic receptors in cancer, modulating these systems may present a potent method to simultaneously improve symptoms and outcomes.
Most ion channel drug development up to this point has focused on small molecule and peptide modulators which have allosteric or direct modulation of the ligand-gated ion channel’s pore function. Initially, these compounds were found serendipitously, mostly from natural compounds (Kalia et al., 2015). However, as more structures of members of these receptor families were determined, biochemists were able to better rationally design compounds based on putative binding sites (Zhao et al., 2021). Recently, there is increased research into employing monoclonal antibodies against ligand-gated ionotropic channels. There is a Phase 1 clinical trial underway employing monoclonal antibodies against P2X7R to treat basal cell carcinoma (Biosceptre, 2016; Hutchings et al., 2018). Other clinical trials have focused on antagonizing the mGluR1 signaling pathway using riluzole with secondary outcomes being tumor shrinkage (Barbara Ann Karmanos Cancer Institute, 2013; Rutgers, The State University of New Jersey, 2014). However, these trials have been terminated due to a lack of funding and accrual. Clinical trials involving GABAAR remain sparse despite promising in vitro data.
There remains much to be discovered both in terms of mechanism and off-target effects of ligand-gated ionotropic channel synthetic modulators. In particular, either a positive or negative allosteric modulator in one cancer subtype may have a differing effect compared to the same compound in another cancer tissue. This may be a product of receptor subtype variants (i.e., splice variants) or differing intracellular signaling mechanisms. Regardless, targeting ligand-gated ionotropic channels remains a promising pathway to offering targeted therapeutics for cancer treatment.
RR and SS drafted and edited the manuscript. DB, DT, RC, DAPK, and SSG contributed to the writing and editing of the manuscript. All authors contributed to the article and approved the submitted version.
SSG was supported by the Harold C. Schott Endowment and the Pam and Tom Mischell Funds. RR thanks the American Brain Tumor Association Jack & Fay Netchin Medical Student Summer Fellowship supported by the Uncle Kory Foundation.
DAPK and SSG were co-founders of Amlal Phamaceuticals, Inc. DAPK is the President/CEO of Amlal Pharmaceuticals, Inc.
The remaining authors declare that the research was conducted in the absence of any commercial or financial relationships that could be construed as a potential conflict of interest.
All claims expressed in this article are solely those of the authors and do not necessarily represent those of their affiliated organizations, or those of the publisher, the editors and the reviewers. Any product that may be evaluated in this article, or claim that may be made by its manufacturer, is not guaranteed or endorsed by the publisher.
Adinolfi, E., Callegari, M. G., Ferrari, D., Bolognesi, C., Minelli, M., Wieckowski, M. R., et al. (2005). Basal activation of the P2X7 ATP receptor elevates mitochondrial calcium and potential, increases cellular ATP levels, and promotes serum-independent growth. Mol. Biol. Cell 16, 3260–3272. doi: 10.1091/mbc.e04-11-1025
Adinolfi, E., Raffaghello, L., Giuliani, A. L., Cavazzini, L., Capece, M., Chiozzi, P., et al. (2012). Expression of P2X7 receptor increases in vivo tumor growth. Cancer Res. 72, 2957–2969. doi: 10.1158/0008-5472.CAN-11-1947
Albayrak, G., Konac, E., Dikmen, A. U., and Bilen, C. Y. (2018). Memantine induces apoptosis and inhibits cell cycle progression in LNCaP prostate cancer cells. Hum. Exp. Toxicol. 37, 953–958. doi: 10.1177/0960327117747025
Amoroso, F., Capece, M., Rotondo, A., Cangelosi, D., Ferracin, M., Franceschini, A., et al. (2015). The P2X7 receptor is a key modulator of the PI3K/GSK3β/VEGF signaling network: evidence in experimental neuroblastoma. Oncogene 34, 5240–5251. doi: 10.1038/onc.2014.444
Andäng, M., Hjerling-Leffler, J., Moliner, A., Lundgren, T. K., Castelo-Branco, G., Nanou, E., et al. (2008). Histone H2AX-dependent GABAA receptor regulation of stem cell proliferation. Nature 451, 460–464. doi: 10.1038/nature06488
Asif, A., Khalid, M., Manzoor, S., Ahmad, H., and Rehman, A. U. (2019). Role of purinergic receptors in hepatobiliary carcinoma in Pakistani population: an approach towards proinflammatory role of P2X4 and P2X7 receptors. Purinerg. Signal. 15, 367–374. doi: 10.1007/s11302-019-09675-0
Azimi, I., Beilby, H., Davis, F. M., Marcial, D. L., Kenny, P. A., Thompson, E. W., et al. (2016). Altered purinergic receptor-Ca2+ signaling associated with hypoxia-induced epithelial-mesenchymal transition in breast cancer cells. Mol. Oncol. 10, 166–178. doi: 10.1016/j.molonc.2015.09.006
Bansal, P., Wang, S., Liu, S., Xiang, Y.-Y., Lu, W.-Y., and Wang, Q. (2011). GABA Coordinates with Insulin in Regulating Secretory Function in Pancreatic INS-1 β-Cells. PLoS One 6:e26225. doi: 10.1371/journal.pone.0026225
Barbara Ann Karmanos Cancer Institute (2013). Metabotropic Glutamate Receptor-1 (mGluR1): validation of a Serendipitously Discovered Molecular Target for Breast Cancer Treatment (Clinical trial registration No. NCT00903214). United States: Barbara Ann Karmanos Cancer Institute.
Bean, B. P. (1990). ATP-activated channels in rat and bullfrog sensory neurons: concentration dependence and kinetics. J. Neurosci. 10, 1–10.
Bele, T., and Fabbretti, E. (2015). P2X receptors, sensory neurons and pain. Curr. Med. Chem. 22, 845–850. doi: 10.2174/0929867321666141011195351
Bettler, B., Collingridge, G. L., Dingledine, R., Heinemann, S. F., Hollmann, M., Lerma, J., et al. (2019). Ionotropic glutamate receptors (version 2019.4) in the IUPHAR/BPS Guide to Pharmacology Database. UPHAR BPS Guide Pharm. CITE 2019. doi: 10.2218/gtopdb/F75/2019.4
Bhat, R., Axtell, R., Mitra, A., Miranda, M., Lock, C., Tsien, R. W., et al. (2010). Inhibitory role for GABA in autoimmune inflammation. Proc. Natl. Acad. Sci. U. S. A. 107, 2580–2585. doi: 10.1073/pnas.0915139107
Bhattacharya, D., Gawali, V. S., Kallay, L., Toukam, D. K., Koehler, A., Stambrook, P., et al. (2021). Therapeutically leveraging GABAA receptors in cancer. Exp. Biol. Med. 246, 2128–2135. doi: 10.1177/15353702211032549
Bianchi, M. T., and Botzolakis, E. J. (2010). Targeting ligand-gated ion channels in neurology and psychiatry: is pharmacological promiscuity an obstacle or an opportunity? BMC Pharmacol. 10:3. doi: 10.1186/1471-2210-10-3
Biosceptre (2016). An Open-Label Phase 1 Investigation of the Safety and Tolerability of BSCT (Anti-nf-P2X7) 10% Ointment When Applied Twice Daily for 28 Days in Male and Female PatientsWith Basal Cell Carcinoma. clinicaltrials.gov.
Blanke, M. L., and VanDongen, A. M. J. (2009). “Activation Mechanisms of the NMDA Receptor” in Biology of the NMDA Receptor. ed. A. M. Van Dongen (Boca Raton: CRC Press/Taylor & Francis).
Bonora, M., Patergnani, S., Rimessi, A., De Marchi, E., Suski, J. M., Bononi, A., et al. (2012). ATP synthesis and storage. Purinergic Signal. 8, 343–357. doi: 10.1007/s11302-012-9305-8
Borboni, P., Porzio, O., Fusco, A., Sesti, G., Lauro, R., and Marlier, L. N. (1994). Molecular and cellular characterization of the GABAA receptor in the rat pancreas. Mol. Cell. Endocrinol. 103, 157–163. doi: 10.1016/0303-7207(94)90083-3
Brake, A. J., Wagenbach, M. J., and Julius, D. (1994). New structural motif for ligand-gated ion channels defined by an ionotropic ATP receptor. Nature 371, 519–523. doi: 10.1038/371519a0
Buckingham, S. C., Campbell, S. L., Haas, B. R., Montana, V., Robel, S., Ogunrinu, T., et al. (2011). Glutamate release by primary brain tumors induces epileptic activity. Nat. Med. 17, 1269–1274. doi: 10.1038/nm.2453
Budczies, J., Pfitzner, B. M., Györffy, B., Winzer, K.-J., Radke, C., Dietel, M., et al. (2015). Glutamate enrichment as new diagnostic opportunity in breast cancer. Int. J. Cancer 136, 1619–1628. doi: 10.1002/ijc.29152
Burnstock, G., Fredholm, B. B., North, R. A., and Verkhratsky, A. (2010). The birth and postnatal development of purinergic signalling. Acta Physiol. 199, 93–147. doi: 10.1111/j.1748-1716.2010.02114.x
Cai, X., Yao, Y., Teng, F., Li, Y., Wu, L., Yan, W., et al. (2021). The role of P2X7 receptor in infection and metabolism: based on inflammation and immunity. Int. Immunopharmacol. 101:108297. doi: 10.1016/j.intimp.2021.108297
Campos-Contreras, A., del, R., Díaz-Muñoz, M., and Vázquez-Cuevas, F. G. (2020). Purinergic Signaling in the Hallmarks of Cancer. Cells 9:1612. doi: 10.3390/cells9071612
Cho, Y.-J., Tsherniak, A., Tamayo, P., Santagata, S., Ligon, A., Greulich, H., et al. (2011). Integrative genomic analysis of medulloblastoma identifies a molecular subgroup that drives poor clinical outcome. J. Clin. Oncol. 29, 1424–1430. doi: 10.1200/JCO.2010.28.5148
Choe, G., Horvath, S., Cloughesy, T. F., Crosby, K., Seligson, D., Palotie, A., et al. (2003). Analysis of the phosphatidylinositol 3’-kinase signaling pathway in glioblastoma patients in vivo. Cancer Res. 63, 2742–2746.
Collingridge, G. L., Olsen, R. W., Peters, J., and Spedding, M. (2009). A nomenclature for ligand-gated ion channels. Neuropharmacology 56, 2–5. doi: 10.1016/j.neuropharm.2008.06.063
Dai, W.-L., Yan, B., Jiang, N., Wu, J.-J., Liu, X.-F., Liu, J.-H., et al. (2017). Simultaneous inhibition of NMDA and mGlu1/5 receptors by levo-corydalmine in rat spinal cord attenuates bone cancer pain. Int. J. Cancer 141, 805–815. doi: 10.1002/ijc.30780
De Marchi, E., Orioli, E., Pegoraro, A., Adinolfi, E., and Di Virgilio, F. (2020). “Detection of Extracellular ATP in the Tumor Microenvironment, Using the pmeLUC Biosensor” in Purinergic Signaling: methods and Protocols, Methods in Molecular Biology. ed. P. Pelegrín (New York: Springer). 183–195. doi: 10.1007/978-1-4939-9717-6_13
Di Virgilio, F., Sarti, A. C., Falzoni, S., De Marchi, E., and Adinolfi, E. (2018a). Extracellular ATP and P2 purinergic signalling in the tumour microenvironment. Nat. Rev. Cancer 18, 601–618. doi: 10.1038/s41568-018-0037-0
Di Virgilio, F., Sarti, A. C., and Grassi, F. (2018b). Modulation of innate and adaptive immunity by P2X ion channels. Curr. Opin. Immunol. 52, 51–59. doi: 10.1016/j.coi.2018.03.026
Di Virgilio, F., Vultaggio-Poma, V., and Sarti, A. C. (2021). P2X receptors in cancer growth and progression. Biochem. Pharmacol. 187:114350. doi: 10.1016/j.bcp.2020.114350
Dionisio, L., José De Rosa, M., Bouzat, C., and Esandi, M. D. C. (2011). An intrinsic GABAergic system in human lymphocytes. Neuropharmacology 60, 513–519. doi: 10.1016/j.neuropharm.2010.11.007
Ellingson, H. M., and Vanderah, T. W. (2020). Potential Therapeutic Treatments of Cancer Induced Bone Pain. Curr. Opin. Supp. Pall. Care 14, 107–111. doi: 10.1097/SPC.0000000000000496
Erlitzki, R., Gong, Y., Zhang, M., and Minuk, G. (2000). Identification of γ-aminobutyric acid receptor subunit types in human and rat liver. Am. J. Physiol. Gastrointest. Liver Physiol. 279, G733–G739. doi: 10.1152/ajpgi.2000.279.4.G733
Farrell, A. W., Gadeock, S., Pupovac, A., Wang, B., Jalilian, I., Ranson, M., et al. (2010). P2X7 receptor activation induces cell death and CD23 shedding in human RPMI 8226 multiple myeloma cells. Biochim. Biophys. Acta 1800, 1173–1182. doi: 10.1016/j.bbagen.2010.07.001
Ganguly, K., Schinder, A. F., Wong, S. T., and Poo, M. (2001). GABA Itself Promotes the Developmental Switch of Neuronal GABAergic Responses from Excitation to Inhibition. Cell 105, 521–532. doi: 10.1016/S0092-8674(01)00341-5
Ganor, Y., Grinberg, I., Reis, A., Cooper, I., Goldstein, R. S., and Levite, M. (2009). Human T-leukemia and T-lymphoma express glutamate receptor AMPA GluR3, and the neurotransmitter glutamate elevates the cancer-related matrix-metalloproteinases inducer CD147/EMMPRIN, MMP-9 secretion and engraftment of T-leukemia in vivo. Leuk Lymphoma 50, 985–997. doi: 10.1080/10428190902878448
Ge, M.-M., Chen, S.-P., Zhou, Y.-Q., Li, Z., Tian, X.-B., Gao, F., et al. (2019). The therapeutic potential of GABA in neuron-glia interactions of cancer-induced bone pain. Eur. J. Pharmacol. 858:172475. doi: 10.1016/j.ejphar.2019.172475
Ghit, A., Assal, D., Al-Shami, A. S., and Hussein, D. E. E. (2021). GABAA receptors: structure, function, pharmacology, and related disorders. J. Genet. Eng. Biotechnol. 19:123. doi: 10.1186/s43141-021-00224-0
Gilon, P., Bertrand, G., Loubatières-Mariani, M. M., Remacle, C., and Henquin, J. C. (1991). The influence of gamma-aminobutyric acid on hormone release by the mouse and rat endocrine pancreas. Endocrinology 129, 2521–2529. doi: 10.1210/endo-129-5-2521
Gómez-Villafuertes, R., García-Huerta, P., Díaz-Hernández, J. I., and Miras-Portugal, M. T. (2015). PI3K/Akt signaling pathway triggers P2X7 receptor expression as a pro-survival factor of neuroblastoma cells under limiting growth conditions. Sci. Rep. 5:18417. doi: 10.1038/srep18417
Gong, B., Li, Y., Cheng, Z., Wang, P., Luo, L., Huang, H., et al. (2017). GRIK3: A novel oncogenic protein related to tumor TNM stage, lymph node metastasis, and poor prognosis of GC. Tumour Biol. 39:1010428317704364. doi: 10.1177/1010428317704364
Gong, D., Zhang, J., Chen, Y., Xu, Y., Ma, J., Hu, G., et al. (2019). The m6A-suppressed P2RX6 activation promotes renal cancer cells migration and invasion through ATP-induced Ca2+ influx modulating ERK1/2 phosphorylation and MMP9 signaling pathway. J. Exp. Clin. Cancer Res. 38:233. doi: 10.1186/s13046-019-1223-y
Grassi, F., and De Ponte Conti, B. (2021). The P2X7 Receptor in Tumor Immunity. Front. Cell. Dev. Biol. 9:694831. doi: 10.3389/fcell.2021.694831
Gu, B., Bendall, L. J., and Wiley, J. S. (1998). Adenosine Triphosphate–Induced Shedding of CD23 and L-Selectin (CD62L) From Lymphocytes Is Mediated by the Same Receptor but Different Metalloproteases. Blood 92, 946–951. doi: 10.1182/blood.V92.3.946
Hattori, F., Ohshima, Y., Seki, S., Tsukimoto, M., Sato, M., Takenouchi, T., et al. (2012). Feasibility study of B16 melanoma therapy using oxidized ATP to target purinergic receptor P2X7. Eur. J. Pharmacol. 695, 20–26. doi: 10.1016/j.ejphar.2012.09.001
Hayes, J. D., Dinkova-Kostova, A. T., and Tew, K. D. (2020). Oxidative Stress in Cancer. Cancer Cell 38, 167–197. doi: 10.1016/j.ccell.2020.06.001
He, Y., Taylor, N., Fourgeaud, L., and Bhattacharya, A. (2017). The role of microglial P2X7: modulation of cell death and cytokine release. J. Neuroinflammation 14:135. doi: 10.1186/s12974-017-0904-8
Hendriks, J., Gravestein, L. A., Tesselaar, K., van Lier, R. A. W., Schumacher, T. N. M., and Borst, J. (2000). CD27 is required for generation and long-term maintenance of T cell immunity. Nat. Immunol. 1, 433–440. doi: 10.1038/80877
Herner, A., Sauliunaite, D., Michalski, C. W., Erkan, M., Oliveira, T. D., Abiatari, I., et al. (2011). Glutamate increases pancreatic cancer cell invasion and migration via AMPA receptor activation and Kras-MAPK signaling. Int. J. Cancer 129, 2349–2359. doi: 10.1002/ijc.25898
Hu, K., Li, K., Lv, J., Feng, J., Chen, J., Wu, H., et al. (2020). Suppression of the SLC7A11/glutathione axis causes synthetic lethality in KRAS-mutant lung adenocarcinoma. J. Clin. Invest 130, 1752–1766. doi: 10.1172/JCI124049
Hu, L.-P., Zhang, X.-X., Jiang, S.-H., Tao, L.-Y., Li, Q., Zhu, L.-L., et al. (2019). Targeting Purinergic Receptor P2Y2 Prevents the Growth of Pancreatic Ductal Adenocarcinoma by Inhibiting Cancer Cell Glycolysis. Clin. Cancer Res. 25, 1318–1330. doi: 10.1158/1078-0432.CCR-18-2297
Huang, Y., Kang, B. N., Tian, J., Liu, Y., Luo, H. R., Hester, L., et al. (2007). The Cationic Amino Acid Transporters CAT1 and CAT3 Mediate NMDA Receptor Activation-Dependent Changes in Elaboration of Neuronal Processes via the Mammalian Target of Rapamycin mTOR Pathway. J. Neurosci. 27, 449–458. doi: 10.1523/JNEUROSCI.4489-06.2007
Huang, Z., Xie, N., Illes, P., Di Virgilio, F., Ulrich, H., Semyanov, A., et al. (2021). From purines to purinergic signalling: molecular functions and human diseases. Sig. Transduct. Target Ther. 6, 1–20. doi: 10.1038/s41392-021-00553-z
Hujber, Z., Horváth, G., Petõvári, G., Krencz, I., Dankó, T., Mészáros, K., et al. (2018). GABA, glutamine, glutamate oxidation and succinic semialdehyde dehydrogenase expression in human gliomas. J. Exp. Clin. Cancer Res. 37:271. doi: 10.1186/s13046-018-0946-5
Huo, J.-F., and Chen, X.-B. (2019). P2X4R silence suppresses glioma cell growth through BDNF/TrkB/ATF4 signaling pathway. J. Cell. Biochem. 120, 6322–6329. doi: 10.1002/jcb.27919
Hutchings, C. J., Colussi, P., and Clark, T. G. (2018). Ion channels as therapeutic antibody targets. MAbs 11, 265–296. doi: 10.1080/19420862.2018.1548232
Ishiuchi, S., Yoshida, Y., Sugawara, K., Aihara, M., Ohtani, T., Watanabe, T., et al. (2007). Ca2+-Permeable AMPA Receptors Regulate Growth of Human Glioblastoma via Akt Activation. J. Neurosci. 27, 7987–8001. doi: 10.1523/JNEUROSCI.2180-07.2007
Hreich, S. J. D., Benzaquen, J., Hofman, P., and Vouret-Craviari, V. (2021). To inhibit or to boost the ATP/P2RX7 pathway to fight cancer-that is the question. Purinergic Signal. 17, 619–631. doi: 10.1007/s11302-021-09811-9
Jelassi, B., Chantôme, A., Alcaraz-Pérez, F., Baroja-Mazo, A., Cayuela, M. L., Pelegrin, P., et al. (2011). P2X7 receptor activation enhances SK3 channels- and cystein cathepsin-dependent cancer cells invasiveness. Oncogene 30, 2108–2122. doi: 10.1038/onc.2010.593
Jiang, S.-H., Hu, L.-P., Wang, X., Li, J., and Zhang, Z.-G. (2020). Neurotransmitters: emerging targets in cancer. Oncogene 39, 503–515. doi: 10.1038/s41388-019-1006-0
Jin, N., Guo, Y., Sun, P., Bell, A., Chintagari, N. R., Bhaskaran, M., et al. (2008). IONOTROPIC GABA RECEPTOR EXPRESSION IN THE LUNG DURING DEVELOPMENT. Gene Expr. Patt. 8, 397–403. doi: 10.1016/j.gep.2008.04.008
Jin, Z., Mendu, S. K., and Birnir, B. (2013). GABA is an effective immunomodulatory molecule. Amino. Acids 45, 87–94. doi: 10.1007/s00726-011-1193-7
Jonas, O., Calligaris, D., Methuku, K. R., Poe, M. M., Francois, J. P., Tranghese, F., et al. (2016). First in vivo testing of compounds targeting group 3 medulloblastomas using an implantable microdevice as a new paradigm for drug development. J. Biomed. Nanotechnol. 12, 1297–1302. doi: 10.1166/jbn.2016.2262
Jouaville, L. S., Pinton, P., Bastianutto, C., Rutter, G. A., and Rizzuto, R. (1999). Regulation of mitochondrial ATP synthesis by calcium: evidence for a long-term metabolic priming. Proc. Natl. Acad. Sci. U. S. A. 96, 13807–13812.
Kaan, T. K. Y., Yip, P. K., Patel, S., Davies, M., Marchand, F., Cockayne, D. A., et al. (2010). Systemic blockade of P2X3 and P2X2/3 receptors attenuates bone cancer pain behaviour in rats. Brain 133, 2549–2564. doi: 10.1093/brain/awq194
Kakimoto, S., Nagakura, Y., Tamura, S., Watabiki, T., Shibasaki, K., Tanaka, S., et al. (2008). Minodronic acid, a third-generation bisphosphonate, antagonizes purinergic P2X(2/3) receptor function and exerts an analgesic effect in pain models. Eur. J. Pharmacol. 589, 98–101. doi: 10.1016/j.ejphar.2008.05.011
Kalia, J., Milescu, M., Salvatierra, J., Wagner, J., Klint, J. K., King, G. F., et al. (2015). From foe to friend: using animal toxins to investigate ion channel function. J. Mol. Biol. 427, 158–175. doi: 10.1016/j.jmb.2014.07.027
Kallay, L., Keskin, H., Ross, A., Rupji, M., Moody, O. A., Wang, X., et al. (2019). Modulating native GABAA receptors in medulloblastoma with positive allosteric benzodiazepine-derivatives induces cell death. J. Neurooncol. 142, 411–422. doi: 10.1007/s11060-019-03115-0
Kandler, K., and Friauf, E. (1995). Development of glycinergic and glutamatergic synaptic transmission in the auditory brainstem of perinatal rats. J. Neurosci. 15, 6890–6904. doi: 10.1523/JNEUROSCI.15-10-06890.1995
Kew, J. N. C., and Kemp, J. A. (2005). Ionotropic and metabotropic glutamate receptor structure and pharmacology. Psychopharmacology 179, 4–29. doi: 10.1007/s00213-005-2200-z
Khalid, M., Manzoor, S., Ahmad, H., Asif, A., Bangash, T. A., Latif, A., et al. (2018). Purinoceptor expression in hepatocellular virus (HCV)-induced and non-HCV hepatocellular carcinoma: an insight into the proviral role of the P2X4 receptor. Mol. Biol. Rep. 45, 2625–2630. doi: 10.1007/s11033-018-4432-0
Kleinerman, R. A., Brinton, L. A., Hoover, R., and Fraumeni, J. F. (1984). Diazepam use and progression of breast cancer. Cancer Res. 44, 1223–1225.
Koochekpour, S., Majumdar, S., Azabdaftari, G., Attwood, K., Scioneaux, R., Subramani, D., et al. (2012). Serum glutamate levels correlate with Gleason score and glutamate blockade decreases proliferation, migration, and invasion and induces apoptosis in prostate cancer cells. Clin. Cancer Res. 18, 5888–5901. doi: 10.1158/1078-0432.CCR-12-1308
Koppenol, W. H., Bounds, P. L., and Dang, C. V. (2011). Otto Warburg’s contributions to current concepts of cancer metabolism. Nat. Rev. Cancer 11, 325–337. doi: 10.1038/nrc3038
Labrakakis, C., Patt, S., Hartmann, J., and Kettenmann, H. (1998). Functional GABA(A) receptors on human glioma cells. Eur. J. Neurosci. 10, 231–238. doi: 10.1046/j.1460-9568.1998.00036.x
Lange, F., Hörnschemeyer, J., and Kirschstein, T. (2021). Glutamatergic Mechanisms in Glioblastoma and Tumor-Associated Epilepsy. Cells 10:1226. doi: 10.3390/cells10051226
LeBoeuf, S. E., Wu, W. L., Karakousi, T. R., Karadal, B., Jackson, S. R., Davidson, S. M., et al. (2020). Activation of Oxidative Stress Response in Cancer Generates a Druggable Dependency on Exogenous Non-essential Amino Acids. Cell Metabol. 31, 339–350.e4. doi: 10.1016/j.cmet.2019.11.012
Ledderose, C., Woehrle, T., Ledderose, S., Strasser, K., Seist, R., Bao, Y., et al. (2016). Cutting off the power: inhibition of leukemia cell growth by pausing basal ATP release and P2X receptor signaling? Purinergic Signal. 12, 439–451. doi: 10.1007/s11302-016-9510-y
Li, S., Qian, J., Yang, Y., Zhao, W., Dai, J., Bei, J.-X., et al. (2012). GWAS identifies novel susceptibility loci on 6p21.32 and 21q21.3 for hepatocellular carcinoma in chronic hepatitis B virus carriers. PLoS Genet. 8:e1002791. doi: 10.1371/journal.pgen.1002791
Liberti, M. V., and Locasale, J. W. (2016). The Warburg Effect: how Does it Benefit Cancer Cells? Trends Biochem. Sci. 41, 211–218. doi: 10.1016/j.tibs.2015.12.001
Liu, H., He, Z., and Simon, H.-U. (2016). “Chapter 14 - The Role of Autophagy in Cancer and Chemotherapy” in Autophagy: cancer, Other Pathologies, Inflammation, Immunity, Infection, and Aging. ed. M. A. Hayat (San Diego: Academic Press). 253–265. doi: 10.1016/B978-0-12-802937-4.00014-4
Longley, D. B., Harkin, D. P., and Johnston, P. G. (2003). 5-Fluorouracil: mechanisms of action and clinical strategies. Nat. Rev. Cancer 3, 330–338. doi: 10.1038/nrc1074
Loomis, W. H., Namiki, S., Ostrom, R. S., Insel, P. A., and Junger, W. G. (2003). Hypertonic Stress Increases T Cell Interleukin-2 Expression through a Mechanism That Involves ATP Release, P2 Receptor, and p38 MAPK Activation*. J. Biol. Chem. 278, 4590–4596. doi: 10.1074/jbc.M207868200
Lustig, K. D., Shiau, A. K., Brake, A. J., and Julius, D. (1993). Expression cloning of an ATP receptor from mouse neuroblastoma cells. Proc. Natl. Acad. Sci. U. S. A. 90, 5113–5117. doi: 10.1073/pnas.90.11.5113
Matyśniak, D., Chumak, V., Nowak, N., Kukla, A., Lehka, L., Oslislok, M., et al. (2021). P2X7 Receptor: the Regulator of Glioma Tumor Development and Survival. Germany: Springer. doi: 10.1007/s11302-021-09834-2
Mayer, M. L. (2005). Glutamate receptor ion channels. Curr. Opin. Neurobiol. Signal. Mech. 15, 282–288. doi: 10.1016/j.conb.2005.05.004
Maynard, J. P., Lee, J.-S., Sohn, B. H., Yu, X., Lopez-Terrada, D., Finegold, M. J., et al. (2015). P2X3 purinergic receptor overexpression is associated with poor recurrence-free survival in hepatocellular carcinoma patients. Oncotarget 6, 41162–41179. doi: 10.18632/oncotarget.6240
Miller, P. S., and Smart, T. G. (2010). Binding, activation and modulation of Cys-loop receptors. Trends Pharmacol. Sci. 31, 161–174. doi: 10.1016/j.tips.2009.12.005
Mimoto, F., Tatsumi, K., Shimizu, S., Kadono, S., Haraya, K., Nagayasu, M., et al. (2020). Exploitation of Elevated Extracellular ATP to Specifically Direct Antibody to Tumor Microenvironment. Cell Rep. 33:108542. doi: 10.1016/j.celrep.2020.108542
Mohammed, A., Janakiram, N. B., Madka, V., Pathuri, G., Li, Q., Ritchie, R., et al. (2017). Lack of chemopreventive effects of P2X7R inhibitors against pancreatic cancer. Oncotarget 8, 97822–97834. doi: 10.18632/oncotarget.22085
Napoleone, P., Bronzetti, E., Cavallotti, C., and Amenta, F. (1990). Predominant epithelial localization of type A gamma-aminobutyric acid receptor sites within rat seminal vesicles and prostate glands. Pharmacology 41, 49–56. doi: 10.1159/000138698
Neman, J., Termini, J., Wilczynski, S., Vaidehi, N., Choy, C., Kowolik, C. M., et al. (2014). Human breast cancer metastases to the brain display GABAergic properties in the neural niche. Proc. Natl. Acad. Sci. U. S. A. 111, 984–989. doi: 10.1073/pnas.1322098111
North, R. A. (2002). Molecular Physiology of P2X Receptors. Physiol. Rev. 82, 1013–1067. doi: 10.1152/physrev.00015.2002
North, R. A. (2016). P2X receptors. Philos. Trans. R. Soc. Lond. B Biol. Sci. 371:20150427. doi: 10.1098/rstb.2015.0427
North, W. G., Gao, G., Jensen, A., Memoli, V. A., and Du, J. (2010). NMDA receptors are expressed by small-cell lung cancer and are potential targets for effective treatment. Clin. Pharmacol. 2, 31–40. doi: 10.2147/CPAA.S6262
North, W. G., Liu, F., Lin, L. Z., Tian, R., and Akerman, B. (2017). NMDA receptors are important regulators of pancreatic cancer and are potential targets for treatment. Clin. Pharmacol. 9, 79–86. doi: 10.2147/CPAA.S140057
Novak, I. (2003). ATP as a Signaling Molecule: the Exocrine Focus. Physiology 18, 12–17. doi: 10.1152/nips.01409.2002
Olsen, R. W. (2018). GABAA receptor: positive and negative allosteric modulators. Neuropharmacology 136, 10–22. doi: 10.1016/j.neuropharm.2018.01.036
Olsen, R. W., and DeLorey, T. M. (1999). GABA Synthesis, Uptake and Release. Basic Neurochemistry: molecular, Cellular and Medical Aspects. 6th edition. America: American Society for Neurochemistry.
Ong, J., and Kerr, D. I. B. (1990). GABA-receptors in peripheral tissues. Life Sci. 46, 1489–1501. doi: 10.1016/0024-3205(90)90421-M
Paoletti, P., Bellone, C., and Zhou, Q. (2013). NMDA receptor subunit diversity: impact on receptor properties, synaptic plasticity and disease. Nat. Rev. Neurosci. 14, 383–400. doi: 10.1038/nrn3504
Park, M., Kim, J., Phuong, N. T. T., Park, J. G., Park, J.-H., Kim, Y.-C., et al. (2019). Involvement of the P2X7 receptor in the migration and metastasis of tamoxifen-resistant breast cancer: effects on small extracellular vesicles production. Sci. Rep. 9:11587. doi: 10.1038/s41598-019-47734-z
Pegoraro, A., De Marchi, E., Ferracin, M., Orioli, E., Zanoni, M., Bassi, C., et al. (2021). P2X7 promotes metastatic spreading and triggers release of miRNA-containing exosomes and microvesicles from melanoma cells. Cell Death Dis. 12:1088. doi: 10.1038/s41419-021-04378-0
Pérez-Hernández, M., Arias, A., Martínez-García, D., Pérez-Tomás, R., Quesada, R., and Soto-Cerrato, V. (2019). Targeting Autophagy for Cancer Treatment and Tumor Chemosensitization. Cancers 11:1599. doi: 10.3390/cancers11101599
Pomeranz Krummel, D. A., Nasti, T. H., Kaluzova, M., Kallay, L., Bhattacharya, D., Melms, J. C., et al. (2021). Melanoma Cell Intrinsic GABAA Receptor Enhancement Potentiates Radiation and Immune Checkpoint Inhibitor Response by Promoting Direct and T Cell-Mediated Antitumor Activity. Int. J. Radiat. Oncol. Biol. Phys. 109, 1040–1053. doi: 10.1016/j.ijrobp.2020.10.025
Purves, D., Augustine, G. J., Fitzpatrick, D., Katz, L. C., LaMantia, A.-S., McNamara, J. O., et al. (2001). Glutamate Receptors. Neuroscience, 2nd Edn. Sunderland: Sinauer Associates.
Qiu, Y., Li, W., Zhang, H., Liu, Y., Tian, X.-X., and Fang, W.-G. (2014). P2X7 Mediates ATP-Driven Invasiveness in Prostate Cancer Cells. PLoS One 9:e114371. doi: 10.1371/journal.pone.0114371
Rabelo, I. L. A., Arnaud-Sampaio, V. F., Adinolfi, E., Ulrich, H., and Lameu, C. (2021). Cancer Metabostemness and Metabolic Reprogramming via P2X7 Receptor. Cells 10:1782. doi: 10.3390/cells10071782
Rutgers, The State University of New Jersey (2014). A Phase I Trial of Riluzole in Combination With Radiation Therapy in Patients Undergoing Whole Brain Radiation Therapy for Brain Metastasis. New Jersey: Rutgers, The State University of New Jersey.
Ryu, J. K., Jantaratnotai, N., Serrano-Perez, M. C., McGeer, P. L., and McLarnon, J. G. (2011). Block of Purinergic P2X7R Inhibits Tumor Growth in a C6 Glioma Brain Tumor Animal Model. J. Neuropathol. Exp. Neurol. 70, 13–22. doi: 10.1097/NEN.0b013e318201d4d4
Rzeski, W., Turski, L., and Ikonomidou, C. (2001). Glutamate antagonists limit tumor growth. Proc. Natl. Acad. Sci. U. S. A. 98, 6372–6377. doi: 10.1073/pnas.091113598
Salahuddin, M. M., Omran, G. A., Helmy, M. W., and Houssen, M. E. (2021). Effect of Regorafenib on P2X7 Receptor Expression and Different Oncogenic Signaling Pathways in a Human Breast Cancer Cell Line: a Potential of New Insight of the Antitumor Effects of Regorafenib. Curr. Issues Mol. Biol. 43, 2199–2209. doi: 10.3390/cimb43030154
Schenk, U., Westendorf, A. M., Radaelli, E., Casati, A., Ferro, M., Fumagalli, M., et al. (2008). Purinergic control of T cell activation by ATP released through pannexin-1 hemichannels. Sci. Signal. 1:ra6. doi: 10.1126/scisignal.1160583
Schousboe, A., Scafidi, S., Bak, L. K., Waagepetersen, H. S., and McKenna, M. C. (2014). Glutamate Metabolism in the Brain Focusing on Astrocytes. Adv. Neurobiol. 11, 13–30. doi: 10.1007/978-3-319-08894-5_2
Sengupta, S., Weeraratne, S. D., Sun, H., Phallen, J., Rallapalli, S. K., Teider, N., et al. (2014). α5-GABAA receptors negatively regulate MYC-amplified medulloblastoma growth. Acta Neuropathol. 127, 593–603. doi: 10.1007/s00401-013-1205-7
Sigel, E., and Steinmann, M. E. (2012). Structure, function, and modulation of GABA(A) receptors. J. Biol. Chem. 287, 40224–40231. doi: 10.1074/jbc.R112.386664
Singleton, D. C., and Harris, A. L. (2012). Targeting the ATF4 pathway in cancer therapy. Expert Opin. Ther. Targets 16, 1189–1202. doi: 10.1517/14728222.2012.728207
Sørensen, M. F., Heimisdóttir, S. B., Sørensen, M. D., Mellegaard, C. S., Wohlleben, H., Kristensen, B. W., et al. (2018). High expression of cystine–glutamate antiporter xCT (SLC7A11) is an independent biomarker for epileptic seizures at diagnosis in glioma. J. Neurooncol. 138, 49–53. doi: 10.1007/s11060-018-2785-9
Stelmashenko, O., Lalo, U., Yang, Y., Bragg, L., North, R. A., and Compan, V. (2012). Activation of Trimeric P2X2 Receptors by Fewer than Three ATP Molecules. Mol. Pharmacol. 82, 760–766. doi: 10.1124/mol.112.080903
Stepulak, A., Luksch, H., Gebhardt, C., Uckermann, O., Marzahn, J., Sifringer, M., et al. (2009). Expression of glutamate receptor subunits in human cancers. Histochem. Cell. Biol. 132, 435–445. doi: 10.1007/s00418-009-0613-1
Stepulak, A., Rola, R., Polberg, K., and Ikonomidou, C. (2014). Glutamate and its receptors in cancer. J. Neural. Transm. 121, 933–944. doi: 10.1007/s00702-014-1182-6
Stepulak, A., Sifringer, M., Rzeski, W., Brocke, K., Gratopp, A., Pohl, E. E., et al. (2007). AMPA antagonists inhibit the extracellular signal regulated kinase pathway and suppress lung cancer growth. Cancer Biol. Ther. 6, 1908–1915. doi: 10.4161/cbt.6.12.4965
Stepulak, A., Sifringer, M., Rzeski, W., Endesfelder, S., Gratopp, A., Pohl, E. E., et al. (2005). NMDA antagonist inhibits the extracellular signal-regulated kinase pathway and suppresses cancer growth. Proc. Natl. Acad. Sci. U. S. A. 102, 15605–15610. doi: 10.1073/pnas.0507679102
Stern-Bach, Y., Bettler, B., Hartley, M., Sheppard, P. O., O’Hara, P. J., and Heinemann, S. F. (1994). Agonist selectivity of glutamate receptors is specified by two domains structurally related to bacterial amino acid-binding proteins. Neuron 13, 1345–1357. doi: 10.1016/0896-6273(94)90420-0
Takano, K., Yatabe, M. S., Abe, A., Suzuki, Y., Sanada, H., Watanabe, T., et al. (2014). Characteristic expressions of GABA receptors and GABA producing/transporting molecules in rat kidney. PLoS One 9:e105835. doi: 10.1371/journal.pone.0105835
Takehara, A., Hosokawa, M., Eguchi, H., Ohigashi, H., Ishikawa, O., Nakamura, Y., et al. (2007). γ-Aminobutyric Acid (GABA) Stimulates Pancreatic Cancer Growth through Overexpressing GABAA Receptor π Subunit. Cancer Res. 67, 9704–9712. doi: 10.1158/0008-5472.CAN-07-2099
Tang, J., Xue, W., Xia, B., Ren, L., Tao, W., Chen, C., et al. (2015). Involvement of normalized NMDA receptor and mTOR-related signaling in rapid antidepressant effects of Yueju and ketamine on chronically stressed mice. Sci. Rep. 5:13573. doi: 10.1038/srep13573
Teng, Y., Zhang, Y., Yue, S., Chen, H., Qu, Y., Wei, H., et al. (2019). Intrathecal injection of bone marrow stromal cells attenuates neuropathic pain via inhibition of P2X4R in spinal cord microglia. J. Neuroinflammation 16:271. doi: 10.1186/s12974-019-1631-0
Thiery, J. P. (2002). Epithelial–mesenchymal transitions in tumour progression. Nat. Rev. Cancer 2, 442–454. doi: 10.1038/nrc822
Thompson, A. J., Lester, H. A., and Lummis, S. C. R. (2010). The structural basis of function in Cys-loop receptors. Quart. Rev. Biophys. 43, 449–499. doi: 10.1017/S0033583510000168
Trang, T., Beggs, S., Wan, X., and Salter, M. W. (2009). P2X4-receptor-mediated synthesis and release of brain-derived neurotrophic factor in microglia is dependent on calcium and p38-mitogen-activated protein kinase activation. J. Neurosci. 29, 3518–3528. doi: 10.1523/JNEUROSCI.5714-08.2009
Traynelis, S. F., Wollmuth, L. P., McBain, C. J., Menniti, F. S., Vance, K. M., Ogden, K. K., et al. (2010). Glutamate Receptor Ion Channels: structure. Regulation, and Function. Pharmacol. Rev. 62, 405–496. doi: 10.1124/pr.109.002451
Triozzi, P. L., Goldstein, D., and Laszlo, J. (1988). Contributions of Benzodiazepines to Cancer Therapy. Cancer Investig. 6, 103–111. doi: 10.3109/07357908809077033
Ulmann, L., Hatcher, J. P., Hughes, J. P., Chaumont, S., Green, P. J., Conquet, F., et al. (2008). Up-regulation of P2X4 receptors in spinal microglia after peripheral nerve injury mediates BDNF release and neuropathic pain. J. Neurosci. 28, 11263–11268. doi: 10.1523/JNEUROSCI.2308-08.2008
Ungard, R. G., Seidlitz, E. P., and Singh, G. (2014). Inhibition of breast cancer-cell glutamate release with sulfasalazine limits cancer-induced bone pain. Pain 155, 28–36. doi: 10.1016/j.pain.2013.08.030
Venkatesh, H. S., Morishita, W., Geraghty, A. C., Silverbush, D., Gillespie, S. M., Arzt, M., et al. (2019). Electrical and synaptic integration of glioma into neural circuits. Nature 573, 539–545. doi: 10.1038/s41586-019-1563-y
Verbruggen, L., Sprimont, L., Bentea, E., Janssen, P., Gharib, A., Deneyer, L., et al. (2021). Chronic Sulfasalazine Treatment in Mice Induces System xc- - Independent Adverse Effects. Front. Pharmacol. 12:1127. doi: 10.3389/fphar.2021.625699
Virgilio, F. D. (2012). Purines, Purinergic Receptors, and Cancer. Cancer Res. 72, 5441–5447. doi: 10.1158/0008-5472.CAN-12-1600
Vultaggio-Poma, V., Sarti, A. C., and Di Virgilio, F. (2020). Extracellular ATP: a Feasible Target for Cancer Therapy. Cells 9:2496. doi: 10.3390/cells9112496
Wang, D. D., Kriegstein, A. R., and Ben-Ari, Y. (2008). GABA Regulates Stem Cell Proliferation before Nervous System Formation. Epilepsy Curr. 8, 137–139. doi: 10.1111/j.1535-7511.2008.00270.x
Watanabe, M., Maemura, K., Kanbara, K., Tamayama, T., and Hayasaki, H. (2002). GABA and GABA receptors in the central nervous system and other organs. Int. Rev. Cytol. 213, 1–47. doi: 10.1016/s0074-7696(02)13011-7
Xiao, B., Kuang, Z., Zhang, W., Hang, J., Chen, L., Lei, T., et al. (2019). Glutamate Ionotropic Receptor Kainate Type Subunit 3 (GRIK3) promotes epithelial-mesenchymal transition in breast cancer cells by regulating SPDEF/CDH1 signaling. Mol. Carcinog. 58, 1314–1323. doi: 10.1002/mc.23014
Yang, S., Liu, F., Wang, Q. J., Rosenberg, S. A., and Morgan, R. A. (2011). The Shedding of CD62L (L-Selectin) Regulates the Acquisition of Lytic Activity in Human Tumor Reactive T Lymphocytes. PLoS One 6:e22560. doi: 10.1371/journal.pone.0022560
Yi, H., Talmon, G., and Wang, J. (2020). Glutamate in cancers: from metabolism to signaling. J. Biomed. Res. 34, 260–270. doi: 10.7555/JBR.34.20190037
Young, S. Z., and Bordey, A. (2009). GABA’s Control of Stem and Cancer Cell Proliferation in Adult Neural and Peripheral Niches. Physiology 24, 171–185. doi: 10.1152/physiol.00002.2009
Zhang, Y., Li, F., Wang, L., and Lou, Y. (2021). A438079 affects colorectal cancer cell proliferation, migration, apoptosis, and pyroptosis by inhibiting the P2X7 receptor. Biochem. Biophys. Res. Commun. 558, 147–153. doi: 10.1016/j.bbrc.2021.04.076
Zhao, Y., Chen, X., Lyu, S., Ding, Z., Wu, Y., Gao, Y., et al. (2021). Identification of novel P2X7R antagonists by using structure-based virtual screening and cell-based assays. Chem. Biol. Drug Des. 98, 192–205. doi: 10.1111/cbdd.13867
Zhong, Y., Dunn, P. M., Xiang, Z., Bo, X., and Burnstock, G. (1998). Pharmacological and molecular characterization of P2X receptors in rat pelvic ganglion neurons. Br. J. Pharmacol. 125, 771–781. doi: 10.1038/sj.bjp.0702118
Keywords: cancer, genetics, ion channels, membrane protein transport, GABA, ligand receptor
Citation: Rao R, Shah S, Bhattacharya D, Toukam DK, Cáceres R, Pomeranz Krummel DA and Sengupta S (2022) Ligand-Gated Ion Channels as Targets for Treatment and Management of Cancers. Front. Physiol. 13:839437. doi: 10.3389/fphys.2022.839437
Received: 20 December 2021; Accepted: 07 February 2022;
Published: 08 March 2022.
Edited by:
John Thomas Weber, Memorial University of Newfoundland, CanadaReviewed by:
Luis A. Pardo, Max Planck Institute for Experimental Medicine, GermanyCopyright © 2022 Rao, Shah, Bhattacharya, Toukam, Cáceres, Pomeranz Krummel and Sengupta. This is an open-access article distributed under the terms of the Creative Commons Attribution License (CC BY). The use, distribution or reproduction in other forums is permitted, provided the original author(s) and the copyright owner(s) are credited and that the original publication in this journal is cited, in accordance with accepted academic practice. No use, distribution or reproduction is permitted which does not comply with these terms.
*Correspondence: Daniel A. Pomeranz Krummel, a3J1bW1lZGxAdWNtYWlsLnVjLmVkdQ==; Soma Sengupta, c2VuZ3Vwc21AdWNtYWlsLnVjLmVkdQ==
Disclaimer: All claims expressed in this article are solely those of the authors and do not necessarily represent those of their affiliated organizations, or those of the publisher, the editors and the reviewers. Any product that may be evaluated in this article or claim that may be made by its manufacturer is not guaranteed or endorsed by the publisher.
Research integrity at Frontiers
Learn more about the work of our research integrity team to safeguard the quality of each article we publish.