- 1Department of Anesthesiology and Intensive Care Medicine, Pulmonary Engineering Group, University Hospital Carl Gustav Carus Dresden at Technische Universität Dresden, Dresden, Germany
- 2Department of Anesthesiology and Intensive Care Medicine, Clinical Sensoring and Monitoring Group, University Hospital Carl Gustav Carus Dresden at Technische Universität Dresden, Dresden, Germany
- 3Department of Intensive Care and Resuscitation, Anesthesiology Institute, Cleveland Clinic, Cleveland, OH, United States
- 4Department of Outcomes Research, Anesthesiology Institute, Cleveland Clinic, Cleveland, OH, United States
Background: Mechanical ventilation (MV) inflicts stress on the lungs, initiating or increasing lung inflammation, so-called ventilator-induced lung injury (VILI). Besides overdistention, cyclic opening-and-closing of alveoli (atelectrauma) is recognized as a potential mechanism of VILI. The dynamic stretch may be reduced by positive end-expiratory pressure (PEEP), which in turn increases the static stretch. We investigated whether static stretch modulates the inflammatory response of rat type 2 alveolar epithelial cells (AECs) at different levels of dynamic stretch and hypothesized that static stretch increases pro-inflammatory response of AECs at given dynamic stretch.
Methods: AECs, stimulated and not stimulated with lipopolysaccharide (LPS), were subjected to combinations of static (10, 20, and 30%) and dynamic stretch (15, 20, and 30%), for 1 and 4 h. Non-stretched AECs served as control. The gene expression and secreted protein levels of interleukin-6 (IL-6), monocyte chemoattractant protein-1 (MCP-1), and macrophage inflammatory protein 2 (MIP-2) were studied by real-time polymerase chain reaction (RT-qPCR) and enzyme-linked immunosorbent assay (ELISA), respectively. The effects of static and dynamic stretch were assessed by two-factorial ANOVA with planned effects post-hoc comparison according to Šidák. Statistical significance was considered for p < 0.05.
Results: In LPS-stimulated, but not in non-stimulated rat type 2 AECs, compared to non-stretched cells: 1) dynamic stretch increased the expression of amphiregulin (AREG) (p < 0.05), MCP-1 (p < 0.001), and MIP-2 (<0.05), respectively, as well as the protein secretion of IL-6 (p < 0.001) and MCP-1 (p < 0.05); 2) static stretch increased the gene expression of MCP-1 (p < 0.001) and MIP-2, but not AREG, and resulted in higher secretion of IL-6 (p < 0.001), but not MCP-1, while MIP-2 was not detectable in the medium.
Conclusion: In rat type 2 AECs stimulated with LPS, static stretch increased the pro-inflammatory response to dynamic stretch, suggesting a potential pro-inflammatory effect of PEEP during mechanical ventilation at the cellular level.
Introduction
Mechanical ventilation (MV) is a lifesaving therapy in respiratory failure. During conventional MV, positive pressure is used to overcome the resistive and elastic properties of the respiratory system, leading to stretch, and strain of lung units. Stress and strain are crucial to keep the homeostasis of the lungs (Hubmayr and Kallet, 2018). Stretching of alveolar epithelial cells (AECs), also during MV, is a trigger of surfactant turnover and release (Abreu et al., 2008). However, MV may also cause injury, amplifying the lung-specific inflammatory response in both healthy and injured lungs, with release of cytokines, and damage to the alveolar integrity (Xuan et al., 2015; Rentzsch et al., 2017).
Lung injury due to MV, so-called ventilator-induced lung injury (VILI), has different pathophysiological mechanisms (Loza et al., 2015; Pelosi et al., 2018; Abreu et al., 2019). These include tidal overdistension, or volutrauma, and cyclic opening and closing of atelectatic lung units, termed atelectrauma (Rocco et al., 2012). Excessive cell stretching increases the release of surfactant proteins (Bartolák-Suki et al., 2017), production of inflammatory cytokines, and other pro-inflammatory molecules through different pathways by modulation of gene expression (Santos and Slutsky, 2000). Thereby, such events also cause numerous cellular and biochemical events in the pathogenesis of acute respiratory distress syndrome (ARDS) (Sipahi, 2014).
Mechanisms of mechano-transduction constitute the basis of the epiphenomenon generally referred to as biotrauma. In clinical practice, positive end expiratory pressure (PEEP) is used in an attempt to protect lungs from atelectrauma (Dreyfuss et al., 1988; Vlahakis et al., 1999; Huhle et al., 2018). However, PEEP strategies have yielded conflicting results regarding lung protection (Spieth et al., 2009; Bugedo et al., 2017). While the effect of PEEP on lung macrostructure is better defined, i.e., the stabilization of lung units and a more even distribution of mechanical stress (Rentzsch et al., 2017), little is known about how static stretch, resulting from use of PEEP, impacts the inflammatory response of AECs under dynamic stretching. Studies revealed that, in cultured AECs, non-variable cyclic stretch, in contrast to non-stretched resting conditions, induces the production of cytokines (Vlahakis et al., 1999; Li et al., 2003), reactive oxygen species (Chapman et al., 2005) and promotes cytoskeleton remodeling (DiPaolo et al., 2010), rendering these cells a valuable model for studying these aspects in vitro. Therefore, in the present study, we aimed to investigate the inflammatory response of rat type 2 AECs cell line to different conditions of stretching. We hypothesized that static stretch increases the pro-inflammatory response of AECs to dynamic stretch.
Materials and Methods
The detailed methodology of the experiments is described in the Supplementary Figure S1.
Cell Culture Stretch and Pre-Stretch
AECs L2 cell line CCL-149™ from rats (ATCC, Wesel, Germany), were grown on BioFlex six-well plates (Flexcell International Corporation, Hillsborough, United States) at a density of 1.3 × 105 cells/well in DMEM (Biochrom, Berlin, Germany) containing 10% fetal bovine serum (FBS, Thermo Fisher Scientific, Bremen, Germany) and 50 μg/ml gentamycin sulfate (Biochrom, Switzerland). Cells were incubated in this medium at 37°C and 6.5% CO2 for a period of 24 h. After, 16–20 h before stretch experiments, cells were washed twice with sterile phosphate buffered saline (PBS) and incubated with DMEM containing 1% FBS, 50 µg gentamycin sulfate/ml, 4 mM L-alanyl-L-glutamine, and 10EU/mL FBS supplementation. Before each stretch experiment, cells were preincubated 1 h with 2 μg/ml LPS (Escherichia coli O111:B4, SIGMA-Aldrich, St. Louis, United States). Plates were stretched by 10, 20, or 30% static and 15, 20, and 30% dynamic stretch for 1 and 4 h. Stretching frequency was 0.5 Hz with a stretching/relaxation ratio 1:1 (sinusoidal pattern).
Stretching Device
The custom designed and made stretching device, three cylindrical intenders were used to apply a homogeneous stretch on three membranes of a BioFlex Culture six-well plate (Flexcell International Corporation, Hillsborough, United States), while three served as non-stretched controls. A stepper motor (Maxon Motor AG, Sachsen, Switzerland) performed a vertical motion of the intender on the plate. A custom program, (LabView, National Instruments, Austix, TX, United States), was used to control the driving stepper motor and allowed the adjustment of the stretching parameters, time, frequency, the stretching amplitude (Figures 1A–B).
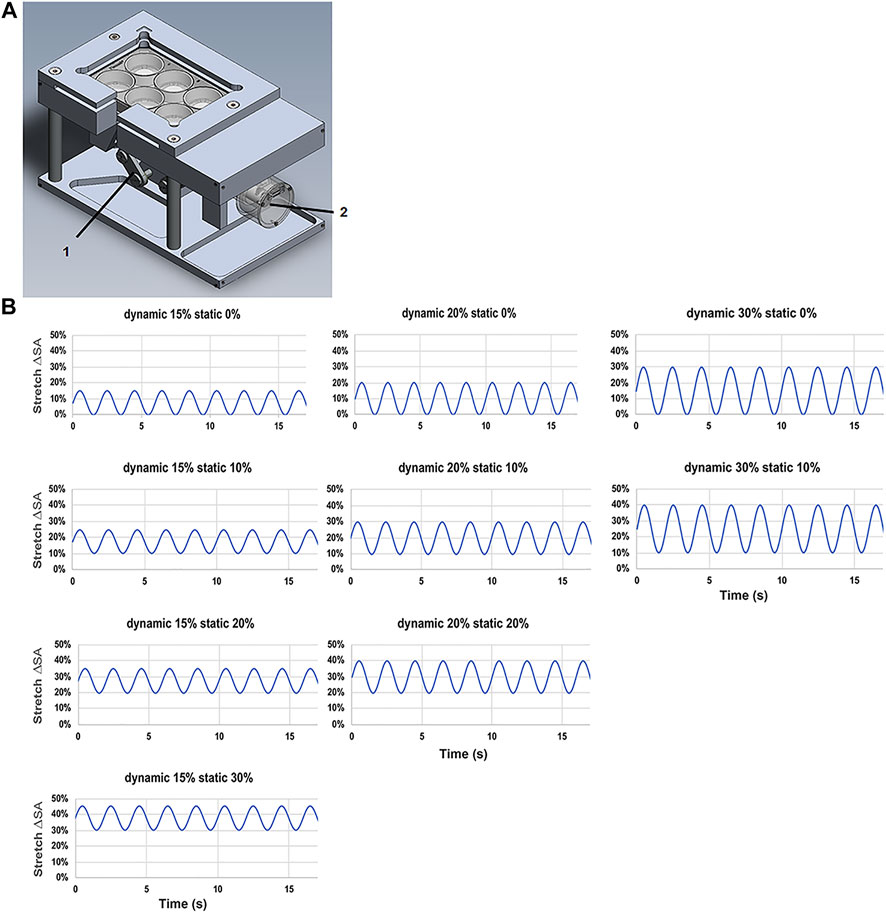
FIGURE 1. Stretching device for L2 alveolar epithelial cells. (A) Stretch chamber device used in the experiments with (1) brushless motor driver and (2) vertical cylindrical indenter. (B) Patterns of the tidal stretch ΔSA (estimated change in cell surface area) with the time (s) of L2 AECs performed on the flexible silicone elastomer membrane with a non-variable static stretch patterns of 0, 10, 20, and 30% with dynamic stretch of 15, 20, and 30%.
Cell Viability Assay
Cell viability assay was performed using the Cytotoxicity Detection Kit Plus (Roche, Mannheim, Germany). Cell viability was assessed by measuring lactate dehydrogenase (LDH) activity in the culture supernatant.
RNA Extraction
Total RNA was extracted using the peqGOLD Total RNA Kit (VWR, Dresden, Germany) and reverse transcribed with the qScript cDNA SuperMix (Beverly, United States). In both cases, we followed the manufacturer’s instructions.
Gene Expression Analyses
The gene expression of amphiregulin (AREG), interleukin-6 (IL-6), chemokine (C-X-C) motif ligand 2, known as monocyte chemoattractant protein-1 (MCP-1), chemokine (C-C motif) ligand 2, known as macrophage inflammatory protein 2 (MIP-2) were detected by quantitative polymerase chain reaction (qPCR). The house-keeping genes, glyceraldehyde-3-phosphate dehydrogenase (GAPDH) and hypoxanthine-guanine phosphoribosyltransferase (HPRT) were used as controls. For Real Time PCR, PerfeCTa SYBR Green FastMix (Quanta Biosciences Inc., Gaithersburg, United States) was used. cDNA products were analyzed by semiquantitative RT-PCR using the delta delta threshold cycles CT (∆∆CT) method (Rao et al., 2013). The primers (Eurofins Genomics, Ebersberg, Germany) used are listed in Table 1. The chosen primer pairs were double-checked in the primer blast tool, to ensure specificity for the target gene.
qRT-PCRs were run on the PCR MyiQ™ 2 Cycler (Biorad, Kabelsketal, Germany), using the IQ 5 software (version: 2.1.97.1001). qRT-PCR was performed in triplicate cDNA samples under the following conditions: 95°C for 30 s, followed by 45 cycles at 95°C for 5 s, 58°C for 15 s, and extension at 68°C for 10 min.
Cytokine and Chemokine Determination
Supernatant was centrifuged at 1,000 g for 5 min to remove the cells debris. Protein secretion of IL-6, MCP-1, and MIP-2 were detected using available commercial Enzyme-linked Immunosorbent Assay ELISA kits (Thermo Scientific; Invitrogen, Darmstadt, Germany).
Immunostaining and Microscopy
First, L2 AECs were washed with phosphate buffered saline (PBS), fixed with 4% (w/v) paraformaldehyde in PBS (15 min at room temperature/RT), and washed with PBS (2 × 3 min). Afterwards, cells were permeabilized with ice-cold 0.1% (v/v) Triton X-100 in PBS (5 min, RT), washed with PBS (2 × 3 min), and then blocked with 3% (w/v) bovine serum albumin source in PBS (20–30 min, RT). Then, cells were incubated with Phalloidin-Alexa 488 (1:500) (Molecular Probes, Invitrogen Corporation, Waltham, Massachusetts, United States), which labels filamentous actin, or with a primary antibody against Zonula Occludens Protein (ZO-2) (1:300) (#Sc-8148 Santa Cruz Biotechnology Inc., Dallas, United States), or anti-Surfactant Protein C (1:200) (#AB3786 Millipore Corporation, United States) a specific L2AECs marker, 1 h at RT, washed with PBS (2 × 3 min), and labelled with the secondary antibody Alexa-Fluor-488 goat anti-rabbit (1:500) (Thermo Fischer Scientific, Waltham, Massachusetts, United States) and DAPI (1:200) (Sigma-Aldrich Chemie GmbH, Munich, Germany), during 30 min at room temperature. Subsequently, cells were washed with PBS (2 times × 3 min) and mounted on microscope slides using MOWIOL (Calbiochem/Merck, Darmstadt, Germany). L2 AECs were imaged using an Olympus SD-OSR Spinning Disc Confocal Microscope equipped with a 60x numerical aperture N.A. = 1.4 oil DIC objective (Carl Zeiss Microimaging, Jena, Germany) at the Imaging Facility “Medizinisch -Theoretisches Zentrum” at Technical Dresden University, Z-stacks were acquired with 1 µm or 30 µm intervals between consecutive focal planes. Images were analyzed, processed, and quantified with ImageJ (Rasband and ImageJ, 2021), and Acrobat Photoshop® 2022 (Adobe, United States). Actin filament orientation was analyzed using the OrientationJ Plugin (Rezakhaniha et al., 2012) and their intensity and length, as well as tight junction intensity, were assessed using FiloQuant (Jacquemet et al., 2017; Jacquemet et al., 2019). The 3D renditions were obtained from 1 µm Z-sections using the ImageJ 3D Viewer plugin (volume view) (Rasband and W.S.; Schneider et al., 2012; Schindelin et al., 2015).
Statistical Analysis
Data is given as mean ± standard deviation (SD), unless otherwise indicated. Comparisons among groups were conducted with three-way general linear model ANOVA for factors condition (LPS, Stretch, LPS + Stretch), static (0, 10, 20, and 30%) and dynamic stretch (15, 20, 30%). Main effects for each model were assessed as marginal means (Dessau and Pipper, 2008; Lenth, 2016) with p-value adjustment according to Šidák for planned comparisons: for each dynamic stretch between available static stretches; and for each combination of static and dynamic stretch levels between LPS + non-Stretch and LPS + Stretch. Cell survival was tested using logistic binomial GLM with factors group (Control, Stretch, LPS or LPS + Stretch), time, dynamic and static stretch. All statistics were performed using R Statistical Programming Language (R Core Team, 2021). Statistical significance was accepted at p < 0.05.
Results
Cell Typification
The lineage of type 2 AECs was confirmed by immunostaining with an antibody against Surfactant Protein C (SP-C), a specific marker for these cells (Supplementary Figure S2).
In vitro Stretch, Tight Junctions, and Cell Viability
As shown in Supplementary Figures S3–S5, and Supplementary Figure S8 the static and dynamic stretch did not affect the organization or intensity of ZO-2-positive intercellular tight junctions in any of the studied experimental conditions. Cytoplasmic actin filaments were intact at a dynamic stretch of 15% (Supplementary Figure S6) or 20% (Supplementary Figure S7). In contrast, a significant reduction in actin filament length, an increase in their intensity, and a change in their orientation, i.e., more actin bundles (Figure 2C, D, G, H), compared to the parallel filaments observed in control cells (Figures 2A, B, E, F), were seen at a dynamic stretch of 30% (Supplementary Figures S9–S11).
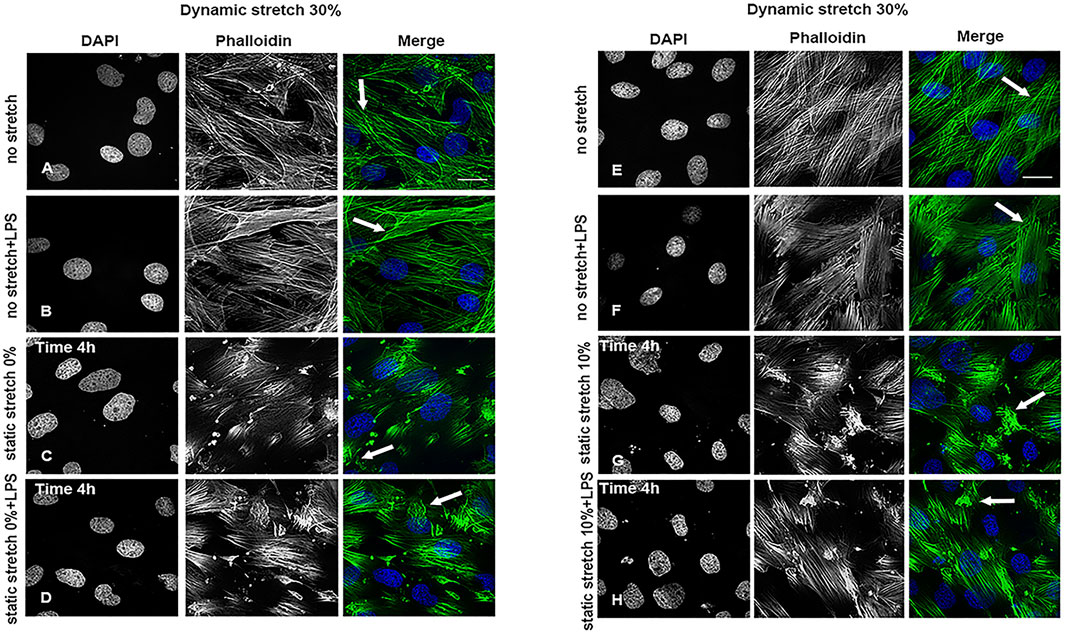
FIGURE 2. Cytoskeleton organization in L2 AECs undergoing stretch, with and without LPS for 4 h. Arrows show changes in actin filament organization in cells undergoing a 30% dynamic stretch and (A,E) non-stretch, (B,F) non-stretch + LPS, (C) 0% static stretch, (D) 0% static stretch + LPS, (G) 10% static stretch, or (H) 10% static stretch + LPS. Cells were fixed and analysed by confocal microscopy. Single channels are displayed in grey scale for DAPI (DNA) and phalloidin (actin filaments); Merge: phalloidin (green) and DAPI (blue). Data are displayed as projections of 1 μm Z-sections (n = 3). Scale bars: 30 μm.
The cell survival of L2 AECs submitted to 1 h or 4 h of static and dynamic stretch was not affected by any of the respective conditions (Supplementary Figures S12A–C).
Gene Expression of Cell Mechanical Stress and Pro-Inflammatory Cytokines
The expression of AREG was significantly increased in LPS-stimulated cells under stretch (p < 0.05), when compared with non-stimulated L2 AECs (Figure 3A, Figure 4A). AREG expression was lower at 4 h than at 1 h in LPS-treated cells, in all studied conditions. Dynamic, but not static, stretch increased AREG expression, irrespective of the duration of experiments or LPS stimulation. The expressions of IL-6 and MCP-1, but not MIP-2, were higher in LPS stimulated cells under different combinations of static and dynamic stretch, compared to non-stimulated, cells (Figures 3B–D; Figures 4B–D). Stretch duration did not affect the expression of IL-6 or MCP-1, but the expression of MIP-2 was higher at 4 h (p < 0.05) compared with 1 h. All data points were normalized to non-stretched, non-LPS treated cells.
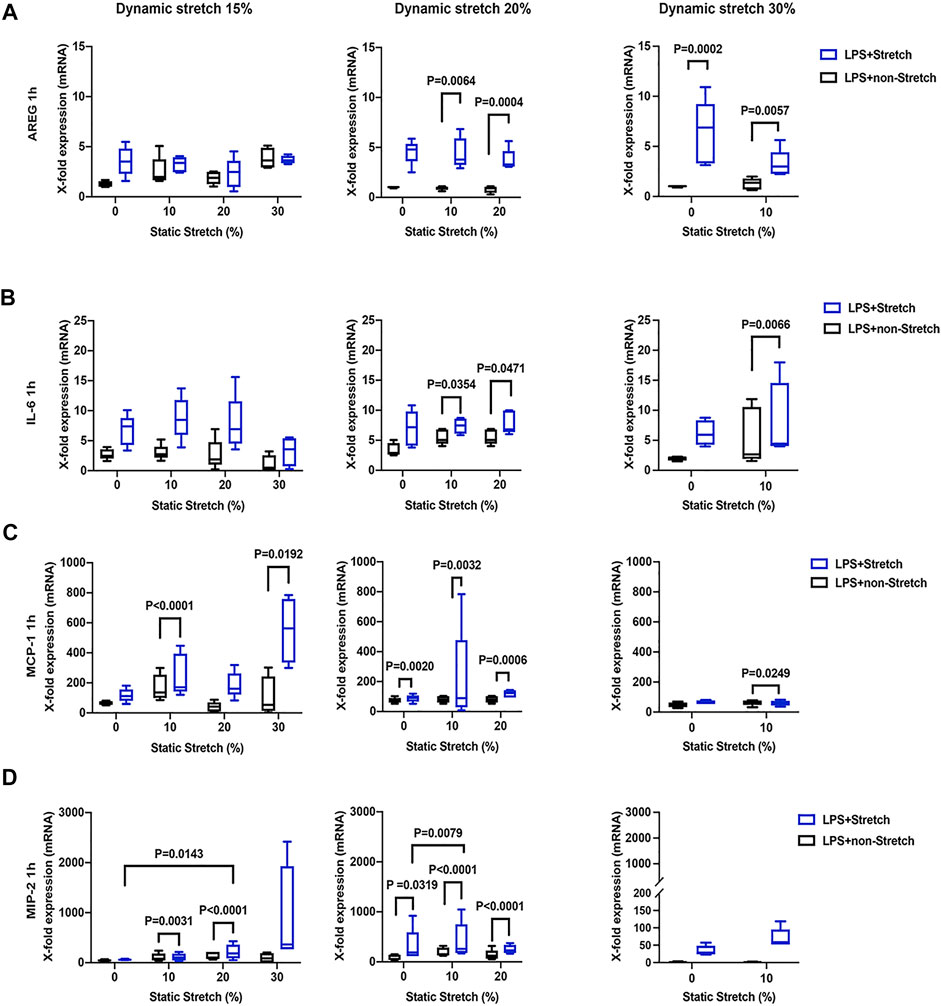
FIGURE 3. Gene expression of AREG, IL-6, MCP-1, and MIP-2 in L2 alveolar epithelial cells treated with LPS and undergoing stretch for 1 h. (A) Amphiregulin (AREG), (B) Interleukin-6 (IL-6), (C) monocyte chemoattractant protein-1 (MCP-1), and (D) macrophage inflammatory protein 2 (MIP-2). mRNA was isolated from cells treated as indicated and analyzed by real-time polymerase chain reaction (RT-qPCR). Data represents the X-fold expression of mRNA compared to control non-treated cells (n = 4). Results were normalized to control non-stretch, non-stimulated cells. Differences were considered statistically significant at p < 0.05.
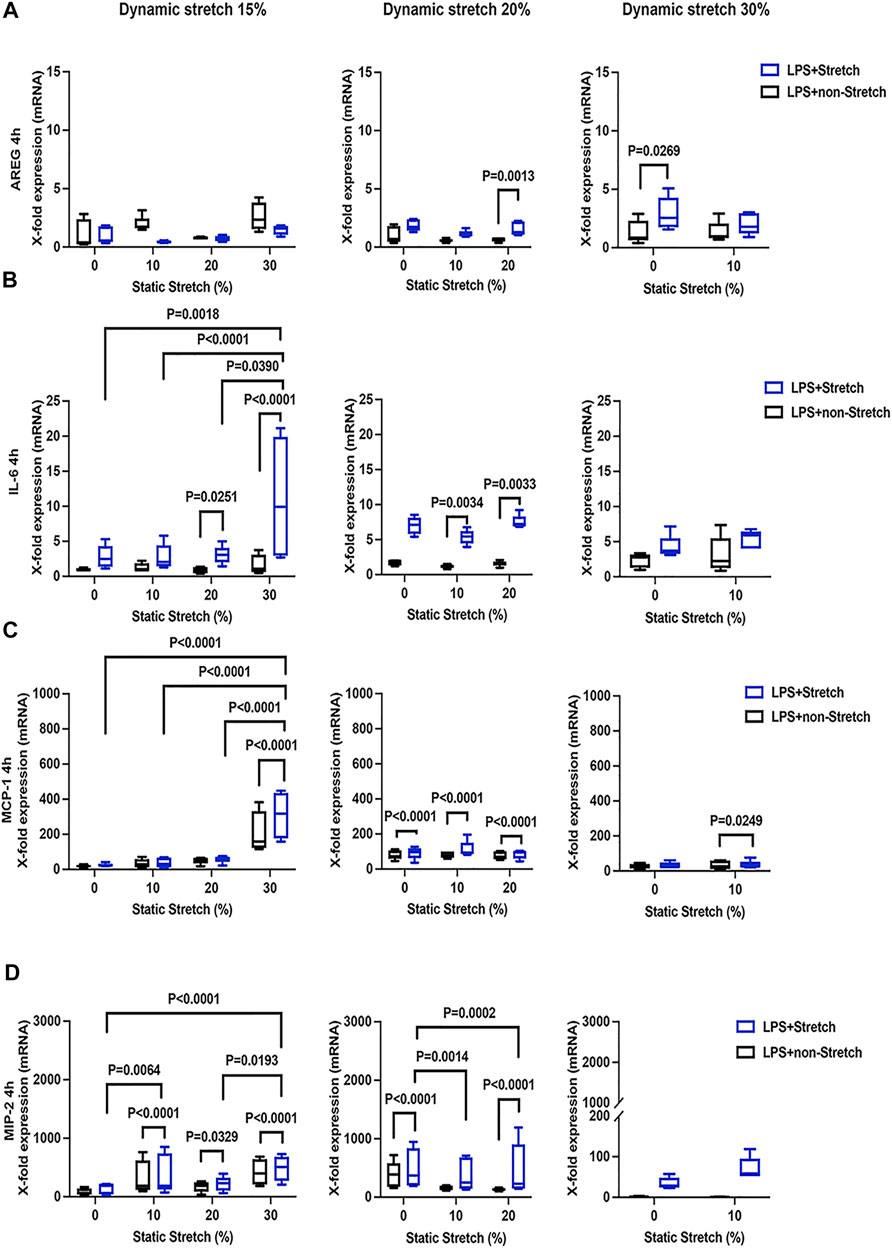
FIGURE 4. Gene expression of AREG, IL-6, MCP-1, and MIP-2 in L2 alveolar epithelial cells treated with LPS and undergoing stretch for 4 h. (A) Amphiregulin (AREG), (B) Interleukin-6 (IL-6), (C) monocyte chemoattractant protein-1 (MCP-1), and (D) macrophage inflammatory protein 2 (MIP-2) mRNA was isolated from cells treated as indicated and analyzed by real-time polymerase chain reaction (RT-qPCR). Data represents the X-fold expression of mRNA compared to control non-treated cells (n = 4). Results were normalized to control non-stretch, non-stimulated cells. Differences were considered statistically significant at p < 0.05.
Protein Concentrations of Pro-Inflammatory Cytokines in the Medium
In LPS-stimulated cells, the release of the pro-inflammatory cytokines IL-6 and MCP-1 was generally higher under different combinations of static and dynamic stretch, compared to non-stretch L2 AECs (p < 0.05) (Figures 5, 6). The secreted protein concentrations of IL-6 and MCP-1 were higher at 4 h than at 1 h (p < 0.001). In contrast to IL-6 release, which was increased by both static and dynamic stretch (p < 0.05), MCP-1 secretion was only increased by dynamic (p < 0.05), but not by static stretch. MIP-2 protein levels were below the detection level in the analysed medium (Supplementary Figure S13).
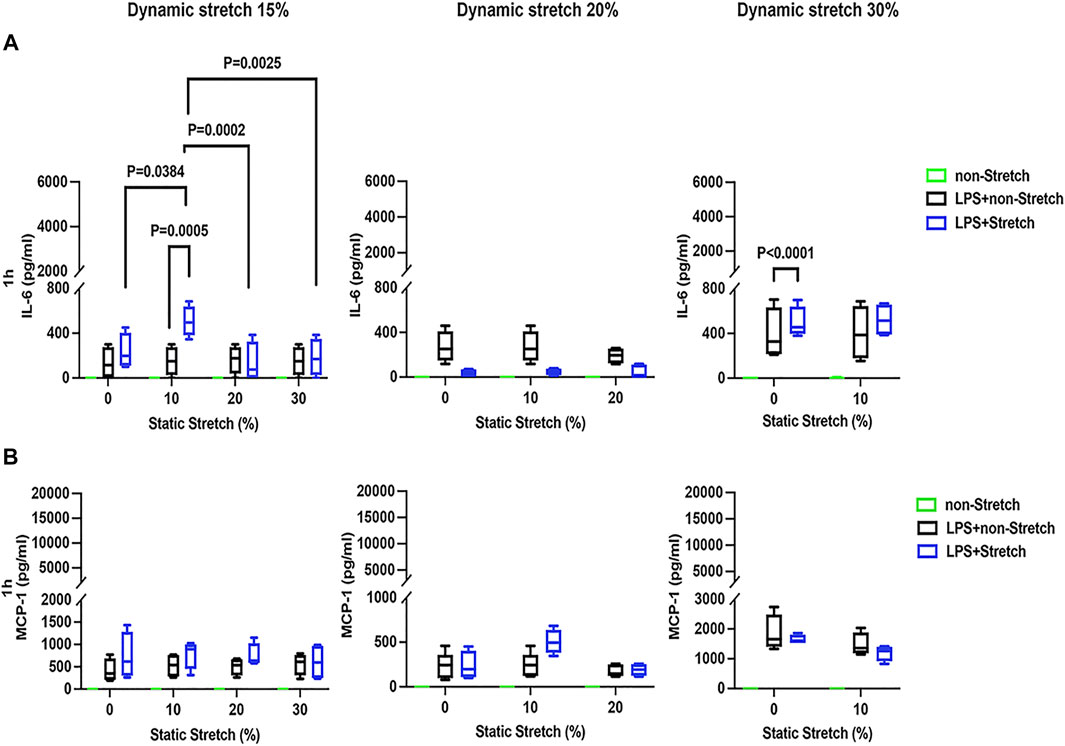
FIGURE 5. IL-6 and MCP-1 secretion in L2 alveolar epithelial cells treated with LPS and undergoing stretch for 1 h. Cells were treated, stretched with different static, and dynamic conditions 1 h, and then culture supernatants were collected: (A) Interleukin-6 (IL-6) and (B) monocyte chemoattractant protein-1 (MCP-1) protein levels were evaluated using Enzyme-linked Immunosorbent Assay (n = 4). Differences were considered statistically significant at p < 0.05.
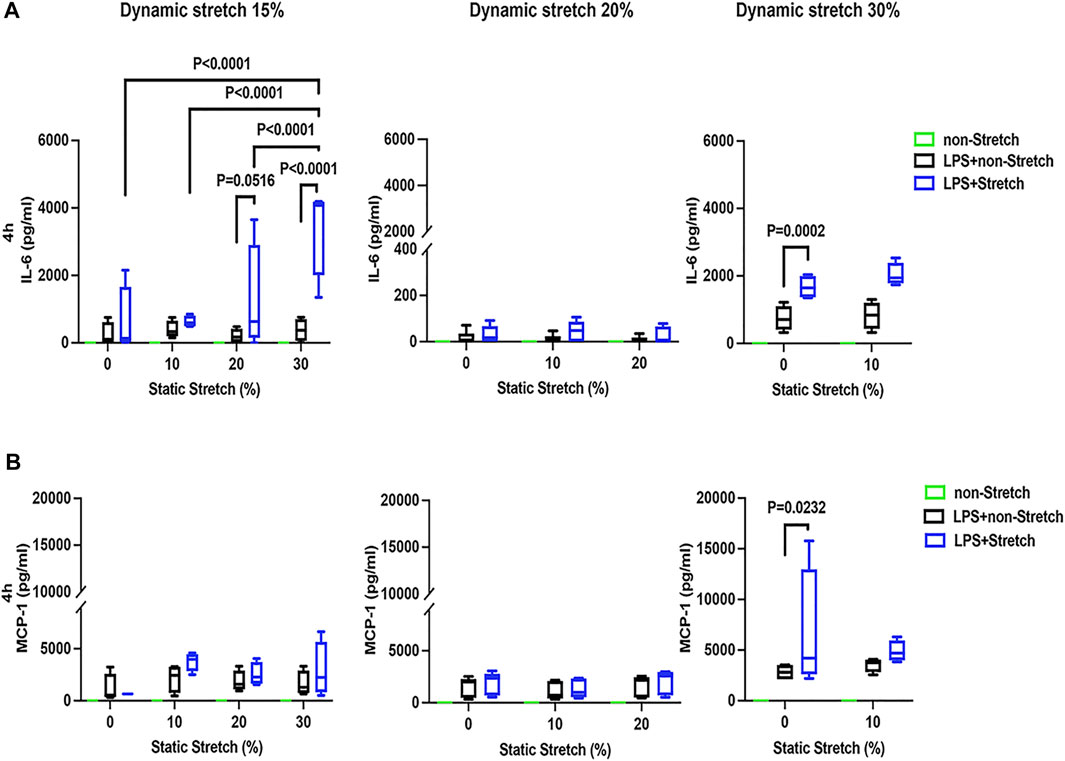
FIGURE 6. IL-6 and MCP-1 secretion in L2 alveolar epithelial cells treated with LPS and undergoing stretch for 4 h. Cells were treated, stretched with different static and dynamic conditions 4 h, then culture supernatants were collected: (A) Interleukin-6 (IL-6) and (B) monocyte chemoattractant protein-1 (MCP-1) protein levels were evaluated using Enzyme-linked Immunosorbent Assay (n = 4). Differences were considered statistically significant at p < 0.05.
Discussion
The main findings of this study were that, in LPS-stimulated, compared to non-stimulated rat type 2 AECs: 1) dynamic stretch increased the expression of AREG, IL-6, MCP-1, MIP-2, as well as the protein concentration of IL-6 and MCP-1 in the medium; 2) static stretch increased gene expression of MCP-1 and MIP-2, but not AREG, and resulted in higher protein secretion of IL-6, but not MCP-1. To our knowledge, this is the first study addressing the effects of static stretch on the pro-inflammatory response of in vitro L2 AECs submitted to dynamic stretch.
Our experiments combining static stretch with different dynamic stretch conditions were conducted in type L2 AECs, because they can easily grow under controlled conditions, and are the main cell type involved in the immune response of the lung alveolar epithelium (Kang and Kim, 2017). A major strength of our study is that neither dynamic nor static stretch, nor the LPS used for stimulation, generated any significant changes in cell survival, thus allowing us to directly assess the pro-inflammatory response. Importantly, static and dynamic stretch were designed to mimic the mechanical stress patterns that AECs are submitted to in vivo, during MV, since there is a considerable body of evidence that mechano-transduction plays a key role in VILI (Chen et al., 2018). Changes in alveolar epithelial cell deformation during modifications of lung volume have been described in vivo (Bartolák-Suki et al., 2017). Studies in isolated lungs suggest that, if the lung volume increases by 40–100% of the total lung capacity, the basal surface area of alveolar epithelial cells increases by 34–35% (Tschumperlin and Margulies, 1999; Tschumperlin et al., 2000; Wirtz and Dobbs, 2000).
On the other hand, if a single alveolus or even the whole lung is modelled as a sphere, it can be shown that an increase of alveolar surface area (SA) by factor a, yields an increase of corresponding volume V by a3/2. Therefore, during spontaneous breathing in humans, at a functional residual capacity (FRC) of 30 ml/kg body weight, a tidal volume of 7 ml/kg body weight results in an increase of lung volume of 23% and an increase of ∼15% of ASA. To increase lung volume from FRC to lung total capacity (TLC) (80 ml/kg body weight) would correspond to a 166% volume increase and a 92% increase of SA. In rats, however during anesthesia a FRC of 11 ml/kg has been reported (Schulz and Muhle, 2000). Ventilation with a tidal volume of 6 ml/kg body weight results in a 54% increase of LV and a 30% increase of SA. Accordingly, an increase of lung volume from FRC to TLC (42 ml/kg body weight in rats) yields a volume increase of 270% and an increase of SA of 140%. Thus, in our experiments a maximal total increase of SA of 45% mirrored dynamic and static mechanical strain occurring during low to low tidal volume ventilation in rats at low PEEP.
Mechanical ventilation may induce an inflammatory response in the lung tissue (Santos and Slutsky, 2000), leading to ARDS (Birukova et al., 2012; Sipahi, 2014). In ARDS patients, high levels of PEEP are used to reduce VILI (Briel et al., 2010). Mechanical ventilation with low tidal volumes and higher PEEP reduced the incidence of pulmonary complications in patients without acute lung injury (Neto et al., 2012). In contrast, other clinical studies have indicated that the level of PEEP is unrelated to the mortality of the ARDS patients (Walkey et al., 2017; Group et al., 2020). Recent data have also shown that higher PEEP promoted higher lung inflammation than lower PEEP, at comparable low tidal volumes and driving pressures (Ding et al., 2013; Ochiai, 2015; Hamlington et al., 2016; Silva et al., 2016). For this reason, we have asked if static stretch (which in vivo is increased by PEEP) modulates the inflammatory response. To mimic in AECs the pathophysiology of clinical ARDS (Costa et al., 2017), we used LPS, frequently utilized to induce inflammation in vitro (Dreyfuss et al., 1988; McRitchie et al., 2000; Birukova et al., 2012; Wong and Johnson, 2013) or ARDS, in rats, in vivo (Pugin et al., 1998; Ding et al., 2013).
We found that, neither dynamic stretch, static stretch, nor LPS significantly affected cell junctions and cell monolayer organization, as previously shown (Rentzsch et al., 2017; Knudsen and Ochs, 2018). Actin filament organization was not modified at lower dynamic stretch (Rentzsch et al., 2017; Knudsen and Ochs, 2018) but only at a 30% dynamic stretch, which corresponds to a high increase in total lung capacity. Previous studies have shown that MV modulates the lung inflammatory response, either through direct cell rupture or through the so-called “mechano-transduction” mechanism, which is not yet fully understood (Santos and Slutsky, 2000; Sutherasan et al., 2014; Tian et al., 2016). Recent data (Tian et al., 2016) suggest that long term (28–72 h) stretching or LPS exposure of cultured human pulmonary artery endothelial cells affect cell junctions and disrupted cell monolayers, a process mediated by RhoA kinase (Marshall et al., 2010). This is in agreement with the changes in actin filament organization we observed at a high dynamic stretch, changes that could be mediated by Rho GTPase (i.e., RhoA) signaling, and could involve crosstalk with extracellular matrix–dependent integrin signaling via SRC (Tehrani et al., 2007). Future studies are necessary to clarify these aspects.
AREG, a protein with tissue protective effects during inflammation (Zaiss et al., 2013), and is involved in the response to mechanical loading in various mesodermal-derived tissues (Deacon and Knox, 2010; Marshall et al., 2010; Shao and Sheng, 2010). We found that AREG expression was increased in LPS-stimulated cells submitted to different dynamic stretch conditions, at 1 h. However, further stimulation (4 h) reduced AREG expression, suggesting that its tissue protective effects may be reduced after long-term stretching. This decrease in AREG expression may be due to reduced transcription, and/or increased mRNA degradation (Nakayama et al., 2013), controlled by cell signaling pathways, as previously observed in other systems (Zhang et al., 2009; Terry et al., 2010; Keren, 2011; Latasa et al., 2012; Zaiss et al., 2013).
The focus of our study was to understand if and how PEEP modulated the inflammatory response (Rentzsch et al., 2017; Knudsen and Ochs, 2018). We have found that, in LPS-stimulated L2 AECs, dynamic and static stretch levels influenced both the expression and secretion of specific cytokines (i.e. IL-6, MCP-1, and MIP-2). This is in agreement with in vivo studies in mice, showing increased expression and release of IL-6 (Tremblay et al., 2002; Heise et al., 2011; Goldman et al., 2014), MCP-1 or MIP-2 (Hegeman et al., 2010; Hawwa et al., 2011; Herbert et al., 2016; Jia et al., 2016; Zhu et al., 2016) during MV. Specifically, in LPS-stimulated L2 AECs, the expression of IL-6, MCP-1, and MIP-2 was significantly increased after static stretch, compared to non-LPS treated cells. Our data are in agreement with in vivo studies showing that the expression of several cytokines (including IL-6, MCP-1, or MIP-2) was significantly enhanced after exposure to LPS (Heise et al., 2011) and that, mechanical ventilation synergistically amplified the release of IL-6, MIP-2, IL-1β, and TNF-α in rats treated with LPS (Pugin et al., 1998; Ding et al., 2013). In addition, our data shows that MCP-1 behaves differently than the other cytokines, i.e., its release from LPS-treated cells was enhanced by dynamic, but not by static stretch, suggesting that the two types of stretch may differentially influence the immune response. Moreover, these findings support previous evidence that plasma membrane tension affects the release of inflammatory cytokines, by orchestrating complex aspects of cell trafficking and motility (Keren, 2011), and modulating cell signaling (Tremblay et al., 1997; Ranieri et al., 1999).
Possible Implications of the Findings
Our findings indicate that the mechanical tension produced by static stretch of AECs, which for example results from the use of PEEP, affects the cellular signalling pathways of inflammation. Therefore, physicians should be aware that not only macro structural changes of lungs that result from PEEP, but also microstructural changes that modulate cellular stretching might play a role in VILI. This finding supports the use of lower PEEP levels in patients with mild lung injury and ventilated with a low protective tidal volume, a concept known as “permissive atelectasis” (Guldner et al., 2016; Pelosi et al., 2018).
Limitations
Our study holds several limitations. Firstly, in vitro conditions do not fully reproduce the complex environment of the lung parenchyma. However, none of the available ARDS animal models replicates the complex pathophysiological changes seen in patients, although, similarly to our model, they were essential for advancing the knowledge in the field (Spieth et al., 2009; Rentzsch et al., 2017; Braune et al., 2019). Secondly, to mimic inflammation, we have used LPS, a complex compound that may contain bacterial DNA, lipo-proteins, etc., and thus has a high cytotoxicity. However, LPS is a valuable inflammatory inducer, largely used in this type of studies. Thirdly, the induced stretch transmitted through an elastic membrane to the epithelial cells differs significantly from in-vivo situations, where the stretching force is applied through epithelial cells onto the extra-cellular matrix.
Conclusion
Static stretch increased the pro-inflammatory response of dynamically stretched LPS-stimulated type L2 AECs which is suggestive of a potential pro-inflammatory effect of PEEP during mechanical ventilation at the cellular level.
Data Availability Statement
The datasets presented in this study can be found in online repositories. The names of the repository/repositories and accession number(s) can be found in the article/Supplementary Material.
Author Contributions
JF and MGA conceived and designed research. JF and SM performed experiments. TK and MGA provided financial resources. CS and MM developed the stretch device hardware and software. JF and RH made the statistics. JF, RH, TK, and MGA interpreted and analyzed the data. JF prepared tables, graphs, and figures. TK and MGA supervised the experiments. JF, RH, and MGA drafted the manuscript. JF, RH, CS, MM, TK, and MGA edited and revised the manuscript. JF, RH, SM, CS, MM, TK, and MGA approved the final version of manuscript. All authors read and approved the submitted manuscript and agree to be accountable for the content of the work and its publication.
Funding
This work was supported by a grant of Deutsche Forschungsgemeinschaft GA 1256/8-1. This funding body supported in the collection, analysis, and interpretation of data.
Conflict of Interest
The authors declare that the research was conducted in the absence of any commercial or financial relationships that could be construed as a potential conflict of interest.
Publisher’s Note
All claims expressed in this article are solely those of the authors and do not necessarily represent those of their affiliated organizations, or those of the publisher, the editors and the reviewers. Any product that may be evaluated in this article, or claim that may be made by its manufacturer, is not guaranteed or endorsed by the publisher.
Acknowledgments
The authors thank the support of all members of the Pulmonary Engineering Group (PEG) from the Department of Anaesthesiology and Intensive Care Medicine at the University Hospital Carl Gustav Carus, Technische Universität Dresden, Dresden, Germany, for their assistance in conducting experiments.
Supplementary Material
The Supplementary Material for this article can be found online at: https://www.frontiersin.org/articles/10.3389/fphys.2022.838834/full#supplementary-material
References
Abreu G., Schultz M., Pelosi P., Groupfor the W. C. P. C. (2019). Intraoperative Ventilation Strategies to Reduce Pulmonary Complications in Obese Patients-Reply. Jama 322, 1829. doi:10.1001/jama.2019.14400
Bartolák-Suki E., Noble P. B., Jawde S. B., Pillow J. J., Suki B. (2017). Optimization of Variable Ventilation for Physiology, Immune Response and Surfactant Enhancement in Preterm Lambs. Front. Physiol. 8, 425. doi:10.3389/fphys.2017.00425
Birukova A. A., Wu T., Tian Y., Meliton A., Sarich N., Tian X., et al. (2012). Iloprost Improves Endothelial Barrier Function in Lipopolysaccharide-Induced Lung Injury. Eur. Respir. J. 41, 165–176. doi:10.1183/09031936.00148311
Braune A., Hofheinz F., Bluth T., Kiss T., Wittenstein J., Scharffenberg M., et al. (2019). Comparison of Static and Dynamic 18F-FDG PET/CT for Quantification of Pulmonary Inflammation in Acute Lung Injury. J. Nucl. Med. 60, 1629–1634. doi:10.2967/jnumed.119.226597
Briel M., Meade M., Mercat A., Brower R. G., Talmor D., Walter S. D., et al. (2010). Higher vs Lower Positive End-Expiratory Pressure in Patients with Acute Lung Injury and Acute Respiratory Distress Syndrome: Systematic Review and Meta-Analysis. Jama 303, 865–873. doi:10.1001/jama.2010.218
Bugedo G., Retamal J., Bruhn A. (2017). Does the Use of High PEEP Levels Prevent Ventilator-Induced Lung Injury? Revista Brasileira De Terapia Intensiva 29, 231–237. doi:10.5935/0103-507x.20170032
Chapman K. E., Sinclair S. E., Zhuang D., Hassid A., Desai L. P., Waters C. M. (2005). Cyclic Mechanical Strain Increases Reactive Oxygen Species Production in Pulmonary Epithelial Cells. Am. J. Physiol-lung C 289, L834–L841. doi:10.1152/ajplung.00069.2005
Chen L., Xia H.-F., Shang Y., Yao S.-L. (2018). Molecular Mechanisms of Ventilator-Induced Lung Injury. Chin. Med J-peking 131, 1225–1231. doi:10.4103/0366-6999.226840
Costa N. de. S. X., Júnior G. R., Alemanydos A. A. S., Belotti L., Zati D. H., Cavalcante M. F., et al. (2017). Early and Late Pulmonary Effects of Nebulized LPS in Mice: An Acute Lung Injury Model. Plos One 12, e0185474. doi:10.1371/journal.pone.0185474
Deacon K., Knox A. J. (2010). Endothelin-1 (ET-1) Increases the Expression of Remodeling Genes in Vascular Smooth Muscle through Linked Calcium and cAMP Pathways: Role of a Phospholipase A(2)(cPLA(2))/cyclooxygenase-2 (COX-2)/prostacyclin Receptor-dependent Autocrine Loop. J. Biol. Chem 285, 25913–25927. doi:10.1074/jbc.m110.139485
Ding N., Wang F., Xiao H., Xu L., She S. (2013). Mechanical Ventilation Enhances HMGB1 Expression in an LPS-Induced Lung Injury Model. Plos One 8, e74633. doi:10.1371/journal.pone.0074633
DiPaolo B. C., Lenormand G., Fredberg J. J., Margulies S. S. (2010). Stretch Magnitude and Frequency-dependent Actin Cytoskeleton Remodeling in Alveolar Epithelia. Am. J. Physiol-cell Ph 299, C345–C353. doi:10.1152/ajpcell.00379.2009
Dreyfuss D., Soler P., Basset G., Saumon G. (1988). High Inflation Pressure Pulmonary Edema: Respective Effects of High Airway Pressure, High Tidal Volume, and Positive End-Expiratory Pressure. Am. Rev. Respir. Dis. 137, 1159–1164. doi:10.1164/ajrccm/137.5.1159
Gama de Abreu M., Spieth P. M., Pelosi P., Carvalho A. R., Walter C., Schreiber-Ferstl A. (2008). Noisy Pressure Support Ventilation: a Pilot Study on a New Assisted Ventilation Mode in Experimental Lung Injury. Crit. Care Med. 36, 818–827. doi:10.1097/01.ccm.0000299736.55039.3a
Goldman J. L., Sammani S., Kempf C., Saadat L., Letsiou E., Wang T., et al. (2014). Pleiotropic Effects of Interleukin-6 in a “Two-hit” Murine Model of Acute Respiratory Distress Syndrome. Pulm. Circ. 4, 280–288. doi:10.1086/675991
Group W. C., Rela. S. C. C., Algera A. G., Pisani L., Neto A. S., Boer S. S., et al. (2020). Effect of a Lower vs Higher Positive End-Expiratory Pressure Strategy on Ventilator-free Days in ICU Patients without ARDS. Jama 324. doi:10.1001/jama.2020.23517
Guldner A., Braune A., Ball L., Silva P. L., Samary C., Insorsi A., et al. (2016). Comparative Effects of Volutrauma and Atelectrauma on Lung Inflammation in Experimental Acute Respiratory Distress Syndrome. Crit. Care Med. 44, e854–65. doi:10.1097/ccm.0000000000001721
Hamlington K. L., Smith B. J., Allen G. B., Bates J. H. T. (2016). Predicting Ventilator-Induced Lung Injury Using a Lung Injury Cost Function. J. Appl. Physiol. 121, 106–114. doi:10.1152/japplphysiol.00096.2016
Hawwa R. L., Hokenson M. A., Wang Y., Huang Z., Sharma S., Sanchez-Esteban J. (2011). IL-10 Inhibits Inflammatory Cytokines Released by Fetal Mouse Lung Fibroblasts Exposed to Mechanical Stretch. Pediatr. Pulm. 46, 640–649. doi:10.1002/ppul.21433
Hegeman M. A., Hennus M. P., Meurs M. van., Cobelens P. M., Kavelaars A., Jansen N. J., et al. (2010). Angiopoietin-1 Treatment Reduces Inflammation but Does Not Prevent Ventilator-Induced Lung Injury. Plos One 5, e15653. doi:10.1371/journal.pone.0015653
Heise R. L., Stober V., Cheluvaraju C., Hollingsworth J. W., Garantziotis S. (2011). Mechanical Stretch Induces Epithelial-Mesenchymal Transition in Alveolar Epithelia via Hyaluronan Activation of Innate Immunity*. J. Biol. Chem. 286, 17435–17444. doi:10.1074/jbc.m110.137273
Herbert J. A., Valentine M. S., Saravanan N., Schneck M. B., Pidaparti R., Fowler A. A., et al. (2016). Conservative Fluid Management Prevents Age-Associated Ventilator Induced Mortality. Exp. Gerontol. 81, 101–109. doi:10.1016/j.exger.2016.05.005
Hubmayr R. D., Kallet R. H. (2018). Understanding Pulmonary Stress-Strain Relationships in Severe ARDS and its Implications for Designing a Safer Approach to Setting the Ventilator. Respir. Care 63, 219–226. doi:10.4187/respcare.05900
Huhle R., Neto A. S., Schultz M. J., Abreu M. G. de. (2018). Is Mechanical Power the Final Word on Ventilator-Induced Lung Injury?-No. Ann. Transl Med. 6, 394. doi:10.21037/atm.2018.09.65
Jacquemet G., Hamidi H., Ivaska J. (2019). Filopodia Quantification Using FiloQuant. Methods Mol. Biol. Clifton N J 2040, 359–373. doi:10.1007/978-1-4939-9686-5_16
Jacquemet G., Paatero I., Carisey A. F., Padzik A., Orange J. S., Hamidi H., et al. (2017). FiloQuant Reveals Increased Filopodia Density during Breast Cancer Progression. J. Cel Biol 216, 3387–3403. doi:10.1083/jcb.201704045
Jia C.-E., Jiang D., Dai H., Xiao F., Wang C. (2016). Pendrin, an Anion Exchanger on Lung Epithelial Cells, Could Be a Novel Target for Lipopolysaccharide-Induced Acute Lung Injury Mice. Am. J. Transl Res. 8, 981–992.
Kang E. K., Kim H. S. (2017). The Effects of Hydrogen Peroxide and Lipopolysaccharide on Rat Alveolar L2 Cells. Exp. Lung Res. 43, 293–300. doi:10.1080/01902148.2017.1368738
Keren K. (2011). Membrane Tension Leads the Way. Proc. Natl. Acad Sci 108, 14379–14380. doi:10.1073/pnas.1111671108
Knudsen L., Ochs M. (2018). The Micromechanics of Lung Alveoli: Structure and Function of Surfactant and Tissue Components. Histochem. Cel Biol 150, 661–676. doi:10.1007/s00418-018-1747-9
Latasa M. U., Salis F., Urtasun R., Garcia-Irigoyen O., Elizalde M., Uriarte I., et al. (2012). Regulation of Amphiregulin Gene Expression by β-Catenin Signaling in Human Hepatocellular Carcinoma Cells: A Novel Crosstalk between FGF19 and the EGFR System. Plos One 7, e52711. doi:10.1371/journal.pone.0052711
Lenth R. V. (2016). Least-Squares Means: The R Package Lsmeans. J. Stat. Softw. 69. doi:10.18637/jss.v069.i01
Li L. F., Ouyang B., Choukroun G., Matyal R., Mascarenhas M., Jafari B., et al. (2003). Stretch-induced IL-8 Depends on C-Jun NH2-terminal and Nuclear Factor-kappaB-Inducing Kinases. Am. J. Physiol-lung C 285, L464–L475. doi:10.1152/ajplung.00031.2003
Loza R. C., Rodriguez G. V., Fernandez N. M. (2015). Ventilator-Induced Lung Injury (VILI) in Acute Respiratory Distress Syndrome (ARDS): Volutrauma and Molecular Effects. Open Respir. Med. J 9, 112–119. doi:10.2174/1874306401509010112
Marshall A. K., Barrett O. P. T., Cullingford T. E., Shanmugasundram A., Sugden P. H., Clerk A. (2010). ERK1/2 Signaling Dominates over RhoA Signaling in Regulating Early Changes in RNA Expression Induced by Endothelin-1 in Neonatal Rat Cardiomyocytes. Plos One 5, e10027. doi:10.1371/journal.pone.0010027
McRitchie D. I., Isowa N., Edelson J. D., Xavier A. M., Cai L., Man H. Y., et al. (2000). Production of Tumour Necrosis Factor Alpha by Primary Cultured Rat Alveolar Epithelial Cells. Cytokine 12, 644–654. doi:10.1006/cyto.1999.0656
Nakayama H., Fukuda S., Matsushita N., Nishida-Fukuda H., Inoue H., Shirakata Y., et al. (2013). Human Antigen R-Mediated mRNA Stabilization Is Required for Ultraviolet B-Induced Autoinduction of Amphiregulin in Keratinocytes. J. Biol. Chem. 288, 10338–10348. doi:10.1074/jbc.m112.417527
Neto A. S., Cardoso S. O., Manetta J. A., Pereira V. G., Esposito D. C., Mde O. P., et al. (2012). Association between Use of Lung-Protective Ventilation with Lower Tidal Volumes and Clinical Outcomes Among Patients without Acute Respiratory Distress Syndrome: a Meta-Analysis. Jama 308, 1651–1659. doi:10.1001/jama.2012.13730
Ochiai R. (2015). Mechanical Ventilation of Acute Respiratory Distress Syndrome. J. Intensive Care 3, 25. doi:10.1186/s40560-015-0091-6
Pelosi P., Rocco P. R. M., Abreu M. G. de. (2018). Close Down the Lungs and Keep Them Resting to Minimize Ventilator-Induced Lung Injury. Crit. Care 22, 72. doi:10.1186/s13054-018-1991-3
Pugin J., Dunn I., Jolliet P., Tassaux D., Magnenat J.-L., Nicod L. P., et al. (1998). Activation of Human Macrophages by Mechanical Ventilation In Vitro. Am. J. Physiol-lung C 275, L1040–L1050. doi:10.1152/ajplung.1998.275.6.l1040
R Core Team (2021). R: A Language and Environment for Statistical Computing. Vienna, Austria: R Foundation for Statistical Computing. Available at: https://www.R-project.org/.
Ranieri V. M., Suter P. M., Tortorella C., Tullio R. D., Dayer J. M., Brienza A., et al. (1999). Effect of Mechanical Ventilation on Inflammatory Mediators in Patients with Acute Respiratory Distress Syndrome: a Randomized Controlled Trial. Jama 282, 54–61. doi:10.1001/jama.282.1.54
Rao X., Huang X., Zhou Z., Lin X. (2013). An Improvement of the 2ˆ(-delta delta CT) Method for Quantitative Real-Time Polymerase Chain Reaction Data Analysis. Biostat Bioinform Biomathematics 3, 71–85.
Rasband A., ImageJ W. S. (2021). National Institutes of Health. Bethesda, Maryland, USA: U.S. Department of Health and Human Services.
Rentzsch I., Santos C. L., Huhle R., Ferreira J. M. C., Koch T., Schnabel C., et al. (2017). Variable Stretch Reduces the Pro-inflammatory Response of Alveolar Epithelial Cells. Plos One 12, e0182369. doi:10.1371/journal.pone.0182369
Rezakhaniha R., Agianniotis A., Schrauwen J. T. C., Griffa A., Sage D., Bouten C. V. C., et al. (2012). Experimental Investigation of Collagen Waviness and Orientation in the Arterial Adventitia Using Confocal Laser Scanning Microscopy. Biomech. Model. Mechan 11, 461–473. doi:10.1007/s10237-011-0325-z
Rocco P. R., Santos C. D., Pelosi P. (2012). Pathophysiology of Ventilator-Associated Lung Injury. Curr. Opin. Anaesthesiol 25, 123–130. doi:10.1097/aco.0b013e32834f8c7f
Santos C. C. D., Slutsky A. S. (2000). Invited Review: Mechanisms of Ventilator-Induced Lung Injury: a Perspective. J. Appl. Physiol. 89, 1645–1655. doi:10.1152/jappl.2000.89.4.1645
Schindelin J., Rueden C. T., Hiner M. C., Eliceiri K. W. (2015). The ImageJ Ecosystem: An Open Platform for Biomedical Image Analysis. Mol. Reprod. Dev. 82, 518–529. doi:10.1002/mrd.22489
Schneider C. A., Rasband W. S., Eliceiri K. W. (2012). NIH Image to ImageJ: 25 Years of Image Analysis. Nat. Methods 9, 671–675. doi:10.1038/nmeth.2089
Schulz M., Muhle R., (2000). Respiration, The Laboratory Rat eds by G. J. Krinke, (Academic Press London). London, 323–344.doi:10.1016/b978-012426400-7.50055-8
Shao J., Sheng H. (2010). Amphiregulin Promotes Intestinal Epithelial Regeneration: Roles of Intestinal Subepithelial Myofibroblasts. Endocrinology 151, 3728–3737. doi:10.1210/en.2010-0319
Silva P. L., Pelosi P., Rocco P. R. (2016). Optimal Mechanical Ventilation Strategies to Minimize Ventilator-Induced Lung Injury in Non-injured and Injured Lungs. Expert Rev. Resp Med. 10, 1243–1245. doi:10.1080/17476348.2016.1251842
Sipahi E. Y. (2014). Experimental Models of Acute Respiratory Distress Syndrome. J. Transl Intern. Med. 2, 154–159. doi:10.4103/2224-4018.147738
Spieth P. M., Carvalho A. R., Pelosi P., Hoehn C., Meissner C., Kasper M., et al. (2009). Variable Tidal Volumes Improve Lung Protective Ventilation Strategies in Experimental Lung Injury. Am. J. Resp Crit. Care 179, 684–693. doi:10.1164/rccm.200806-975oc
Sutherasan Y., Vargas M., Pelosi P. (2014). Protective Mechanical Ventilation in the Non-injured Lung: Review and Meta-Analysis. Crit. Care 18, 211. doi:10.1186/cc13778
Tehrani S., Tomasevic N., Weed S., Sakowicz R., Cooper J. A. (2007). Src Phosphorylation of Cortactin Enhances Actin Assembly. Proc. Natl. Acad Sci 104, 11933–11938. doi:10.1073/pnas.0701077104
Terry S., Nie M., Matter K., Balda M. S. (2010). Rho Signaling and Tight Junction Functions. Physiology 25, 16–26. doi:10.1152/physiol.00034.2009
Tian Y., Gawlak G., O’Donnell J. J., Mambetsariev I., Birukova A. A. (2016). Modulation of Endothelial Inflammation by Low and High Magnitude Cyclic Stretch. Plos One 11, e0153387. doi:10.1371/journal.pone.0153387
Tremblay L. N., Miatto D., Hamid Q., Govindarajan A., Slutsky A. S. (2002). Injurious Ventilation Induces Widespread Pulmonary Epithelial Expression of Tumor Necrosis Factor-Alpha and Interleukin-6 Messenger RNA. Crit. Care Med. 30, 1693–1700. doi:10.1097/00003246-200208000-00003
Tremblay L., Valenza F., Ribeiro S. P., Li J., Slutsky A. S. (1997). Injurious Ventilatory Strategies Increase Cytokines and C-Fos M-RNA Expression in an Isolated Rat Lung Model. J. Clin. Invest. 99, 944–952. doi:10.1172/jci119259
Tschumperlin D. J., Margulies S. S. (1999). Alveolar Epithelial Surface Area-Volume Relationship in Isolated Rat Lungs. J. Appl. Physiol. 86, 2026–2033. doi:10.1152/jappl.1999.86.6.2026
Tschumperlin D. J., Oswari J., Margulies A. S. S. (2000). Deformation-Induced Injury of Alveolar Epithelial Cells. Am. J. Resp Crit. Care 162, 357–362. doi:10.1164/ajrccm.162.2.9807003
Vlahakis N. E., Schroeder M. A., Limper A. H., Hubmayr R. D. (1999). Stretch Induces Cytokine Release by Alveolar Epithelial Cells In Vitro. Am. J. Physiol-lung C 277, L167–L173. doi:10.1152/ajplung.1999.277.1.l167
Walkey A. J., Sorbo L. D., Hodgson C. L., Adhikari N. K. J., Wunsch H., Meade M. O., et al. (2017). Higher PEEP versus Lower PEEP Strategies for Patients with Acute Respiratory Distress Syndrome. A Systematic Review and Meta-Analysis. Ann. Am. Thorac. Soc. 14, S297–S303. doi:10.1513/annalsats.201704-338ot
Wirtz H. R., Dobbs L. G. (2000). The Effects of Mechanical Forces on Lung Functions. Resp Physiol. 119, 1–17. doi:10.1016/s0034-5687(99)00092-4
Wong M. H., Johnson M. D. (2013). Differential Response of Primary Alveolar Type I and Type II Cells to LPS Stimulation. Plos One 8, e55545. doi:10.1371/journal.pone.0055545
Xuan W., Zhou Q., Yao S., Deng Q., Wang T., Wu Q. (2015). Mechanical Ventilation Induces an Inflammatory Response in Preinjured Lungs in Late Phase of Sepsis. Oxid Med. Cel Longev 2015, 364020. doi:10.1155/2015/364020
Zaiss D. M., Loosdregt J. V., Gorlani A., Bekker C. P., Grone A., Sibilia M., et al. (2013). Amphiregulin Enhances Regulatory T Cell-Suppressive Function via the Epidermal Growth Factor Receptor. Immunity 38, 275–284. doi:10.1016/j.immuni.2012.09.023
Zhang J., Ji J.-Y., Yu M., Overholtzer M., Smolen G. A., Wang R., et al. (2009). YAP-dependent Induction of Amphiregulin Identifies a Non-cell-autonomous Component of the Hippo Pathway. Nat. Cel Biol 11, 1444–1450. doi:10.1038/ncb1993
Keywords: modulation, VILI, static stretch, dynamic stretch, alveolar epithelial cells type 2, inflammation
Citation: Ferreira JMC, Huhle R, Müller S, Schnabel C, Mehner M, Koch T and Gama de Abreu M (2022) Static Stretch Increases the Pro-Inflammatory Response of Rat Type 2 Alveolar Epithelial Cells to Dynamic Stretch. Front. Physiol. 13:838834. doi: 10.3389/fphys.2022.838834
Received: 18 December 2021; Accepted: 28 March 2022;
Published: 11 April 2022.
Edited by:
Béla Suki, Boston University, United StatesReviewed by:
Ramaswamy Krishnan, Beth Israel Deaconess Medical Center and Harvard Medical School, United StatesSam Bayat, Université Grenoble Alpes, France
Copyright © 2022 Ferreira, Huhle, Müller, Schnabel, Mehner, Koch and Gama de Abreu. This is an open-access article distributed under the terms of the Creative Commons Attribution License (CC BY). The use, distribution or reproduction in other forums is permitted, provided the original author(s) and the copyright owner(s) are credited and that the original publication in this journal is cited, in accordance with accepted academic practice. No use, distribution or reproduction is permitted which does not comply with these terms.
*Correspondence: Jorge M. C. Ferreira, Sm9yZ2UuRmVycmVpcmFAdW5pa2xpbmlrdW0tZHJlc2Rlbi5kZQ==
†These authors have contributed equally to this work