- DMEM, INRAE, Montpellier University, Montpellier, France
Physical activity is now recognized as an essential element of healthy lifestyles. However, intensive and repeated exercise practice produces a high level of stress that must be managed, particularly oxidative damage and inflammation. Many studies investigated the effect of antioxidants, but reported only few positive effects, or even muscle recovery impairment. Secondary antioxidants are frequently highlighted as a way to optimize these interactions. Ergothioneine is a potential nutritional supplement and a secondary antioxidant that activates the cellular NRF2 pathway, leading to antioxidant response gene activation. Here, we hypothesized that ergothioneine could improve performance during aerobic exercise up to exhaustion and reduce exercise-related stress without impairing early muscle recovery signaling. To test this hypothesis, 5-month-old C56B6J female mice were divided in two groups matched for maximal aerobic speed (MAS): control group (Ctrl; n = 9) and group supplemented with 70 mg ergothioneine/kg/day (ET; n = 9). After 1 week of supplementation (or not), mice performed a maximum time-to-exhaustion test by running on a treadmill at 70% of their MAS, and gastrocnemius and soleus muscles were collected 2 h after exercise. Time to exhaustion was longer in the ET than Ctrl group (+41.22%, p < 0.01). Two hours after exercise, the ET group showed higher activation of protein synthesis and satellite cells, despite their longer effort. Conversely, expression in muscles of metabolic stress and inflammation markers was decreased, as well as oxidative damage markers in the ET group. Moreover, ergothioneine did not seem to impair mitochondrial recovery. These results suggest an important effect of ergothioneine on time-to-exhaustion performance and improved muscle recovery after exercise.
Introduction
Physical activity is now recognized as an essential element of healthy lifestyles. However, the frequent practice of intensive physical activity produces high stress levels, particularly oxidative stress and inflammation, that must be managed (Wang et al., 2006; Sureda et al., 2009; Kawamura and Muraoka, 2018). Many athletes in aerobic-dominant sports empirically use anti-oxidant supplementation to counteract the overproduction of reactive oxygen and nitric species (RONS) and the inflammation occurring during exercise (Macera et al., 2003; Bassel-Duby and Olson, 2006; Warburton et al., 2006; Schnohr et al., 2015; Ranchordas et al., 2017). These supplementation strategies may have several objectives, such as performance gain, or improving muscle adaptation/recovery after a training session and/or competition (Knapik et al., 2016). RONS overproduction during exercise can affect performance, especially in long endurance events, exhaustive exercise, or extended and repeated high intensity exercise bouts (McKenna et al., 2006; Paschalis et al., 2016; Reid, 2016). Indeed, RONS overproduction, especially by mitochondria, NADPH and xanthine oxidase systems, during exercise can induce muscle strength loss and fatigue (Powers and Jackson, 2008; Powers et al., 2011, 2016). This is explained by the many effects of oxidizing molecules, leading to protein, lipid and DNA damage, and also by their implication in different pathways, for instance, muscle protein synthesis, protein degradation, excitation-contraction coupling (calcium movements) and apoptosis (Powers et al., 2011, 2016).
Several groups have investigated the effect of antioxidants as a strategy to reduce exercise-related damage (Braakhuis and Hopkins, 2015; Ranchordas et al., 2017). However, they found only few positive effects, and these results are debated. Some studies, mainly using N-acetylcysteine (a glutathione precursor), reported a performance increase or fatigue delay during aerobic exercise (e.g., cycling or running time to exhaustion; Medved et al., 2004; Braakhuis and Hopkins, 2015; Rhodes and Braakhuis, 2017; Paschalis et al., 2018). However, antioxidant use in training is currently not recommended, despite the fact that they can increase performance during aerobic exercise. Indeed, RONS are essential components of the muscular adaptations associated with exercise (Margaritelis et al., 2020). During aerobic exercise, the transient RONS overproduction stimulates PGC1α (peroxisome proliferator-activated receptor gamma coactivator 1-alpha) production in skeletal muscle cells, the major regulator of mitochondrial biogenesis and metabolism adaptation (Pilegaard et al., 2003; Lin et al., 2005) through induction of specific genes, such as NRF-1 (nuclear respiratory factor 1) and mtTFA (mitochondrial transcription factor A; Pilegaard et al., 2003; Lin et al., 2005; Silveira et al., 2006; Kang et al., 2009; Gomez-Cabrera et al., 2015). Some studies have shown that primary antioxidants, such as vitamin C and E, may prevent the activation of the PGC1α pathway, thus blunting mitochondrial adaptations to exercise and consequently reducing the gain in maximal oxygen uptake and maximal endurance time (Gomez-Cabrera et al., 2008; Ristow et al., 2009; Strobel et al., 2011; Merry and Ristow, 2016). Moreover, other studies using a resistance training protocol and primary antioxidant supplementation highlighted the inhibition of some training adaptation (e.g., muscle power increase, muscle fatigue delay, and muscle hypertrophy). This inhibition seems to act through a lower increase in insulin sensitivity that normally stimulates the activation of the mTOR signaling pathway (Malm et al., 1997; Wang and Proud, 2006; Schiaffino et al., 2013; Paulsen et al., 2014a,b; Bjørnsen et al., 2016). For example, after a 10-week strength training protocol in women, the gains in peak torque and total work were lower in the group with vitamin C and E supplementation than in the placebo group (Dutra et al., 2018). In addition, Arc-Chagnaud et al. showed that during chronic inactivity, RONS are necessary to maintain muscle function (Arc-Chagnaud et al., 2020). They also found that expression of the main components of the PGC1α and mTOR pathways were decreased in the group with antioxidant supplementation during the re-loading phase, in agreement with the results by Gomez-Cabrera et al. on adaptation to exercise. These findings highlight again the importance of the interactions between redox balance, physical exercise/mechanical constraints, and antioxidant effects (Reid, 2001, 2016; Schnohr et al., 2015).
These interactions might be optimized by personalizing the supplement cocktail (Paschalis et al., 2016; Margaritelis et al., 2018a,b), or by developing new secondary antioxidants that interact with the antioxidant response elements (ARE) of genes rather than activate RONS scavengers (Merry and Ristow, 2016). Secondary antioxidants (e.g., resveratrol) show beneficial effects on exercise performance and oxidative stress (Wu et al., 2013; Baltaci et al., 2016). However, due to their low bioavailability, their utilization in humans is limited and other molecules must be tested/developed (Walle et al., 2004). Ergothioneine, a secondary antioxidant derived from fungi and bacteria, is a potential candidate (Tang et al., 2018). Although ergothioneine is not produced by mammals, it can be found at high concentrations in human and animal tissues through the action of a specific transporter (OCTN1; Halliwell et al., 2016). This molecule is a nuclear factor erythroid 2-related factor 2 (NRF2) pathway activator and allows the activation of genes implicated in the cell antioxidant response (Ma, 2013; Hseu et al., 2015; Crilly et al., 2016). Several studies have described its antioxidant and anti-inflammatory properties in vitro and in vivo (Cheah and Halliwell, 2012; Halliwell et al., 2016). For example, ergothioneine protects several cell types against oxidative damage and apoptosis after exposure to RONS or UV radiation (Aruoma et al., 1999; Markova et al., 2009). Moreover, administration of pure ergothioneine in middle-aged healthy individuals decreases oxidative stress and inflammation markers (Cheah et al., 2017).
The objectives of this study were to test ergothioneine effects on the aerobic performance and to analyze its impact on muscle molecular adaptations to exercise, which are normally blunted by primary antioxidants. We hypothesized that pure ergothioneine does not impair the early adaptations to aerobic exercise and improves performance in a time-to-exhaustion treadmill protocol.
Materials and Methods
Ethical Review and Study Design
This study was approved by the Languedoc-Roussillon ethics committee (APAFIS#28764–-2020122115407491). Five-month-old C56B6J female mice were acclimated and assigned to two groups: control group (Ctrl; n = 9) and group supplemented with ergothioneine (ET; n = 9). After four habituations sessions to treadmill exercise, the maximal aerobic speed (MAS) on the treadmill was measured 1 week before supplementation initiation. Mice in the two groups were MAS-matched. After 1 week of supplementation with pure ergothioneine, mice performed a double-blind time-to-exhaustion exercise on the treadmill at 70% of their individual MAS. Then, mice were sacrificed 2 h after the exercise end, and muscle samples were collected. Puromycin (40 nmol/g of body weight) was injected with intraperitoneal injection (i.p) 20 min before euthanasia (Merle et al., 2019; Figure 1).
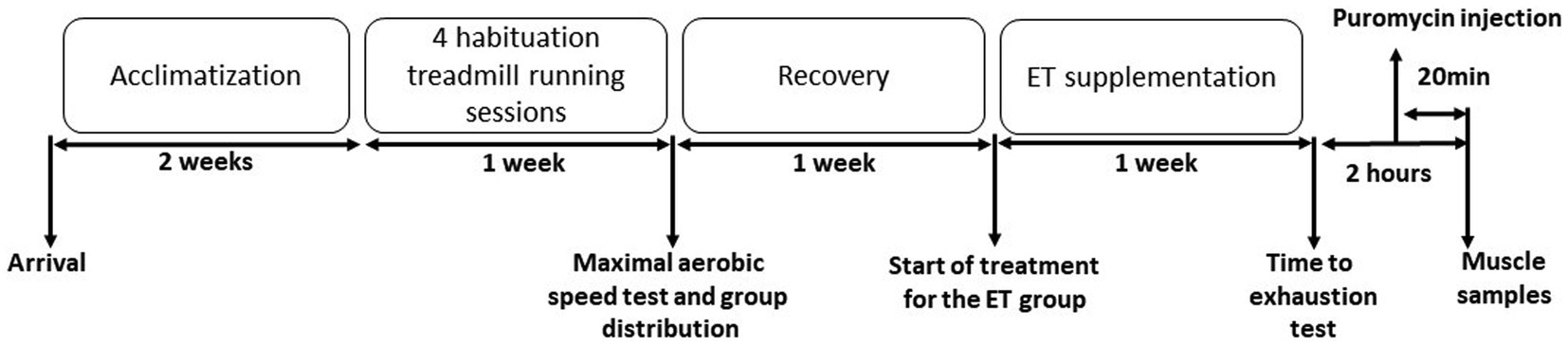
Figure 1. Experimental protocol. Mice were acclimatized to their cages for 2 weeks, before undergoing four treadmill running habituation sessions. One week before supplementation initiation, the maximal aerobic speed (MAS) was measured by running on the treadmill. Then, mice were distributed in the control (Ctrl) and ergothioneine (ET) supplementation group according to their MAS. After 1 week of ET supplementation (or not for the Ctrl group), mice performed a time to exhaustion test at 70% of their individual MAS. Puromycin was injected 100 min after the test end and muscles were collected after sacrifice at 120 min after the exercise end.
Supplementation
Pure ergothioneine was provided by Tetrahedron (Paris, France). Ergothioneine was given at the concentration of 70 mg/kg/day for 1 week, as previously described (Tang et al., 2018). Ergothioneine was diluted in the drinking water (each mouse was in one cage) and adjusted according to variations in water intake and body weight.
Maximal Aerobic Speed Measurement
MAS was measured on a treadmill (Exer-6 M Treadmill; Columbus instruments, Oh, United States) using a standard protocol (Gouraud et al., 2019). Mice were progressively acclimatized to treadmill running by increasing the speed and duration of exercise (four habituation sessions). The MAS measurement protocol began with a warming up time (5 min at 6 m·min−1, 2 min at 8 m·min−1, and 2 min at 10 m·min−1). Then, the treadmill running velocity was increased by 2 m·min−1 every min until exhaustion, defined as the inability to start running again after 10 s. The speed at exhaustion was considered to be the MAS.
Time-to-Exhaustion Test
The time-to-exhaustion test was performed on the treadmill. After the warming up step (5 min at 6 m·min−1 and 5 min at 10 m·min−1), running speed was increased by 2 m·min−1 every minute until 70% of each mouse MAS. Then, mice run at this speed until exhaustion. Exhaustion was defined as the inability to return to treadmill running after 10 s.
Muscle Samples
Soleus and gastrocnemius muscles were collected straight after sacrifice. Samples were rapidly frozen in liquid nitrogen and stored at −80°C for mRNA and protein extraction.
Western Blot Analysis
Muscles (n = 1 gastrocnemius and n = 2 soleus/mouse) were homogenized in 10 volumes of lysis buffer [50 mM Tris–HCl (pH 7.5), 150 mM NaCl, 1 mM egtazic acid, 1 mM EDTA, 100 mM NaF, 5 mM Na3VO4, 1% Triton X-100, 1% sodium dodecyl sulphate (SDS), 40 mM β-glycerophosphate, and a protease inhibitor mixture (P8340; Sigma-Aldrich, Saint-Louis, MO, United States)] and centrifuged at 10,000 × g for 10 min (4°C). Fifty micrograms of each protein extract were loaded on stain-free 4–20% precast gels (4568095; Bio-Rad, Hercules, CA, United States) for protein separation by electrophoresis followed by transfer to nitrocellulose membranes (Trans-Blot Turbo Blotting System; Bio-Rad). Membranes were blocked with 50 mM Tris–HCl (pH 7.5), 150 mM NaCl, and 0.1% Tween-20 containing 5% skim milk or bovine serum albumin and incubated at 4°C with primary antibodies overnight (Table 1). Membranes were then incubated with the relevant secondary antibodies at room temperature for 1 h (Table 1), followed by the Pierce ECL kit (32106; Thermo Fisher Scientific, Waltham, MA, United States). Bands were visualized with the ChemiDoc Touch Imaging System (Bio-Rad) and quantified with Image Lab™ Touch (version 5.2.1). The Stain-Free technology was used as loading control (Gilda and Gomes, 2015; Vigelsø et al., 2015; Faden et al., 2016; Pagano et al., 2018).
Carbonylated Proteins
The Oxyblot Protein Oxidation Detection Kit (Millipore, United States) was used for carbonylated protein analysis. Protein samples were denatured with 12% SDS (final concentration: 6% SDS). Samples were then derivatized by adding 2,4-dinitrophenylhydrazine (DNPH) and incubated at room temperature for 15 min. The reaction was stopped by adding a neutralization solution before electrophoretic separation on 4–20% precast gels (5678094; Bio-Rad) followed by transfer onto nitrocellulose membranes (Bio-Rad; Trans-Blot Turbo Blotting System). Membranes were washed in PBS-T and incubated with the primary antibody diluted (1:150) in blocking solution at room temperature for 1 h. Membranes were then washed in PBS-T and incubated with a secondary antibody diluted in blocking solution (1:300) at room temperature for 1 h. Then, membranes were washed and proteins were visualized with the ChemiDoc Touch Imaging System after incubation with the Pierce ECL kit (32106; Thermo Scientific) for 5 min. Carbonylated proteins were quantified with Image Lab™ Touch (version 5.2.1) relative to Ponceau Red staining (loading control).
Gastrocnemius Muscle RNA Extraction and Reverse Transcription
RNA could be extracted only from gastrocnemius samples because both soleus muscles were used for protein analysis. Each muscle sample was crushed in 1 ml of TRIzol in a FastPrep-24 device and incubated at room temperature for 5 min. This was followed by addition of 0.2 ml chloroform and incubation at room temperature for 3 min. Tubes were centrifuged at 9,384 g, 4°C for 15 min. The upper phase was collected and incubated at room temperature with 0.5 ml of isopropanol for 1 h, followed by centrifugation at 9,384 g, 4°C, for 5 min. Pellets were washed twice with 75% ethanol followed by centrifugation at 7,500 g for 5 min. RNA pellets were resuspended in 30 μl of RNA-free water and RNA quantified with a spectrophotometer (260 nm). After reverse transcription of each RNA sample using the High-Capacity cDNA Reverse Transcription Kit (4368813, Applied Biosystem), samples were stored at −20°C.
Quantitative PCR Analysis
Quantitative PCR (qPCR) was performed with the SensiFAST SYBR Hi-ROX Kit (Bioline) and the primers are listed in Table 2 using a StepOnePlus Real-Time PCR system. Data were analyzed with the StepOnePlus 2.3 software. The relative mRNA levels were normalized to the levels of the Rps9 and tubulin housekeeping genes that were unaffected by the experimental protocol. Results were expressed using the comparative cycle threshold. The relative changes in the level of a specific gene were calculated with the ΔΔCT formula.
Statistical Analyses
All data are expressed as the mean ± SD and the significance level was set at p < 0.05. Differences between groups were evaluated using the t-test or the Mann–Whitney U test when the data deviated from the normal distribution. For weight comparison, two-way ANOVA for paired data was used. Statistical analyses were done with the Statistica software 7.1, and graphs were generated with GraphPad Prism4 (San Diego, United States).
Results
Mouse Body Weight
The mean body weight was not different between groups (Ctrl and ET) throughout the protocol: 23.66 ± 1.90 g and 23.7 ± 2.08 g before the 1-week supplementation and 23.56 ± 1.34 g and 23.52 ± 1.61 g after the 1-week supplementation in the Ctrl and ET group, respectively.
Maximal Aerobic Speed and Time to Exhaustion
MAS was exactly the same in the Ctrl and ET group at baseline (26.66 ± 3.32 m/min). Conversely, after 1 week of supplementation, time to exhaustion at 70% of MAS was significantly higher in the ET than Ctrl group: 71.55 ± 14 min and 50.4 ± 8.41 min (+41,22%; p < 0.01; Figure 2).
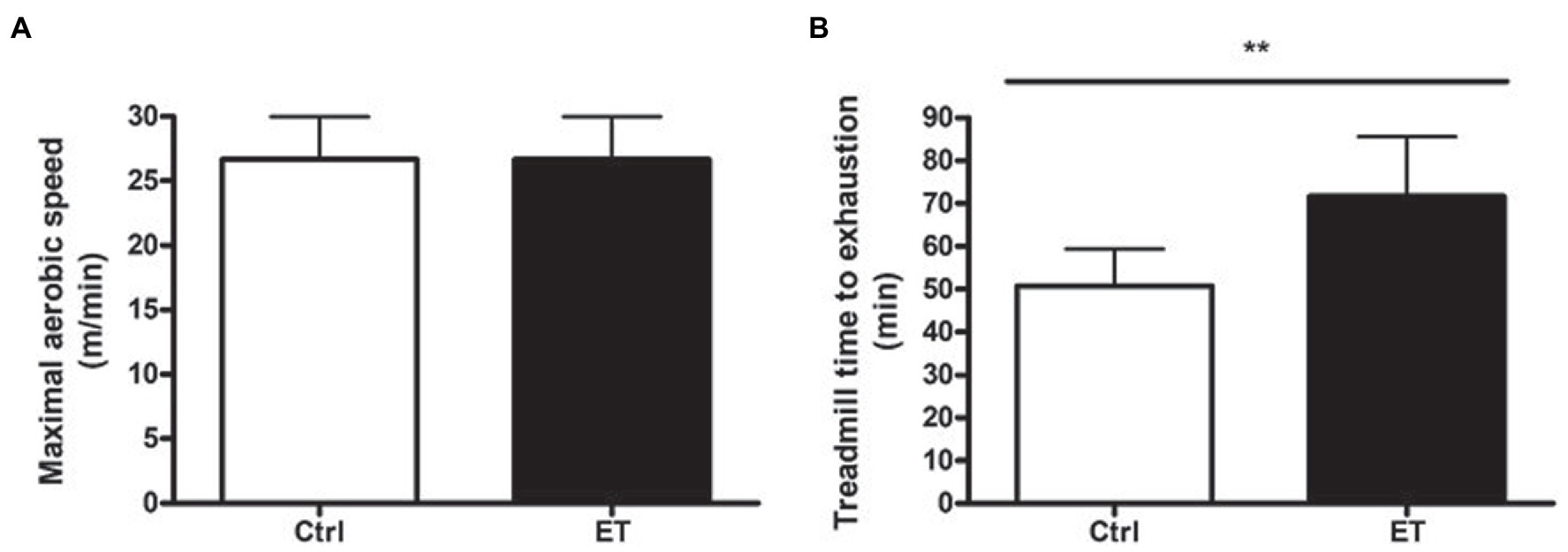
Figure 2. Functional tests. (A) Maximal aerobic speed (treadmill running; m/min) in the ergothioneine (ET; n = 9) and control (Ctrl; n = 9) groups before starting ergothioneine supplementation. (B) Time to exhaustion test (min) by running on a treadmill at 70% of the maximal aerobic speed after 1 week of ergothioneine supplementation (ET) or not (Ctrl). *p < 0.05, **p < 0.01 vs. Ctrl.
Muscle Protein Synthesis and Regulating Pathway
After 1 week of supplementation, puromycin incorporation (as a marker of global protein synthesis) in gastrocnemius and soleus was higher in the ET than Ctrl group (+12% and + 17.8%, respectively; p < 0.05 for both) and RPS6 phosphorylation (+14.1%, p < 0.01; and + 15.7%, p < 0.05, respectively; Figure 3). 4EBP1 phosphorylation showed no difference between groups and muscles. Akt, Mtor, Rps6, 4ebp1, and IGF1 mRNA levels in gastrocnemius were not different (Figure 4).
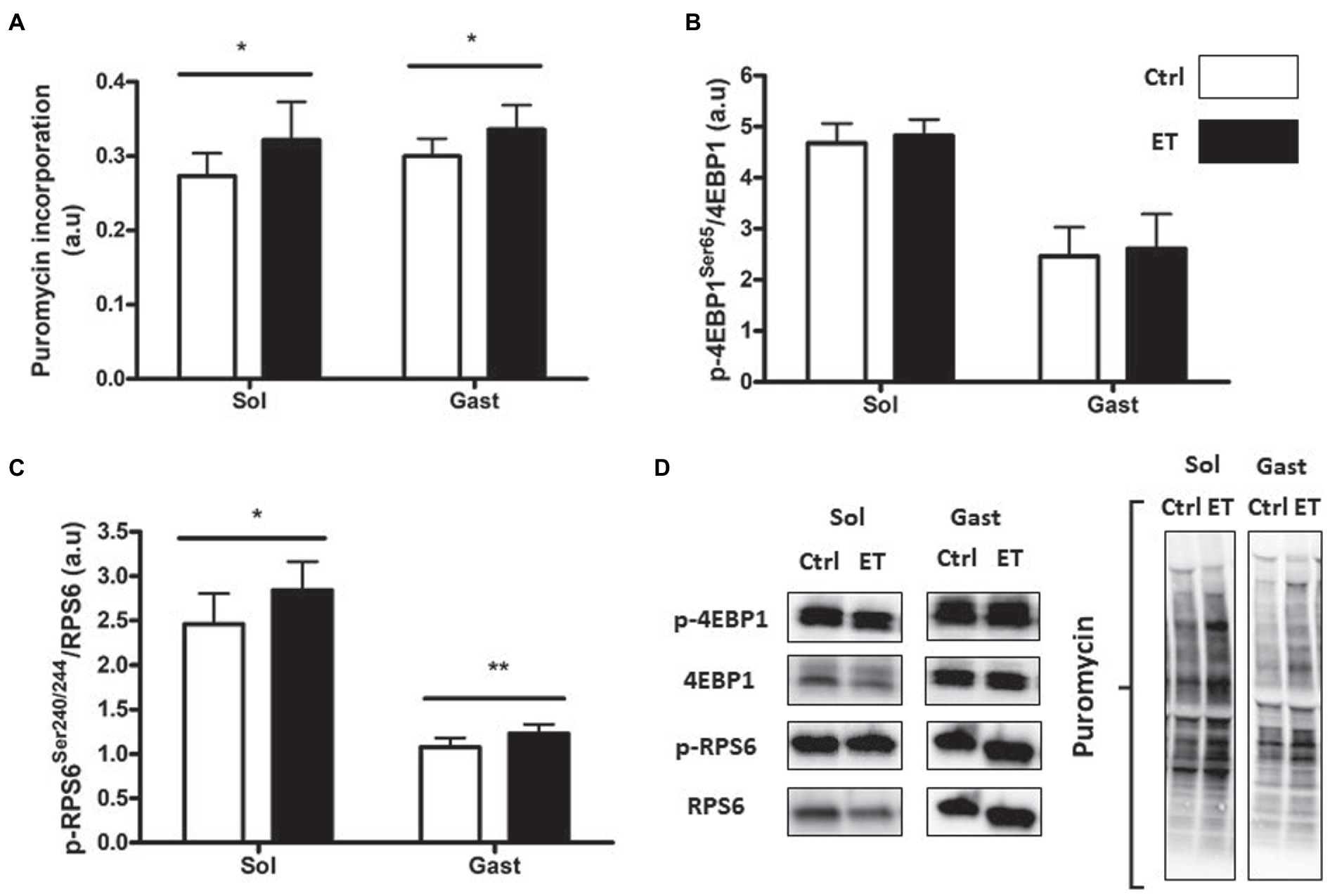
Figure 3. Ergothioneine effect on protein synthesis markers at 2 h post-exercise. Evaluation of puromycin incorporation and protein synthesis marker expression in soleus (n = 2/mouse) and gastrocnemius (n = 1/mouse) samples. (A) Quantification of protein synthesis by measuring puromycin incorporation in muscles. (B) Phosphorylated 4EBP1 (Ser65)/total 4EBP1 protein ratio post-exercise. (C) Phosphorylated RPS6 (Ser240/244)/total RPS6 protein ratio. (D) Representative Western Blots. *p < 0.05; ** p < 0.01 vs. Ctrl.
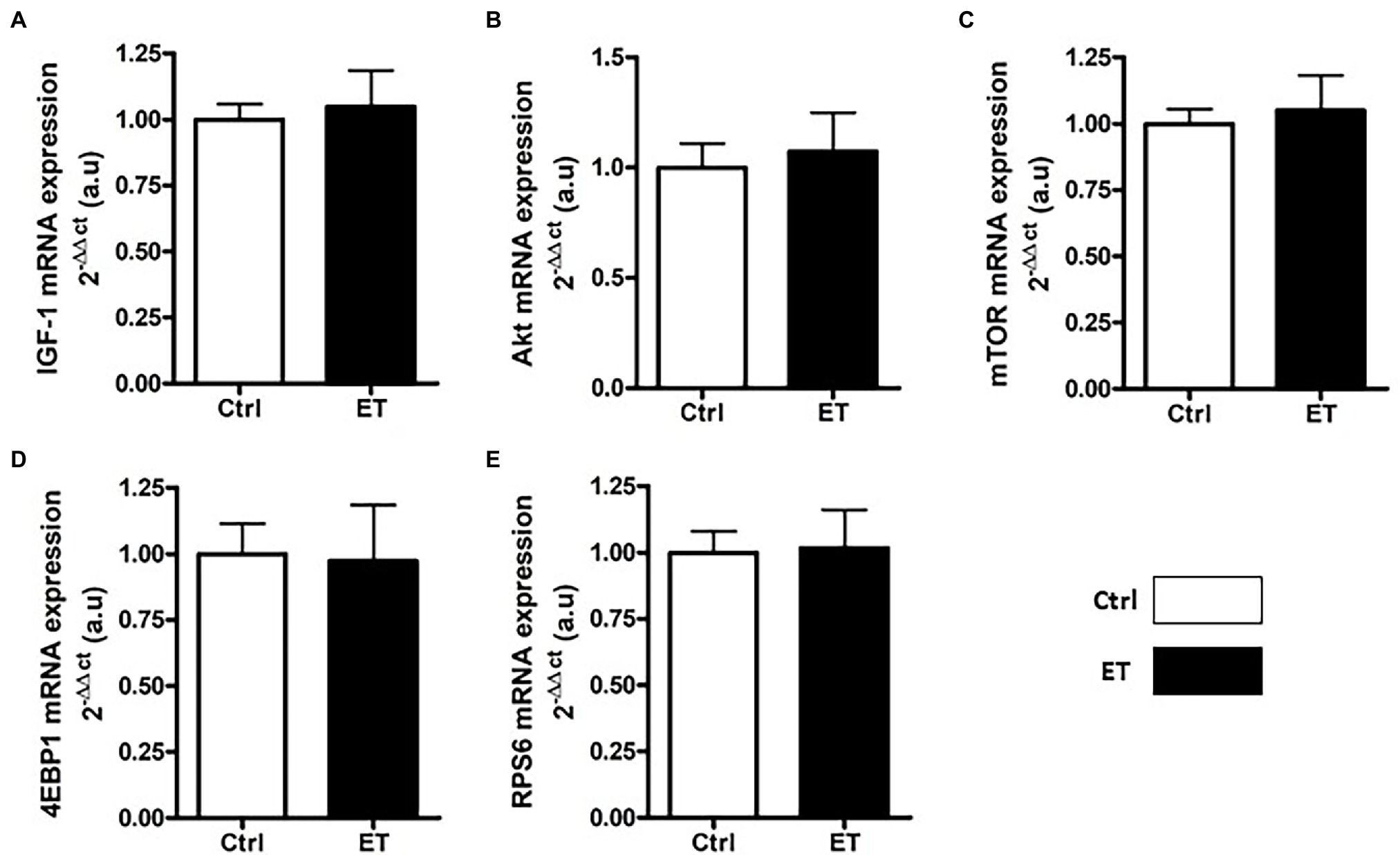
Figure 4. Ergothioneine effect on transcription of protein synthesis markers. Expression of genes encoding protein synthesis markers in gastrocnemius samples (n = 1/mouse). (A) IGF1 mRNA level. (B) Akt mRNA level. (C) Mtor mRNA level. (D) 4ebp1 mRNA level. (E) Rps6 mRNA level; *p < 0.05 vs. Ctrl.
Muscle Protein Breakdown Markers
Two hours after the time-to-exhaustion-test, the muscle expression (gene and protein) of the E3 ligase MurF1 and MAFbx, markers of the Ubiquitin Proteasome System (UPS), was comparable in Ctrl and ET mice (Figure 5), as well as total ubiquitinated protein content. Moreover, ULK1 phosphorylated at Ser757, LC3.2/LC3.1 expression ratio, and p62 protein level, three autophagy markers, were comparable in gastrocnemius and soleus samples from both groups (Figure 6). Analysis of two mitochondrial autophagy markers showed that Parkin mRNA level in gastrocnemius was lower in the ET group than Ctrl group (−8%; p < 0.01), whereas Pink1 expression was comparable between groups (Figure 6).
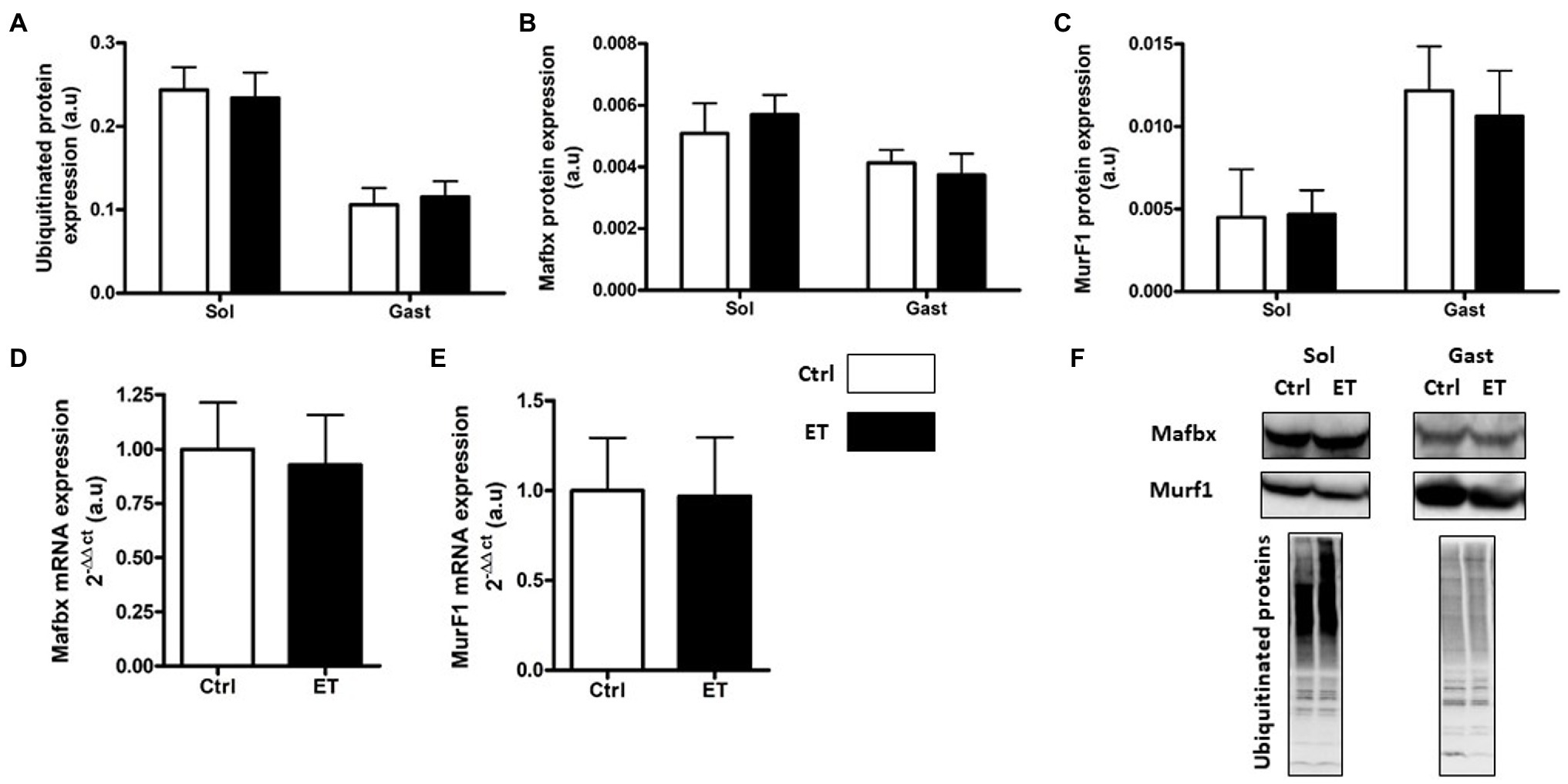
Figure 5. Ergothioneine effect on ubiquitin proteasome markers (UPS) 2 h after exercise. UPS markers in soleus and gastrocnemius samples and expression of genes encoding UPS markers in gastrocnemius samples. (A) Total ubiquitinated proteins. (B) MAFbx protein expression. (C) MurF1 protein expression. (D) Mafbx mRNA expression. (E) MurF1 mRNA expression. (F) Representative Western Blots; *p < 0.05 vs. Ctrl.
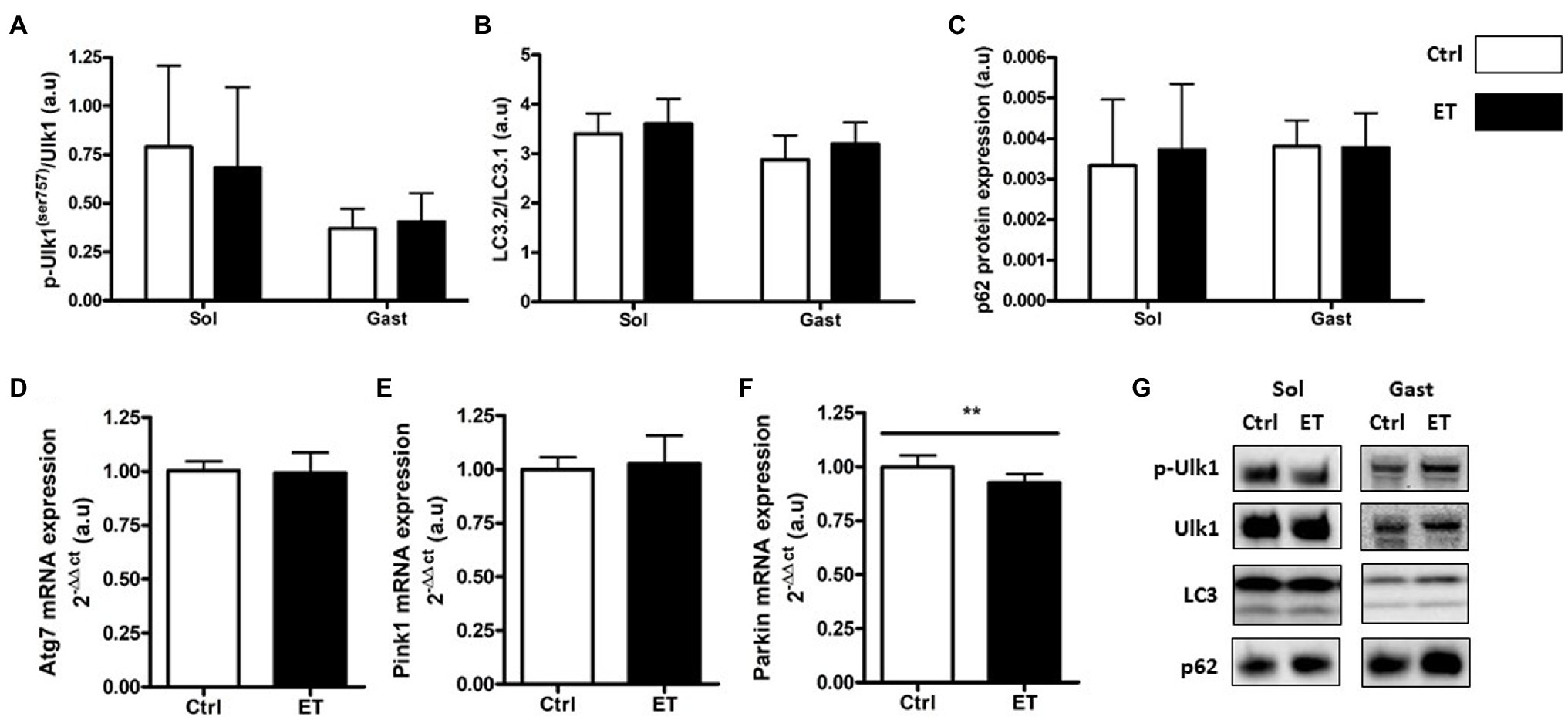
Figure 6. Ergothioneine effect on autophagy markers 2 h after exercise. Autophagy markers in soleus and gastrocnemius muscle and expression of genes encoding autophagy markers in gastrocnemius muscle. (A) Phosphorylated ULK1 (Ser757)/total ULK1 ratio. (B) LC3.2/LC3.1 protein ratio. (C) p62 protein expression. (D) Atg7 mRNA level. (E) Pink1 mRNA level. (F) Parkin mRNA level. (G) Representative Western Blots; **p < 0.01 vs. Ctrl.
Metabolic Stress Markers
In the ET group, AMPKα phosphorylation was significantly lower in gastrocnemius samples (−22.05%; p < 0.01 vs. Ctrl group) but not in soleus samples (−41.9%; p = 0.054; Figure 7). Conversely, the expression of its gene AMPKα was comparable in ET and Ctrl gastrocnemius samples. Redd1 protein and mRNA expression levels were similar between groups. GSK3 phosphorylation was comparable between groups in gastrocnemius, but was reduced in soleus samples from ET mice (−30.45%; p < 0.05 vs. Ctrl). Gsk3 mRNA levels in gastrocnemius samples were similar between groups.
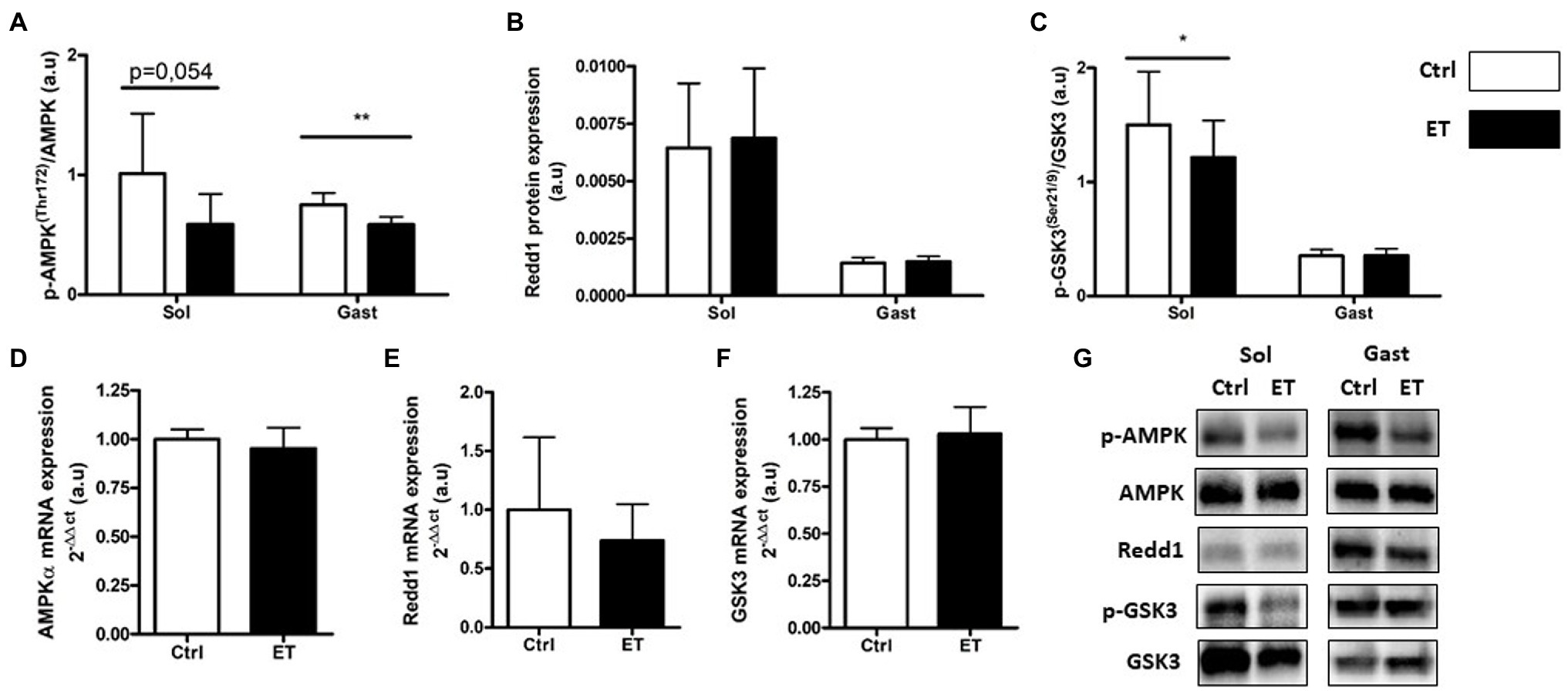
Figure 7. Ergothioneine effect on metabolic stress markers 2 h after exercise. Metabolic stress markers in soleus and gastrocnemius muscle and expression of genes encoding metabolic markers in gastrocnemius muscle. (A) Phosphorylated AMPKa (Thr172)/total AMPKa ratio. (B) Redd1 protein expression. (C) Phosphorylated GSK3 (Ser21/9)/total GSK3 ratio. (D) AMPKa mRNA level. (E) Redd1 mRNA level. (F) Gsk3 mRNA level. (G) Representative Western Blots; *p < 0.05; **p <0.01 vs. Ctrl.
Inflammation Markers
TNF-α protein expression in gastrocnemius and soleus samples was comparable between groups (Figure 8). Conversely, Tnf-α mRNA level in gastrocnemius samples was significantly lower in the ET group (−34%; p < 0.05). IL-1β protein expression was lower in ET than Ctrl soleus samples (−16.4%; p < 0.05), but not in gastrocnemius samples. Il1β and Il6 mRNA levels were decreased in ET gastrocnemius samples (−56%, p < 0.01; and − 22%, p < 0.05 vs. Ctrl samples, respectively).
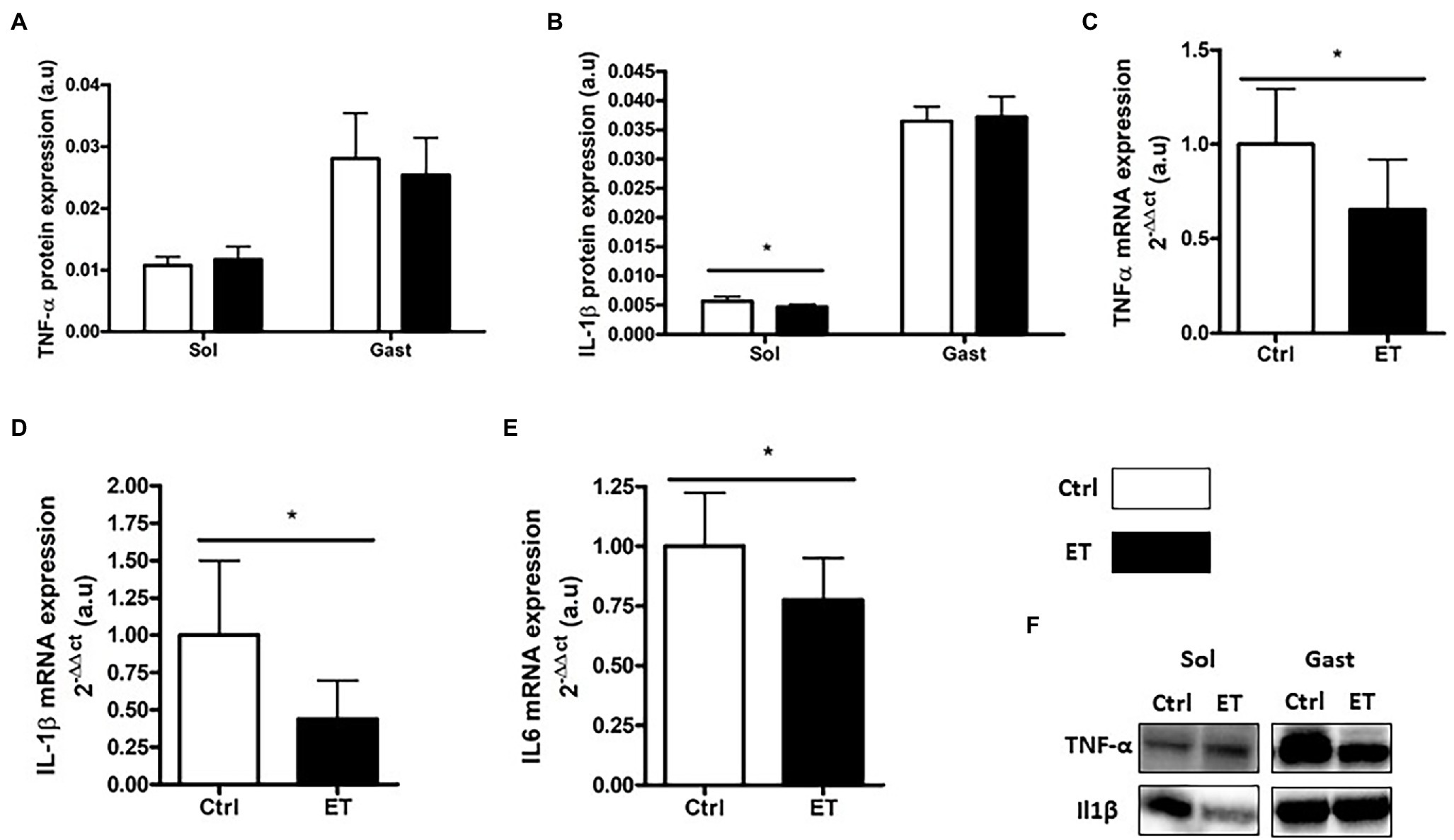
Figure 8. Ergothioneine effect on muscle inflammatory markers 2 h after exercise. Inflammatory marker (protein and gene) expression in soleus and gastrocnemius samples. (A) TNF-a protein expression. (B) IL-1b protein expression. (C) Tnfa mRNA level. (D) IL-1b mRNA level. (E) IL6 mRNA level. (F) Representative Western Blots; *p < 0.05 vs. Ctrl.
Oxidative Stress Markers
Lipid (4HNE adduct quantification) and protein peroxidation (oxidized protein expression) in gastrocnemius and in soleus samples were comparable between groups (Figure 9). The p53 phosphorylated on Ser15/total p53 and the p38 phosphorylated on Thr180/Tyr182/total p38 ratios were similar in gastrocnemius and soleus samples from both groups.
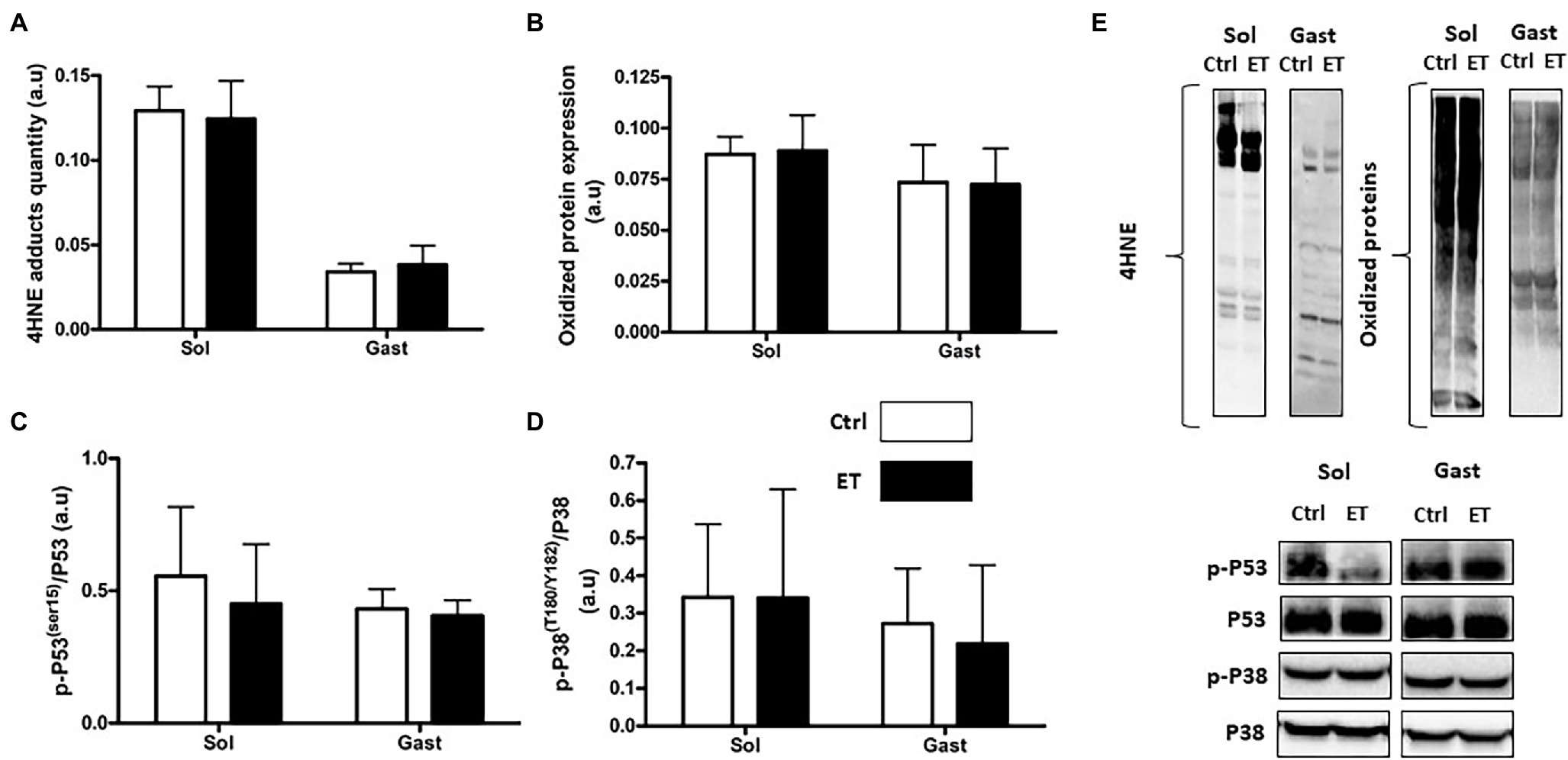
Figure 9. Ergothioneine effect on oxidative stress markers 2 h after exercise. Oxidative stress markers in soleus and gastrocnemius samples. (A) 4HNE adduct quantification. (B) Oxidized protein expression. (C) Phosphorylated p53 (Ser15)/total p53 ratio. (D) Phosphorylated p38(Thr180/Tyr182)/p38 ratio. (E) Representative Western Blots; *p < 0.05 vs. Ctrl.
Antioxidant Cell Defenses
SOD1 and SOD2 protein expression in gastrocnemius and in soleus showed no difference between groups (Figure 10) as well as SOD1, SOD2 and Gpx1 mRNA levels in gastrocnemius. Conversely, Nrf2 mRNA level was significantly higher in the ET group (+14%; p < 0.05 vs. Ctrl).
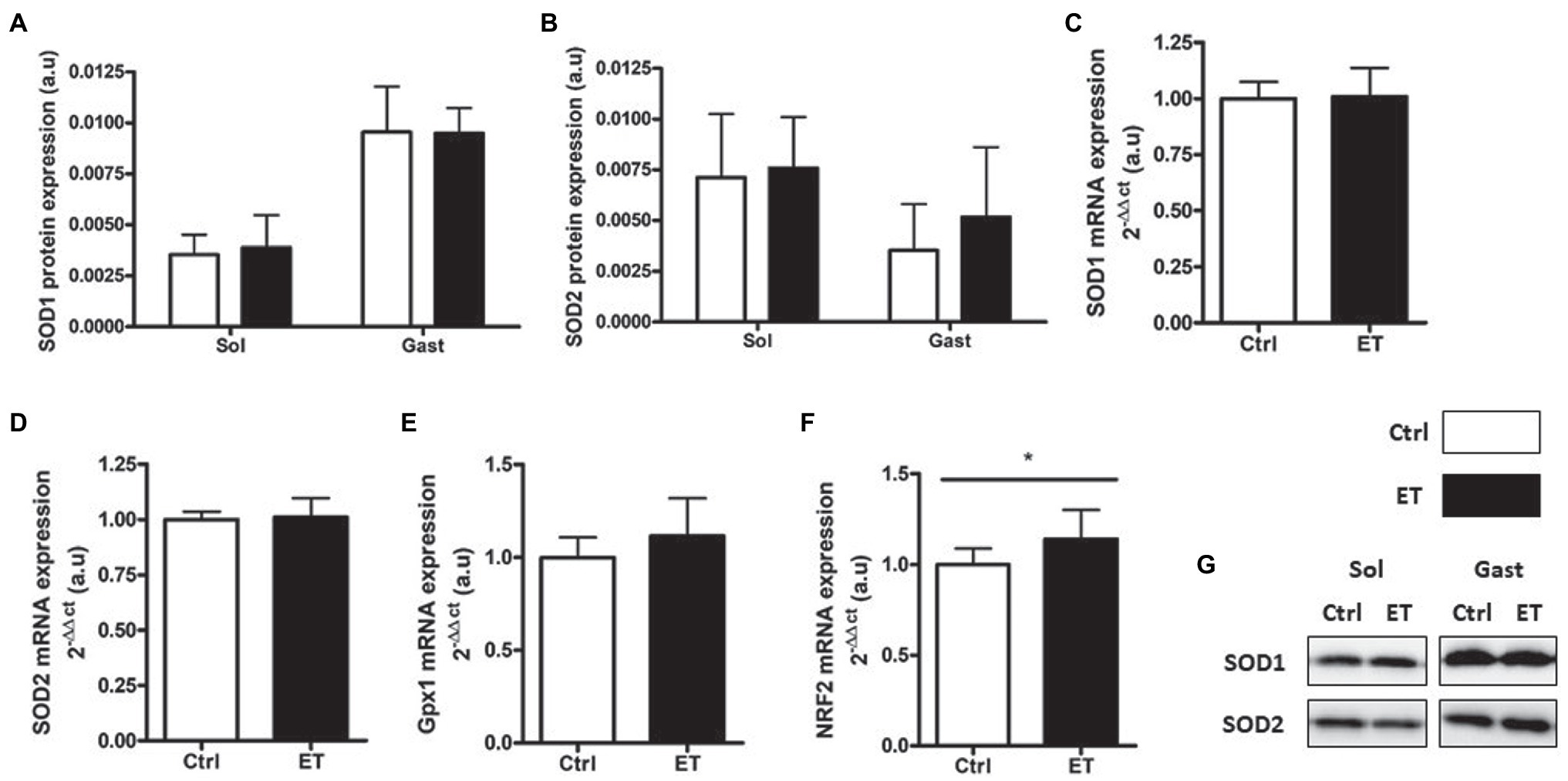
Figure 10. Ergothioneine effect on antioxidant defense markers 2 h after exercise. Antioxidant defense markers in soleus and gastrocnemius samples. (A) SOD1 protein expression. (B) SOD2 protein expression. (C) SOD1 mRNA level. (D) SOD2 mRNA level. (E) Gpx1 mRNA level. (F) Nrf2 mRNA level. (G) Representative Western Blots; *p < 0.05 vs. Ctrl.
Mitochondrial Pathway
PGC1α, cytochrome C and COX IV protein content were similar in ET and Ctrl gastrocnemius and soleus samples (Figure 11) as well as Tfam, Pgc1α and Nrf1 mRNA levels in gastrocnemius.
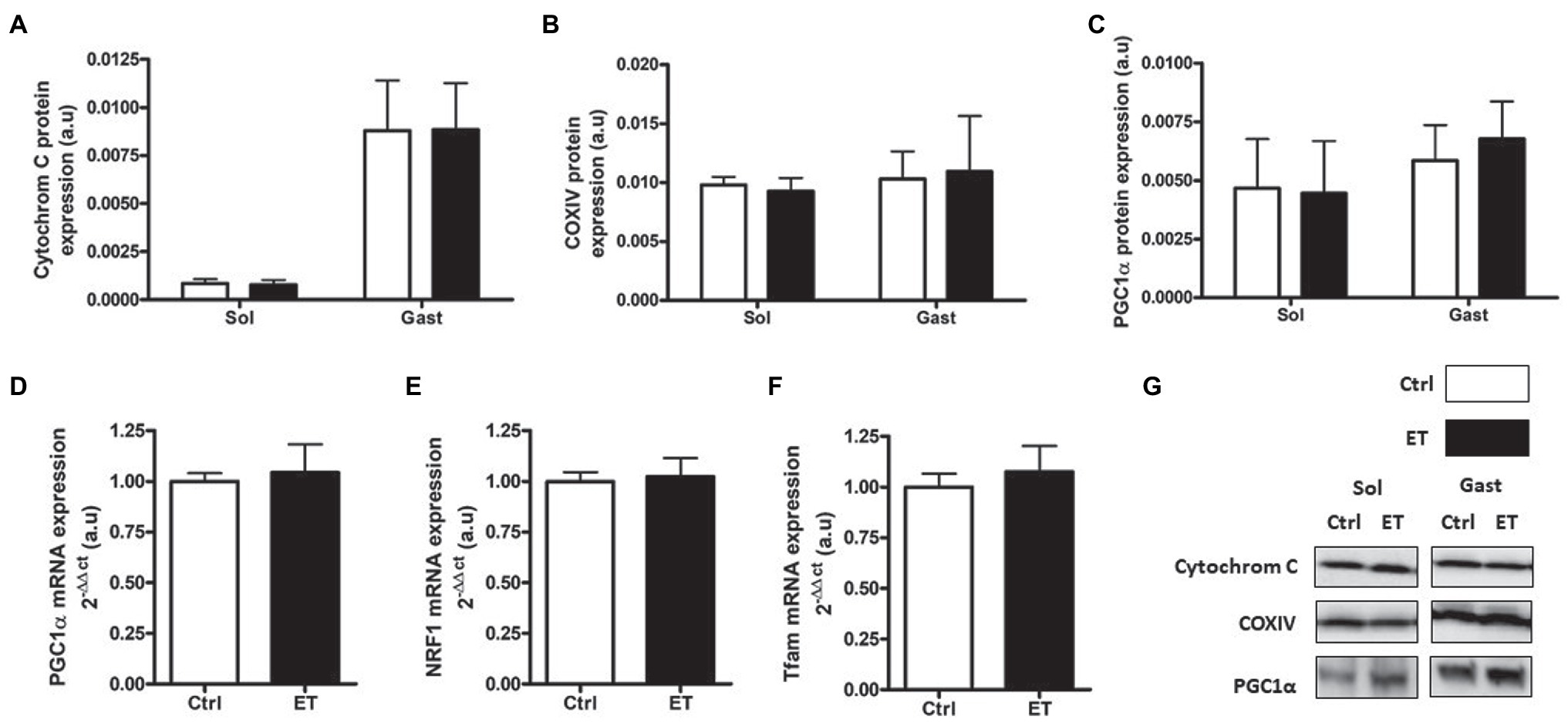
Figure 11. Ergothioneine effect on mitochondrial markers 2 h after exercise. Mitochondrial markers. (A) Cytochrome C protein expression. (B) COX IV protein expression. (C) PGC1a protein expression. (D) PGC1a mRNA level. (E) NRF1 mRNA level. (F) Tfam mRNA level. (G) Representative Western Blots; *p < 0.05 vs. Ctrl.
Muscle Satellite Cells
PAX7, MyoD, Myogenin and Spy-1 protein expression levels were all increased in ET soleus (+35.1%, p < 0.05; +72.8%, p < 0.05; +26.6%, p < 0.01; +67,4%, p < 0.05 vs. Ctrl, respectively), but only MyoD in gastrocnemius (+116.2%, p < 0.05 vs. Ctrl; Figure 12). Pax7, MyoD and Myogenin mRNA levels in gastrocnemius were similar between groups (Figure 13).
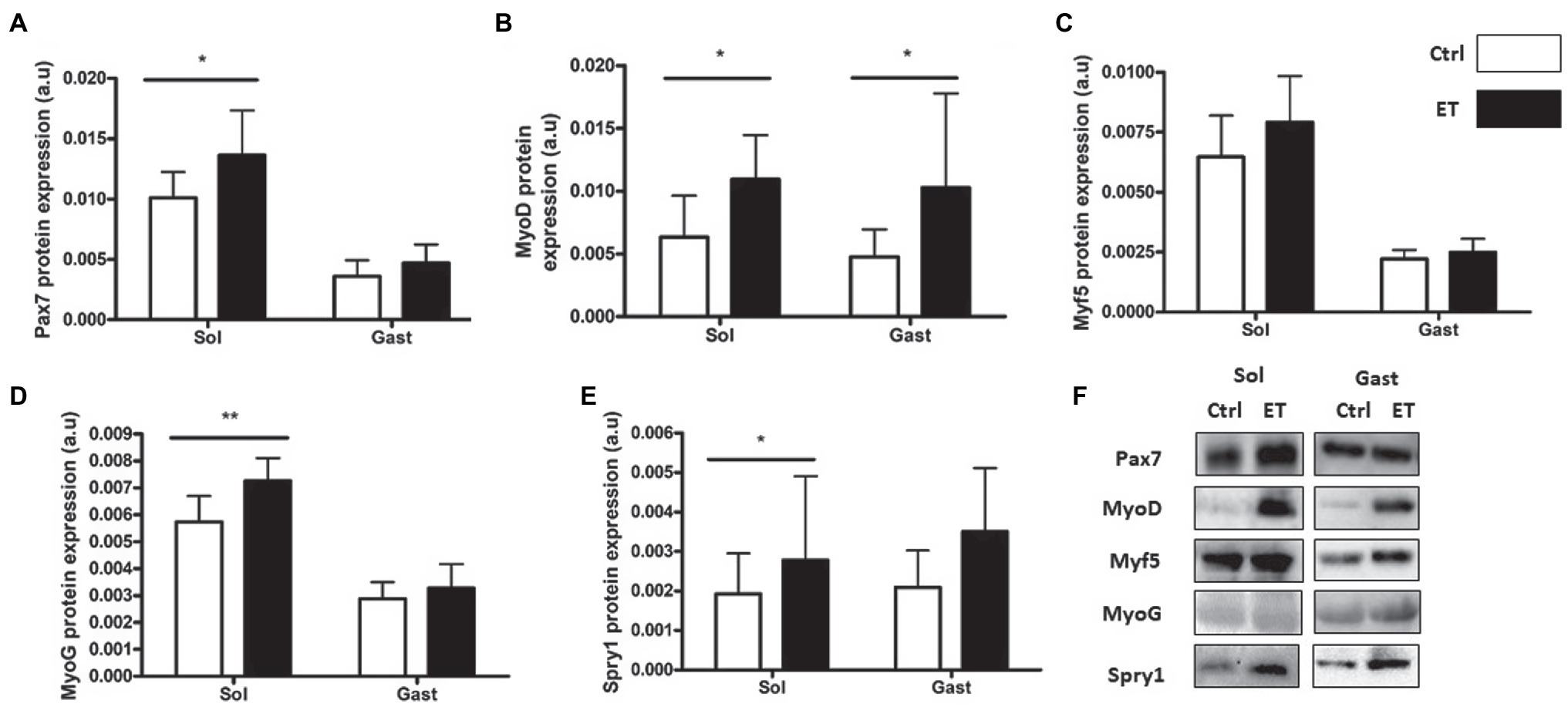
Figure 12. Ergothioneine effect on satellite cell markers 2 h after exercise. Satellite cell markers in soleus and gastrocnemius samples. (A) PAX7 protein expression. (B) MyoD protein expression. (C) Myf-5 protein expression. (D) Myogenin protein expression. (E) Spry-1 protein expression. (F) Representative Western Blots; *p < 0.05; **p < 0.01 vs. Ctrl.
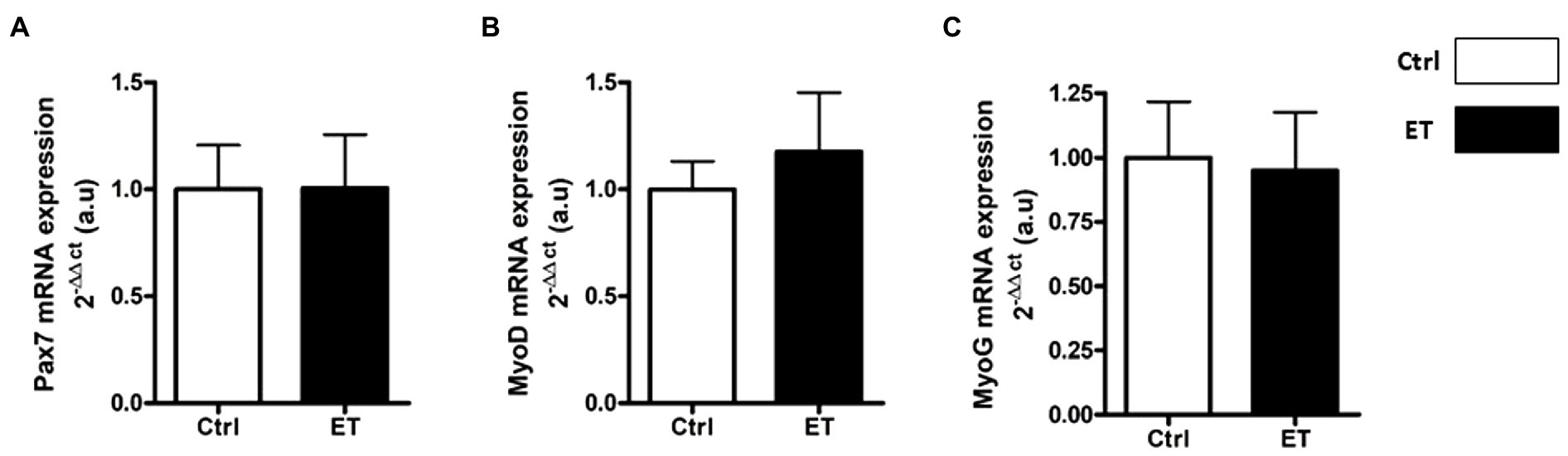
Figure 13. Ergothioneine effect on transcription regulation of satellite cell markers. Satellite cell marker transcription regulation in gastrocnemius muscle. (A) Pax7 mRNA level. (B) MyoD mRNA level. (C) Myogenin mRNA level. *p < 0.05 vs. Ctrl.
Discussion
This study evaluated the effect of 1 week of ergothioneine supplementation on time to exhaustion (treadmill running) and on the profile of early markers of muscle recovery after exercise.
At baseline, MAS was identical in Ctrl and ET mice (26.66 ± 3.32 m/min). After 1 week of supplementation, time to exhaustion was 41.22% longer in the ET than Ctrl group (tested at 70% of the individual MAS), thus supporting our hypothesis that ergothioneine might increase performance in exhaustive aerobic exercise. This result is similar to what reported using antioxidant molecules, such as N-acetylcysteine (McKenna et al., 2006), resveratrol (Wu et al., 2013), and natural food extracts that contain antioxidants (Huang et al., 2012; Wang et al., 2012).
Following exhaustive exercise, the expression of oxidative stress and oxidative damage markers in muscle increases in function of the exercise intensity (Merle et al., 2019) and duration (Vezzoli et al., 2016; Thirupathi et al., 2021). In our study, exercise-induced oxidative damage (4HNE, oxidized proteins and p53 phosphorylation on Ser15) in muscle was similar in ET and Ctrl mice, despite the longer intense effort performed by ET animals that should have led to higher RONS production (Fisher-Wellman and Bloomer, 2009). Similarly, the protein and mRNA levels of factors implicated in the enzymatic antioxidant defenses were similar between groups. However, Nrf2 mRNA expression was upregulated in gastrocnemius in the ET group, in agreement with the study by Hseu et al. (2015) that investigated ergothioneine role as NRF2 stimulator and confirmed a greater REDOX management. However, one of the limitations of our study was the absence of a control group that did not allow determining the extent of exercise-induced RONS production and its time course (Michailidis et al., 2007). On the other hand, exercise-induced inflammatory response markers (Tnfα, Il1β, and Il6 mRNA) were lower in the ET than Ctrl group as well as IL-1β protein content in soleus. This finding could be explained not only by ergothioneine anti-inflammatory properties (Cheah et al., 2017) but also by a lower metabolic stress induced by exercise (Pagano et al., 2014; Merle et al., 2019), suggesting a better adaptation to exhaustive effort in the ET group. Indeed, the metabolic stressor sensor AMPKα was less activated in the ET than Ctrl group, reflecting a less depleted energy state. GSK3 phosphorylation also was decreased in the ET group, which results in lower glycogen synthase activation (Rayasam et al., 2009). These findings suggest that exercise-induced metabolic stress was less important in the ET group, despite their longer physical effort. More studies are needed to precisely understand ergothioneine role in metabolic stress.
Many studies have investigated the use of antioxidants and anti-inflammatory drugs for muscle recovery after physical exercise and found that they inhibit mitochondrial adaptations (Gomez-Cabrera et al., 2008; Merry and Ristow, 2016). Here, 2 h after exercise, we found that PGC1α protein level (the main mitochondriogenesis marker) was comparable in ET and Ctrl mice as well as Pgc1α, Nrf1, and Tfam mRNA expression. This suggests that ergothioneine did not impair early mitochondrial adaptations, unlike primary antioxidants, such as vitamin C and E (Gomez-Cabrera et al., 2015). The comparable COX IV, cytochrome C and citrate synthase protein levels in muscles between groups indicates that 1 week of ergothioneine supplementation did not affect mitochondrial content and capacity. Therefore, ergothioneine may increase time to exhaustion mainly by regulating REDOX balance and by managing muscle fatigue, rather than by increasing the muscle oxidative capacity. More studies are needed to test this hypothesis.
Several studies reported that during muscle recovery, primary antioxidants slow down the protein synthesis pathway. Two hours after exercise, protein synthesis markers were upregulated in the ET group compared with Ctrl, despite the longer effort of ET mice that might decrease protein synthesis (Merle et al., 2019). This is consistent with the lower metabolic stress observed in ET muscles, because such stress normally delays protein synthesis activation after physical activity (MacDougall et al., 1995; Bolster et al., 2002; Dreyer et al., 2006; Thomson, 2018). This finding also indicates that ergothioneine antioxidant action did not impair early protein synthesis after exercise, unlike primary antioxidants (Pagano et al., 2014; Bjørnsen et al., 2016). Conversely, expression of UPS and autophagy markers, the two main pathways involved in protein degradation after exercise, was comparable between groups. As autophagy and UPS are redox-sensitive pathways, this finding confirms that the REDOX status in the ET group was not impaired despite the longer exercise (Powers et al., 2016). On the other hand, Parkin mRNA expression (a mitochondrial degradation marker) was downregulated in the ET group, possibly suggesting a protective effect of ergothioneine against mitochondrial RONS production (Barodia et al., 2017).
Finally, assessment of muscle regeneration through quantification of muscle satellite cell markers showed higher PAX7 and Spry-1 protein levels in the ET group. This may suggest a greater pool of quiescent satellite cells and promotion of their asymmetrical division (Shea et al., 2010; Dumont et al., 2015). MyoD and Myogenin expression levels (two satellite cell activation markers) also were increased in the ET group suggesting a better regeneration activation. As RONS promotes MyoD and Myogenin activation (Anderson, 2000; Moal et al., 2017), this finding may indicate that ergothioneine did not inhibit RONS beneficial effects on muscle regeneration through satellite cell activity. Moreover, ergothioneine intake might favor satellite cell asymmetrical division and thus the maintenance of the satellite cell pool (Troy et al., 2012). In our experimental conditions, our findings suggest that this effect of ergothioneine may be greater in soleus muscle through Myogenin upregulation after exercise (Snijders et al., 2015), but more data are needed to validate this hypothesis. MyoD and Myogenin increased expression is also in accordance with NRF2 function in satellite cells (Dai et al., 2020; Kourakis et al., 2020). Indeed, NRF2 is needed to maintain PAX7 and MyoD expression in muscle (Narashimhan et al., 2014) and to promote satellite cell proliferation and differentiation (Murakami and Motohashi, 2015).
Conclusion
To conclude, ergothioneine supplementation significantly improved time-to-exhaustion in mice. Moreover, the post-exercise inflammatory response and metabolic stress were less important in the ET group despite the longer exercise time. Ergothioneine also slightly improved early protein synthesis and did not impair mitochondrial recovery. Moreover, ergothioneine promoted the quiescent pool maintenance and activation after exercise. These results suggest that ergothioneine could help to better manage exercise-induced muscle damage and recovery. In addition, ergothioneine anti-inflammatory and antioxidant effects could be interesting for limiting muscle deconditioning related to hypoactivity or ageing. Ergothioneine might be useful also for the management of diseases in which inflammatory and oxidative stress play a major role, such as chronic obstructive pulmonary disease and type 2 diabetes.
Data Availability Statement
The original contributions presented in the study are included in the article/supplementary material, further inquiries can be directed to the corresponding authors.
Ethics Statement
The animal study was reviewed and approved by the Languedoc-Roussillon Ethics Committee (APAFIS#28764-2020122115407491).
Author Contributions
TF: study design, experimentation, analysis, writing, and validation. CG, PD, GP, and AC: experimentation and validation. TB: study design, experimentation, writing, and validation. All authors contributed to the article and approved the submitted version.
Funding
This study was funded by the French Centre National d’Etudes Spatiales (CNES), 4800000797.
Conflict of Interest
The authors declare that the research was conducted in the absence of any commercial or financial relationships that could be construed as a potential conflict of interest.
Publisher’s Note
All claims expressed in this article are solely those of the authors and do not necessarily represent those of their affiliated organizations, or those of the publisher, the editors and the reviewers. Any product that may be evaluated in this article, or claim that may be made by its manufacturer, is not guaranteed or endorsed by the publisher.
Acknowledgments
We thank Biocampus (Montpellier, France), INM-MRI (Montpellier, France), and the RHEM (Montpellier, France) facilities for technical support. A special thanks to Tetrahedron (Paris, France) for providing pure ergothioneine and their expertise on this molecule.
References
Anderson, J. E. (2000). A role for nitric oxide in muscle repair: nitric oxide–mediated activation of muscle satellite cells. Mol. Biol. Cell 11, 1859–1874. doi: 10.1091/mbc.11.5.1859
Arc-Chagnaud, C., Py, G., Fovet, T., Roumanille, R., Demangel, R., Pagano, A. F., et al. (2020). Evaluation of an antioxidant and anti-inflammatory cocktail against human Hypoactivity-induced skeletal muscle deconditioning. Front. Physiol. 11:71. doi: 10.3389/fphys.2020.00071
Aruoma, O. I., Spencer, J. P. E., and Mahmood, N. (1999). Protection against oxidative damage and cell death by the natural antioxidant ergothioneine. Food Chem. Toxicol. 37, 1043–1053. doi: 10.1016/S0278-6915(99)00098-8
Baltaci, S. B., Mogulkoc, R., and Baltaci, A. K. (2016). Resveratrol and exercise. Biomed. Rep. 5, 525–530. doi: 10.3892/br.2016.777
Barodia, S. K., Creed, R. B., and Goldberg, M. S. (2017). Parkin and Pink1 functions in oxidative stress and neurodegeneration. Brain Res. Bull. 133, 51–59. doi: 10.1016/j.brainresbull.2016.12.004
Bassel-Duby, R., and Olson, E. N. (2006). Signaling pathways in skeletal muscle remodeling. Annu. Rev. Biochem. 75, 19–37. doi: 10.1146/annurev.biochem.75.103004.142622
Bjørnsen, T., Salvesen, S., Berntsen, S., Hetlelid, K. J., Stea, T. H., Lohne-Seiler, H., et al. (2016). Vitamin C and E supplementation blunts increases in total lean body mass in elderly men after strength training. Scand. J. Med. Sci. Sports 26, 755–763. doi: 10.1111/sms.12506
Bolster, D. R., Crozier, S. J., Kimball, S. R., and Jefferson, L. S. (2002). AMP-activated protein kinase suppresses protein synthesis in rat skeletal muscle through down-regulated mammalian target of rapamycin (MTOR) signaling. J. Biol. Chem. 277, 23977–23980. doi: 10.1074/jbc.C200171200
Braakhuis, A. J., and Hopkins, W. G. (2015). Impact of dietary antioxidants on sport performance: a review. Sports Med. 45, 939–955. doi: 10.1007/s40279-015-0323-x
Cheah, I. K., and Halliwell, B. (2012). Ergothioneine; antioxidant potential, physiological function and role in disease. Biochim. Biophys. Acta 1822, 784–793. doi: 10.1016/j.bbadis.2011.09.017
Cheah, I. K., Tang, R. M. Y., Yew, T. S. Z., Lim, K. H. C., and Halliwell, B. (2017). Administration of pure ergothioneine to healthy human subjects: uptake, metabolism, and effects on biomarkers of oxidative damage and inflammation. Antioxid. Redox Signal. 26, 193–206. doi: 10.1089/ars.2016.6778
Crilly, M. J., Tryon, L. D., Erlich, A. T., and Hood, D. A. (2016). The role of Nrf2 in skeletal muscle contractile and mitochondrial function. J. Appl. Physiol. 121, 730–740. doi: 10.1152/japplphysiol.00042.2016
Dai, X., Yan, X., Wintergerst, K. A., Cai, L., Keller, B. B., and Tan, Y. (2020). Nrf2: redox and metabolic regulator of stem cell state and function. Trends Mol. Med. 26, 185–200. doi: 10.1016/j.molmed.2019.09.007
Dreyer, H. C., Fujita, S., Cadenas, J. G., Chinkes, D. L., Volpi, E., and Rasmussen, B. B. (2006). Resistance exercise increases AMPK activity and reduces 4E-BP1 phosphorylation and protein synthesis in human skeletal muscle. J. Physiol. 576, 613–624. doi: 10.1113/jphysiol.2006.113175
Dumont, N. A., Wang, Y. X., and Rudnicki, M. A. (2015). Intrinsic and extrinsic mechanisms regulating satellite cell function. Development 142, 1572–1581. doi: 10.1242/dev.114223
Dutra, M. T., Alex, S., Mota, M. R., Sales, N. B., Brown, L. E., and Bottaro, M. (2018). Effect of strength training combined with antioxidant supplementation on muscular performance. Appl. Physiol. Nutr. Metab. 43, 775–781. doi: 10.1139/apnm-2017-0866
Faden, F., Eschen-Lippold, L., and Dissmeyer, N. (2016). Normalized quantitative Western blotting based on standardized fluorescent labeling. Methods Mol. Biol. 1450, 247–258. doi: 10.1007/978-1-4939-3759-2_20
Fisher-Wellman, K., and Bloomer, R. J. (2009). Acute exercise and oxidative stress: a 30 year history. Dyn. Med. 8:1. doi: 10.1186/1476-5918-8-1
Gilda, J. E., and Gomes, A. V. (2015). Western blotting using in-gel protein labeling as a normalization control: stain-free technology. Methods Mol. Biol. 1295, 381–391. doi: 10.1007/978-1-4939-2550-6_27
Gomez-Cabrera, M.-C., Domenech, E., Romagnoli, M., Arduini, A., Borras, C., Pallardo, F. V., et al. (2008). Oral administration of vitamin C decreases muscle mitochondrial biogenesis and hampers training-induced adaptations in endurance performance. Am. J. Clin. Nutr. 87, 142–149. doi: 10.1093/ajcn/87.1.142
Gomez-Cabrera, M. C., Salvador-Pascual, A., Cabo, H., Ferrando, B., and Viña, J. (2015). Redox modulation of mitochondriogenesis in exercise. Does antioxidant supplementation blunt the benefits of exercise training? Free Radic. Biol. Med. 86, 37–46. doi: 10.1016/j.freeradbiomed.2015.04.006
Gouraud, E., Charrin, E., Dubé, J. J., Ofori-Acquah, S. F., Martin, C., Skinner, S., et al. (2019). Effects of individualized treadmill endurance training on oxidative stress in skeletal muscles of transgenic sickle mice. Oxid. Med. Cell. Longev. 2019, 1–9. doi: 10.1155/2019/3765643
Halliwell, B., Cheah, I. K., and Drum, C. L. (2016). Ergothioneine, an adaptive antioxidant for the protection of injured tissues? A hypothesis. Biochem. Biophys. Res. Commun. 470, 245–250. doi: 10.1016/j.bbrc.2015.12.124
Hseu, Y.-C., Lo, H.-W., Korivi, M., Tsai, Y.-C., Tang, M.-J., and Yang, H.-L. (2015). Dermato-protective properties of ergothioneine through induction of Nrf2/ARE-mediated antioxidant genes in UVA-irradiated human keratinocytes. Free Radic. Biol. Med. 86, 102–117. doi: 10.1016/j.freeradbiomed.2015.05.026
Huang, C.-C., Hsu, M.-C., Huang, W.-C., Yang, H.-R., and Hou, C.-C. (2012). Triterpenoid-rich extract from Antrodia Camphorata improves physical fatigue and exercise performance in mice. Evid. Based Complement. Alternat. Med. 2012:364741. doi: 10.1155/2012/364741
Kang, C., O’Moore, K. M., Dickman, J. R., and Ji, L. L. (2009). Exercise activation of muscle peroxisome proliferator-activated receptor-γ coactivator-1α signaling is redox sensitive. Free Radic. Biol. Med. 47, 1394–1400. doi: 10.1016/j.freeradbiomed.2009.08.007
Kawamura, T., and Muraoka, I. (2018). Exercise-induced oxidative stress and the effects of antioxidant intake from a physiological viewpoint. Antioxidants 7:119. doi: 10.3390/antiox7090119
Knapik, J. J., Steelman, R. A., Hoedebecke, S. S., Austin, K. G., Farina, E. K., and Lieberman, H. R. (2016). Prevalence of dietary supplement use by athletes. Sports Med. 46, 103–123. doi: 10.1007/s40279-015-0387-7
Kourakis, S., Timpani, C. A., de Haan, J. B., Gueven, N., Fischer, D., and Rybalka, E. (2020). Targeting Nrf2 for the treatment of duchenne muscular dystrophy. Redox Biol. 38:101803. doi: 10.1016/j.redox.2020.101803
Lin, J., Handschin, C., and Spiegelman, B. M. (2005). Metabolic control through the PGC-1 family of transcription coactivators. Cell Metab. 1, 361–370. doi: 10.1016/j.cmet.2005.05.004
Ma, Q. (2013). Role of Nrf2 in oxidative stress and toxicity. Annu. Rev. Pharmacol. Toxicol. 53, 401–426. doi: 10.1146/annurev-pharmtox-011112-140320
MacDougall, J. D., Gibala, M. J., Tarnopolsky, M. A., MacDonald, J. R., Interisano, S. A., and Yarasheski, K. E. (1995). The time course for elevated muscle protein synthesis following heavy resistance exercise. Can. J. Appl. Physiol. 20, 480–486. doi: 10.1139/h95-038
Macera, C. A., Hootman, J. M., and Sniezek, J. E. (2003). Major public health benefits of physical activity. Arthritis Rheum. 49, 122–128. doi: 10.1002/art.10907
Malm, C., Svensson, M., Ekblom, B., and Sjödin, B. (1997). Effects of Ubiquinone-10 supplementation and high intensity training on physical performance in humans. Acta Physiol. Scand. 161, 379–384. doi: 10.1046/j.1365-201X.1997.00198.x
Margaritelis, N. V., Paschalis, V., Theodorou, A. A., Kyparos, A., and Nikolaidis, M. G. (2018a). Antioxidants in personalized nutrition and exercise. Adv. Nutr. 9, 813–823. doi: 10.1093/advances/nmy052
Margaritelis, N. V., Paschalis, V., Theodorou, A. A., Kyparos, A., and Nikolaidis, M. G. (2020). Redox basis of exercise physiology. Redox Biol. 35:101499. doi: 10.1016/j.redox.2020.101499
Margaritelis, N. V., Theodorou, A. A., Paschalis, V., Veskoukis, A. S., Dipla, K., Zafeiridis, A., et al. (2018b). Adaptations to endurance training depend on exercise-induced oxidative stress: exploiting redox Interindividual variability. Acta Physiol (Oxf.) 222:e12898. doi: 10.1111/apha.12898
Markova, N. G., Karaman-Jurukovska, N., Dong, K. K., Damaghi, N., Smiles, K. A., and Yarosh, D. B. (2009). Skin cells and tissue are capable of using L-ergothioneine as an integral component of their antioxidant defense system. Free Radic. Biol. Med. 46, 1168–1176. doi: 10.1016/j.freeradbiomed.2009.01.021
McKenna, M. J., Medved, I., Goodman, C. A., Brown, M. J., Bjorksten, A. R., Murphy, K. T., et al. (2006). N-acetylcysteine attenuates the decline in muscle Na+, K+-pump activity and delays fatigue during prolonged exercise in humans. J. Physiol. 576, 279–288. doi: 10.1113/jphysiol.2006.115352
Medved, I., Brown, M. J., Bjorksten, A. R., Murphy, K. T., Petersen, A. C., Sostaric, S., et al. (2004). N-acetylcysteine enhances muscle cysteine and glutathione availability and attenuates fatigue during prolonged exercise in endurance-trained individuals. J. Appl. Physiol. 97, 1477–1485. doi: 10.1152/japplphysiol.00371.2004
Merle, A., Jollet, M., Britto, F. A., Goustard, B., Bendridi, N., Rieusset, J., et al. (2019). Endurance exercise decreases protein synthesis and ER-mitochondria contacts in mouse skeletal muscle. J. Appl. Physiol. 127, 1297–1306. doi: 10.1152/japplphysiol.00196.2019
Merry, T. L., and Ristow, M. (2016). Do antioxidant supplements interfere with skeletal muscle adaptation to exercise training? J. Physiol. 594, 5135–5147. doi: 10.1113/JP270654
Michailidis, Y., Jamurtas, A. Z., Nikolaidis, M. G., Fatouros, I. G., Koutedakis, Y., Papassotiriou, I., et al. (2007). Sampling time is crucial for measurement of aerobic exercise-induced oxidative stress. Med. Sci. Sports Exerc. 39, 1107–1113. doi: 10.1249/01.mss.0b013e318053e7ba
Moal, L., Emmeran, V. P., Juban, G., Groussard, C., Zouhal, H., Chazaud, B., et al. (2017). Redox control of skeletal muscle regeneration. Antioxid. Redox Signal. 27, 276–310. doi: 10.1089/ars.2016.6782
Murakami, S., and Motohashi, H. (2015). Roles of Nrf2 in Cell Proliferation and Differentiation. Free Radic. Biol. Med. 88, 168–178. doi: 10.1016/j.freeradbiomed.2015.06.030
Narashimhan, M., Hong, J., Atieno, N., Muthusamy, V. R., Davidson, C. J., Abu-Rmaileh, N., et al. (2014). Nrf2 deficiency promotes apoptosis and impairs Pax7/MyoD expression in aging skeletal muscle cells. Free Radic. Biol. Med. 71, 402–414. doi: 10.1016/j.freeradbiomed.2014.02.023
Pagano, A. F., Brioche, T., Arc-Chagnaud, C., Demangel, R., Chopard, A., and Py, G. (2018). Short-term disuse promotes fatty acid infiltration into skeletal muscle. J. Cachexia. Sarcopenia Muscle 9, 335–347. doi: 10.1002/jcsm.12259
Pagano, A. F., Py, G., Bernardi, H., Candau, R. B., and Sanchez, A. M. J. (2014). Autophagy and protein turnover signaling in slow-twitch muscle during exercise. Med. Sci. Sports Exerc. 46, 1314–1325. doi: 10.1249/MSS.0000000000000237
Paschalis, V., Theodorou, A. A., Kyparos, A., Dipla, K., Zafeiridis, A., Panayiotou, G., et al. (2016). Low vitamin C values are linked with decreased physical performance and increased oxidative stress: reversal by vitamin C supplementation. Eur. J. Nutr. 55, 45–53. doi: 10.1007/s00394-014-0821-x
Paschalis, V., Theodorou, A. A., Margaritelis, N. V., Kyparos, A., and Nikolaidis, M. G. (2018). N-acetylcysteine supplementation increases exercise performance and reduces oxidative stress only in individuals with low levels of glutathione. Free Radic. Biol. Med. 115, 288–297. doi: 10.1016/j.freeradbiomed.2017.12.007
Paulsen, G., Cumming, K. T., Holden, G., Hallén, J., Rønnestad, B. R., Sveen, O., et al. (2014a). Vitamin C and E supplementation hampers cellular adaptation to endurance training in humans: a double-blind, randomised, controlled trial. J. Physiol. 592, 1887–1901. doi: 10.1113/jphysiol.2013.267419
Paulsen, G., Hamarsland, H., Cumming, K. T., Johansen, R. E., Hulmi, J. J., Børsheim, E., et al. (2014b). Vitamin C and E supplementation alters protein signalling after a strength training session, but not muscle growth during 10 weeks of training. J. Physiol. 592, 5391–5408. doi: 10.1113/jphysiol.2014.279950
Pilegaard, H., Saltin, B., and Darrell Neufer, P. (2003). Exercise induces transient transcriptional activation of the PGC-1alpha gene in human skeletal muscle. J. Physiol. 546, 851–858. doi: 10.1113/jphysiol.2002.034850
Powers, S. K., and Jackson, M. J. (2008). Exercise-induced oxidative stress: cellular mechanisms and impact on muscle force production. Physiol. Rev. 88, 1243–1276. doi: 10.1152/physrev.00031.2007
Powers, S. K., Morton, A. B., Ahn, B., and Smuder, A. J. (2016). Redox control of skeletal muscle atrophy. Free Radic. Biol. Med. 98, 208–217. doi: 10.1016/j.freeradbiomed.2016.02.021
Powers, S. K., Smuder, A. J., and Criswell, D. S. (2011). Mechanistic links between oxidative stress and disuse muscle atrophy. Antioxid. Redox Signal. 15, 2519–2528. doi: 10.1089/ars.2011.3973
Ranchordas, M. K., Rogerson, D., Soltani, H., and Costello, J. T. (2017). Antioxidants for preventing and reducing muscle soreness after exercise. Cochrane Database Syst. Rev. 2017:CD009789. doi: 10.1002/14651858.CD009789.pub2
Rayasam, G. V., Tulasi, V. K., Sodhi, R., Davis, J. A., and Ray, A. (2009). Glycogen synthase kinase 3: more than a namesake. Br. J. Pharmacol. 156, 885–898. doi: 10.1111/j.1476-5381.2008.00085.x
Reid, M. B. (2001). Invited review: redox modulation of skeletal muscle contraction: what we know and what we Don’t. J. Appl. Physiol. 90, 724–731. doi: 10.1152/jappl.2001.90.2.724
Reid, M. B. (2016). Redox interventions to increase exercise performance. J. Physiol. 594, 5125–5133. doi: 10.1113/JP270653
Rhodes, K., and Braakhuis, A. (2017). Performance and side effects of supplementation with N-acetylcysteine: a systematic review and meta-analysis. Sports Med. 47, 1619–1636. doi: 10.1007/s40279-017-0677-3
Ristow, M., Zarse, K., Oberbach, A., Klöting, N., Birringer, M., Kiehntopf, M., et al. (2009). Antioxidants prevent health-promoting effects of physical exercise in humans. Proc. Natl. Acad. Sci. U. S. A. 106, 8665–8670. doi: 10.1073/pnas.0903485106
Schiaffino, S., Dyar, K. A., Ciciliot, S., Blaauw, B., and Sandri, M. (2013). Mechanisms regulating skeletal muscle growth and atrophy. FEBS J. 280, 4294–4314. doi: 10.1111/febs.12253
Schnohr, P., O’Keefe, J. H., Marott, J. L., Lange, P., and Jensen, G. B. (2015). Dose of jogging and long-term mortality: The Copenhagen City Heart Study. J. Am. Coll. Cardiol. 65, 411–419. doi: 10.1016/j.jacc.2014.11.023
Shea, K. L., Xiang, W., LaPorta, V. S., Licht, J. D., Charles Keller, M., Basson, A., et al. (2010). Sprouty1 regulates reversible quiescence of a self-renewing adult muscle stem cell Pool during regeneration. Cell Stem Cell 6, 117–129. doi: 10.1016/j.stem.2009.12.015
Silveira, L. R., Pilegaard, H., Kusuhara, K., Curi, R., and Hellsten, Y. (2006). The contraction induced increase in gene expression of peroxisome proliferator-activated receptor (PPAR)-γ coactivator 1α (PGC-1α), mitochondrial uncoupling protein 3 (UCP3) and hexokinase II (HKII) in primary rat skeletal muscle cells is dependent on reactive oxygen species. Biochim. Biophys. Acta 1763, 969–976. doi: 10.1016/j.bbamcr.2006.06.010
Snijders, T., Nederveen, J. P., McKay, B. R., Joanisse, S., Verdijk, L. B., van Loon, L. J. C., et al. (2015). Satellite cells in human skeletal muscle plasticity. Front. Physiol. 6:283. doi: 10.3389/fphys.2015.00283
Strobel, N. A., Peake, J. M., Matsumoto, A., Marsh, S. A., Coombes, J. S., and Wadley, G. D. (2011). Antioxidant supplementation reduces skeletal muscle mitochondrial biogenesis. Med. Sci. Sports Exerc. 43, 1017–1024. doi: 10.1249/MSS.0b013e318203afa3
Sureda, A., Ferrer, M. D., Tauler, P., Romaguera, D., Drobnic, F., Pujol, P., et al. (2009). Effects of exercise intensity on lymphocyte H2O2 production and antioxidant Defences in soccer players. Br. J. Sports Med. 43, 186–190. doi: 10.1136/bjsm.2007.043943
Tang, R. M. Y., Cheah, I. K.-M., Yew, T. S. K., and Halliwell, B. (2018). Distribution and accumulation of dietary ergothioneine and its metabolites in mouse tissues. Sci. Rep. 8:1601. doi: 10.1038/s41598-018-20021-z
Thirupathi, A., Pinho, R. A., Ugbolue, U. C., He, Y., Meng, Y., and Yaodong, G. (2021). Effect of running exercise on oxidative stress biomarkers: a systematic review. Front. Physiol. 11:610112. doi: 10.3389/fphys.2020.610112
Thomson, D. M. (2018). The role of AMPK in the regulation of skeletal muscle size, hypertrophy, and regeneration. Int. J. Mol. Sci. 19:3125. doi: 10.3390/ijms19103125
Troy, A., Cadwallader, A. B., Fedorov, Y., Tyner, K., Tanaka, K. K., and Olwin, B. B. (2012). Coordination of satellite cell activation and self-renewal by par-complex-dependent asymmetric activation of P38α/β MAPK. Cell Stem Cell 11, 541–553. doi: 10.1016/j.stem.2012.05.025
Vezzoli, A., Dellanoce, C., Mrakic-Sposta, S., Montorsi, M., Moretti, S., Tonini, A.,, et al. (eds.) (2016). Oxidative stress assessment in response to ultraendurance exercise: thiols redox status and ROS production according to duration of a competitive race. Oxid. Med. Cell. Longev. 2016:e6439037. doi: 10.1155/2016/6439037
Vigelsø, A., Dybboe, R., Hansen, C. N., Dela, F., Helge, J. W., and Grau, A. G. (2015). GAPDH and β-actin protein decreases with aging, making stain-free technology a superior loading control in Western blotting of human skeletal muscle. J. Appl. Physiol. 118, 386–394. doi: 10.1152/japplphysiol.00840.2014
Walle, T., Hsieh, F., DeLegge, M. H., Oatis, J. E., and Kristina Walle, U. (2004). High absorption but very low bioavailability of oral resveratrol in humans. Drug Metab. Dispos. 32, 1377–1382. doi: 10.1124/dmd.104.000885
Wang, S.-Y., Huang, W.-C., Liu, C.-C., Wang, M.-F., Ho, C.-S., Huang, W.-P., et al. (2012). Pumpkin (Cucurbita Moschata) fruit extract improves physical fatigue and exercise performance in mice. Molecules 17, 11864–11876. doi: 10.3390/molecules171011864
Wang, X., and Proud, C. G. (2006). The mTOR pathway in the control of protein synthesis. Physiology 21, 362–369. doi: 10.1152/physiol.00024.2006
Wang, J.-S., Tan, L., and Chow, S.-E. (2006). Role of exercise intensities in oxidized low-density lipoprotein-mediated redox status of monocyte in men. J. Appl. Physiol. 101, 740–744. doi: 10.1152/japplphysiol.00144.2006
Warburton, D. E. R., Nicol, C. W., and Bredin, S. S. D. (2006). Health benefits of physical activity: the evidence. CMAJ. Can. Med. Assoc. J. 174, 801–809. doi: 10.1503/cmaj.051351
Keywords: ergothioneine, muscle, exercise, antioxidant, exercise performance, exercise recovery
Citation: Fovet T, Guilhot C, Delobel P, Chopard A, Py G and Brioche T (2022) Ergothioneine Improves Aerobic Performance Without Any Negative Effect on Early Muscle Recovery Signaling in Response to Acute Exercise. Front. Physiol. 13:834597. doi: 10.3389/fphys.2022.834597
Edited by:
Mahdi Garelnabi, University of Massachusetts Lowell, United StatesReviewed by:
Nikos Margaritelis, Aristotle University of Thessaloniki, GreeceZhi Xia, Chengdu Sport University, China
Emmanuelle Charrin, Karolinska Institutet (KI), Sweden
Copyright © 2022 Fovet, Guilhot, Delobel, Chopard, Py and Brioche. This is an open-access article distributed under the terms of the Creative Commons Attribution License (CC BY). The use, distribution or reproduction in other forums is permitted, provided the original author(s) and the copyright owner(s) are credited and that the original publication in this journal is cited, in accordance with accepted academic practice. No use, distribution or reproduction is permitted which does not comply with these terms.
*Correspondence: Théo Fovet, dGhlby5mb3ZldEB1bW9udHBlbGxpZXIuZnI=
Thomas Brioche, dGhvbWFzLmJyaW9jaGVAdW1vbnRwZWxsaWVyLmZy