- 1Laboratory of Nutrition, Physical Activity and Phenotypic Plasticity, Department of Physical Education and Sport Sciences, Universidade Federal de Pernambuco UFPE, Vitória de Santo Antão, Brazil
- 2Department of Physiology and Pharmacology, Universidade Federal de Pernambuco UFPE, Recife, Brazil
In recent decades, the high incidence of infectious and parasitic diseases has been replaced by a high prevalence of chronic and degenerative diseases. Concomitantly, there have been profound changes in the behavior and eating habits of families around the world, characterizing a “nutritional transition” phenomenon, which refers to a shift in diet in response to modernization, urbanization, or economic development from undernutrition to the excessive consumption of hypercaloric and ultra-processed foods. Protein malnutrition that was a health problem in the first half of the 20th century has now been replaced by high-fat diets, especially diets high in saturated fat, predisposing consumers to overweight and obesity. This panorama points us to the alarming coexistence of both malnutrition and obesity in the same population. In this way, individuals whose mothers were undernourished early in pregnancy and then exposed to postnatal hyperlipidic nutrition have increased risk factors for developing metabolic dysfunction and cardiovascular diseases in adulthood. Thus, our major aim was to review the cardiometabolic effects resulting from postnatal hyperlipidic diets in protein-restricted subjects, as well as to examine the epigenetic repercussions occasioned by the nutritional transition.
Introduction
In the second half of the 20th century, a change in the dietary habits of the population, mainly in the western part of the world, was observed, called a phenomenon of “nutrition transition” (Hasan et al., 2016; Herran et al., 2016). The nutritional transition was characterized by a reduction in the prevalence of malnutrition in its various dimensions (energy and macro- or micronutrients), accompanied by excessive consumption of hypercaloric and ultra-processed foods, with a consequent increase in body weight (Abdullah, 2015), a high incidence of chronic diseases, and a high prevalence of obesity (Batista Filho and Rissin, 2003a; Batista Filho and Batista, 2010; Ribeiro et al., 2015). Concomitantly, it was possible to observe profound changes in the behavior and eating habits of families around the world (Popkin, 2015). Higher levels of undernutrition have been replaced by higher rates of overweight and obesity related to hyperlipidic and hypercaloric food consumption (Di Pietro et al., 2015). Epidemiological evidence has shown that nutritional deficiency in the first years of life accompanied by overnutrition, a posteriori, may increase the risk of dyslipidemia and other cardiometabolic diseases in adulthood, such as hypertension and type 2 diabetes (Figure 1) (Barker, 2007). In this context, individuals subjected to maternal protein undernutrition in utero have been considered those with a high risk of developing cardiometabolic dysfunctions in adulthood (Ashton, 2000; Hemachandra et al., 2006; Antony and Laxmaiah, 2008; Conde and Monteiro, 2014; Costa-Silva et al., 2015; Parra et al., 2015). Furthermore, studies have suggested that when these individuals are additionally subjected to inappropriate postnatal nutrition, especially hyperlipidic diets, they may significantly suffer heightened energy balance dysfunctions in adulthood (Desai et al., 2007). Thus, the eating habits and nutritional conditions in early phases of life play a key role in the etiology of these diseases by inducing physiological dysfunctions (Lucas, 1998; Victora et al., 2008; Wells, 2012). Many studies have suggested that external environmental inputs, such as nutrition, may modify the phenotype, leading to physiological adaptations without genetic changes. This phenomenon can be understood in the context of phenotypic plasticity. (West-Eberhard, 2003).
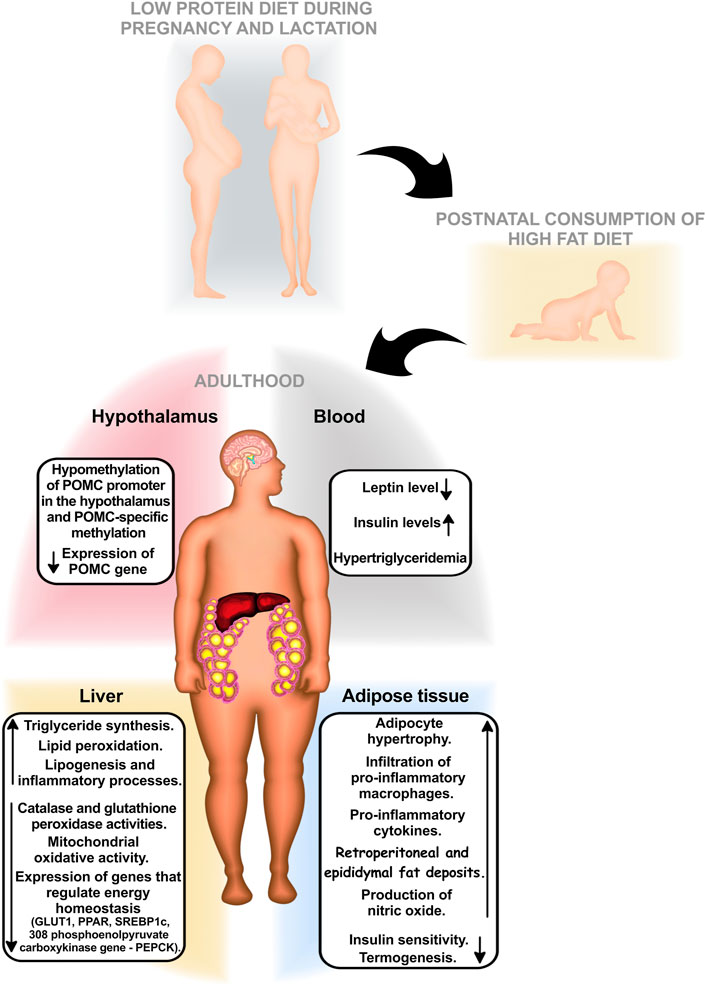
FIGURE 1. Diets low in protein in the first years of life, followed by high consumption of high-fat diets, can increase the risk of cardiometabolic diseases in adulthood, such as hypertension, dyslipidemia, and type 2 diabetes.
Phenotypic plasticity is molecularly based on epigenetic changes, such as DNA methylation, post-translational histone modifications, and microRNA expression (Wells, 2011). The epigenetic landscape was first described by Conrad Waddington in 1940 who studied the relationship between the cause and effect in genes to produce a phenotype (Jablonka and Lamb, 2002). Currently, this concept is used for the process of gene expression and its link to modifications in the chromatin structure without altering the DNA sequence (Chong and Whitelaw, 2004; Egger et al., 2004). Changes in the chromatin structure are related to the increase or decrease in the electrostatic affinity of the DNA structure. DNA methylation, post-translational modifications of histones, and expression of microRNAs are capable of altering a chromatin structure (Sadakierska-Chudy and Filip, 2015). DNA methylation is related to the addition of methyl groups to DNA cytosine residues, usually cytosine, followed by the guanine residue (CpG dinucleotides), which can produce inhibition of gene expression by impairing transcription factor binding (Waterland and Michels, 2007; Mansego et al., 2013; Chango and Pogribny, 2015; Mitchell et al., 2016). As post-translational histone modifications (acetylation, methylation, ubiquitination, and phosphorylation) correspond to the addition of methyl, acetyl, or other groups to the histone tails, they increase or decrease the electrostatic affinity between histone proteins and DNA, thus promoting a structure of chromatin that is more or less permissive to gene transcription (Bowman and Poirier, 2015). The addition of acetyl groups to histones is regulated by the action of histone acetyltransferases (HATs), while the removal of acetyl groups is catalyzed by histone deacetylases (HDACs) (Graff and Tsai, 2013). MicroRNAs are small endogenous non-coding RNA molecules involved in gene regulation and function in protein-coding introns, non-coding gene introns, or non-coding gene exons; they have been implicated in many cellular processes, including proliferation, apoptosis, differentiation, senescence, and responses to stress and immunological stimuli (D'Ippolito and Iorio, 2013).
This provides the basis for an investigation on how the nutritional aspect can induce these epigenetic changes. The hypothesis is that epigenetic modifications are an extended mechanism that links maternal nutrition to the modulation of phenotypes in the offspring (Mazzio E. A. and Soliman K. F. A., 2014; Szarc vel Szic et al., 2015). Thus, this review will address the main cardiometabolic effects elicited by postnatal hyperlipidic diets in protein-restricted subjects during pregnancy and lactation.
Maternal Protein Undernutrition and Development of Cardiometabolic Diseases
Developmental origins of health and diseases as proposed by Barker and colleagues in 1986 have been extensively studied as physiological consequences of perinatal nutritional factors (Barker and Osmond, 1986; Barker et al., 1989; Barker et al., 1993; Barker, 2007). This field of research proposes that cardiometabolic diseases can be “programmed” by the “adaptive” effects of both under- and overnutrition during early phases of growth and development, changing the cell physiology in the phenotype but without altering the genotype (Barker et al., 2005; West-Eberhard, 2005; Labayen et al., 2006; Andersen et al., 2009; Biosca et al., 2011). In this context, phenotypic plasticity has molecular basis, epigenetic alterations such as DNA methylation, histone acetylation, and microRNA expression (Wells, 2011). These epigenetic marks are established early in development and can persist for a lengthy period of time (John and Rougeulle, 2018).
Epigenetic modifications are widely hypothesized to be an overarching mechanism linking maternal nutrition to metabolic health phenotypes in the offspring (Mazzio EA. and Soliman KF., 2014; Szarc vel Szic et al., 2015). In this context, a low-protein diet (8% protein) during gestation and lactation has been associated with growth restriction, asymmetric reduction in organ growth, elevated systolic blood pressure, dyslipidemia, and increased fasting plasma insulin concentrations in most studies on rat offspring (Ozanne and Hales, 2004; Costa-Silva et al., 2009; Falcao-Tebas et al., 2012; Leandro et al., 2012; Fidalgo et al., 2013; de Brito Alves et al., 2014; Ferreira et al., 2015; de Brito Alves et al., 2016; Paulino-Silva and Costa-Silva, 2016; Barbosa et al., 2020). However, it is known that the magnitude of the cardiovascular and metabolic outcomes is dependent on both time exposure to a protein restricted-diet (Zohdi et al., 2012; Zohdi et al., 2014) and the growth trajectory throughout the postnatal period (Wells, 2007; Wells, 2011).
A relationship between maternal protein restriction, sympathetic overactivity, and hypertension has been suggested (Johansson et al., 2007; Franco et al., 2008; Barros et al., 2015). Currently, it is well accepted that perinatal protein malnutrition (6–9% protein) raises the risks of hypertension by mechanisms that have been shown to include abnormal vascular function in the adult rat male (Brawley et al., 2003; Franco et al., 2008), altered nephron morphology and function, and stimulation of the renin-angiotensin system in the adult rat male (Nuyt and Alexander, 2009; Siddique et al., 2014) as well as disruption in respiratory control in the rat male at 30 and 90 days of life (Chen et al., 2010; de Brito Alves et al., 2014; Barros et al., 2015; Costa-Silva et al., 2015; Paulino-Silva and Costa-Silva, 2016; de Brito Alves and Costa-Silva, 2018). Offspring from dams subjected to perinatal protein restriction had relevant short-term effects on the carotid body (CB) and sensitivity and respiratory functions as well as enhanced baseline sympathetic activity and amplified ventilatory and sympathetic responses to peripheral chemoreflex activation, prior to the establishment of hypertension (de Brito Alves et al., 2014; de Brito Alves et al., 2015). The mechanism involved in these effects seems to be linked with upregulation of the hypoxic inducible factor (HIF-1α) in the CB peripheral chemoreceptors (Ito et al., 2011; Ito et al., 2012; de Brito Alves et al., 2015; Nogueira et al., 2018). Studies showed that CB peripheral chemoreceptors in malnourished offspring were responsible for the enhanced respiratory frequency and CO2 chemosensitivity in early life and the production of autonomic imbalance and the development of hypertension in adulthood (Nogueira et al., 2018). In addition, it was demonstrated that these cardiorespiratory disruptions observed in offspring were attenuated from mothers who performed physical activity during the perinatal period (Nogueira et al., 2019). Regarding epigenetics mechanisms involved in cardiometabolic effects elicited by protein malnutrition, it was described as a decrease in the methylation at various positions of the ACE-1 promoter region in rat brain and an increase in transcription of this gene involved in the renin-angiotensin system and the maintenance of arterial blood pressure (Goyal et al., 2010). Similarly, in humans, the DNA methylation in the ACE gene promoter of peripheral blood leukocytes seems to be significantly lower in children of age ranging from 6 to 12 years born with low birth weight, resulting in greater ACE activity (Ajala et al., 2012; Rangel et al., 2014).
Maternal protein restriction affects insulin sensitivity in the offspring. Previous studies found that in rats, maternal protein restriction throughout pregnancy and lactation induced insulin resistance in the male offspring (Zambrano et al., 2006; Berends et al., 2018). This central insulin resistance is related to reduced protein levels of the p110β subunit of phosphoinositide 3-kinase (PI3K) and increased serine phosphorylation of IRS-1 in the arcuate nucleus (ARC) of the hypothalamus. The expression of the gene encoding protein tyrosine phosphatase 1B (PTP1B; Ptpn1) was also increased in the region of the hypothalamus (Berends et al., 2018); the mechanism appeared to increase insulin receptor signaling mediated by protein kinase C (PKC)-ζ in skeletal muscle of the offspring of rats fed with a low-protein diet during pregnancy and lactation (Chen et al., 2009). The ability of skeletal muscle to respond to maternal protein restriction is an adaptation to optimize the use of nutrients available during the life-span, and an important response in this process is the activation of genes that ameliorate or compensate for protein deficit by stimulating the expression of glucose transporters and glycolytic and lipolytic enzymes that attenuate the altered function of the mitochondria (da Silva Aragao et al., 2014; Claycombe et al., 2015). At 30 days, the transcriptional key enzymes of the glycolytic pathway were downregulated in extensor digitorum longus muscle in offspring. However, these effects were only observed at 90 days in soleus muscle of rats subjected to protein maternal undernutrition. PDK4 was the enzyme that was more affected. One important finding was that the observed acute (30 days) transcriptional changes did not remain in adult LP rats (90 days), except for PDK4. The robust PDK4 mRNA downregulation, observed in both soleus and EDL, at both ages, and the consequent downregulation of the PDK4 protein expression can be responsible for a state of reduced metabolic flexibility of skeletal muscle in response to maternal low-protein diet (de Brito Alves et al., 2017).
Studies in rodents subjected to perinatal protein malnutrition have observed an impact on liver function with the suppression of gluconeogenesis by a mechanism mediated mainly by the decrease in the level of hepatic phosphoenolpyruvate carboxykinase mRNA (Toyoshima et al., 2010), increase in blood cholesterol and triglycerides in the offspring at 110 days of life, and reduced gene expression for the glucokinase (GCK) enzyme, the glucose sensor in the liver, impairing the detection of glucose levels (Sosa-Larios et al., 2017); these effects have been accompanied by DNA hypomethylation and increased expression of genes involved in lipid metabolism (Radford et al., 2014) and hypomethylation of glucocorticoid receptors and PPAR-α promoters, which conditioned changes in the expression of their target genes (Sandovici et al., 2011); at 21 days, the mice showed a reduction in the microRNAs, namely, mmu-miR-615, mmu-miR-124, mmu-miR-376b, and mmu-let-7e, while mmu-miR-708 and mmu-miR-879 were increased after microarray analysis; bioinformatics analysis showed that target genes were mapped to inflammatory pathways, accompanied by elevation of serum levels of tumor necrosis factor-α (TNF-α) (Zheng et al., 2017).
Postnatal Overnutrition and Development of Cardiometabolic Diseases in Maternal Protein-Restricted Subjects
Nutritional transition is a phenomenon well documented in developing countries in the 20th and 21st centuries and has induced a high incidence of the chronic diseases and a high prevalence of obesity (Batista Filho and Rissin, 2003b; Batista Filho and Batista, 2010; Ribeiro et al., 2015; Leocádio et al., 2021). It is evident that protein malnutrition was a health problem in the first half of the 20th century. Now, this has been replaced by a diet enriched in saturated fat or other high-fat diets, predisposing the population to overweight and obesity (Batista et al., 2013). Nowadays, it is suggested that two billion people in the world are overweight and obese individuals, with a major prevalence related to diet-induced obesity, which has been associated with cardiovascular and endocrine dysfunctions (Hotamisligil, 2006; Aubin et al., 2008; Zhang et al., 2012; Ng et al., 2014; Wensveen et al., 2015).
In the late 1980s–1990s, Barker et al. provided epidemiologic evidence of the programming of offspring metabolic syndrome, demonstrating that low birth weight was a significant predictor of adult obesity, diabetes, and cardiovascular disease, for promoting changes in the fetal environment, which can trigger genetic alterations and reflect on the maturation of fetal organs and systems (Barker, 1999; Barker, 2007). In a recent global survey conducted in 30 low-income countries, Abdullah (2015) related that the pattern of body weight gain of the population of developing countries is almost identical to that found in those countries and calculated the estimate of overweight over time, being higher in groups with less and less education. The coexistence of malnourished children and obese mothers in the same residence is a reality in Mexico (Kroker-Lobos et al., 2014), Colombia (Sarmiento et al., 2014), China (Feng et al., 2015), sub-Saharan Africa (Steyn and McHiza, 2014), and also in Brazil (Sousa et al., 2016). Short stature and obesity may reflect malnutrition and a poor quality diet in the first 2 years of life, followed by excess energy intake later in childhood (Abdullah, 2015).
Some studies have tried to mimic the nutritional transition, with peri- and postnatal nutritional mismatch, producing animal models that are based on maternal protein restriction during pregnancy and/or lactation, followed by the consumption of high-fat diets by the offspring, after weaning. Epidemiological studies have demonstrated that low birth weight makes individuals more susceptible to hypercholesterolemia only when combined with postnatal consumption of a high-fat diet (Robinson et al., 2006). These results suggest that the postnatal nutritional environment may affect cholesterol metabolism differently in low birth weight individuals compared to their normal weight peers. One mechanism that may explain the link between prenatal growth and adult disease is a permanent change in gene expression in response to the early environment (Gluckman and Hanson, 2004).
Animal studies have shown that a high-fat diet significantly increased weights and body fat of malnourished rats in gestation and/or lactation, reduced lean body mass, and accentuated plasma leptin, an increase in glucose levels with increased insulin levels and hypertriglyceridemia in male rats (Desai et al., 2015). Assessing the effects of a nutritional transition model with incompatibility between peri- and postnatal diets in mice, Sellayah et al. (2014) offered a control diet (18% protein) or protein-restricted diet (9% protein) during pregnancy and lactation to mothers. After weaning, the male pups began to receive a standard diet (7% fat) or a high-fat diet (45% fat) until 30 weeks of age. The authors observed that offspring maternal protein restriction to those who consumed high-fat diet resulted in an increase in body adiposity, even without changing the total weight or increasing the lipid content in muscle tissue. While the consumption of a high-fat diet by animals that had not suffered previous malnutrition promoted an increase in energy expenditure and expression of proteins related to thermogenesis (uncoupling protein 1 - UCP1; adrenergic receptor beta 3 - β-3AR) in brown adipose tissue, the maternal protein restriction did not show the same response. These results suggested that a mismatch can attenuate diet-induced thermogenesis and contribute to the development of obesity. Animals with maternal protein restriction (5% protein) fed for 4 weeks with a high-fat diet showed a relatively dangerous increase in the white adipose tissue and a decrease in gross gastrocnemius muscle weight in males even without causing changes in bodyweight; in addition, males and females exhibited anxiety-like behaviors (de Almeida Silva et al., 2020).
The increase in white adipose tissue may be one of the factors that contribute to the development of cardiometabolic diseases in maternal protein-restricted individuals. A continuous intake of an HF diet can promote adipocyte hypertrophy and dysfunction and induce the infiltration of pro-inflammatory macrophages in adipose tissue, increasing the production of pro-inflammatory cytokines in this tissue (Ouchi et al., 2011). An increased immune activity is associated with a high consumption of high-fat diets and favors the maintenance of chronic systemic inflammatory processes, originating in adipose tissue (Wensveen et al., 2015; Ertunc and Hotamisligil, 2016; Lyons et al., 2016). Using a mouse model of a prenatal low-protein diet (LP, 8% protein) followed by a normal or postnatal diet high-energy in fat (HE, 45% fat) for 12 weeks, maternal protein restriction added to a high-fat diet interacts to affect growth recovery and leads to an increase of the offspring’s adipocyte tissue, which correlates with the phenotype of inflammation in adipose tissue (Xie et al., 2017). In this study, adipose tissue macrophage infiltration was not affected by the LP diet, as evidenced by the lack of difference in the number of CD68 cells in the adipose tissue. However, after postnatal treatment of HE, there were fewer cells of adipose tissue macrophages M1b subtypes (CD11c + CD206+) in f1 offspring from maternal LP dam than those on the normal protein maternal diet. The maternal LP diet interacts with the postnatal high-fat diet to impact the macrophage phenotypes of the existing adipose tissue, although the prenatal LP diet may not influence the ability of monocyte/macrophage migration from adipose tissue in F1 mouse offspring. At 60 days of age, the interaction between low maternal protein during pregnancy and lactation (9% casein) and offspring’s high-fat diet, after weaning (45% lipids), increased retroperitoneal and epididymal fat deposits and increased the production of nitric oxide by adipocyte macrophages (Alheiros-Lira et al., 2017). Adipose tissue with dysfunctional signaling has been considered to trigger reduced insulin sensitivity (Caricilli et al., 2008; Calder, 2012) and change the plasma and tissue lipid profile (Ralston et al., 2017) in addition to promoting cardiovascular disorders (Wang and Hu, 2017). A high production of nitric oxide has been implicated in the apoptosis of macrophages (Sarih et al., 1993) and pathogenesis of inflammatory diseases (Borges et al., 2013), suggesting high immunoreactivity induced by dietary fatty acids after metabolic programming with maternal protein restriction. Maternal prenatal malnutrition appears to modify the programs of the gene expression profile of offspring adipose tissue over the long term; this, combined with obesogenic nutrition, predisposes prenatal malnourished individuals to an altered lipid metabolism and fat accumulation in adulthood (Lukaszewski et al., 2011).
Maternal protein restriction (8% casein) during pregnancy and lactation followed by a post-weaning HF diet (41% fat) induced an increase in the percentage of visceral fat, reduced insulin sensitivity, and increased food intake in rat males at 90 days of age (Gosby et al., 2010). In an experimental design with Sprague–Dawley rats, Iwasa et al. (2017) demonstrated that the consumption of a post-weaning high-fat diet (62.2% of calories from fat) induced an increase in the serum leptin level and a trend toward a reduction in the hypothalamic POMC mRNA level in the offspring of undernourished dams, whereas it had no effect on the levels of orexigenic factors or other anorexigenic factors. Male mice at 220 days of life malnourished during pregnancy and lactation and fed with a high-fat postnatal diet showed increased gene expression of POMC and MC4R in the hypothalamus and hypomethylation of the POMC promoter in the hypothalamus (Zheng et al., 2021). Vickers et al. (2000) studied the interaction between restricted maternal diet and amplification by a post-weaning high-fat diet and demonstrated that deep adult hyperphagia is a consequence of fetal programming and an essential contributing factor to adult pathophysiology.
At the serum level, the activity of adipokines, such as leptin, in malnourished organisms, presents divergent behavior and suggests action and selective sensitivity, both with endocrine regulation function (do Prado et al., 2009), in both immune (Arroyo Hornero et al., 2020) and physiological systems. A study by Sellayah et al. (2014) sought to evaluate the effects of incompatibility between the fetal nutritional environment poor in proteins and a lipid-rich environment in the post-weaning period; Wistar rats received a control diet (18% casein) or with protein restriction (9%) during pregnancy and standard diet during lactation. Pups, including males and females, started to receive a control diet (7% lipids and 15% casein) or a high-fat diet (45% lipids and 26% casein), from weaning to 16 weeks of age, when they were evaluated. The study demonstrated that the nutritional mismatch exacerbates the increase in blood pressure promoted by maternal malnutrition, regardless of increased body adiposity, glucose, or leptin levels, modulated upward only by the high-fat diet and not by the maternal diet during pregnancy. Post-weaning hyperlipidic diets have numerous impacts on glycolipid metabolism in offspring with maternal protein restriction, insulin intolerance, decreased insulin sensitivity, higher triglyceride/high-density lipoprotein ratio and high levels of leptin and interleukin-6 in adipose tissue and low adiponectin (Wu et al., 2020).
Another organ that appears to be particularly affected by the nutritional transition and which is at the central core of the development of a range of metabolic diseases is the liver. The liver is sensitive to dietary modulations. A shortage of amino acids, for example, has been related to liver diseases (Nishi et al., 2018). In an experimental model of protein malnutrition, hepatocytes isolated from rats fed with 5% casein protein for 14 days showed increased triglyceride synthesis (Taguchi et al., 2017). Transcription factors, such as the carbohydrate-sensitive response element-binding protein (ChREBp), peroxisome proliferator-activated receptors (PPAR), and the sterol regulating element-binding protein, (SREBp) respond to excessive lipid consumption, and they control energetic homeostasis and can activate pathways related to lipogenesis and inflammatory processes in liver tissue (Mello et al., 2016; Shimano and Sato, 2017; Li et al., 2019). Chmurzynska et al. (2012) showed that the altered response to a high-fat diet programmed by maternal nutrition during pregnancy was detected as an altered gene expression in 10-week-old rats and central adiposity in 16-week-old rats. Hepatic transcription of PPARγ in response to the high-fat diet was dependent on maternal nutrition. Male rats subjected to maternal protein restriction and fed with a high-fat diet showed an increase in PPAR levels, while rats with a normoprotein maternal diet and a postnatal diet rich in fatty acids showed a reduction in PPAR levels. Likewise, activation of the PPARα gene by a high-fat diet was dependent on prenatal nutrition (Chmurzynska et al., 2012).
Assessing the structure of liver tissue, a study by Souza-Mello et al. (2007) demonstrated that nutritional mismatch with a low protein maternal diet (9% during pregnancy and lactation) and a postnatal diet rich in lipids (50% of the total caloric component) induces an increase in blood pressure and gonadal adiposity, in addition to worsening liver steatosis in male rats. However, the mechanisms are still unclear, but they may be related to an increased activity of lipid synthesis and oxidation as a strategy to save proteins in conditions of scarcity and in the regulation of transcription factors and gene expression related to glycolipid metabolism. In an experimental study with sheep, a 50% reduction in dietary proteins during pregnancy and lactation, followed by post-weaning consumption of a high-fat diet (38% fat), increased the hepatic triglyceride content in lambs, with permanent reduction induction in the expression of genes that regulate energy homeostasis (GLUT1, PPAR, SREBP1c, and phosphoenolpyruvate carboxykinase gene—PEPCK) (Souza-Mello et al., 2007).
During fasting states, the use of fatty acids as an energy substrate occurs through β-oxidation, mainly in a mitochondrial environment (Reddy and Rao, 2006), with the action of key enzymes such as acyl-CoA dehydrogenase, enoyl-CoA hydrolase, and β-hydroxycil CoA-dehydrogenase (Jones, 2016). Elevated insulin and glucose levels regulate the synthesis of triacylglycerols, stimulating the activity of transcription factors such as ChREBp and the sterol regulatory element-binding protein SREBp and activated by peroxisome proliferator receptors PPAR. These factors increase the expression of lipogenic enzymes such as acetyl-coenzyme A carboxylase (Zhao et al., 2012) and fatty acid synthase FAS (Canbay et al., 2007). Imbalances in transport mechanisms or in the activation of transcription factors can then induce excessive accumulation of triglycerides within hepatocytes and trigger liver diseases (Titchenell et al., 2017).
The increase in energy levels induces a reduction in mitochondrial oxidative activity, while the increase in lipid peroxidation induces damage to mitochondria. In the liver, a maternal protein restriction of Wistar rats (casein 8%) during pregnancy and lactation, followed by a diet with post-weaning HF until 90 days of life (32% of the caloric percentage originated in lipids and 59% at more than saturated fatty acids), induced a reduction in all respiratory states of liver mitochondria, (Vickers et al., 2000) greater mitochondrial edema compared to controls enhanced after the addition of Ca2+ and prevented in the presence of EGTA (calcium chelator) and cyclosporine A (transition pore inhibitor of mitochondrial permeability), and greater oxidation of liver proteins and lipid peroxidation, with a reduction in catalase and glutathione peroxidase activities. These data suggest that adult rats subjected to maternal protein restriction were more susceptible to liver mitochondrial damage caused by a diet rich in saturated fatty acids after weaning (Simoes-Alves et al., 2019). Figure 2 summarizes the repercussions of the consumption of high-fat diets postnatally in individuals subjected to protein restriction during pregnancy and lactation.
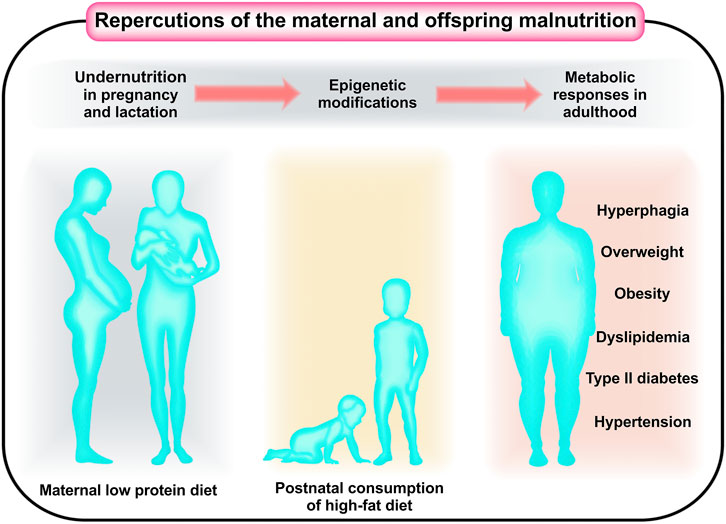
FIGURE 2. Graphic summary of the repercussions of the postnatal consumption of high-fat diets in individuals subjected to protein restriction during pregnancy and lactation.
Conclusion
A shift in the nutritional status of the perinatal and postnatal environment induces accelerated recovery growth and adjustments in autonomic modulation and insulin sensitivity as well as mitochondrial dysfunction. A post-weaning high-caloric/high-fat diet potentiates these adjustments, exacerbating deleterious changes in important metabolic organs, namely, hepatic, adipose, and muscular tissue. The epigenetic repercussions of postnatal metabolic overload may be etiological sources of cardiometabolic diseases, which affect the subjects suffering nutritional transition.
Author Contributions
AS-A, AA-M, JC, AW, CL, JC-S, and VO drafted the manuscript, critically revised the intellectual content and performed final review of the manuscript.
Funding
This study was supported financially by Fundação de Amparo the Ciência e Tecnologia de Pernambuco-PE-Brazil (PRONEM 0797-4.05/14), the Conselho Nacional de Desenvolvimento Científico e Tecnológico—Brazil (CNPq n° 311386/2019-9), and the CAPES/COFECUB Program (number: 797-14 and 923-20). ACSA, APFCAM, and JOC are graduate students supported by fellowship grants from the Coordination for the Improvement of Higher Education Personnel (Scholarship—CAPES).
Conflict of Interest
The authors declare that the research was conducted in the absence of any commercial or financial relationships that could be construed as a potential conflict of interest.
Publisher’s Note
All claims expressed in this article are solely those of the authors and do not necessarily represent those of their affiliated organizations, or those of the publisher, the editors and the reviewers. Any product that may be evaluated in this article, or claim that may be made by its manufacturer, is not guaranteed or endorsed by the publisher.
Acknowledgments
The authors thank Marcela Lima, for graphical support with the figures in this study. The English text of this study has been revised by Sidney Pratt, Canadian, MAT (The Johns Hopkins University) and RSAdip—TESL (Cambridge University).
References
Abdullah A. (2015). The Double Burden of Undernutrition and Overnutrition in Developing Countries: an Update. Curr. Obes. Rep. 4 (3), 337–349. doi:10.1007/s13679-015-0170-y
Ajala A. R., Almeida S. S., Rangel M., Palomino Z., Strufaldi M. W. L., Puccini R. F., et al. (2012). Association of ACE Gene Insertion/deletion Polymorphism with Birth Weight, Blood Pressure Levels, and ACE Activity in Healthy Children. Am. J. Hypertens. 25 (7), 827–832. doi:10.1038/ajh.2012.50
Alheiros-Lira M. C., Jurema-Santos G. C., da-Silva H. T., da-Silva A. C., Moreno Senna S., Ferreira e Silva W. T., et al. (2017). Effects of High-Fat Diet on Somatic Growth, Metabolic Parameters and Function of Peritoneal Macrophages of Young Rats Submitted to a Maternal Low-Protein Diet. Br. J. Nutr. 117 (6), 796–803. doi:10.1017/S0007114517000708
Almeida Silva L. C., Oliveira A. C., Cavalcante‐Silva V., Franco M. d. C., D'Almeida V. (2020). Hyperlipidic Diet Affects Body Composition and Induces Anxiety‐like Behaviour in Intrauterine Growth‐restricted Adult Mice. Exp. Physiol. 105 (12), 2061–2072. doi:10.1113/EP088859
Andersen L. G., Ängquist L., Gamborg M., Byberg L., Bengtsson C., Canoy D., et al. (2009). Birth Weight in Relation to Leisure Time Physical Activity in Adolescence and Adulthood: Meta-Analysis of Results from 13 Nordic Cohorts. PloS one 4 (12), e8192. doi:10.1371/journal.pone.0008192
Antony G. M., Laxmaiah A. (2008). Human Development, Poverty, Health & Nutrition Situation in India. Indian J. Med. Res. 128, 198–205.
Arroyo Hornero R., Hamad I., Côrte-Real B., Kleinewietfeld M. (2020). The Impact of Dietary Components on Regulatory T Cells and Disease. Front. Immunol. 11, 253. doi:10.3389/fimmu.2020.00253
Ashton N. (2000). Perinatal Development and Adult Blood Pressure. Braz. J. Med. Biol. Res. 33, 731–740. doi:10.1590/s0100-879x2000000700002
Aubin M. C., Lajoie C., Clément R., Gosselin H., Calderone A., Perrault L. P. (2008). Female Rats Fed a High-Fat Diet Were Associated with Vascular Dysfunction and Cardiac Fibrosis in the Absence of Overt Obesity and Hyperlipidemia: Therapeutic Potential of Resveratrol. J. Pharmacol. Exp. Therjournal Pharmacol. Exp. Ther. 325 (3), 961–968. doi:10.1124/jpet.107.135061
Barbosa S. d. S., Mello A. P. d. F. A. C., Nogueira V. d. O., da Silva I. F., de Melo P. E. D., Dos Santos C. R., et al. (2020). Consumption of a High‐fat Diet Does Not Potentiate the Deleterious Effects on Lipid and Protein Levels and Body Development in Rats Subjected to Maternal Protein Restriction. Clin. Exp. Pharmacol. Physiol. 47 (3), 412–421. doi:10.1111/1440-1681.13210
Barker D. J. P. (1999). Fetal Origins of Cardiovascular Disease. Ann. Med. 31 (Suppl. 1), 3–6. doi:10.1080/07853890.1999.11904392
Barker D. J. P., Godfrey K. M., Gluckman P. D., Harding J. E., Owens J. A., Robinson J. S. (1993). Fetal Nutrition and Cardiovascular Disease in Adult Life. The Lancet 341 (8850), 938–941. doi:10.1016/0140-6736(93)91224-a
Barker D. J. P., Osmond C., Forsén T. J., Kajantie E., Eriksson J. G. (2005). Trajectories of Growth Among Children Who Have Coronary Events as Adults. N. Engl. J. Med. 353 (17), 1802–1809. doi:10.1056/NEJMoa044160
Barker D. J. P., Osmond C., Winter P. D., Margetts B., Simmonds S. J. (1989). WEIGHT IN INFANCY AND DEATH FROM ISCHAEMIC HEART DISEASE. The Lancet 334 (8663), 577–580. doi:10.1016/S0140-6736(89)90710-1
Barker D. J. P. (2007). The Origins of the Developmental Origins Theory. J. Intern. Med. 261 (5), 412–417. doi:10.1111/j.1365-2796.2007.01809.x
Barker D., Osmond C. (1986). Infant Mortality, Childhood Nutrition, and Ischaemic Heart Disease in England and Wales. The Lancet 327 (8489), 1077–1081. doi:10.1016/s0140-6736(86)91340-1
Barros M. A. V., De Brito Alves J. L., Nogueira V. O., Wanderley A. G., Costa-Silva J. H. (2015). Maternal Low-Protein Diet Induces Changes in the Cardiovascular Autonomic Modulation in Male Rat Offspring. Nutr. Metab. Cardiovasc. Dis. 25 (1), 123–130. doi:10.1016/j.numecd.2014.07.011
Batista Filho M., Batista L. V. (2010). Transição Alimentar/Nutricional Ou Mutação Antropológica? J. Ciência e Cultura 62, 26–30.
Batista Filho M., Rissin A. (2003a). A transição nutricional no Brasil: tendências regionais e temporais. Cad. Saúde Pública 19, S181–S191. doi:10.1590/s0102-311x2003000700019
Batista Filho M., Rissin A. (2003b). A transição nutricional no Brasil: tendências regionais e temporais. J. Cadernos de Saúde Pública 19, S181–S191.
Batista T. M., Ribeiro R. A., da Silva P. M. R., Camargo R. L., Lollo P. C. B., Boschero A. C., et al. (2013). Taurine Supplementation Improves Liver Glucose Control in normal Protein and Malnourished Mice Fed a High-Fat Diet. Mol. Nutr. Food Res. 57 (3), 423–434. doi:10.1002/mnfr.201200345
Berends L. M., Dearden L., Tung Y. C. L., Voshol P., Fernandez-Twinn D. S., Ozanne S. E. (2018). Programming of central and Peripheral Insulin Resistance by Low Birthweight and Postnatal Catch-Up Growth in Male Mice. Diabetologia 61 (10), 2225–2234. doi:10.1007/s00125-018-4694-z
Biosca M., Rodríguez G., Ventura P., Samper M. P., Labayen I., Collado M. P., et al. (2011). Central Adiposity in Children Born Small and Large for Gestational Age. Nutr. Hosp. 26 (5), 971–976. doi:10.1590/s0212-16112011000500008
Borges M. C., Vinolo M. A. R., Crisma A. R., Fock R. A., Borelli P., Tirapegui J., et al. (2013). High-fat Diet Blunts Activation of the Nuclear Factor-Κb Signaling Pathway in Lipopolysaccharide-Stimulated Peritoneal Macrophages of Wistar Rats. Nutrition 29 (2), 443–449. doi:10.1016/j.nut.2012.06.008
Bowman G. D., Poirier M. G. (2015). Post-translational Modifications of Histones that Influence Nucleosome Dynamics. Chem. Rev. 115 (6), 2274–2295. doi:10.1021/cr500350x
Brawley L., Itoh S., Torrens C., Barker A., Bertram C., Poston L., et al. (2003). Dietary Protein Restriction in Pregnancy Induces Hypertension and Vascular Defects in Rat Male Offspring. Pediatr. Res. 54 (1), 83–90. doi:10.1203/01.PDR.0000065731.00639.02
Calder P. C. (2012). The Role of marine omega-3 ( N -3) Fatty Acids in Inflammatory Processes, Atherosclerosis and Plaque Stability. Mol. Nutr. Food Res. 56 (7), 1073–1080. doi:10.1002/mnfr.201100710
Canbay A., Bechmann L., Gerken G. (2007). Lipid Metabolism in the Liver. Z. Gastroenterol. 45 (1), 35–41. doi:10.1055/s-2006-927368
Caricilli A. M., Nascimento P. H., Pauli J. R., Tsukumo D. M. L., Velloso L. A., Carvalheira J. B., et al. (2008). Inhibition of Toll-like Receptor 2 Expression Improves Insulin Sensitivity and Signaling in Muscle and white Adipose Tissue of Mice Fed a High-Fat Diet. J. Endocrinol. 199, 399–406. doi:10.1677/JOE-08-0354
Chango A., Pogribny I. (2015). Considering Maternal Dietary Modulators for Epigenetic Regulation and Programming of the Fetal Epigenome. Nutrients 7 (4), 2748–2770. doi:10.3390/nu7042748
Chen J.-H., Martin-Gronert M. S., Tarry-Adkins J., Ozanne S. E. (2009). Maternal Protein Restriction Affects Postnatal Growth and the Expression of Key Proteins Involved in Lifespan Regulation in Mice. PLoS One 4 (3), e4950. doi:10.1371/journal.pone.0004950
Chen J.-H., Tarry-Adkins J. L., Matharu K., Yeo G. S. H., Ozanne S. E. (2010). Maternal Protein Restriction Affects Gene Expression Profiles in the Kidney at Weaning with Implications for the Regulation of Renal Function and Lifespan. Clin. Sci. (Lond) 119, 373–387. doi:10.1042/CS20100230
Chmurzynska A., Stachowiak M., Gawecki J., Pruszynska-Oszmalek E., Tubacka M. (2012). Protein and Folic Acid Content in the Maternal Diet Determine Lipid Metabolism and Response to High-Fat Feeding in Rat Progeny in an Age-dependent Manner. Genes Nutr. 7 (2), 223–234. doi:10.1007/s12263-011-0253-7
Chong S., Whitelaw E. (2004). Epigenetic Germline Inheritance. Curr. Opin. Genet. Development 14 (6), 692–696. doi:10.1016/j.gde.2004.09.001
Claycombe K. J., Roemmich J. N., Johnson L., Vomhof-DeKrey E. E., Johnson W. T. (2015). Skeletal Muscle Sirt3 Expression and Mitochondrial Respiration Are Regulated by a Prenatal Low-Protein Diet. J. Nutr. Biochem. 26 (2), 184–189. doi:10.1016/j.jnutbio.2014.10.003
Conde W. L., Monteiro C. A. (2014). Nutrition Transition and Double burden of Undernutrition and Excess of Weight in Brazil. Am. J. Clin. Nutr. 100 (6), 1617S–22S. doi:10.3945/ajcn.114.084764
Costa-Silva J. H., de Brito-Alves J. L., Barros M. A. d. V., Nogueira V. O., Paulino-Silva K. M., de Oliveira-Lira A., et al. (2015). New Insights on the Maternal Diet Induced-Hypertension: Potential Role of the Phenotypic Plasticity and Sympathetic-Respiratory Overactivity. Front. Physiol. 6 (345). doi:10.3389/fphys.2015.00345
Costa-Silva J. H., Silva P. A., Pedi N., Luzardo R., Einicker-Lamas M., Lara L. S., et al. (2009). Chronic Undernutrition Alters Renal Active Na+ Transport in Young Rats: Potential Hidden Basis for Pathophysiological Alterations in Adulthood? Eur. J. Nutr. 48, 437–445. doi:10.1007/s00394-009-0032-z
D'Ippolito E., Iorio M. (2013). MicroRNAs and Triple Negative Breast Cancer. Ijms 14 (11), 22202–22220. doi:10.3390/ijms141122202
da Silva Aragão R., Guzmán-Quevedo O., Pérez-García G., Manhães-de-Castro R., Bolaños-Jiménez F. (2014). Maternal Protein Restriction Impairs the Transcriptional Metabolic Flexibility of Skeletal Muscle in Adult Rat Offspring. Br. J. Nutr. 112 (3), 328–337. doi:10.1017/S0007114514000865
de Brito Alves J. L., Costa-Silva J. H. (2018). Maternal Protein Malnutrition Induced-Hypertension: New Evidence about the Autonomic and Respiratory Dysfunctions and Epigenetic Mechanisms. Clin. Exp. Pharmacol. Physiol. 45 (5), 422–429. doi:10.1111/1440-1681.12892
de Brito Alves J. L., de Oliveira J. M. D., Ferreira D. J. S., Barros M. A. d. V., Nogueira V. O., Alves D. S., et al. (2016). Maternal Protein Restriction Induced-Hypertension Is Associated to Oxidative Disruption at Transcriptional and Functional Levels in the Medulla Oblongata. Clin. Exp. Pharmacol. Physiol. 43 (12), 1177–1184. doi:10.1111/1440-1681.12667
de Brito Alves J. L., Nogueira V. O., Cavalcanti Neto M. P., Leopoldino A. M., Curti C., Colombari D. S., et al. (2015). Maternal Protein Restriction Increases Respiratory and Sympathetic Activities and Sensitizes Peripheral Chemoreflex in Male Rat Offspring. J. Nutr. 145 (5), 907–914. doi:10.3945/jn.114.202804
de Brito Alves J. L., Nogueira V. O., de Oliveira G. B., da Silva G. S. F., Wanderley A. G., Leandro C. G., et al. (2014). Short- and Long-Term Effects of a Maternal Low-Protein Diet on Ventilation, O2/CO2chemoreception and Arterial Blood Pressure in Male Rat Offspring. Br. J. Nutr. 111 (4), 606–615. doi:10.1017/S0007114513002833
de Brito Alves J. L., Toscano A. E., da Costa-Silva J. H., Vidal H., Leandro C. G., Pirola L. (2017). Transcriptional Response of Skeletal Muscle to a Low Protein Perinatal Diet in Rat Offspring at Different Ages: The Role of Key Enzymes of Glucose-Fatty Acid Oxidation. J. Nutr. Biochem. 41, 117–123. doi:10.1016/j.jnutbio.2016.12.002
Desai M., Babu J., Ross M. G. (2007). Programmed Metabolic Syndrome: Prenatal Undernutrition and Postweaning Overnutrition. Am. J. Physiology-Regulatory, Integr. Comp. Physiol. 293 (6), R2306–R2314. doi:10.1152/ajpregu.00783.2006
Desai M., Jellyman J. K., Ross M. G. (2015). Epigenomics, Gestational Programming and Risk of Metabolic Syndrome. Int. J. Obes. 39 (4), 633–641. doi:10.1038/ijo.2015.13
Di Pietro G., Cardoso D. S., da Silva H. M. B. S., Santos J. C., Dos Santos J. R., Simões R. A. (2015). Profile Development of Noncommunicable Chronic Diseases in a Brazilian Rural Town. J. Am. Coll. Nutr. 34 (3), 191–198. doi:10.1080/07315724.2014.926162
do Prado W. L., de Piano A., Lazaretti-Castro M., de Mello M. T., Stella S. G., Tufik S., et al. (2009). Relationship between Bone mineral Density, Leptin and Insulin Concentration in Brazilian Obese Adolescents. J. Bone Miner Metab. 27 (5), 613–619. doi:10.1007/s00774-009-0082-6
Egger G., Liang G., Aparicio A., Jones P. A. (2004). Epigenetics in Human Disease and Prospects for Epigenetic Therapy. Nature 429 (6990), 457–463. doi:10.1038/nature02625
Ertunc M. E., Hotamisligil G. S. (2016). Lipid Signaling and Lipotoxicity in Metaflammation: Indications for Metabolic Disease Pathogenesis and Treatment. J. lipid Res. 57, 2099–2114. doi:10.1194/jlr.R066514
Falcão-Tebas F., Bento-Santos A., Antônio Fidalgo M., de Almeida M. B., Antônio dos Santos J., Lopes de Souza S., et al. (2012). Maternal Low-Protein Diet-Induced Delayed Reflex Ontogeny Is Attenuated by Moderate Physical Training during Gestation in Rats. Br. J. Nutr. 107 (3), 372–377. doi:10.1017/s0007114511002947
Feng A., Wang L., Chen X., Liu X., Li L., Wang B., et al. (2015). Developmental Origins of Health and Disease (DOHaD): Implications for Health and Nutritional Issues Among Rural Children in China. Bst 9, 82–87. doi:10.5582/bst.2015.01008
Ferreira D. S., Liu Y., Fernandes M. P., Lagranha C. J. (2015). Perinatal Low-Protein Diet Alters Brainstem Antioxidant Metabolism in Adult Offspring. Nutr. Neurosci. 19, 369–375. doi:10.1179/1476830515Y.0000000030
Fidalgo M., Falcão-Tebas F., Bento-Santos A., de Oliveira E., Nogueira-Neto J. F., de Moura E. G., et al. (2013). Programmed Changes in the Adult Rat Offspring Caused by Maternal Protein Restriction during Gestation and Lactation Are Attenuated by Maternal Moderate-Low Physical Training. Br. J. Nutr. 109 (3), 449–456. doi:10.1017/s0007114512001316
Franco M. C. P., Casarini D. E., Carneiro-Ramos M. S., Sawaya A. L., Barreto-Chaves M. L. M., Sesso R. (2008). Circulating Renin-Angiotensin System and Catecholamines in Childhood: Is There a Role for Birthweight? Clin. Sci. 114, 375–380. doi:10.1042/CS20070284
Gluckman P. D., Hanson M. A. (2004). Living with the Past: Evolution, Development, and Patterns of Disease. Science 305 (5691), 1733–1736. doi:10.1126/science.1095292
Gosby A. K., Maloney C. A., Caterson I. D. (2010). Elevated Insulin Sensitivity in Low-Protein Offspring Rats Is Prevented by a High-Fat Diet and Is Associated with Visceral Fat. Obesity (Silver Spring) 18 (8), 1593–1600. doi:10.1038/oby.2009.449
Goyal R., Goyal D., Leitzke A., Gheorghe C. P., Longo L. D. (2010). Brain Renin-Angiotensin System: Fetal Epigenetic Programming by Maternal Protein Restriction during Pregnancy. Reprod. Sci. 17 (3), 227–238. doi:10.1177/1933719109351935
Gräff J., Tsai L.-H. (2013). Histone Acetylation: Molecular Mnemonics on the Chromatin. Nat. Rev. Neurosci. 14 (2), 97–111. doi:10.1038/nrn3427
Hasan M. T., Soares Magalhães R. J., Williams G. M., Mamun A. A. (2016). Long-term Changes in Childhood Malnutrition Are Associated with Long-Term Changes in Maternal BMI: Evidence from Bangladesh, 1996-2011. Am. J. Clin. Nutr. 104 (4), 1121–1127. doi:10.3945/ajcn.115.111773
Hemachandra A. H., Klebanoff M. A., Duggan A. K., Hardy J. B., Furth S. L. (2006). The Association between Intrauterine Growth Restriction in the Full-Term Infant and High Blood Pressure at Age 7 years: Results from the Collaborative Perinatal Project. Int. J. Epidemiol. 35 (4), 871–877. doi:10.1093/ije/dyl080
Herrán O. F., Patiño G. A., Del Castillo S. E. (20162010). Transición alimentaria y exceso de peso en adultos. Encuesta de la Situación Nutricional en Colombia, 2010. Biomedica 36 (1), 109–120. doi:10.7705/biomedica.v36i1.2579
Hotamisligil G. S. (2006). Inflammation and Metabolic Disorders. Nature 444 (7121), 860–867. doi:10.1038/nature05485
Ito T., Funamoto K., Sato N., Nakamura A., Tanabe K., Hoshiai T. (2012). Maternal Undernutrition Induces the Expression of Hypoxia-Related Genes in the Fetal Brain. Tohoku J. Exp. Med. 226 (1), 37–44. doi:10.1620/tjem.226.37
Ito T., Tanabe K., Nakamura A., Funamoto K., Aoyagi A., Sato K., et al. (2011). Aberrant Expression of Hypoxia-Inducible Factor 1.ALPHA. In the Fetal Heart Is Associated with Maternal Undernutrition. Tohoku J. Exp. Med. 224 (3), 163–171. doi:10.1620/tjem.224.163
Iwasa T., Matsuzaki T., Yano K., Tungalagsuvd A., Munkhzaya M., Mayila Y., et al. (2017). The Effects of Prenatal Undernutrition and a High-Fat Postnatal Diet on central and Peripheral Orexigenic and Anorexigenic Factors in Female Rats. Endocr. J. 64 (6), 597–604. doi:10.1507/endocrj.EJ16-0593
Jablonka E., Lamb M. J. (2002). The Changing Concept of Epigenetics. Ann. N. Y Acad. Sci. 981, 82–96. doi:10.1111/j.1749-6632.2002.tb04913.x
Johansson S., Norman M., Legnevall L., Dalmaz Y., Lagercrantz H., Vanpée M. (2007). Increased Catecholamines and Heart Rate in Children with Low Birth Weight: Perinatal Contributions to Sympathoadrenal Overactivity. J. Intern. Med. 261 (5), 480–487. doi:10.1111/j.1365-2796.2007.01776.x
John R. M., Rougeulle C. (2018). Developmental Epigenetics: Phenotype and the Flexible Epigenome. Front. Cel Dev. Biol. 6, 130. doi:10.3389/fcell.2018.00130
Jones J. G. (2016). Hepatic Glucose and Lipid Metabolism. Diabetologia 59 (6), 1098–1103. doi:10.1007/s00125-016-3940-5
Kroker-Lobos M. F., Pedroza-Tobías A., Pedraza L. S., Rivera J. A. (2014). The Double burden of Undernutrition and Excess Body Weight in Mexico. Am. J. Clin. Nutr. 100 (6), 1652s–1658s. doi:10.3945/ajcn.114.083832
Labayen I., Moreno L. A., Blay M. G., Blay V. A., Mesana M. I., González-Gross M., et al. (2006). Early Programming of Body Composition and Fat Distribution in Adolescents. J. Nutr. 136 (1), 147–152. doi:10.1093/jn/136.1.147
Leandro C. G., da Silva Ribeiro W., Dos Santos J. A., Bento-Santos A., Lima-Coelho C. H., Falcão-Tebas F., et al. (2012). Moderate Physical Training Attenuates Muscle-specific Effects on Fibre Type Composition in Adult Rats Submitted to a Perinatal Maternal Low-Protein Diet. Eur. J. Nutr. 51 (7), 807–815. doi:10.1007/s00394-011-0259-3
Leocádio P. C. L., Lopes S. C., Dias R. P., Alvarez-Leite J. I., Guerrant R. L., Malva J. O., et al. (2021). The Transition from Undernutrition to Overnutrition under Adverse Environments and Poverty: The Risk for Chronic Diseases. Front. Nutr. 8, 676044. doi:10.3389/fnut.2021.676044
Li G., Jiang Q., Xu K. (2019). CREB Family: A Significant Role in Liver Fibrosis. Biochimie 163, 94–100. doi:10.1016/j.biochi.2019.05.014
Lucas A. (1998). Programming by Early Nutrition: An Experimental Approach. J. Nutr. 128 (2), 401S–406S. doi:10.1093/jn/128.2.401S
Lukaszewski M.-A., Mayeur S., Fajardy I., Delahaye F., Dutriez-Casteloot I., Montel V., et al. (2011). Maternal Prenatal Undernutrition Programs Adipose Tissue Gene Expression in Adult Male Rat Offspring under High-Fat Diet. Am. J. Physiology-Endocrinology Metab. 301, E548–E559. doi:10.1152/ajpendo.00011.2011
Lyons C., Kennedy E., Roche H. (2016). Metabolic Inflammation-Differential Modulation by Dietary Constituents. Nutrients 8 (5), 247. doi:10.3390/nu8050247
Mansego M. L., Milagro F. I., Campión J., Martínez J. A. (2013). Techniques of DNA Methylation Analysis with Nutritional Applications. J. Nutrigenet Nutrigenomics 6 (2), 83–96. doi:10.1159/000350749
Mazzio E. A., Soliman K. F. (2014b). Epigenetics and Nutritional Environmental Signals. Integr. Comp. Biol. 54 (1), 21–30. doi:10.1093/icb/icu049
Mazzio E. A., Soliman K. F. A. (2014a). Epigenetics and Nutritional Environmental Signals. Integr. Comp. Biol. 54 (1), 21–30. doi:10.1093/icb/icu049
Mello T., Materozzi M., Galli A. (2016). PPARs and Mitochondrial Metabolism: From NAFLD to HCC. PPAR Res. 2016, 1–18. doi:10.1155/2016/7403230
Mitchell C., Schneper L. M., Notterman D. A. (2016). DNA Methylation, Early Life Environment, and Health Outcomes. Pediatr. Res. 79 (1-2), 212–219. doi:10.1038/pr.2015.193
Ng M., Fleming T., Robinson M., Thomson B., Graetz N., Margono C., et al. (2014). Global, Regional, and National Prevalence of Overweight and Obesity in Children and Adults during 1980-2013: a Systematic Analysis for the Global Burden of Disease Study 2013. Lancet 384 (9945), 766–781. doi:10.1016/S0140-6736(14)60460-8
Nishi H., Yamanaka D., Kamei H., Goda Y., Kumano M., Toyoshima Y., et al. (2018). Importance of Serum Amino Acid Profile for Induction of Hepatic Steatosis under Protein Malnutrition. Sci. Rep. 8 (1), 5461. doi:10.1038/s41598-018-23640-8
Nogueira V., Brito-Alves J., Fontes D., Oliveira L., Lucca W., Tourneur Y., et al. (2018). Carotid Body Removal Normalizes Arterial Blood Pressure and Respiratory Frequency in Offspring of Protein-Restricted Mothers. Hypertens. Res. 41 (12), 1000–1012. doi:10.1038/s41440-018-0104-7
Nogueira V. O., Andrade L. D. S., Rocha-Júnior R. L., Melo P. E. D., Helvécio E., Fontes D. A. F., et al. (2019). Maternal Physical Activity Prevents the Overexpression of Hypoxia-Inducible Factor 1-α and Cardiorespiratory Dysfunction in Protein Malnourished Rats. Sci. Rep. 9 (1), 14406. doi:10.1038/s41598-019-50967-7
Nuyt A. M., Alexander B. T. (2009). Developmental Programming and Hypertension. Curr. Opin. Nephrol. Hypertens. 18 (2), 144–152. doi:10.1097/MNH.0b013e328326092c
Ouchi N., Parker J. L., Lugus J. J., Walsh K. (2011). Adipokines in Inflammation and Metabolic Disease. Nat. Rev. Immunol. 11 (2), 85–97. doi:10.1038/nri2921
Ozanne S. E., Hales C. N. (2004). Catch-up Growth and Obesity in Male Mice. Nature 427, 411–412. doi:10.1038/427411b
Parra D. C., Iannotti L., Gomez L. F., Pachón H., Haire-Joshu D., Sarmiento O. L., et al. (2015). The Nutrition Transition in Colombia over a Decade: a Novel Household Classification System of Anthropometric Measures. Arch. Public Health 73 (1), 12. doi:10.1186/s13690-014-0057-5
Paulino-Silva K. M., Costa-Silva J. H. (2016). Hypertension in Rat Offspring Subjected to Perinatal Protein Malnutrition Is Not Related to the Baroreflex Dysfunction. Clin. Exp. Pharmacol. Physiol. 43 (11), 1046–1053. doi:10.1111/1440-1681.12628
Popkin B. M. (2015). Nutrition Transition and the Global Diabetes Epidemic. Curr. Diab Rep. 15 (9), 64. doi:10.1007/s11892-015-0631-4
Radford E. J., Ito M., Shi H., Corish J. A., Yamazawa K., Isganaitis E., et al. (2014). In Utero undernourishment Perturbs the Adult Sperm Methylome and Intergenerational Metabolism. Science 345 (6198), 1255903. doi:10.1126/science.1255903
Ralston J. C., Lyons C. L., Kennedy E. B., Kirwan A. M., Roche H. M. (2017). Fatty Acids and NLRP3 Inflammasome-Mediated Inflammation in Metabolic Tissues. Annu. Rev. Nutr. 37, 77–102. doi:10.1146/annurev-nutr-071816-064836
Rangel M., dos Santos J. C., Ortiz P. H. L., Hirata M., Jasiulionis M. G., Araujo R. C., et al. (2014). Modification of Epigenetic Patterns in Low Birth Weight Children: Importance of Hypomethylation of the ACE Gene Promoter. PLoS One 9 (8), e106138. doi:10.1371/journal.pone.0106138
Reddy J. K., Sambasiva Rao M. (2006). Lipid Metabolism and Liver Inflammation. II. Fatty Liver Disease and Fatty Acid Oxidation. Am. J. Physiology-Gastrointestinal Liver Physiol. 290 (5), G852–G858. doi:10.1152/ajpgi.00521.2005
Ribeiro A. M., Lima M. d. C., Lira P. I. C. d., Silva G. A. P. d. (2015). Baixo peso ao nascer e obesidade: associação causal ou casual? Revista Paulista de Pediatria 33 (3), 340–348. doi:10.1016/j.rpped.2014.09.007
Robinson S. M., Robinson S. M., Batelaan S. F., Syddall H. E., Sayer A. A., Dennison E. M., et al. (2006). Combined Effects of Dietary Fat and Birth Weight on Serum Cholesterol Concentrations: the Hertfordshire Cohort Study. Am. J. Clin. Nutr. 84 (1), 237–244. doi:10.1093/ajcn/84.1.237
Sadakierska-Chudy A., Filip M. (2015). A Comprehensive View of the Epigenetic Landscape. Part II: Histone post-translational Modification, Nucleosome Level, and Chromatin Regulation by ncRNAs. Neurotox Res. 27 (2), 172–197. doi:10.1007/s12640-014-9508-6
Sandovici I., Smith N. H., Nitert M. D., Ackers-Johnson M., Uribe-Lewis S., Ito Y., et al. (2011). Maternal Diet and Aging Alter the Epigenetic Control of a Promoter-Enhancer Interaction at the Hnf4a Gene in Rat Pancreatic Islets. Proc. Natl. Acad. Sci. U.S.A. 108 (13), 5449–5454. doi:10.1073/pnas.1019007108
Sarih M., Souvannavong V., Adam A. (1993). Nitric Oxide Synthase Induces Macrophage Death by Apoptosis. Biochem. Biophysical Res. Commun. 191 (2), 503–508. doi:10.1006/bbrc.1993.1246
Sarmiento O. L., Parra D. C., González S. A., González-Casanova I., Forero A. Y., Garcia J. (2014). The Dual burden of Malnutrition in Colombia. Am. J. Clin. Nutr. 100 (6), 1628s–1635s. doi:10.3945/ajcn.114.083816
Sellayah D., Dib L., Anthony F. W., Watkins A. J., Fleming T. P., Hanson M. A., et al. (2014). Effect of Maternal Protein Restriction during Pregnancy and Postweaning High-Fat Feeding on Diet-Induced Thermogenesis in Adult Mouse Offspring. Eur. J. Nutr. 53 (7), 1523–1531. doi:10.1007/s00394-014-0657-4
Shimano H., Sato R. (2017). SREBP-regulated Lipid Metabolism: Convergent Physiology - Divergent Pathophysiology. Nat. Rev. Endocrinol. 13 (12), 710–730. doi:10.1038/nrendo.2017.91
Siddique K., Guzman G. L., Gattineni J., Baum M. (2014). Effect of Postnatal Maternal Protein Intake on Prenatal Programming of Hypertension. Reprod. Sci. 21 (12), 1499–1507. doi:10.1177/1933719114530186
Simões-Alves A. C., Costa-Silva J. H., Barros-Junior I. B., da Silva Filho R. C., Vasconcelos D. A. A., Vidal H., et al. (2019). Saturated Fatty Acid-Enriched Diet-Impaired Mitochondrial Bioenergetics in Liver from Undernourished Rats during Critical Periods of Development. Cells 8 (4), 335. doi:10.3390/cells8040335
Sosa-Larios T. C., Miliar-Garcia A. A., Morimoto S., Jaramillo-Flores M. E. (2017). Retracted Article: Alterations in Lipid Metabolism Due to a Protein-Restricted Diet in Rats during Gestation And/or Lactation. Food Funct. 9, 1274. doi:10.1039/c7fo01513e
Sousa C. P., Olinda R. A., Pedraza D. F. (2016). Prevalence of Stunting and Overweight/obesity Among Brazilian Children According to Different Epidemiological Scenarios: Systematic Review and Meta-Analysis. Sao Paulo Med. J. 134, 251–262. doi:10.1590/1516-3180.2015.0227121
Souza-Mello V., Mandarim-de-Lacerda C. A., Aguila M. B. (2007). Hepatic Structural Alteration in Adult Programmed Offspring (Severe Maternal Protein Restriction) Is Aggravated by post-weaning High-Fat Diet. Br. J. Nutr. 98 (6), 1159–1169. doi:10.1017/s0007114507771878
Steyn N. P., McHiza Z. J. (2014). Obesity and the Nutrition Transition in Sub‐Saharan Africa. Ann. N.Y. Acad. Sci. 1311, 88–101. doi:10.1111/nyas.12433
Szarc vel Szic K. S., Declerck K., Vidaković M., Vanden Berghe W. (2015). From Inflammaging to Healthy Aging by Dietary Lifestyle Choices: Is Epigenetics the Key to Personalized Nutrition? Clin. Epigenet 7, 33. doi:10.1186/s13148-015-0068-2
Taguchi Y., Toyoshima Y., Tokita R., Kato H., Takahashi S.-I., Minami S. (2017). Triglyceride Synthesis in Hepatocytes Isolated from Rats Fed a Low-Protein Diet Is Enhanced Independently of Upregulation of Insulin Signaling. Biochem. Biophysical Res. Commun. 490 (3), 800–805. doi:10.1016/j.bbrc.2017.06.120
Titchenell P. M., Lazar M. A., Birnbaum M. J. (2017). Unraveling the Regulation of Hepatic Metabolism by Insulin. Trends Endocrinol. Metab. 28 (7), 497–505. doi:10.1016/j.tem.2017.03.003
Toyoshima Y., Tokita R., Ohne Y., Hakuno F., Noguchi T., Minami S., et al. (2010). Dietary Protein Deprivation Upregulates Insulin Signaling and Inhibits Gluconeogenesis in Rat Liver. J. Mol. Endocrinol. 45 (5), 329–340. doi:10.1677/JME-10-0102
Vickers M. H., Breier B. H., Cutfield W. S., Hofman P. L., Gluckman P. D. (2000). Fetal Origins of Hyperphagia, Obesity, and Hypertension and Postnatal Amplification by Hypercaloric Nutrition. Am. J. Physiology-Endocrinology Metab. 279 (1), E83–E87. doi:10.1152/ajpendo.2000.279.1.E83
Victora C. G., Adair L., Fall C., Hallal P. C., Martorell R., Richter L., et al. (2008). Maternal and Child Undernutrition: Consequences for Adult Health and Human Capital. The Lancet 371 (9609), 340–357. doi:10.1016/S0140-6736(07)61692-4
Wang D. D., Hu F. B. (2017). Dietary Fat and Risk of Cardiovascular Disease: Recent Controversies and Advances. Annu. Rev. Nutr. 37 (1), 423–446. doi:10.1146/annurev-nutr-071816-064614
Waterland R. A., Michels K. B. (2007). Epigenetic Epidemiology of the Developmental Origins Hypothesis. Annu. Rev. Nutr. 27, 363–388. doi:10.1146/annurev.nutr.27.061406.093705
Wells J. C. K. (2012). Obesity as Malnutrition: the Role of Capitalism in the Obesity Global Epidemic. Am. J. Hum. Biol. 24 (3), 261–276. doi:10.1002/ajhb.22253
Wells J. C. K. (2007). The Programming Effects of Early Growth. Early Hum. Development 83 (12), 743–748. doi:10.1016/j.earlhumdev.2007.09.002
Wells J. C. K. (2011). The Thrifty Phenotype: An Adaptation in Growth or Metabolism? Am. J. Hum. Biol. 23 (1), 65–75. doi:10.1002/ajhb.21100
Wensveen F. M., Jelenčić V., Valentić S., Šestan M., Wensveen T. T., Theurich S., et al. (2015). NK Cells Link Obesity-Induced Adipose Stress to Inflammation and Insulin Resistance. Nat. Immunol. 16 (4), 376–385. doi:10.1038/ni.3120
West-Eberhard M. J. (2005). Phenotypic Accommodation: Adaptive Innovation Due to Developmental Plasticity. J. Exp. Zool. 304B (6), 610–618. doi:10.1002/jez.b.21071
Wu Y., Yin G., Wang P., Huang Z., Lin S. (2020). Effects of Different Diet Induced Postnatal Catch up Growth on Glycolipid Metabolism in Intrauterine Growth Retardation Male Rats. Exp. Ther. Med. 20 (6), 1. doi:10.3892/etm.2020.9263
Xie L., Zhang K., Rasmussen D., Wang J., Wu D., Roemmich J. N., et al. (2017). Effects of Prenatal Low Protein and Postnatal High Fat Diets on Visceral Adipose Tissue Macrophage Phenotypes and IL-6 Expression in Sprague Dawley Rat Offspring. PLoS One 12 (1), e0169581. doi:10.1371/journal.pone.0169581
Zambrano E., Bautista C. J., Deás M., Martínez-Samayoa P. M., González-Zamorano M., Ledesma H., et al. (2006). A Low Maternal Protein Diet during Pregnancy and Lactation Has Sex- and Window of Exposure-specific Effects on Offspring Growth and Food Intake, Glucose Metabolism and Serum Leptin in the Rat. J. Physiol. 571 (Pt 1), 221–230. doi:10.1113/jphysiol.2005.100313
Zhang Y., Yuan M., Bradley K. M., Dong F., Anversa P., Ren J. (2012). Insulin-Like Growth Factor 1 Alleviates High-Fat Diet-Induced Myocardial Contractile Dysfunction. Hypertension 59 (3), 680–693. doi:10.1161/HYPERTENSIONAHA.111.181867
Zhao J., Goldberg J., Bremner J. D., Vaccarino V. (2012). Global DNA Methylation Is Associated with Insulin Resistance. Diabetes 61 (2), 542–546. doi:10.2337/db11-1048
Zheng J., Xiao X., Zhang Q., Wang T., Yu M., Xu J. (2017). Maternal Low-Protein Diet Modulates Glucose Metabolism and Hepatic MicroRNAs Expression in the Early Life of Offspring †. Nutrients 9 (3), 205. doi:10.3390/nu9030205
Zheng J., Zhang L., Liu J., Li Y., Zhang J. (2021). Long-Term Effects of Maternal Low-Protein Diet and Post-weaning High-Fat Feeding on Glucose Metabolism and Hypothalamic POMC Promoter Methylation in Offspring Mice. Front. Nutr. 8, 657848. doi:10.3389/fnut.2021.657848
Zohdi V., Lim K., Pearson J., Black M. (2014). Developmental Programming of Cardiovascular Disease Following Intrauterine Growth Restriction: Findings Utilising a Rat Model of Maternal Protein Restriction. Nutrients 7 (1), 119–152. doi:10.3390/nu7010119
Keywords: hypertension, obesity, nutrition transition, saturated fatty acids, dyslipidemia
Citation: Simões-Alves AC, Arcoverde-Mello APFC, Campos JdO, Wanderley AG, Leandro CVG, da Costa-Silva JH and de Oliveira Nogueira Souza V (2022) Cardiometabolic Effects of Postnatal High-Fat Diet Consumption in Offspring Exposed to Maternal Protein Restriction In Utero. Front. Physiol. 13:829920. doi: 10.3389/fphys.2022.829920
Received: 06 December 2021; Accepted: 29 March 2022;
Published: 10 May 2022.
Edited by:
Jacqueline Kathleen Phillips, Macquarie University, AustraliaReviewed by:
Keshari Thakali, University of Arkansas for Medical Sciences, United StatesCopyright © 2022 Simões-Alves, Arcoverde-Mello, Campos, Wanderley, Leandro, da Costa-Silva and de Oliveira Nogueira Souza. This is an open-access article distributed under the terms of the Creative Commons Attribution License (CC BY). The use, distribution or reproduction in other forums is permitted, provided the original author(s) and the copyright owner(s) are credited and that the original publication in this journal is cited, in accordance with accepted academic practice. No use, distribution or reproduction is permitted which does not comply with these terms.
*Correspondence: Viviane de Oliveira Nogueira Souza, dml2aWFuZS5ub2d1ZWlyYUB1ZnBlLmJy