- 1Division of Sports and Rehabilitation Medicine, Ulm University Medical Center, Ulm, Germany
- 2Department of Sport and Health Sciences, Technical University of Munich, Munich, Germany
- 3Institute of Sports Medicine, Prevention and Rehabilitation, Paracelsus Medical University, Salzburg, Austria
Purpose: Elite rowers have large body dimensions, a high metabolic capacity, and they realize high training loads. These factors suggest a high total energy requirement (TER), due to high exercise energy expenditure (EEE) and additional energetic needs. We aimed to study EEE and intensity related substrate utilization (SU) of elite rowers during rowing (EEEROW) and other (EEENON-ROW) training.
Methods: We obtained indirect calorimetry data during incremental (N = 174) and ramp test (N = 42) ergometer rowing in 14 elite open-class male rowers (body mass 91.8 kg, 95% CI [87.7, 95.9]). Then we calculated EEEROW and SU within a three-intensity-zone model. To estimate EEENON-ROW, appropriate estimates of metabolic equivalents of task were applied. Based on these data, EEE, SU, and TER were approximated for prototypical high-volume, high-intensity, and tapering training weeks. Data are arithmetic mean and 95% confidence interval (95% CI).
Results: EEEROW for zone 1 to 3 ranged from 15.6 kcal·min−1, 95% CI [14.8, 16.3] to 49.8 kcal·min−1, 95% CI [48.1, 51.6], with carbohydrate utilization contributing from 46.4%, 95% CI [42.0, 50.8] to 100.0%, 95% CI [100.0, 100.0]. During a high-volume, a high-intensity, or a taper week, TER was estimated to 6,775 kcal·day−1, 95% CI [6,651, 6,898], 5,772 kcal·day−1, 95% CI [5,644, 5,900], or 4,626 kcal∙day−1, 95% CI [4,481, 4,771], respectively.
Conclusion: EEE in elite open-class male rowers is remarkably high already during zone 1 training and carbohydrates are dominantly utilized, indicating relatively high metabolic stress even during low intensity rowing training. In high-volume training weeks, TER is presumably at the upper end of the sustainable total energy expenditure. Periodized nutrition seems warranted for rowers to avoid low energy availability, which might negatively impact performance, training, and health.
Introduction
Elite open-class male rowers are characterized by large body dimensions of about 193 cm standing height and 94 kg body mass (Kerr et al., 2007). They also have a high aerobic capacity or maximum oxygen consumptions (V̇O2max) of up to 6.9 L·min−1 (Nielsen and Christensen, 2020), as a consequence of their high blood volume and muscle mass (Treff et al., 2014), high percentages of oxidative muscle fibers (Roth et al., 1993; Maciejewski et al., 2020), and high cardiac output (Volianitis et al., 2020). This unique combination of high aerobic and endurance capacity and overall muscular strength allows elite rowers to generate approximately 892 W peak power per rowing stroke (Lawton et al., 2013). Mechanical power output averages at approximately 590 W during a rowing race (Steinacker, 1993) in which the athletes cover the 2,000-m distance in about 5.5–6.5 min. Such performance necessitates a considerable anaerobic contribution of 12–33% (Roth et al., 1983; Pripstein et al., 1999) and the ability to tolerate extreme metabolic acidosis with a pH as low as 6.74 (Nielsen, 1999). This energy demand stresses the metabolic pathways extremely and consequently Olympic rowing has been deemed the ultimate challenge to the human body (Volianitis and Secher, 2009).
To maximize performance and to prepare for racing, rowers train 15–30 h per week. Rowing clearly dominates their training routine, but unspecific endurance training, resistance training, and stretching complement the program (Fiskerstrand and Seiler, 2004; Tran et al., 2015). Training intensity distribution, commonly accessed by a three-zone model, has been reported to follow a pyramidal distribution, with ∼ 85% low intensity training, ∼ 12% threshold training, and ∼ 3% spent at high intensities (Plews et al., 2014; Treff et al., 2017). It is worth mentioning that training intensity distributions differ considerably among international rowing programs (Treff et al., 2021b) and shifts towards a polarized distribution during certain phases of the competition period have been reported (Treff et al., 2017).
Due to the volume and complexity of a rower’s training, dedicated planning and monitoring of the total training load is warranted. This necessitates an integrated approach of external and internal training load (Bourdon et al., 2017). While the external training load (e.g., training distance or duration) is generally well assessable (Treff et al., 2017), the quantification of internal load is far more challenging. Tools like the recovery stress questionnaire (Kellmann et al., 2001), measures of the autonomous nervous system (Plews et al., 2014), or biomedical markers (Hecksteden et al., 2016; Bizjak et al., 2021) that have been proposed to mirror acute or midterm stress, are frequently applied. While all these markers are surrogates for the organism’s response to repeated exercise, they fail to quantify the energetic load of training, which is on the cellular level the major stimulus connecting nutrient intake and training adaption (Hawley et al., 2018).
The energetic load of training is reflected by the energy expenditure. Messonnier and colleagues (Messonnier et al., 2005a) aimed to assess the training load in international open-class and lightweight rowers based on questionnaires. They reported a mean habitual weekly energy expenditure of 5,388 ± 159 kcal∙day−1. Others reported a nutrient intake of 7,000 kcal·day−1 in elite open-class male rowers during high-volume training (Boegman and Dziedzic, 2016).
To assess the total energy requirement (TER) of a rower precisely, non-exercise and exercise energy expenditure (EEE) need to be considered. EEE in elite open-class male rowers is presumably high, because of the large body dimensions, the high training volume, the involvement of approximately 70% active muscle mass during rowing (Steinacker, 1993), and the low mechanical efficiency of rowing of about 16–24% (Di Prampero et al., 1971). Taken altogether this suggests a very high EEE already at moderate training intensities, which represent the major part of the TER in elite rowers (Messonnier et al., 2005a). The non-exercise energy expenditure is more complex and thus difficult to predict. On the one hand, a high resting metabolic rate of 2,675 kcal∙day−1 has already been reported in open-class male rowers (Carlsohn et al., 2011), but this high energy demand is contrasted by a sedentary behavior outside of the daily training routine (i.e., off-training) (Sperlich et al., 2017). On the other hand, a more recent study reported 2.2 h∙week−1of moderate to vigorous off-activities above 60% of maximal heart rate (Treff et al., 2021a), which in turn already in itself corresponds to a rather active lifestyle.
In the light of the high EEE and possibly high TER, an energetic limitation of rowing training volume seems possible (Mader and Hollmann, 1977) and sufficient energy intake becomes a potential issue when training volume is high. Thus, more adequate data on EEE and TER in elite open-class male rowers are needed to adjust energy supply and expenditure, because otherwise risk of inadequate dietary energy intake is apparent (Braakhuis et al., 2013).
We therefore aimed to quantify EEE and substrate utilization of rowing training in elite open-class male rowers during laboratory-based ergometer testing, and aimed to approximate the accumulated TER for prototypical periodized training. To the best of our knowledge, this is the first study in elite open-class male rowers dedicated to this aim.
Materials and Methods
Overview
We retrospectively analyzed data from 174 incremental step and 42 incremental ramp tests conducted repeatedly in 14 elite open-class male rowers. All tests were part of the German Rowing federation’s testing routines between 2013 and 2020. Step tests were used to determine lactate thresholds, mechanical power output, heart rate (HR), oxygen uptake (V̇O2), carbon dioxide production (V̇CO2), and respiratory exchange ratio (RER) at each stage, while ramp tests were used to measure V̇O2max. Based on LTs and V̇O2max, the intensity continuum was divided into three zones adapting previous recommendations (Seiler, 2010; Manunzio et al., 2016).
EEE during ergometer rowing (EEEROW) was accessed via indirect calorimetry, which also allowed for an estimation of the substrate utilization at a given workload. The percentage and absolute increase in carbohydrate metabolism, with a concomitant decrease in fatty acid utilization above moderate intensities (Brooks and Trimmer, 1996), is reflected by an increase in RER. Accordingly, RER allows for a specification of substrate utilization, thereby providing a further measure of (relative) exercise intensity in addition to percentage of V̇O2max, ventilatory, or lactate “threshold”.
For a given EEE, a corresponding amount of stored energy in the form of body fat and glycogen is required. We approximated the glycogen depletion associated with a given EEEROW based on body composition data and empirical data of liver and muscle glycogen, and blood glucose.
EEE for typical non-rowing sessions (EEENON-ROW) was calculated based on metabolic equivalents of task (MET) corrected for resting metabolic rate of the appropriate sports mode (e.g., indoor cycling). Using EEEROW and EEENON-ROW, we approximated daily TER for prototypical training weeks focusing either on high volume, high intensity, or on taper training.
Participants
Fourteen elite open-class male rowers (body mass 91.8 kg, 95% confidence interval (95%CI) [87.7, 95.9], fat free mass 83.5 kg, 95% CI [80.1, 86.9], V̇O2max 6.6 L·min−1, 95% CI [6.5, 6.7] or 72.0 ml·min−1∙kg−1 [69.6, 74.5]) decorated with several medals from Olympic Games and/or World Championships participated in this study. Participants completed a varying number of tests during the observation period, depending on whether the participants continued to qualify for the national team and/or because of absences from tests for health or other reasons. All rowers were familiar with the pre-testing and testing procedures and maintained a balanced diet. They conducted no high-intensity training 48 h prior testing and the last low intensity training session ended 20 h before each test or earlier to avoid fatigue, glycogen deficiency, and hypohydration. The study was conducted according to the declaration of Helsinki and approved by the ethical board of the University of Ulm (##267/11). All participants gave written informed consent to participate in the testing and the retrospective data analyses.
Equipment
All tests were conducted on a Concept 2 rowing ergometer (indoor rower, model D, Concept 2, Morrisville, United States). As described elsewhere (Mentz et al., 2020), the ergometer was equipped with a load cell and a rotary transducer to measure force and the travel distance of the handlebar, thereby allowing to calculate mechanical power output (Institut für Forschung und Entwicklung von Sportgeräten (FES), Berlin, Germany).
An automated metabolic analyzer equipped with a dynamic micro mixing chamber (Metamax 3x, Cortex Biophysics, Leipzig, Germany) measured V̇O2 and V̇CO2. Validity has been reported as 1.98 ± 2.98% difference to the Douglas bag method (Larsson et al., 2004). Blood lactate was measured using an amperometric-enzymatical analyzer (C-Line, EKF, Barleben, Germany). Reliability expressed as coefficient of variation (CV) of the device amounts to < 1.5% (Kohler and Boutellier, 2004). Calibration and maintenance of the ergometer setup and the metabolic and lactate analyzers were performed according to the manufacturers’ guidelines (EKF-diagnostic GmbH, 2013; Cortex Medical, 2015).
Procedures and Testing
Determination of Lactate Thresholds
After a 30-min standardized warm-up at 150 W, all rowers performed a submaximum incremental test on the rowing ergometer. Steps lasted 4 min and increased by 50 W. Workload ranged 200–400 W in ten rowers or 200–450 W in five rowers with exceptionally high endurance performance, respectively. Gas exchange and ventilation were measured continuously and data were averaged over the last 30 s of each stage. During a 30-s break after each stage, 20 µL of capillary blood were drawn from the hyperemic earlobe and the concentration of capillary blood lactate was analyzed. Lactate “thresholds” 1 and 2 (LT1 and LT2) were calculated according to Dickhuth and colleagues (Dickhuth et al., 1991), using a polynomic fitting of the data (Winlactat, Mesics, Münster, Germany). Data of mechanical power output, ventilation, and gas exchange were aligned to LT1 and LT2, respectively.
Measurement of Maximum Oxygen Uptake
After a 30-min standardized warm-up at 150 W on a rowing ergometer, all rowers performed an incremental ramp test on the same rowing ergometer used for the incremental step tests. Gas exchange and ventilation were measured with the same metabolic analyzer applied in the step tests. The initial target power output was 160 W and increased by 30, 35, or 40 W·min−1, depending on the individual rower’s estimated performance level. The test was automatically terminated if a rower failed to increase mechanical power output within a 7-W range of five strokes (Treff et al., 2018). V̇O2max was defined as the highest 30-s moving average and considered as maximum if V̇O2 failed to increase with progressive work rate (leveling-off) or at least a plateau (i.e., increase in V̇O2 < 150 ml·min−1) was observed (Midgley et al., 2007).
Calculations of Exercise Energy Expenditure, Glycogen Depletion, and Total Energy Requirement
Intensity-Zones
The metabolic load at LT1, 50% of LT2 (LT250%) (Manunzio et al., 2016), LT2 and V̇O2max was applied to a three-zone model (Seiler, 2010), with zone 1 ranging from LT250% to LT1, zone 2 ranging from LT1 to LT2, and zone 3 ranging from LT2 to V̇O2max.
Calculation of EEE and SU for Rowing
EEEROW and substrate utilization at LT1, LT250%, LT2, and V̇O2max were calculated using a non-protein table by Péronnet and colleagues (Péronnet and Massicotte, 1991). EEEROW was corrected by adding additional energy derived from the anaerobic energy contribution (ECLac) and subtracting the resting metabolic rate. ECLac was derived by applying a O2-lactate equivalent according to Equation 1:
Where Δblood lactate is the difference between rest and post-step or post-test blood lactate concentration, 1 mmol · L−1 Δblood lactate is equivalent to the energy released by the uptake of 0.0033 L of O2 per kg body mass (BM) (Margaria et al., 1963; Di Prampero and Ferretti, 1999), and 5.04 kcal represents the caloric equivalent of 1 liter O2. Resting metabolic rate was calculated according to Cunningham (Cunningham, 1980).
We calculated EEEROW and corresponding substrate utilization for typical steady-state sessions within the three intensity zones for different durations.
Calculation of EEENON-ROW
We calculated EEENON-ROW for steady-state exercise at a given intensity and duration using estimated METs that were corrected for resting metabolic rate according to Equation 2 (Ainsworth et al., 2011):
where MET values were taken from the Compendium of Physical Activities (Ainsworth et al., 2011) for cycling (compendium codes 1,050/1,040), strength training (2,050), calisthenics (2,030), stretching (2,101), and soccer (15,610). Again, resting metabolic rate (RMR) was calculated according to Cunningham (Cunningham, 1980).
Calculation of Glycogen Depletion
Glycogen depletion was calculated as a function of glycogen consumption (g·min−1) and duration of rowing training (minutes), for a given intensity zone based on the sum of carbohydrates from blood glucose, liver, and active skeletal muscle glycogen stores. Blood glucose mass was calculated based on plasma volume according to Equation 3:
with the first term representing the plasma volume estimated from lean body mass (LBM) (data provided from Treff et al., 2014) and the second term (1 g·L−1). which is the upper limit of the normal glucose concentration in the blood plasma in the fasting state. LBM was determined via bioimpedance measurements (InBody 720, BioSpace, Seoul, Korea). Liver glycogen energy was calculated based on Equation 4:
with 0.025 corresponding to the proportion of liver vs. body mass (BM) (Valentin, 2002), assuming 2.3 kg, 95% [2.2; 2.4] liver mass and 195 kcal·kg−1 or 365 kcal·kg−1 representing the lower and upper end of liver glycogen density (Rapoport, 2010). For muscle glycogen, we determined an active skeletal muscle mass (SMMActive) of 33.7 kg, 95% [32.2, 35.2] via bioimpedance measurements and we assumed 70% active muscle mass in rowing (Steinacker, 1993). The lower and upper muscle glycogen content was further calculated according to Equation 5:
were 0.2 is the transformation factor from wet to dry weight (dw), 0.18 g·mmol−1 is the molecular weight of glucose, 500 mmol · kg−1 or 700 mmol · kg−1 are the assumed lower and upper limits of glycogen density per kg dw (Hearris et al., 2018).
To convert mass (g) of carbohydrates into energy (kcal), or vice versa, we applied the caloric equivalent of 4.1 kcal·g−1.
Calculation of Recommended Energy Availability and Total Energy Need
To account for the additional energy requirements of activities outside of EEEROW and EEENON-ROW, we calculated the TER as the sum of EEE and the recommended energy availability (EAREC). The latter describes the amount of energy that is available for all other physical functions after subtracting the EEE from total energy expenditure (TEE) (Loucks, 2013) and was calculated according to Equation 6 (Areta et al., 2020):
where FFM depicts the fat free mass that was determined via bio impedance measurements. Based on these calculations, we approximated accumulated daily TER according to Equation 7:
Prototypical Training Sessions and Weeks
As a blueprint for typical training weeks we used original training plans of elite German rowers and calculated TER according to the previous equations. We selected exemplary high volume, high intensity, and tapering weeks. Training volume of these weeks amounted to 1,605, 1,055, and 555 min·week−1, respectively. Percentage of training spent in Zone 1–Zone 2–Zone 3 was 97.5%-2.5%-0.0% in the high-volume, 92.8%-0.0%-7.2% in the high-intensity, and 97.8%-0.0%-2.2% in the tapering week, respectively. Additional information is given in Table 1.
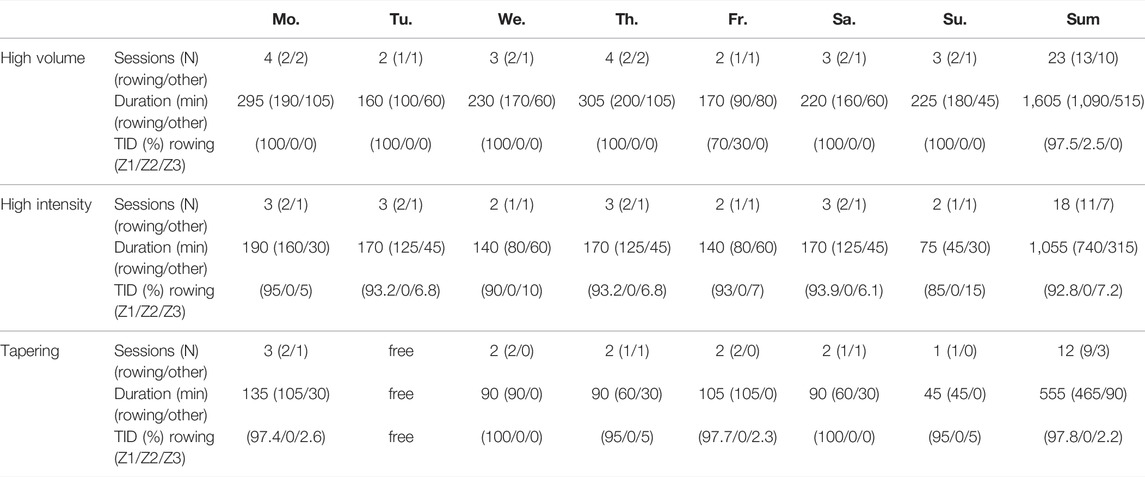
TABLE 1. Training indices for exemplary high volume, high intensity, and tapering rowing training weeks.
Statistical Analysis
To account for dependency of repeated step test (4–20 tests per rower) and ramp test (1-4 test per rower) measurements, unweighted individual mean values were calculated (Supplementary Table S1), with homogeneous individual standard deviations indicating no dependency of measurement variation on individual test repetitions. Consequently, individual mean values were further used to calculate robust descriptive data by the arithmetic mean with 95% CI. Descriptive data were calculated using SPSS (IBM Corp. Released 2017. IBM SPSS Statistics for Windows, Version 25.0. Armonk, NY: IBM Corp.).
Results
Table 2 includes mechanical power output, cardiorespiratory, metabolic, and RPE data aligned to LT1, LT2, and V̇O2max.
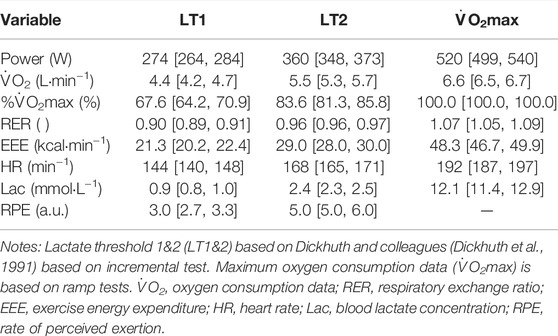
TABLE 2. Mechanical, cardiorespiratory, metabolic demand and perceived rate of exhaustion [mean (95% CI)] for rowing at individual lactate thresholds and maximum oxygen consumption.
Table 3 includes mechanical power output, cardiorespiratory, and metabolic data with corresponding EEEROW and SU for a three-zone model based on LT1, LT2, and V̇O2max. EEEROW ranged from 15.6 kcal·min−1, 95% CI [14.8, 16.3] to 49.8 kcal·min−1, 95% CI [48.1, 51.6] and carbohydrate utilization ranged from 46.4%, 95% CI [42.0, 50.8] to 100%, 95% CI [100.0, 100.0], respectively.
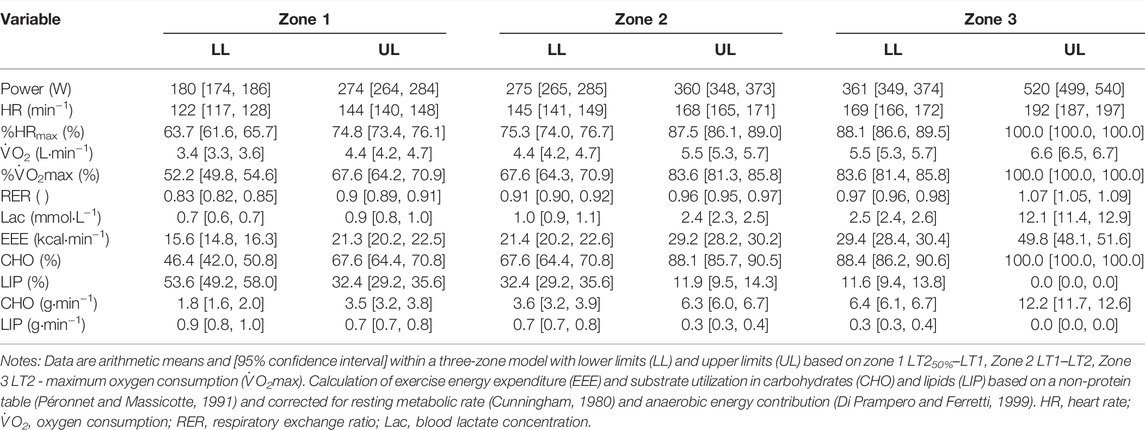
TABLE 3. Mechanical, cardiorespiratory and metabolic demand of rowing corresponding to a three-zone model.
Figure 1 shows the accumulated daily mean EEE, separated for EEEROW in zones 1-3 and EEENON-ROW for each of the prototypical training weeks. Descriptive training data for these weeks are shown in Table 1. The estimated mean weekly EEEROW during a high volume, high intensity, or tapering week amounted to 2,899 kcal∙day−1, 95% CI [2,749, 3,049], 2,110 kcal∙day−1, 95% CI [1,970, 2,251], or 1,257 kcal∙day−1, 95% CI [1,157, 1,356]. EEENON-ROW was 533 kcal∙day−1, 95% CI [455, 612], 319 kcal∙day−1, 95% CI [268, 371], or 27 kcal∙day−1, 95% CI [27, 27], respectively. Summarized with an estimated EAREC of 3,343 kcal∙day−1, 95% CI [3,211, 3,474], mean weekly TER amounted to 6,775 kcal·day−1, 95% CI [6,651, 6,898], 5,772 kcal·day−1, 95% CI [5,644, 5,900] or 4,626 kcal∙day−1, 95% CI [4,481, 4,771] for these prototypical weeks.
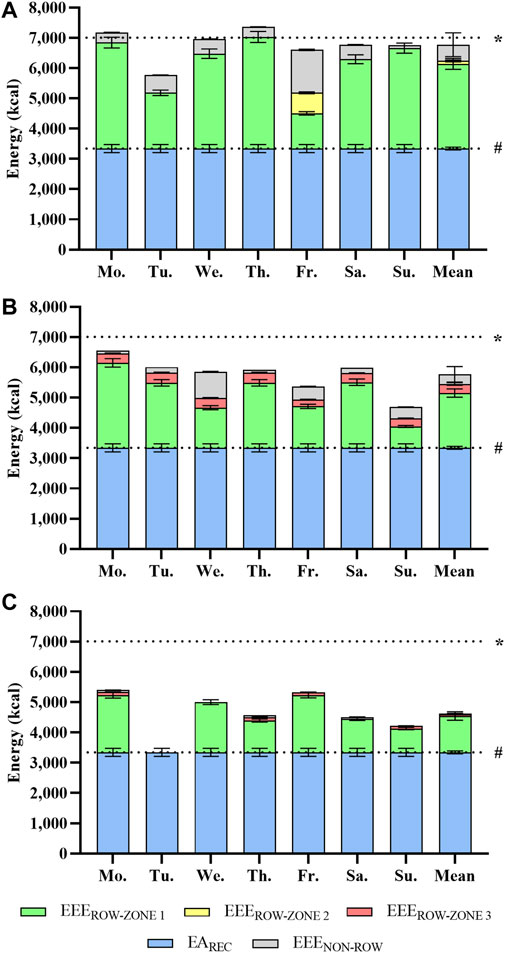
FIGURE 1. Total energy requirement by exercise energy expenditure and recommended energy availability (EAREC) for an exemplary high volume (A), high intensity (B), and tapering rowing training week (C) (mean [95% confidence interval]). Calculation of exercise energy expenditure for rowing (EEEROW) training based on a non-protein table (Péronnet and Massicotte, 1991) and corrected for resting metabolic rate (RMR) and anaerobic energy contribution (Di Prampero and Ferretti, 1999). EEE for other training (EEENON-ROW) is approximated using corrected MET data (Kozey et al., 2010). EAREC (dashed line #) is given as 40 kcal·kg−1 fat free mass·day−1 (Koehler et al., 2016). A total energy expenditure of three times the RMR (7,011 kcal·day−1, dashed line *) was assumed to reflect the upper limit of the manageable total energy expenditure (Thurber et al., 2019).
Figure 2 illustrates the accumulated EEEROW as a function of rowing training duration for each intensity zone.
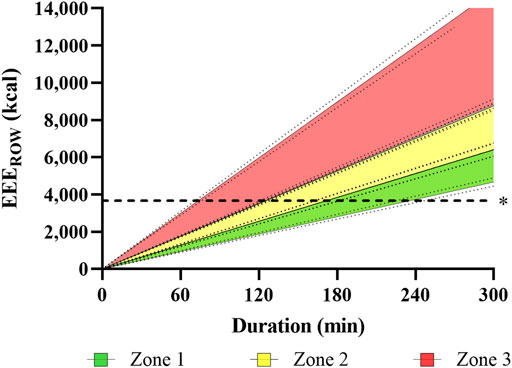
FIGURE 2. Illustration of estimated, accumulated exercise energy expenditure over time during rowing (EEEROW) for a three-zone model, based on indirect calorimetry data obtained in incremental step testing. The Figure visualizes accumulated EEE for each training zone over time, assuming a linear progression with 95% confidence intervals. R2 ranged 0.968–0.988. Dashed line represents EEE of 3,668 kcal·day−1, 95% CI [3,580, 3,756], which is assumably the maximum refuelable EEE.
Figure 3 illustrates the muscle glycogen depletion as a function of the intensity zones, utilization rate of carbohydrates, and duration of rowing training. Approximated blood glucose, liver, and muscle glycogen stores amounted to 6 g, 95% CI [5, 6], 109 g, 95% CI (104, 114) to 201 g, 95% CI (193, 210), and 607 g, 95% CI [580, 634] to 850 g, 95% CI [812, 888], respectively. In total, this corresponded to 2,959 kcal, 95% CI [2,828, 3,091] to 4,333 kcal, 95% CI [4,141, 4,526].
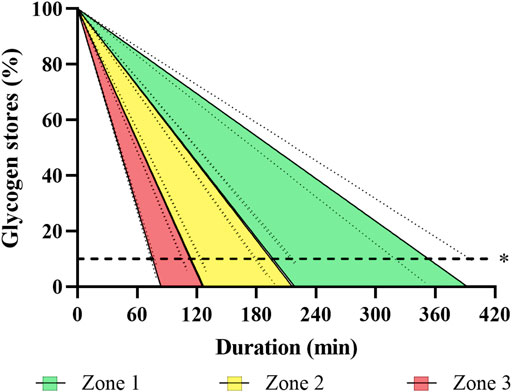
FIGURE 3. Illustration of estimated glycogen depletion over time during rowing for a three-zone model, based on indirect calorimetry data obtained in incremental step testing. The Figure visualizes depletion of individually estimated glycogen stores (including blood, liver, and muscle; see text for details) over time, assuming a linear progression with 95% confidence intervals. R2 ranges 0.781–0.985. *Dashed line represents the 90% maximum depletion threshold of glycogen stores (Burke et al., 2017).
Discussion
We studied EEE and substrate utilization of ergometer rowing in elite male open-class rowers. Indirect calorimetry data were obtained during repeated incremental step and ramp testing to calculate EEEROW and substrate utilization in three intensity zones. Further, we approximated daily TER as the sum of EEEROW, EEENON-ROW, and EAREC for prototypical high volume, high intensity, and tapering training weeks.
The main results are a high EEEROW in elite open-class male rowers ranging 15.6 kcal·min−1, 95% CI [14.8, 16.3] to 48.8 kcal·min−1, 95% CI [48.1, 51.6] from zone 1 to 3. The energetic contribution of fat metabolism was never higher than 53.6 ± 7.6%. Furthermore, we calculated a high TER ranging 4,626 kcal∙day−1, 95% CI [4,481, 4,771] to 6,775 kcal·day−1, 95% CI [6,651, 6,898] during prototypical training weeks. This indicates that isocaloric energy intake may become challenging during high volume training weeks and points to a metabolic limitation of reasonable rowing training volume.
Metabolic Demand of Ergometer Rowing
The metabolic demand within ergometer rowing is already high at LT1 and LT2. The mechanical power output at these “thresholds” (Table 2) corresponds well with previous data published for elite male open-class rowers (Tran et al., 2015). However, for the first time, we report V̇O2- and RER-data aligned to these points as well as to V̇O2max. While the high metabolic demand at V̇O2max is apparently related to the remarkably high absolute aerobic capacity of elite male open-class rowers, sub-maximum V̇O2 data at LT1 and LT2 are far more interesting. Here, the metabolic demand is considerably higher than corresponding results for e.g., cyclists, ranging 3.6–4.3 L·min−1 (Garvican et al., 2013). This is attributable to the relatively large body dimensions of rowers, especially their high body and muscle mass. Nevertheless, also a particularly of ergometer rowing — or more exactly its measurement — contributes to this phenomenon: During the drive phase, when the rower pushes off from the foot stretcher with his legs and moves backwards on his sliding seat, the rower exerts force on the handle or oar and this force is measured. Afterwards, the rower has to bring himself back to the starting position, which also requires force and energy. This additional mechanical power output—amounting to roughly 38 W (Mentz et al., 2021)—is not measured by common ergometers. Consequently, the actual mechanical power output is underestimated and the metabolic load of ergometer rowing vs. cycling at a given (displayed power output is considerably higher (Turner and Rice, 2021). Thus, the mechanical efficiency is significantly lower in ergometer rowing vs. cycling (Lindenthaler et al., 2018). Nevertheless, it is worth mentioning that ergometer rowing has been reported to induce similar metabolic stress as on-water rowing (Vogler et al., 2010). Another factor that contributes to the high V̇O2 data at LT1, LT2, and V̇O2max during rowing is the high amount of muscle mass recruited and the high venous return due to the sitting position of the rower (Volianitis and Secher, 2009), thereby resulting in a relatively high EEE at any given mechanical power output.
Metabolic Demand of Ergometer Rowing at Low, Moderate, and High Intensities
The V̇O2max percentages derived from the lactate thresholds in our study fit well to previous categorizations (Seiler, 2010), underlining the validity of our data that allowed us to estimate the metabolic demand within each zone of the three-zone model (Table 2). EEEROW in all intensity zones was apparently high, ranging from 15.6 kcal·min−1, 95% [14.8.16.3] to 49.8 kcal·min−1, 95% [48.1, 51.6], equaling to an 10- to 31-fold increase in RMR, respectively. It seems worth to highlight that a “basic” endurance training in an elite open-class male rower at the upper range of zone 1 around LT1 already necessitates a V̇O2 of 4.4 L·min−1 something that is simply not possible for e.g., an elite distance runner with a V̇O2max of 4.2 L·min−1 (Jones et al., 2021). This illustrates the energetic differences between these elite athletes of different endurance sports disciplines.
However, aside from EEE, substrate utilization is of particular importance to rate the metabolic stress of exercise. While we estimate lipid stores in this sample of elite open-class male rowers as high as ∼ 77,000 kcal (9.3 kcal·g−1 x 8,300 g body fat mass), maximum energy stores from glycogen and blood glucose are limited to 2,959 kcal, 95% CI [2,828, 3,091] to 4,333 kcal, 95% CI [4,141, 4,526] with almost perfectly filled glycogen stores. Since glycogen stores largely depend on the loading status and assuming that no more than 90% depletion of the initial muscle glycogen store are tolerable (Burke et al., 2017), the lower value seems to reflect a more realistic scenario.
Our data indicate that energy during ergometer rowing training is mainly derived from carbohydrates, because even during a zone 1 training, about 47–68% of the energy originates from carbohydrates equaling 1.8 g·min−1, 95% CI [1.6, 2.0] to 3.5 g·min−1, 95% CI [3.2, 3.8]. Leaving aside other factors of fatigue or refueling, available energy stores from glycogen and blood glucose in this sample of elite rowers will theoretically allow for about 5.7 h, 95% CI [5.1, 6.3] to 3.1 h, 95% CI [2.8, 3.4] training in zone 1. In zone 2 and 3 theoretical maximum duration will be reduced by 47–74% (3.1 h, 95% CI [2.8, 3.4] to 1.8 h, 95% CI [1.6, 1.9]) and 70–86% (1.8 h, 95% CI [1.6, 1.9] to 1.0 [0.8, 1.0]), respectively. Again, due to other factors of fatigue and the unlikelihood of perfectly filled glycogen stores, the lower values are more realistic for common training scenarios.
Especially long-distance runners and cyclists try to maximize the fat oxidation rate through very low training intensities (Jeukendrup and Achten, 2001). Due to the high utilization of CHO observed in our rowers, such a “FatMax” training is apparently unlikely to be realized even during low zone 1 rowing. In line with this notion, Dandanell and colleagues (Dandanell et al., 2018) reported FatMax at 46% of V̇O2max in highly trained cross-country skiers. That is about 6% lower than the bottom of zone 1 training in our study (Table 3). While beyond the scope of this study, it seems worth to evaluate if such low intensities are warranted to prepare for high-intensity rowing races lasting not more than 7 min (Messonnier et al., 2005b).
Metabolic Demand of Rowing Training Sessions
Based on the metabolic demand at each intensity zone, we calculated the EEEROW and substrate utilization for prototypical rowing training sessions and weeks, assuming that the metabolic demand of ergometer rowing generally reflects on water rowing (Vogler et al., 2010). Due to the high metabolic demand, a long basic endurance session lasting 100 min constantly rowed in zone 1 will result in an EEEROW ranging from 1,556 kcal, 95% CI [1,479, 1,670] to 2,133 kcal, 95% CI [2,019, 2,300]. Notably, such rowing sessions — at least in German rowing — are usually not performed at intensities considerably lower than LT1, because this would not allow to row the boat with the targeted propulsion and speed, corresponding to 72–80% of a boat’s world best time (Schwarzrock et al., 2017). Hence, the upper limit of the range is more likely to reflect the EEEROW of a realistic, 100-min basic endurance rowing session.
During such a 100-min zone 1 rowing session, the carbohydrate stores (muscle and liver glycogen as well as blood glucose) of the rowers will deplete with a degradation rate of 2.2 g·min−1, 95% CI [2.0, 2.4] to 3.9 g·min−1, 95% CI [3.6, 4.2], when accounting for EEE and RMR. This corresponds to a reduction to 75%, 95% CI [72, 77] to 54%, 95% CI [50, 59] of initial values (Figure 3)—with the latter being more realistic, as discussed before. Similar results were reported by Stepto and colleagues (Stepto et al., 2001) for aerobic interval training in competitive cyclists with muscle glycogen depletion ranging from 36 to 64%. Hence, not only the muscular impact of long rowing endurance sessions is demanding - they also include the risk of glycogen depletion. Consequently, sufficient carbohydrate ingestion during such exercises seems crucial to maintain glucose availability and pace. Importantly, in case of insufficient refueling, low pre-exercise muscle glycogen concentrations will not only result in reduced high-intensity performance (Maughan and Poole, 1981), but might also compromise immune function and training readiness (Stellingwerff et al., 2011).
Metabolic Demand During Prototypical Training Weeks
Elite rowers regularly perform 2-4 sessions a day and they usually train for at least 6 days a week (Tran et al., 2015). To estimate the TER, we added the accumulated EEE of EEEROW and EEENON-ROW to the resting metabolic rate and EAREC. As shown in Figure 1 this leads to an energy demand that increases from tapering week, to high-intensity week, and finally peaks in the high-volume week with 6,778 kcal·day−1, 95% CI [6,651, 6,905]. If we assume that zone 1 training is in fact performed at the upper end of its intensity range, TER for the high-volume week would be even higher, and amount to 7,231 kcal·days-1, 95% CI [7,082, 7,380]. This is three times the resting metabolic rate of 2,337 kcal·day-1, 95% CI [2,262, 2,411].
While these TER values are very high, results from other sports underline their plausibility. Aside from extreme values of 9,869 ± 4,129 or 11,246 ± 1,083 kcal·day−1 reported during ultra-endurance competitions (Heydenreich et al., 2017; Geesmann et al., 2014), high caloric needs were also reported for elite male cross-country skiers. Sjödin and colleagues (Sjödin et al., 1994), for example, determined TEE via double labelled water during a 6-days training camp with an average training volume of 212 min·day−1 to be as high as 7,213 ± 1,003 kcal·day−1, notably with a slightly negative energy balance of -0.6%. These data fit well to TER estimated in our elite open-class male rowers. The “manageable” TEE during daily training conditions by resupplied energy through nutrition can be assumed to be three times the RMR (Thurber et al., 2019), amounting to 7,011 kcal·day−1, 95% CI [6,787, 7,234] in our elite open-class rowers (Figure 1). Assuming an EAREC of 3,343 kcal·day−1, 95% CI [3,207, 3,479], about 3,668 kcal·day−1, 95% CI [3,580, 3,756] will remain for EEE (Figure 2). Hence, it becomes apparent that high-volume training of 1,605 min·week−1 in elite open-class male rowers is at the upper end of what is energetically possible or–more precisely–sustainable. Moreover, insufficient dietary energy intake with suppressed energy availability can disrupt different aspects of homeostasis in humans, a topic which is discussed as relative energy deficiency in sport (RED-S) (Mountjoy et al., 2018) and the female athlete triad (De Souza et al., 2014; De Souza et al., 2019a; De Souza et al., 2019b), both providing theoretical frameworks for physiological dysregulations and adverse effects on training capacity, performance, and health.
Considering the high EEEROW and the suspected need to exercise with relatively high intensities in order to provide an effective stimulus for improvements of already highly trained athletes (Treff et al., 2021a), it becomes clear that the capacity of elite athletes to complete high training volume is relatively lower than in untrained persons. The untrained can exercise with a lower absolute intensity, therefore need less energy and yet profit more from low metabolic demands. This dilemma highlights the limiting role of a high TEE in elite sports.
Practically, it is worth to consider that the differences in TER calculated for the three exemplary training weeks require an adjustment of energy intake. The latter is known as periodized nutrition (Jeukendrup, 2017). To account for fluctuations in training load, periodized nutrition necessitates at least an approximated TER. Thereby, estimates of an adequate reduction in energy intake from a high intensity to a tapering week can prevent unwarranted body mass gain. Vice versa, periodized nutrition based on valid data can help to prevent energy deficiency or RED-S (Mountjoy et al., 2018).
Our data also indicate that availability and recovery of glycogen stores may be a key factor for successful rowing training. With a mean rate of muscle glycogen synthesis ranging 5–6 mmol·kg−1·h−1 of wet muscle mass, normalization of muscle glycogen levels after extreme depletion will require 20–24 h (Coyle, 1991). Consequently, the calculated depletion to 75–54% of initial glycogen and blood glucose energy stores after 100 min zone 1 rowing training is unlikely to be sufficiently restored between daily training sessions. Especially during high volume training camps with repeated sessions on consecutive days (Table 3), athletes may not be able to fully restore muscle glycogen. Hence, glycogen concentration in active muscle will fall progressively over the day or a period of days. This may accidentally lead to a situation where glycogen stores are low in the second or third daily training session, where the achievable metabolic rate and exercise capacity are limited, because fat and protein metabolism are augmented. This is — in the end—an extended catabolic situation.
Practical Implications
Due to the dominant contribution of carbohydrates during basic endurance training, their availability through restoration of glycogen stores and immediate provision is warranted during low-intensity rowing training lasting longer than 60 min. Of note, and in contrast to e.g., cyclists or triathletes (Sareban et al., 2016), such procedures seem to be not very common in the rowing culture, at least according to our own observations. Daily recommendation for the macronutrients range from 6–12 g carbohydrates ·kg−1 body mass·day−1, 1.5–1.7 g protein·kg−1 body mass·day−1, and 0.8–2.0 g fat·kg−1 body mass·day−1 (Stellingwerf et al., 2011). If athletes fail repeatedly to consume the recommended amounts of dietary carbohydrates (Baranauskas et al., 2015), the EEE of rowing may become a limiting factor. We recommend to consider this when planning training and nutrition for rowers. Otherwise, EEE and TER might surpass the maximum energy intake leading to potentially adverse effects for both health and performance.
Limitations
As the intensity zones were calculated based on lactate "thresholds," we want to underline that we do not uncritically use the threshold term, and that we are aware of the undeniable changes associated with its conceptual basis (Poole et al., 2021). However, LT1 and LT2 still provide useful key measures for exercise description and definition of intensity zones.
We approximated EEEROW based on repeated ergometer tests, EEENON-ROW via corrected METs and we calculated EAREC. Due to the assumptions underlying such calculations, uncertainties are inherent. However, the repeated measurements in elite athletes during highly standardized laboratory conditions provide a previously unseen data quality. The transfer of ergometer rowing to on-water rowing may also be deemed a limitation, however Vogler and colleagues (Vogler et al., 2010) reported strong correlations between ergometer vs. on-water based incremental tests (blood lactate concentration r = 0.84, V̇O2 r = 0.91) and trivial to small differences of metabolic variables (blood lactate concentration −4.4% to 23.1%, V̇O2 −1.1% to −1.2%). Nevertheless, further individual validation seems warranted.
Notably, we simplified our calculations, assuming continuous, even paced steady-state training sessions with constant EEE and substrate utilization, neglecting that real-life training sessions are often characterized by changing pace, cardiovascular drift, and slow component kinetics in V̇O2 for intensities above LT1, surely causing fluctuations in EEE and substrate utilization. Finally, it was shown that the percentage of training spent below zone 1 may be as high as 30% of the total training time (Treff et al., 2021a), thereby suggesting another real-life scenario we did not account for. Though including the lactate equivalent, we did not precisely account for the oxidation of lactate, which spares glucose and glycogen during intense exercise (Miller et al., 2002) and we did not include the impact of lactate as a gluconeogenic precursor, as we are not aware of any data for sufficiently precise quantification that may be applied for our context.
Although our projections of EEE, TER, and substrate utilization during different training scenarios were made on the basis of highly unique data set of laboratory data designed to closely monitor real-life scenarios in elite athletes and include well-established literature references, external validation is warranted. The current gold standard for quantifying TEE that can be implemented in free-living individuals with minimal subject burden is the doubly labelled water method (Ainslie et al., 2003). In contrast, techniques to measure substrate utilization and specifically glycogen depletion are to this date highly invasive and require repeated collection of muscle biopsies (Hearris et al., 2018) and highly specialized imaging equipment and training (van Zijl et al., 2007). Future studies in elite rowers should aim to include these measures to confirm our predictions.
Conclusion
Due to their large body dimensions and high metabolic capacity with high V̇O2max, the outstanding metabolic demand of rowing, and high training loads, the energy expenditure of elite open-class male rowers is extraordinarily high. At least in high volume weeks a metabolic limitation is likely, as the energy need is at the upper range of the maximum daily nutrient intake.
Importantly, the percentage of carbohydrates oxidized during slow long-distance rowing training is always dominant and rowing training mainly utilizing fat metabolism seems unrealistic. A single training session is suspected to cause a relevant glycogen depletion. Therefore, we recommend the consumption of carbohydrates during long rowing session and a systematic refilling between daily sessions. These notions should also be considered when planning training volume at a given intensity and the timing of individual sessions, because otherwise hypocaloric conditions and adverse effects are likely.
Data Availability Statement
The raw data supporting the conclusions of this article will be made available by the authors, without undue reservation.
Ethics Statement
The studies involving human participants were reviewed and approved by Ethical board Ulm University. The patients/participants provided their written informed consent to participate in this study.
Author Contributions
KW collecte data, performed the calculations, statistical analysis and wrote the first draft of the manuscript. JS contributed to manuscript revision. KK contributed to the calculations, manuscript revision. GT performed the conception and design of the study, collected data, and revised the manuscript.
Conflict of Interest
The authors declare that the research was conducted in the absence of any commercial or financial relationships that could be construed as a potential conflict of interest.
Publisher’s Note
All claims expressed in this article are solely those of the authors and do not necessarily represent those of their affiliated organizations, or those of the publisher, the editors and the reviewers. Any product that may be evaluated in this article, or claim that may be made by its manufacturer, is not guaranteed or endorsed by the publisher.
Acknowledgments
The authors would like to acknowledge the contribution of Mark Amort (Olympic Training Center Hamburg) and our former colleague Nadine Rolser to the project. Furthermore, we highly appreciate the collaboration with all athletes and coaches, especially Marcus Schwarzrock. We also acknowledge the statistical advice of Benjamin Mayer.
Supplementary Material
The Supplementary Material for this article can be found online at: https://www.frontiersin.org/articles/10.3389/fphys.2022.829757/full#supplementary-material
Abbreviations
EEE, Exercise energy expenditure; EEEROW, Exercise energy expenditure during rowing; EEENON-ROW, Exercise energy expenditure during training other than rowing; EAREC, Recommended energy availability; MET, Metabolic equivalent of task; TEE, Total energy expenditure; TER, Total energy requirement.
References
Ainslie P. N., Reilly T., Westerterp K. R. (2003). Estimating Human Energy Expenditure. Sports Med. 33, 683–698. doi:10.2165/00007256-200333090-00004
Ainsworth B. E., Haskell W. L., Herrmann S. D., Meckes N., Bassett D. R., Tudor-Locke C., et al. (2011). 2011 Compendium of Physical Activities. Med. Sci. Sports Exerc. 43, 1575–1581. doi:10.1249/MSS.0b013e31821ece12
Areta J. L., Taylor H. L., Koehler K. (2020). Low Energy Availability: History, Definition and Evidence of its Endocrine, Metabolic and Physiological Effects in Prospective Studies in Females and Males. Eur. J. Appl. Physiol. 121, 1–21. doi:10.1007/s00421-020-04516-0
Baranauskas M., Stukas R., Tubelis L., Žagminas K., Šurkienė G., Švedas E., et al. (2015). Nutritional Habits Among High-Performance Endurance Athletes. Medicina 51, 351–362. doi:10.1016/j.medici.2015.11.004
Bizjak D. A., Zügel M., Treff G., Winkert K., Jerg A., Hudemann J., et al. (2021). Effects of Training Status and Exercise Mode on Global Gene Expression in Skeletal Muscle. IJMS 22, 12578. doi:10.3390/ijms222212578
Boegman S., Dziedzic C. E. (2016). Nutrition and Supplements for Elite Open-Weight Rowing. Curr. Sports Med. Rep. 15, 252–261. doi:10.1249/JSR.0000000000000281
Bourdon P. C., Cardinale M., Murray A., Gastin P., Kellmann M., Varley M. C., et al. (2017). Monitoring Athlete Training Loads: Consensus Statement. Int. J. Sports Physiol. Perform. 12, S2–S161. doi:10.1123/IJSPP.2017-0208
Braakhuis A. J., Hopkins W. G., Lowe T. E. (2013). Effect of Dietary Antioxidants, Training, and Performance Correlates on Antioxidant Status in Competitive Rowers. Int. J. Sports Physiol. Perform. 8, 565–572. doi:10.1123/ijspp.8.5.565
Brooks G. A., Trimmer J. K. (1996). Glucose Kinetics during High-Intensity Exercise and the Crossover Concept. J. Appl. Physiol. 80, 1073–1075. doi:10.1152/jappl.1996.80.3.1073
Burke L. M., van Loon L. J. C., Hawley J. A. (2017). Postexercise Muscle Glycogen Resynthesis in Humans. J. Appl. Physiol. 122, 1055–1067. doi:10.1152/japplphysiol.00860.2016
Carlsohn A., Scharhag-Rosenberger F., Cassel M., Mayer F. (2011). Resting Metabolic Rate in Elite Rowers and Canoeists: Difference between Indirect Calorimetry and Prediction. Ann. Nutr. Metab. 58, 239–244. doi:10.1159/000330119
Coyle E. F. (1991). Timing and Method of Increased Carbohydrate Intake to Cope with Heavy Training, Competition and Recovery. J. Sports Sci. 9, 29–52. doi:10.1080/02640419108729865
Cunningham J. J. (1980). A Reanalysis of the Factors Influencing Basal Metabolic Rate in normal Adults. Am. J. Clin. Nutr. 33, 2372–2374. doi:10.1093/ajcn/33.11.2372
Dandanell S., Meinild-Lundby A.-K., Andersen A. B., Lang P. F., Oberholzer L., Keiser S., et al. (2018). Determinants of Maximal Whole-Body Fat Oxidation in Elite Cross-Country Skiers: Role of Skeletal Muscle Mitochondria. Scand. J. Med. Sci. Sports 28, 2494–2504. doi:10.1111/sms.13298
De Souza M. J., Koltun K. J., Williams N. I. (2019a). The Role of Energy Availability in Reproductive Function in the Female Athlete Triad and Extension of its Effects to Men: An Initial Working Model of a Similar Syndrome in Male Athletes. Sports Med. 49, 125–137. doi:10.1007/s40279-019-01217-3
De Souza M. J., Koltun K. J., Williams N. I. (2019b). What Is the Evidence for a Triad-like Syndrome in Exercising Men? Curr. Opin. Physiol. 10, 27–34. doi:10.1016/j.cophys.2019.04.002
De Souza M. J., Nattiv A., Joy E., Misra M., Williams N. I., Mallinson R. J., et al. (2014). 2014 Female Athlete Triad Coalition Consensus Statement on Treatment and Return to Play of the Female Athlete Triad: 1st International Conference Held in San Francisco, California, May 2012 and 2nd International Conference Held in Indianapolis, Indiana, May 2013. Br. J. Sports Med. 48, 289. doi:10.1136/bjsports-2013-093218
Di Prampero P. E., Cortili G., Celentano F., Cerretelli P. (1971). Physiological Aspects of Rowing. J. Appl. Physiol. 31, 853–857. doi:10.1152/jappl.1971.31.6.853
Di Prampero P. E., Ferretti G. (1999). The Energetics of Anaerobic Muscle Metabolism: a Reappraisal of Older and Recent Concepts. Respiration Physiol. 118, 103–115. doi:10.1016/s0034-5687(99)00083-3
Dickhuth H. H., Huonker M., Münzel T. (1991). “Individual Anaerobic Threshold for Evaluation of Competitive Athletes and Patients with Left Ventricular Dysfunctions,” in Hg. 1991 – Advances in Ergometry. Editor N. Bachl. 173–179. doi:10.1007/978-3-642-76442-4_26
Ekf-diagnostic GmbH (2013). Biosen C-Line - Clinic / GP+ Bedienungsanleitung. Unpublished manuscript, last modified 07/2013.
Fiskerstrand A., Seiler K. S. (2004). Training and Performance Characteristics Among Norwegian International Rowers 1970-2001. Scand. J. Med. Sci. Sports 14, 303–310. doi:10.1046/j.1600-0838.2003.370.x
Garvican L. A., Ebert T. R., Quod M. J., Gardner S. A., Gregory J., Osborne M. A., et al. (2013). “High-Performance Cyclists,” in Physiological Tests for Elite Athletes Champaign: Human Kinetics, 299–322. Editors R. Tanner, and C. Gore. 2nd ed.
Geesmann B., Mester J., Koehler K. (2014). Energy Balance, Macronutrient Intake, and Hydration Status during a 1,230 Km Ultra-endurance Bike marathon. Int. J. Sport Nutr. Exerc. Metab. 24, 497–506. doi:10.1123/ijsnem.2013-0169
Hawley J. A., Lundby C., Cotter J. D., Burke L. M. (2018). Maximizing Cellular Adaptation to Endurance Exercise in Skeletal Muscle. Cel. Metab. 27, 962–976. doi:10.1016/j.cmet.2018.04.014
Hearris M., Hammond K., Fell J., Morton J. (2018). Regulation of Muscle Glycogen Metabolism during Exercise: Implications for Endurance Performance and Training Adaptations. Nutrients 10, 298. doi:10.3390/nu10030298
Hecksteden A., Skorski S., Schwindling S., Hammes D., Pfeiffer M., Kellmann M., et al. (2016). Blood-Borne Markers of Fatigue in Competitive Athletes - Results from Simulated Training Camps. PloS One 11, e0148810. doi:10.1371/journal.pone.0148810
Heydenreich J., Kayser B., Schutz Y., Melzer K. (2017). Total Energy Expenditure, Energy Intake, and Body Composition in Endurance Athletes across the Training Season: A Systematic Review. Sports Med. - Open 3, 8. doi:10.1186/s40798-017-0076-1
Jeukendrup A., Achten J. (2001). Fatmax : A New Concept to Optimize Fat Oxidation during Exercise? Eur. J. Sport Sci. 1, 1–5. doi:10.1080/17461390100071507
Jeukendrup A. E. (2017). Periodized Nutrition for Athletes. Sports Med. 47, 51–63. doi:10.1007/s40279-017-0694-2
Jones A. M., Kirby B. S., Clark I. E., Rice H. M., Fulkerson E., Wylie L. J., et al. (2021). Physiological Demands of Running at 2-hour marathon Race Pace. J. Appl. Physiol. 130, 369–379. doi:10.1152/japplphysiol.00647.2020
Kellmann M., Altenburg D., Lormes W., Steinacker J. M. (2001). Assessing Stress and Recovery during Preparation for the World Championships in Rowing. Sport Psychol. 15, 151–167. doi:10.1123/tsp.15.2.151
Kerr D. A., Ross W. D., Norton K., Hume P., Kagawa M., Ackland T. R. (2007). Olympic Lightweight and Open-Class Rowers Possess Distinctive Physical and Proportionality Characteristics. J. Sports Sci. 25, 43–53. doi:10.1080/02640410600812179
Koehler K., Hoerner N. R., Gibbs J. C., Zinner C., Braun H., De Souza M. J., et al. (2016). Low Energy Availability in Exercising Men Is Associated with Reduced Leptin and Insulin but Not with Changes in Other Metabolic Hormones. J. Sports Sci. 34, 1921–1929. doi:10.1080/02640414.2016.1142109
Kohler G., Boutellier U. (2004). Bestimmung der Genauigkeit von Blutlaktatmessgeräten am Beispiel des Laktatanalyzer Biosen 5040. Schweizerische Z. für «Sportmedizin Sporttraumatologie 52, 149–153.
Kozey S., Lyden K., Staudenmayer J., Freedson P. (2010). Errors in MET Estimates of Physical Activities Using 3.5 Ml·kg−1·min−1 as the Baseline Oxygen Consumption. J. Phys. Activity Health 7, 508–516. doi:10.1123/jpah.7.4.508
Larsson P. U., Wadell K. M. E., Jakobsson E. J. I., Burlin L. U., Henriksson-Larsén K. B. (2004). Validation of the MetaMax II Portable Metabolic Measurement System. Int. J. Sports Med. 25, 115–123. doi:10.1055/s-2004-819953
Lawton T. W., Cronin J. B., McGuigan M. R. (2013). Strength, Power, and Muscular Endurance Exercise and Elite Rowing Ergometer Performance. J. Strength Conditioning Res. 27, 1928–1935. doi:10.1519/JSC.0b013e3182772f27
Lindenthaler J. R., Rice A. J., Versey N. G., McKune A. J., Welvaert M. (2018). Differences in Physiological Responses during Rowing and Cycle Ergometry in Elite Male Rowers. Front. Physiol. 9, 1010. doi:10.3389/fphys.2018.01010
Loucks A. B. (2013). “Energy Balance and Energy Availability,” in The Encyclopaedia of Sports Medicine. Editor R. J. Maughan (Chichester, UK: John Wiley & Sons), 72–87. doi:10.1002/9781118692318.ch5
Maciejewski H., Bourdin M., Féasson L., Dubouchaud H., Messonnier L. A. (2020). Non-oxidative Energy Supply Correlates with Lactate Transport and Removal in Trained Rowers. Int. J. Sports Med. 41, 936–943. doi:10.1055/a-1186-1761
Mader A., Hollmann W. (1977). Zur Bedeutung der Stoffwechselleistungsfähigkeit des Eliteruderers im Training und Wettkampf. Leistungssport 9, 8–62.
Manunzio C., Mester J., Kaiser W., Wahl P. (2016). Training Intensity Distribution and Changes in Performance and Physiology of a 2nd Place Finisher Team of the Race across America over a 6 Month Preparation Period. Front. Physiol. 7, 642. doi:10.3389/fphys.2016.00642
Margaria R., Cerretelli P., Diprampero P. E., Massari C., Torelli G. (1963). Kinetics and Mechanism of Oxygen Debt Contraction in Man. J. Appl. Physiol. 18, 371–377. doi:10.1152/jappl.1963.18.2.371
Maughan R. J., Poole D. C. (1981). The Effects of a Glycogen-Loading Regimen on the Capacity to Perform Anaerobic Exercise. Europ. J. Appl. Physiol. 46, 211–219. doi:10.1007/BF00423397
Mentz L., Engleder T., Schulz G., Winkert K., Steinacker J. M., Treff G. (2020). The Mechanical Rower: Construction, Validity, and Reliability of a Test Rig for Wind Braked Rowing Ergometers. J. Biomech. 106, 109833. doi:10.1016/j.jbiomech.2020.109833
Mentz L., Winkert K., Treff G., Steinacker J. M. (2021). Determining the Mechanical Work and Power of the Recovery Phase in Ergometer Rowing [Conference presentation]. 2022 ACSM Annual Meeting. San Diego, CA, United States.
Messonnier L., Aranda-Berthouze S. E., Bourdin M., Bredel Y., Lacour J.-R. (2005a). Rowing Performance and Estimated Training Load. Int. J. Sports Med. 26, 376–382. doi:10.1055/s-2004-821051
Messonnier L., Denis C., Prieur F., Lacour J.-R. (2005b). Are the Effects of Training on Fat Metabolism Involved in the Improvement of Performance during High-Intensity Exercise? Eur. J. Appl. Physiol. 94, 434–441. doi:10.1007/s00421-005-1325-4
Midgley A. W., McNaughton L. R., Polman R., Marchant D. (2007). Criteria for Determination of Maximal Oxygen Uptake. Sports Med. 37, 1019–1028. doi:10.2165/00007256-200737120-00002
Miller B. F., Fattor J. A., Jacobs K. A., Horning M. A., Navazio F., Lindinger M. I., et al. (2002). Lactate and Glucose Interactions during Rest and Exercise in Men: Effect of Exogenous Lactate Infusion. J. Physiol. 544, 963–975. doi:10.1113/jphysiol.2002.027128
Mountjoy M., Sundgot-Borgen J. K., Burke L. M., Ackerman K. E., Blauwet C., Constantini N., et al. (2018). IOC Consensus Statement on Relative Energy Deficiency in Sport (RED-S): 2018 Update. Br. J. Sports Med. 52, 687–697. doi:10.1136/bjsports-2018-099193
Nielsen H. B., Christensen P. M. (2020). Rower with Danish Record in Maximal Oxygen Uptake. Ugeskr Laeger 182, V10190610.
Nielsen H. B. (1999). pH after Competitive Rowing: the Lower Physiological Range? Acta Physiol. Scand. 165, 113–114. doi:10.1046/j.1365-201x.1999.00485.x
Péronnet F., Massicotte D. (1991). Table of Nonprotein Respiratory Quotient: an Update. Can. J. Sport Sci. = J. canadien des Sci. du Sport 16, 23–29.
Plews D. J., Laursen P. B., Kilding A. E., Buchheit M. (2014). Heart-rate Variability and Training-Intensity Distribution in Elite Rowers. Int. J. Sports Physiol. Perform. 9, 1026–1032. doi:10.1123/ijspp.2013-0497
Poole D. C., Rossiter H. B., Brooks G. A., Gladden L. B. (2021). The Anaerobic Threshold: 50+ Years of Controversy. J. Physiol. 599, 737–767. doi:10.1113/JP279963
Pripstein L. P., Rhodes E. C., McKenzie D. C., Coutts K. D. (1999). Aerobic and Anaerobic Energy during a 2-km Race Simulation in Female Rowers. Eur. J. Appl. Physiol. 79, 491–494. doi:10.1007/s004210050542
Rapoport B. I. (2010). Metabolic Factors Limiting Performance in marathon Runners. Plos Comput. Biol. 6, e1000960. doi:10.1371/journal.pcbi.1000960
Roth W., Hasart E., Wolf W., Pansold B. (1983). Untersuchungen zur Dynamik der Energiebereitstellung waehrend maximaler Mittelzeitausdauerbelastung. Medizin und Sport 23, 107–114.
Roth W., Schwanitz P., Pas P., Bauer P. (1993). Force-time Characteristics of the Rowing Stroke and Corresponding Physiological Muscle Adaptations. Int. J. Sports Med. 14 (Suppl. 1), S32–S34. doi:10.1055/s-2007-1021221
Sareban M., Zügel D., Koehler K., Hartveg P., Zügel M., Schumann U., et al. (2016). Carbohydrate Intake in Form of Gel Is Associated with Increased Gastrointestinal Distress but Not with Performance Differences Compared with Liquid Carbohydrate Ingestion during Simulated Long-Distance Triathlon. Int. J. Sport Nutr. Exerc. Metab. 26, 114–122. doi:10.1123/ijsnem.2015-0060
Schwarzrock M., Treff G., Viedt C., Bielig B., Reyher D., Meyer-Kopp H., et al. (2017). Trainingsmethodische Grundkonzeption 2016-2020. Hannover.
Seiler S. (2010). What Is Best Practice for Training Intensity and Duration Distribution in Endurance Athletes? Int. J. Sports Physiol. Perform. 5, 276–291. doi:10.1123/ijspp.5.3.276
Sjödin A. M., Andersson A. B., Högberg J. M., Westerterp K. R. (1994). Energy Balance in Cross-Country Skiers: a Study Using Doubly Labeled Water. Med. Sci. Sports Exerc. 26, 720–724. doi:10.1249/00005768-199406000-00011
Sperlich B., Becker M., Hotho A., Wallmann-Sperlich B., Sareban M., Winkert K., et al. (2017). Sedentary Behavior Among National Elite Rowers during Off-Training-A Pilot Study. Front. Physiol. 8, 655. doi:10.3389/fphys.2017.00655
Steinacker J. M. (1993). Physiological Aspects of Training in Rowing. Int. J. Sports Med. 14 (Suppl. 1), S3–S10. doi:10.1055/s-2007-1021214
Stellingwerff T., Maughan R. J., Burke L. M. (2011). Nutrition for Power Sports: Middle-Distance Running, Track Cycling, Rowing, Canoeing/kayaking, and Swimming. J. Sports Sci. 29 (Suppl. 1), S79–S89. doi:10.1080/02640414.2011.589469
Stepto N. K., Martin D. T., Fallon K. E., Hawley J. A. (2001). Metabolic Demands of Intense Aerobic Interval Training in Competitive Cyclists. Med. Sci. Sports Exerc. 33, 303–310. doi:10.1097/00005768-200102000-00021
Thurber C., Dugas L. R., Ocobock C., Carlson B., Speakman J. R., Pontzer H. (2019). Extreme Events Reveal an Alimentary Limit on Sustained Maximal Human Energy Expenditure. Sci. Adv. 5, eaaw0341. doi:10.1126/sciadv.aaw0341
Tran J., Rice A. J., Main L. C., Gastin P. B. (2015). Profiling the Training Practices and Performance of Elite Rowers. Int. J. Sports Physiol. Perform. 10, 572–580. doi:10.1123/ijspp.2014-0295
Treff G., Leppich R., Winkert K., Steinacker J. M., Mayer B., Sperlich B. (2021a). The Integration of Training and Off-Training Activities Substantially Alters Training Volume and Load Analysis in Elite Rowers. Sci. Rep. 11, 17218. doi:10.1038/s41598-021-96569-0
Treff G., Schmidt W., Wachsmuth N., Völzke C., Steinacker J. (2014). Total Haemoglobin Mass, Maximal and Submaximal Power in Elite Rowers. Int. J. Sports Med. 35, 571–574. doi:10.1055/s-0033-1358476
Treff G., Winkert K., Machus K., Steinacker J. M. (2018). Computer-Aided Stroke-By-Stroke Visualization of Actual and Target Power Allows for Continuously Increasing Ramp Tests on Wind-Braked Rowing Ergometers. Int. J. Sports Physiol. Perform. 13, 729–734. doi:10.1123/ijspp.2016-0716
Treff G., Winkert K., Sareban M., Steinacker J. M., Becker M., Sperlich B. (2017). Eleven-Week Preparation Involving Polarized Intensity Distribution Is Not Superior to Pyramidal Distribution in National Elite Rowers. Front. Physiol. 8, 515. doi:10.3389/fphys.2017.00515
Treff G., Winkert K., Steinacker J. (2021b). Olympic Rowing - Maximum Capacity over 2000 Meters. Dtsch Z. Sportmed. 72, 203–211. doi:10.5960/dzsm.2021.485
Turner K. J., Rice A. J. (2021). Physiological Responses on the Concept II BikeErg and Concept II RowErg in Well-Trained Male Rowers. Int. J. Sports Sci. Coaching 16, 741–748. doi:10.1177/1747954120968183
Valentin J. e. (2002). Basic Anatomical and Physiological Data for Use in Radiological protection: Reference Values. A Report of Age- and Gender-Related Differences in the Anatomical and Physiological Characteristics of Reference Individuals. ICRP Publication 89. Ann. ICRP 32, 5–265. doi:10.1016/s0146-6453(03)00002-2
van Zijl P. C. M., Jones C. K., Ren J., Malloy C. R., Sherry A. D. (2007). MRI Detection of Glycogen In Vivo by Using Chemical Exchange Saturation Transfer Imaging (glycoCEST). Proc. Natl. Acad. Sci. U.S.A. 104, 4359–4364. doi:10.1073/pnas.0700281104
Vogler A. J., Rice A. J., Gore C. J. (2010). Physiological Responses to Ergometer and On-Water Incremental Rowing Tests. Int. J. Sports Physiol. Perform. 5, 342–358. doi:10.1123/ijspp.5.3.342
Volianitis S., Secher N. H. (2009). Rowing, the Ultimate challenge to the Human Body - Implications for Physiological Variables. Clin. Physiol. Funct. Imaging 29, 241–244. doi:10.1111/j.1475-097X.2009.00867.x
Keywords: elite sport, indirect calorimetry, exercise energy expenditure, rowers, nutrition
Citation: Winkert K, Steinacker JM, Koehler K and Treff G (2022) High Energetic Demand of Elite Rowing – Implications for Training and Nutrition. Front. Physiol. 13:829757. doi: 10.3389/fphys.2022.829757
Received: 06 December 2021; Accepted: 02 March 2022;
Published: 19 April 2022.
Edited by:
Stefanos Volianitis, Qatar University, QatarReviewed by:
Basilio Pueo, University of Alicante, SpainLaurent André Messonnier, Université Savoie Mont Blanc, France
Léo Blervaque, Savoie Mont-Blanc University, France
Copyright © 2022 Winkert, Steinacker, Koehler and Treff. This is an open-access article distributed under the terms of the Creative Commons Attribution License (CC BY). The use, distribution or reproduction in other forums is permitted, provided the original author(s) and the copyright owner(s) are credited and that the original publication in this journal is cited, in accordance with accepted academic practice. No use, distribution or reproduction is permitted which does not comply with these terms.
*Correspondence: Kay Winkert, a2F5LndpbmtlcnRAdW5pLXVsbS5kZQ==