- 1Aging Institute of UPMC, The University of Pittsburgh School of Medicine, Pittsburgh, PA, United States
- 2Division of Geriatric Medicine, Department of Medicine, University of Pittsburgh School of Medicine, Pittsburgh, PA, United States
- 3Geriatric Research, Education and Clinical Center, Veterans Affairs Pittsburgh Healthcare System, Pittsburgh, PA, United States
Lipids are key macromolecules that perform a multitude of biological functions ranging from maintaining structural integrity of membranes, energy storage, to signaling molecules. Unsurprisingly, variations in lipid composition and its levels can influence the functional and physiological state of the cell and its milieu. Cellular senescence is a permanent state of cell cycle arrest and is a hallmark of the aging process, as well as several age-related pathologies. Senescent cells are often characterized by alterations in morphology, metabolism, chromatin remodeling and exhibit a complex pro-inflammatory secretome (SASP). Recent studies have shown that the regulation of specific lipid species play a critical role in senescence. Indeed, some lipid species even contribute to the low-grade inflammation associated with SASP. Many protein regulators of senescence have been well characterized and are associated with lipid metabolism. However, the link between critical regulators of cellular senescence and senescence-associated lipid changes is yet to be elucidated. Here we systematically review the current knowledge on lipid metabolism and dynamics of cellular lipid content during senescence. We focus on the roles of major players of senescence in regulating lipid metabolism. Finally, we explore the future prospects of lipid research in senescence and its potential to be targeted as senotherapeutics.
Introduction
Senescence is a cell-fate decision that is triggered in response to many different stressors including genotoxic stress, telomere attrition/damage, oncogene activation, and mitochondrial dysfunction (Herranz and Gil, 2018). In contrast to quiescence (cell cycle arrest), cellular senescence is a terminal state of growth arrest, where cells cannot re-enter the cell cycle despite mitogenic growth signals (Pack et al., 2019). Senescent cells resist apoptosis, at least partially due to an upregulation of survival pathways such as the BCL-2 family (Zhu et al., 2015; Yosef et al., 2016). Cellular senescence has been documented to have both beneficial and detrimental roles in maintaining health (He and Sharpless, 2017), and has been linked to embryonic development, wound healing, tumor suppression, as well as metastasis and aging. “Acute” senescence is a programmed cell-fate decision and is usually associated with tissue repair and homeostasis (Krizhanovsky et al., 2008; Jun and Lau, 2010; Rajagopalan and Long, 2012). In contrast, “chronic” senescence is associated with stochastic, persistent macromolecular damage and often associated with disease and aging (van Deursen, 2014). The permanent cell-cycle arrest of senescent cells is accompanied by a senescence associated secretory program (SASP). The SASP consists of pro-inflammatory factors such as cytokines, chemokines and extracellular matrix remodeling factors.
Identification of Senescent Cells
The field is challenged by the lack of a single universal marker for cellular senescence. Currently, senescent cells are identified based on multiple markers (Figure 1), that allow for distinction between quiescent and senescent cells. The most prominent features of senescent cells include, an enlarged, flat cell morphology with an increase in the cytoplasm-to-nucleus ratio. A recent study suggested that the ratio of DNA to cytoplasm is critical to maintain cell function, and dysregulation of this ratio possibly contributes toward aging (Neurohr et al., 2019). Senescent cells also display an increase in the lysosomal enzyme, senescence-associated-β-galactosidase (SA-β-gal), and thus display increased SA-β-gal activity (Dimri et al., 1995; Hall et al., 2017). Single cell analysis, identified that cells with high SA-β-gal activity also exhibit larger cell size (Biran et al., 2017). It is important to note that SA-β-gal is often associated with senescent cells, but can be observed upon serum starvation or upon confluency in cell culture. Another feature of senescent cells is the accumulation of pigment granules in the lysosomes known as lipofuscin (Evangelou et al., 2017). However, lipofuscin accumulation is observed with age in many cells and organisms, including those that do not typically have other features of cellular senescence, e.g., C. elegans (Pincus et al., 2016). Lack of DNA replication and arrest of the cell cycle usually in G0/G1 phase are features of senescent cells, but do not distinguish between quiescence and senescence.
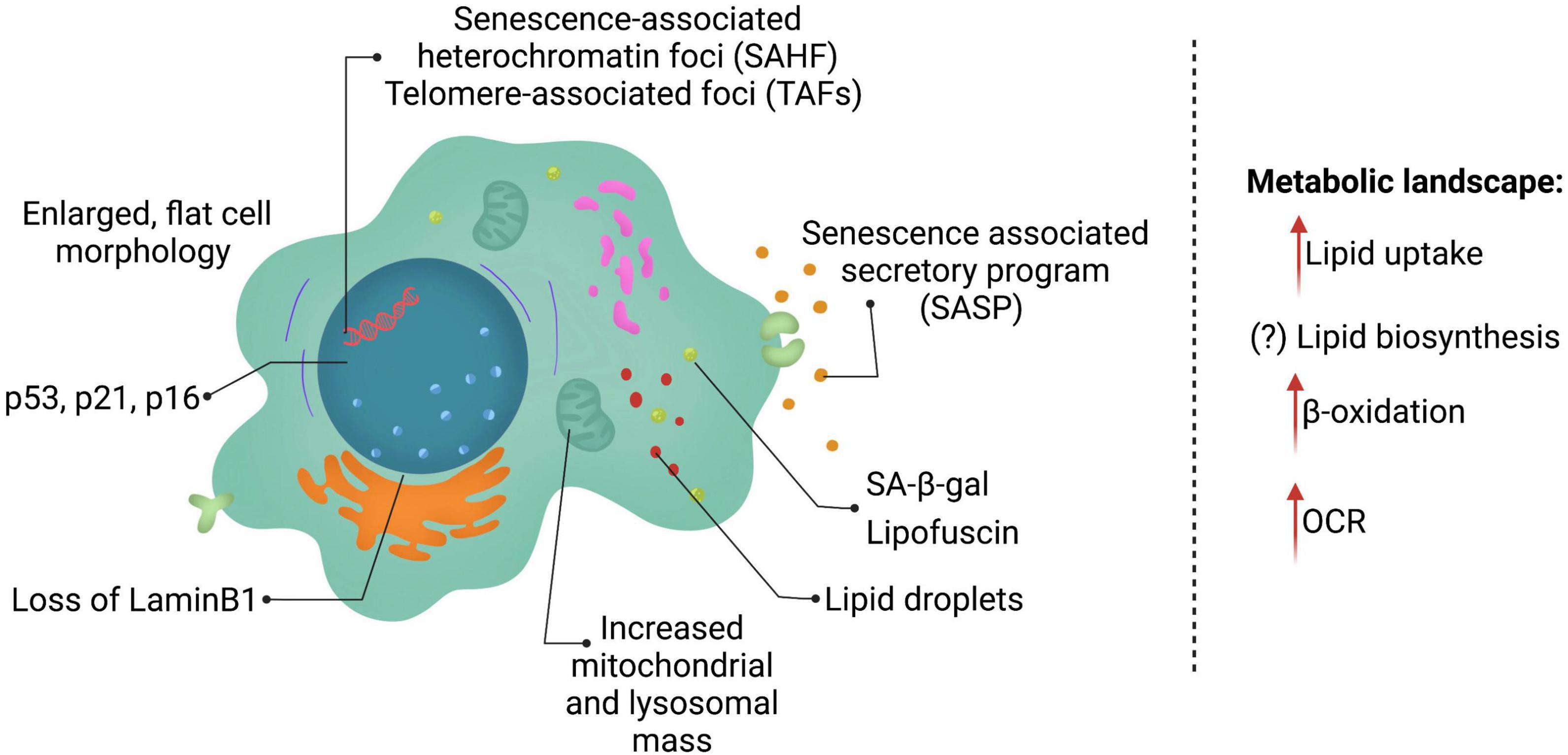
Figure 1. Features of senescent cells. Senescent cells are in a state of permanent cell cycle arrest and display several key features including lysosomal changes (SA-β-gal and lipofuscin), p53, p21, and/or p16 expression and a SASP program consisting of multiple cytokines, chemokines, and ECM remodeling enzymes. Senescent cells reprogram their metabolism as well heavily altering lipid metabolism.
The two key signaling pathways upregulated for establishment and maintenance of senescence are p53/p21 and p16 (discussed in detail below). The burden of p21- and p16- positive expressing cells increases with age in mammals, however, currently the understanding of what percentage of cells are senescent is incomplete. A recent cross-sectional study in humans, examining three different age groups and multiple tissues, identified that p21- and p16-positive cells increase with age in the skin, pancreas and kidney (Idda et al., 2020). In contrast, p16-positive cells are observed in brain cortex, liver, spleen, and intestine (colon), whereas p21-positive cells increase in the dermis with age. Interestingly, some tissues, such as lung and muscle did not show any changes in these markers. Although a comprehensive study, it is important to note that this study was cross-sectional in design, as well as underlying health conditions may not have been fully examined. Nonetheless, a conclusion to be drawn from this study is that different cells and tissues accumulate different markers of senescence and may display varying burden of senescent cells.
Dynamic chromatin changes are a hallmark of senescent cells. Senescence-associated heterochromatin foci (SAHF) and telomere-associated foci (TAFs) are commonly used as markers for cellular senescence. However, these heterochromatin changes have been associated with senescence caused by nuclear DNA damage or telomere dysfunction, rather than other senescence-inducing stressors (Di Micco et al., 2011). A common chromatin feature in senescent cells is the generation of chromatin fragments that are released into the cytoplasm. The loss of nuclear lamina (Lamin B1) is observed in senescent cells, leading to “leaky” nucleus, and is thought to promote chromatin fragments (CCFs) in the cytoplasm (Di Micco et al., 2011; Dou et al., 2015, 2017). The CCFs activate the cyclic GMP–AMP synthase (cGAS) and the stimulator of interferon genes (STING) pathway (cGAS-STING pathway) and further establish SASP factors (Vizioli et al., 2020), thus reinforcing senescence and affecting neighboring cells.
Last but not least, an integral part of senescent cells is their ability to drive the SASP phenotype. SASP consist of pro-inflammatory cytokines [interleukin (IL)-1α, IL-1β, IL-6, and IL-8], chemokines (CCL2), and extracellular matrix remodeling factors such as matrix metalloproteinases (MMPs), serine/cysteine proteinase inhibitors (SERPINs) such as plasminogen activator inhibitor-1 (PAI-1) and tissue inhibitors of metalloproteinases (TIMPs) (Coppe et al., 2010; Eren et al., 2014). With advances in technology, recent studies have uncovered that different senescence-inducing stressors, as well as different cell types may lead to unique SASP being expressed (Basisty et al., 2020). SASP is thought to be transcriptionally controlled and released into the cytoplasm, as soluble factors or in exosomes (Lehmann et al., 2008; Ozcan et al., 2016; Takahashi et al., 2017), to influence neighboring cells (Ito et al., 2017). Understanding of SASP regulation is incomplete, although, p53, NF-κB, P38 MAPK, NOTCH, mTOR, and mitochondrial NAD+/NADH ratio have recently emerged as modulators of the SASP phenotype (Freund et al., 2011; Herranz et al., 2015; Nacarelli et al., 2019).
In this Review, we first describe the rewiring of lipid metabolism during cellular senescence. This review mostly focuses on cellular senescence observed with aging. We then review the link between major regulators of senescence and their role in lipid metabolism. Lastly, we discuss lipid classes that are altered in senescent cells and how they contribute to the senescence phenotype and outline tools that can help examine the causal role of lipids in driving cellular senescence.
Metabolic Landscape of Senescent Cells
Aging is associated with dysregulated lipid metabolism (Mutlu et al., 2021). Several studies show that lipid composition changes with age in several species, including humans. Particularly, increased ratio of poly- and mono- unsaturated fatty acids have been recently found to be increased with age (Papsdorf and Brunet, 2019). However, whether cellular senescence, a key feature of aging is responsible for these lipid changes has been enigmatic so far. Lipids are an important fuel source that generate energy, as well as create crucial biological intermediates including lipid mediators, second messengers, and ketone bodies. Lipids can act as central metabolites that play a role in cellular signaling, both in an autocrine and paracrine manner, provide cell and organelle structure, and are fundamental for maintaining cellular homeostasis. Recent studies highlight the role of lipids in establishing cell fate decisions, such as cell division, apoptosis and cellular senescence (Ito and Ito, 2016). As described above, hallmarks of cellular senescence consists of increased mass of organelles such as, lysosomes and mitochondria, increased cellular size, loss of nuclear membrane integrity and SASP factors that can be “transported” by exosomes (Lizardo et al., 2017). Lipids are essential to each of these “senescence features,” including exosomes that are typically lipid enriched extracellular vesicles (Skotland et al., 2019) and therefore lipids are inextricably linked to the process of cellular senescence.
Several studies report an accumulation of lipid droplets in senescent cells compared to proliferating cells (Ogrodnik et al., 2014, 2019; Cox and Redman, 2017; Flor et al., 2017; Lizardo et al., 2017; Chee et al., 2021). Deregulated lipid accumulation in senescent cells can be due to increase in lipid uptake, upregulated lipid biosynthesis pathways or de-regulated lipid breakdown. Interestingly, senescent cells display increased lipid uptake (Flor et al., 2017), however, whether inhibition of lipid uptake can prevent or delays cellular senescence is unclear. Lipid levels are also controlled through a complex mechanism of lipid biosynthesis and breakdown. Advances in technology such as non-targeted “omic” approaches have highlighted the importance of lipid metabolism in cellular senescence. For example, a recent proteomic study of senescent cells, induced by DNA damaging agents, uncovered that lipid processing and metabolism genes including those involved in lipid binding, storage, biosynthesis and breakdown are severely altered (Flor et al., 2017). Fatty acids (FA) are essential building blocks for triglycerides (TGs) and phospholipids, and act as an energy source. Fatty acids are synthesized by the addition of malonyl-CoA to an acyl chain by one of two enzymes: acetyl-CoA carboxylase (ACCase) and fatty acid synthase (FAS). Although understudied, the role of FA biosynthesis in cellular senescence is possibly regulated in a context- and stress-dependent manner. Enlargement of cells and accumulation of membranous organelles such as, lysosomes and mitochondria are key hallmarks of senescence, and rely on lipid biosynthesis (Kim et al., 2010; Correia-Melo et al., 2016). Consistent with this theory, mouse hepatic stellate cells and human primary fibroblasts showed an increase in lipid biosynthesis, dependent on FASN, during initial establishment of cellular senescence (Fafian-Labora et al., 2019). FASN is upregulated prior to induction of late-stage senescence, and is increased with age in mouse liver. Interestingly, pharmacological or genetic inhibition of FASN, reduced p53-induced senescence, as well as, SASP (Borghesan et al., 2019; Fafian-Labora et al., 2019). Whether FASN is required for maintenance of cellular senescence and upregulated in a p53-independent manner is currently unknown. Oncogene-induced senescence (OIS) is a robust mechanism activated by oncogenic signaling due to an activating mutation of an oncogene or an inactivation of a tumor suppressor. Targeted metabolomic and bioenergetic analysis revealed that different modalities of senescence such as OIS and replicative senescence, led to very different metabolite patterns. In this study, Ras-induced OIS elevated levels of long chain free fatty acids (LCFA). Concomitantly, OIS reduced lipid biosynthesis by negatively regulating ACC, a rate limiting step for lipid biosynthesis (Quijano et al., 2012). It is important to note that these contradictory results about the role of lipid biosynthesis in senescence may be due to analyzing a different “stage” of cellular senescence, or different stress modality of senescence (aging versus OIS) or because of differences in cell-type (primary cells versus cancer cells).
Although the role of lipid biosynthesis in cellular senescence may not be straight-forward, FA breakdown has been consistently observed during senescence. A few reports indicate that lipid breakdown particularly through mitochondrial β-oxidation plays a paramount role in cellular senescence. Increased mitochondrial function has long been associated with senescence. Fatty acid oxidation (β-oxidation) is the mitochondrial multi-step process of breaking down a fatty acid into acetyl-CoA units. Long chain fatty acids (LCFA) are activated by acyl-carnitines and imported into the mitochondria for β-oxidation via a rate limiting enzyme carnitine palmitoyltransferase (CPT). Upon completion of β-oxidation, the generated acetyl-CoA can enter the TCA cycle, link to oxidative phosphorylation (OXPHOS) or can lead to acetylation of histones and other proteins. Indeed, recent data suggests that lipids contribute heavily toward histone acetylation and control gene transcriptional programs (McDonnell et al., 2016). During replicative senescence in myoblasts, several acyl-carnitines were increased, suggesting that senescent cells rely heavily on β-oxidation (Baraibar et al., 2016). Additionally, these senescent myoblasts had increased levels of monoacyl glycerols and glycerol-3-phosphate, as well as decreased mono- and polyunsaturated long chain fatty acids (>18 carbons), whereas medium chain fatty acids remained unaffected. This suggests that senescent cells increase lipid breakdown, however, whether this lipid mobilization and breakdown is used for energy production, gene transcription or generation of SASP (bioactive lipids) is unclear. Interestingly, an increase in FASN during establishment of the senescence program is also associated with an increase in CPT function and oxygen consumption rate (OCR). Consistent with this, inhibition of CPT1A during OIS, prevented cellular senescence and SASP establishment (Quijano et al., 2012). Collectively, these studies suggest that β-oxidation is required to drive cellular senescence (Fafian-Labora et al., 2019). It is important to note that one study reported that inhibition of β-oxidation could possibly promote senescence (Baraibar et al., 2016), Here, loss of CPT1C in lung fibroblasts, promoted lipid accumulation in cells and induced cellular senescence through lipotoxicity. One possibility is that lung fibroblasts uniquely depend on lipid β-oxidation, and inhibition of this process drives lipotoxicity and cellular senescence (Chen et al., 2021). Overall, these studies point toward major rewiring of lipid metabolism during establishment and maintenance of cellular senescence.
Lipid Compositional Changes During Cellular Senescence
Although several studies show accumulation of lipid droplets (LDs) during cellular senescence, the content of these LDs may be far more critical. Lipids are divided into three groups, triglycerides (TAGs) (utilized for energy), steroids (cholesterol and hormones) and phospholipids (utilized for membrane structures). Here, we summarize the associated changes in composition of these lipids during senescence.
Triglycerides
A study to decipher the transcriptomic and lipidomic changes during replicative senescence showed that lipid uptake mediated by CD36 is upregulated in senescent cells (Saitou et al., 2018). This feature is also consistent with DNA damage, oncogenic and chemical stress-induced senescence (Lizardo et al., 2017; Chong et al., 2018). Moreover, there is an enrichment of TAGs containing long- and very long-chain fatty acids, with at least one polyunsaturated fatty acyl (PUFA) chain (Saitou et al., 2018). Another study reported that DNA damage-induced senescence is accompanied with the upregulation of the triglyceride synthesis enzyme, diacylglycerol acyltransferase 1 (DGAT1). This study suggests that rather than lipid uptake, TAG biosynthesis may play a crucial role in establishment of senescence (Flor et al., 2017). The caveat though is that in this study the TAG species were not directly measured. It is therefore possible that both uptake and biosynthesis of TAGs may play a role in promoting cellular senescence.
Cholesterol
Cholesterol homeostasis is critical to health. It is an important building block for hormones, vitamin D and integral to cell membrane integrity; however, excess cholesterol can have detrimental effects. Few studies have examined the role of cholesterol on cellular senescence. Naked mole rats (NMR) are known for their exceptional longevity, resilience to endogenous and exogenous stressors and cancer resistance. Interestingly, NMR fibroblasts had elevated levels of cholesterol-enriched lipid droplets (LDs), dependent on the Wnt/β-catenin pathway (Chee et al., 2021). Inhibition of cholesterol synthesis using lovastatin, an HMG-CoA reductase inhibitor, promoted senescence-like phenotype in NMR fibroblasts. Of note, only SA β-gal and 8-hydroxy-2′-deoxyguanosine (8-OHdG), a biomarker for DNA damaged by oxidative stress was measured in this study. Furthermore, the Wnt/β-catenin-cholesterol axis seems specific to NMR, as overexpression of β-catenin in mice or rat fibroblasts did not show any elevation in LDs (Chee et al., 2021). On the contrary, lipid profiling of OIS induced by ERBB2 (a member of the epidermal growth factor receptor), revealed increased cholesterol levels accumulated in vacuoles of senescent cells (Cadenas et al., 2012). This study suggests that high cholesterol levels are possibly a feature of senescence, although whether this accumulated cholesterol is a driver of senescence is not fully understood. In contrast, when mouse bone marrow stem cells (BMSCs) were exposed to low dose of cholesterol, it delayed oxidative stress-induced cellular senescence. Indeed, low levels of cholesterol promoted proliferation of BMSCs. Oxidative stress is known to damage macromolecular structures including cellular membranes, and since cholesterol is a major component of cell membranes, exogenous supplementation of cholesterol may help recovery from acute damage (Zhang et al., 2016). Further studies to examine the impact of cholesterol dosage on senescence in vivo is required to ascertain its exact role in the senescence phenotype.
Phospholipids
Phospholipids are amphiphilic lipids that play important metabolic and structural roles. In a study looking at ERBB2 induced senescence (OIS), phospholipid with shortened acyl chains and unsaturated acyl chains in phosphatidylglycerol was observed (Cadenas et al., 2012), accompanied by increased membrane fluidity. This study also identified that mitochondrial lipids were altered, specifically, PG(34:1), PG(36:1) (increased) and LPE(18:1), PG(40:7) and PI(36:1) were reduced in senescent cells leading to loss of membrane potential. A comprehensive study compared and analyzed metabolites in replicative senescence, DNA damage-induced senescence and OIS in a human embryonic lung fibroblast (WI-38), using NMR spectroscopy. Interestingly, senescent cells displayed increased glycerophosphorylcholine-to-phosphocholine ratio. Importantly, glycerophosphorylcholine levels were particularly elevated in senescent cells compared to proliferating or quiescent cells, suggesting that this could be a unique feature of senescence (James et al., 2015). Bioactive phospholipids play an important role in “communication” and consist of lysophospholipids, ceramides, and sphingomyelin (Horn and Jaiswal, 2019). They can be released as secreted molecules or part of exosomes, and possibly play a role in extravesicular formation and release observed during senescence (Tepper et al., 2000; Nurminen et al., 2002; Trajkovic et al., 2008; Bianco et al., 2009; Hirsova et al., 2016). Hydrolysis of phospholipids by phospholipases, such as cPLA2, leads to lysophospholipids. An increase in lysophospholipids has been observed in senescent cells (Buratta et al., 2017; Narzt et al., 2021), and furthermore, lysophospholipids are shown to induce senescence in cholangiocytes (Shimizu et al., 2015). They can also act as a “find me” signal, but impair phagocytosis, possibly leading to inefficient clearance of senescent cells with age (Narzt et al., 2021). Phospholipids also influence the synthesis of eicosanoids that are known inflammatory mediators, thus contributing to the SASP phenotype (discussed in detail below). Thus, phospholipids play a varied role during senescence, including establishment of senescence, paracrine signaling and evasion of senescent cells.
Major Lipid Players in Senescence
Oxidized Fatty Acids
One of the major components of complex lipids in mammalian cells are polyunsaturated fatty acids (PUFAs) with atleast one bis allelic carbon and chain lengths ranging from 18 and above. PUFAs are precursors for potent metabolites that are involved in numerous pathophysiological events. One of the most well-studied families are eicosanoids, a class of oxygenated derivatives of arachidonic acid (C20) that include prostaglandins, leukotriens, lipoxins, hydroxy eicosatetraenoic acids, hydroperoxy eicosatetraenoic acids, and epoxy eicosatetraenoic acids. These bioactive lipids are derived enzymatically through the cyclooxygenase (COX), lipoxygenase (LOX), and cytochrome P450 (CYP) reactions or non-enzymatically through free radical reactions. Together, these bioactive lipids regulate diverse sets of both homeostatic and inflammatory processes. Pharmacological inhibition of the cysteinyl leukotrienes via LT antagonists, LY255283 and montelukast sodium, or ALOX5 inhibitor, BW-B70C, reduced the profibrotic effect of senescent cells on naive fibroblasts in vitro (Wiley et al., 2019) and attenuated TNFα-induced up-regulation of SA-β-Gal, p53, p21, and PAI-1 expression in primary human chondrocytes associated with osteoarthritis (Song et al., 2018). Increase in COX-2 activity has been shown to be associated with aging (Badawi et al., 2005; Chung et al., 1999; Baek et al., 2001). For example, COX-2 expression was upregulated in kidney, liver, heart and prostrate in old rodents (Hayek et al., 1997; Kim et al., 2001; Badawi et al., 2004; Choi et al., 2014; Tung et al., 2015), and in aging human tissues as well, especially kidney and skin (Melk et al., 2004; Kang et al., 2006; Surowiak et al., 2014). Although the cause- or-causal relationship is not clear, it was shown that in an inducible Cox-2 transgenic mice, post-natal expression of COX-2 led to several premature aging phenotypes (Kim et al., 2016). Further, the lung fibroblasts from these animals expressed higher levels of senescence-associated SA-β-Gal, p16, p53, and phospho-H2Ax (Kim et al., 2016).
The age-related changes in COX-2 are often accompanied by an elevation in the prostaglandin synthesis. COX system is the major pathway catalyzing the conversion of arachidonic acid into prostaglandins, PGG2 and subsequently to PGH2 followed by the production of the bioactive lipids—PGE2, PGI2, PGD2, PGF2α—and thromboxane A2 by tissue-specific synthases. An upregulation in COX-2 expression and ensuing PGE2 production was observed during normal and stress-induced senescence of dermal, prostrate and lung human fibroblasts. Exogenous supplementation of arachidonic acid enhanced COX-2 activity and PGE2 production and subsequently accelerated the incidence of key senescence features like flat and enlarged cell morphology, SA-β-Gal activity and the cell cycle arrest (Dagouassat et al., 2013). In a study to understand the mechanisms of lung senescence linked with chronic obstructive pulmonary disease (COPD), it was shown that prostaglandin E2 released by pulmonary fibroblasts exerted both autocrine and paracrine effects to induce fibroblast senescence. This was accompanied with inflammation by EP2 and EP4 receptors, COX-2–dependent reactive oxygen species signaling (Dagouassat et al., 2013). Wiley et al. recently showed that senescent cells produced and accumulated 1a,1b-dihomo-15-deoxy-delta-12,14-prostaglandin J2, which together with other prostaglandin D2-related lipids promoted senescence arrest and SASP through activation of RAS signaling. In mice with drug-induced senescence, a dose of Navitoclax, a senolytic drug (eliminates senescent cells) elevated the levels of prostaglandin in blood and in urine. This study highlights the importance of prostaglandin lipids as biomarkers of senolysis.
Another primary oxidation product of arachidonic acid is hydroperoxy-eicosatetraenoic acid (hydroperoxyeicosatetraenoic acid, HpETE), generated via lipoxygenase (LOX) activity. Due to its regiospecificity nature, lipoxygenases are classified as arachidonate 5-, 8, 12-, and 15-lipoxygenase (5-LOX, 8-LOX, 12-LOX, and 15-LOX), which inserts oxygen at carbon 5, 8, 12, or 15 of arachidonic acid peroxidases. The primary oxidation products of HpETE are unstable and reduced further to its corresponding alcohol hydroxy-eicosatetraenoic acid (5-, 8-, 12-, and 15- hydroxy-6-trans-8,11,14-cis-eicosatetranoic acid, HETE) by glutathione peroxidase. The enzyme 5-LOX can act on 5-HETE to generate an unstable epoxide leukotriene A4 (LTA4), which can serve either as an intracellular intermediate in the synthesis of leukotriene B4/C4 (LTB4 and LTC4). Leukoterines (LTs) are the second major class of biologically active signaling lipids that plays a key role in inflammation and fibrosis. Idiopathic pulmonary fibrosis (IPF) is an interstitial lung disease with elevated senescent cell burden. It was shown that leukoterines secreted by senescent cells exacerbated IPF by promoting pulmonary fibrosis (Wiley et al., 2019) and kidney fibrosis by unilateral ureteral obstruction in mice (Kamata et al., 2019). Senescence induced irrespective of different modalities (oncogene-, stress-, and radiation-induced) in IMR-90 fibroblasts promoted secretion of leukoterines, as well as expression of enzymes involved in the biosynthesis of LTs (Wiley et al., 2019). Cysteinyl leukoterines such as LTC4 has been shown to accelerate acute kidney injury and drive renal pathogenesis in response to chemotherapy-induced senescence (Rubinstein and Dvash, 2018; Wiley, 2020). In line with these findings, leukotrienes increasingly released from senescent cells, irrespective of the senescence-inducer, and appear to function in parallel to SASP factors.
Besides lipoxygenases and cyclooxygenases, the third class of enzymes involved in eicosanoid synthesis are epoxygenases or cytochrome P450 (CYP) enzymes. Cytochrome P450 epoxygenases (CYP2C and CYP2J) synthesize epoxides, such as epoxyeicosatrienoic acid (EETs) in four different regioisomers (5, 6-, 8, 9-, 11, 12-, and 14,15-) that are recognized as specialized pro-resolving mediators (Alkayed et al., 1996; Zhang et al., 2007; Li et al., 2012). EETs have a wide range of protective actions such as anti-inflammatory, anti-apoptotic, vasodilatory and pro-angiogenic (Node et al., 1999; Iliff et al., 2009). The levels of EETs are often determined through production of dihydroxyeicosatrienoic acid (DHET) isoforms via soluble epoxide hydrolase (sEH) (Morisseau and Hammock, 2013). Gene deletion of soluble epoxide hydrolase was shown to improve blood flow and reduce infarct size following cerebral ischemia in reproductively senescent female mice (Zuloaga et al., 2014). 14,15-EET inhibited rat mesenteric arterial endothelial senescence, down-regulated p53 expression in aged endothelial cells and improved the impaired endothelium-dependent vasodilatation in aged rats through activation of mTORC2/Akt signaling pathway (Yang et al., 2014). Injection of 11,12-EET accelerated wound healing, tissue repair and reduced production of proinflammatory factors TNFα, IL-6, MMP and IL-1β in type 2 diabetic models of B6.VLepob/J (leptin-deficient, ob/ob) mice. In another study, both forms of EETs 11,12 and 14,15 epoxyeicosatrienoic acid rescued deteriorated wound healing in ischemic conditions (Sommer et al., 2019). Orally active subset of 14,15-EET analogs, termed EET-A, EET-B, and EET-C22 reduced cardiac and renal injury in spontaneous and angiotensin hypertension as well as promoted recovery after Ischemia/Reperfusion Injury by reducing contractile dysfunction (Campbell et al., 2017). Although several studies have highlighted the deleterious impact of cellular senescence in numerous chronic diseases, it is also recognized for its beneficial role in tumor suppression, wound healing and tissue regeneration. These studies highlight the contribution of CYP generated pro-resolvin lipid mediators in wound healing.
Oxidized Phospholipids
Polyunsaturated fatty acyl containing phospholipids are prone to lipid peroxidation and form oxidized phospholipids (OxPLs) that play a significant role in apoptosis and inflammation. OxPLs are generated enzymatically through lipoxygenases or non-enzymatic attack through reactive oxygen species. However, irrespective of the mode, identical primary oxidation products (i.e., peroxyl radicals and hydroperoxides) are produced initially that subsequently undergo further oxidation in a stochastic manner and release a heterogenous mixture of bioactive OxPL species. In general, lipid peroxidation and levels of OxPLs increase with age. OxPLs generated via cytoplasmic hydroxyl radicals exacerbated cellular senescence in Toll-like receptor 4-knockout (TLR4-/-) OKD48- and IDOL-Tg mouse models (Sakai et al., 2019). Inhibition of TLR2 with oxid ized-1-palmitoyl-2-arachidonyl-sn-glycero-3-phosphorylcholine (OxPAPC), a well-studied oxidized product of a common phospholipid in mammalian cell membranes, 1-palmitoyl-2-arachidonoyl-sn-phosphatidylcholine (PAPC) significantly reduced IL-1α and IL-1β mRNA induction in oncogene induced senescence of IMR90 cells. In another study targeting OxPLs by transgenic overexpressing of E06-svFv, a single chain variable fragment that binds to phosphatidyl choline head group of OxPL attenuated age-associated trabecular bone loss in both female and male mice (Palmieri et al., 2021). Similar E06-svFv transgene mechanisms to inactivate OxPLs have been studied with age-associated diseases such as atheroscleosis, steatohepatitis (Sun et al., 2020) and macular degeneration (Handa et al., 2015) suggesting neutralizing OxPLs as a promising therapeutic target for some of the age-associated diseases. Cardiolipins, major phospholipids in mitochondria are extremely prone to lipid oxidation. With mitochondria being the critical organelle handling oxidative stress, oxidation of cardiolipin can lead to organelle dysfunction and generate lipid peroxides further exacerbating oxidative stress and promoting senescence. Knock out of ALCAT-1, an enzyme that promotes cardiolipin peroxidation protected against onset of cellular senescence and preserved mitochondrial function by reducing sensitivity to lipid oxidation.
Greenberg et al. (2008) proposed the “lipid whisker” hypothesis as a novel feature of membrane architecture in senescent or apoptotic cells. These cells are distinguished by the presence of protruding “whiskers” as a result of phospholipid oxidation via lipid peroxidation or oxidative stress. The oxPL whiskers such as oxidized phosphatidylserine contribute to pattern recognition for common receptors like CD36 that play a major role in engulfment and phagocytosis of senescent cells (Podrez et al., 2002; Greenberg et al., 2006, 2008).
Sphingolipids
Sphingolipids are a class of complex phospholipids with a hydrophobic core consisting of an amino alcohol, sphingosine and a long-chain fatty acid chain. Bioactive forms this class such as sphingosine, sphingosine-1-phosphate, ceramide, ceramide-1-phosphate, dihydroceramide are known to modulate a plethora of cellular functions such as cell migration, growth regulation, adhesion, apoptosis, senescence and inflammatory responses (Hannun and Obeid, 2018).
Several studies have highlighted the role of sphingolipids in both development and aging (Giusto et al., 1992; Lightle et al., 2000; Cutler and Mattson, 2001; Hannun and Obeid, 2018). For example, aging induces accumulation of sphingosine and ceramides in liver and brain tissues and alterations in sphingolipid metabolism increases the risk and progression of age-related disease (Giusto et al., 1992; Lightle et al., 2000). A systemic study on sphingolipid metabolic enzymes in kidney, liver and brain tissues of day-1 to 720-day-old rats revealed a significant increase in activities of the sphingolipid catabolic enzymes (SMase and ceramidases) (Sacket et al., 2009). Impairment of ceramide and sphingolipid synthesis genes in yeast, worms and flies extended lifespan (Cutler and Mattson, 2001; Rao et al., 2007; Yi et al., 2016).
In senescent human dermal fibroblasts (HDF), ceramide levels and a sphingomyelinase activity were markedly elevated. Exogenous ceramide induced senescent phenotype in young HDF at concentrations that mimics endogenous ceramide levels in senescent cells and recapitulated molecular changes of senescence, suggesting ceramides act as mediators of senescence (Venable et al., 1995). Of note, in therapy-induced senescence, exogenous addition of sphingomyelin increased ceramide levels that induced apoptosis and decreased senescence. This is possibly explained by ceramide levels- no inhibition of cell cycle progression at low ceramide levels, induction of senescence at moderate levels, and initiation of apoptosis at high levels (Modrak et al., 2009). In another study, deficiencies in ceramide transfer protein (CERT) in primary mouse embryonic fibroblasts led to senescence and dysregulated sphingolipid metabolism by increasing hexosylceramides production (Rao et al., 2007). Increased hexoceramides such as glucosylceramide led to age-related impairments in CD4(+) T cell function and immunosenescence (Molano et al., 2012). Several studies have suggested that sphingolipid metabolism as effectors or modulators of the p53 tumor suppressor pathway, particularly sphingomyelinase and sphingosine kinase I (SK1), which are directly regulated by p53, contributing to ceramide-induced senescence in cells (Dbaibo et al., 1998; Oskouian et al., 2006; Heffernan-Stroud and Obeid, 2011). For example, the expression of sphingosine kinase I (SK1), a downstream target of p53, was significantly elevated in p53-knockout mice that developed thymic lymphoma, and p53-deficient mice lacking SK1 were protected from thymic lymphoma. Mechanistic studies revealed that p53 tumor suppression caused by loss of SK1 was mediated by increased sphingosine and ceramides, resulting in tumor senescence. Non-canonical sphingolipids such as deoxyceramides has been shown to be depleted during tumor-induced senescence and increasing the levels of 1-deoxyceramides reduced the senescence phenotype in human colorectal cancer cells. The imbalance in deoxyceramide levels can affect sphingolipid signaling and alter membrane remodeling (Millner et al., 2020). Despite the fact that several evidences point to the multiple roles of bioactive sphingolipids in aging, these intriguing findings warrant more research into the functional role of sphingolipids in senescence.
Lysophospholipids
Lysophospholipids are multifunctional lipid mediators and are formed from hydrolyzed phospholipids with either an alkyl or acyl chain (Rivera and Chun, 2008). The lysophospholipids (LPLs) are broadly categorized as lysoglycerophospholipids and lysosphingolipids. The common ones in circulation are lysophosphatidylcholine (LPC), lysophosphatidic acid (LPA), lysophosphatidylserine (LPS), sphingosine, sphingosine-1 phosphate (S1P), and sphingosylphosphorylcholine (SPC) that are derived from corresponding phospholipids. Among the LPLs, lysophosphatidylcholine is the most abundant class (Ojala et al., 2007) and activates multiple signaling pathways that are involved in oxidative stress and inflammatory responses (Kakisaka et al., 2012; Bansal et al., 2016). They are also major secretory components of extracellular vesicles, which has recently gained a lot of attention as key players within the secretome of senescent cells (Urbanelli et al., 2016; Buratta et al., 2017). For example, biochemical characterization of extracellular vesicles released by fibroblasts subjected to H-Ras induced senescence indicated a significant enrichment in LPCs species (16:0; 16:1; 18:1; 18:2; 20:4, and 26:0). Both, replicative- and stress- induced-senescent dermal fibroblasts showed elevated levels of lysophosphatidylcholines. The LPC exhibited SASP activity by eliciting chemokine release in non-senescent fibroblasts and obstructed TLR2–6/CD36 signaling in macrophages (Narzt et al., 2021). Cholangiocyte senescence in biliary tract cancer has been linked to an increase in LPC levels and the resulting cytotoxicity. LPCs induced upregulation of components of SASP interleukin-8 (IL-8), IL-6, transforming growth factor-β and PAI-1, as well as p21 and SA-β-gal activity in MMNK-1 human cholangiocyte cells.
Studies have also shown association between aging and lysophosphatidic acid (LPA) signaling. For instance, alterations in LPA1 receptor was linked to the presence of depression and cognitive deficits in the elderly population (Moreno-Fernandez et al., 2018). Age-dependent changes in cAMP profiles were induced by LPA as noted in young and senescent human fibroblasts (Jang et al., 2006). LPA were detected at significantly higher concentrations in the cerebral cortex synaptosomes of aged rats (Pasquare et al., 2009). Knockdown of LPA3 led to cell senescence in mesenchymal stromal cells (Kanehira et al., 2017). Another finding showed that LPA regulates ROS levels and cell senescence through LPA3 to alleviate cell aging in Hutchinson–Gilford progeria syndrome (Chen et al., 2020). Together these studies reveal a key role of lysophospholipid signaling and its wide spread effects in the process of aging and senescence. Figure 2 illustrates the key lipid players involved in senescence.
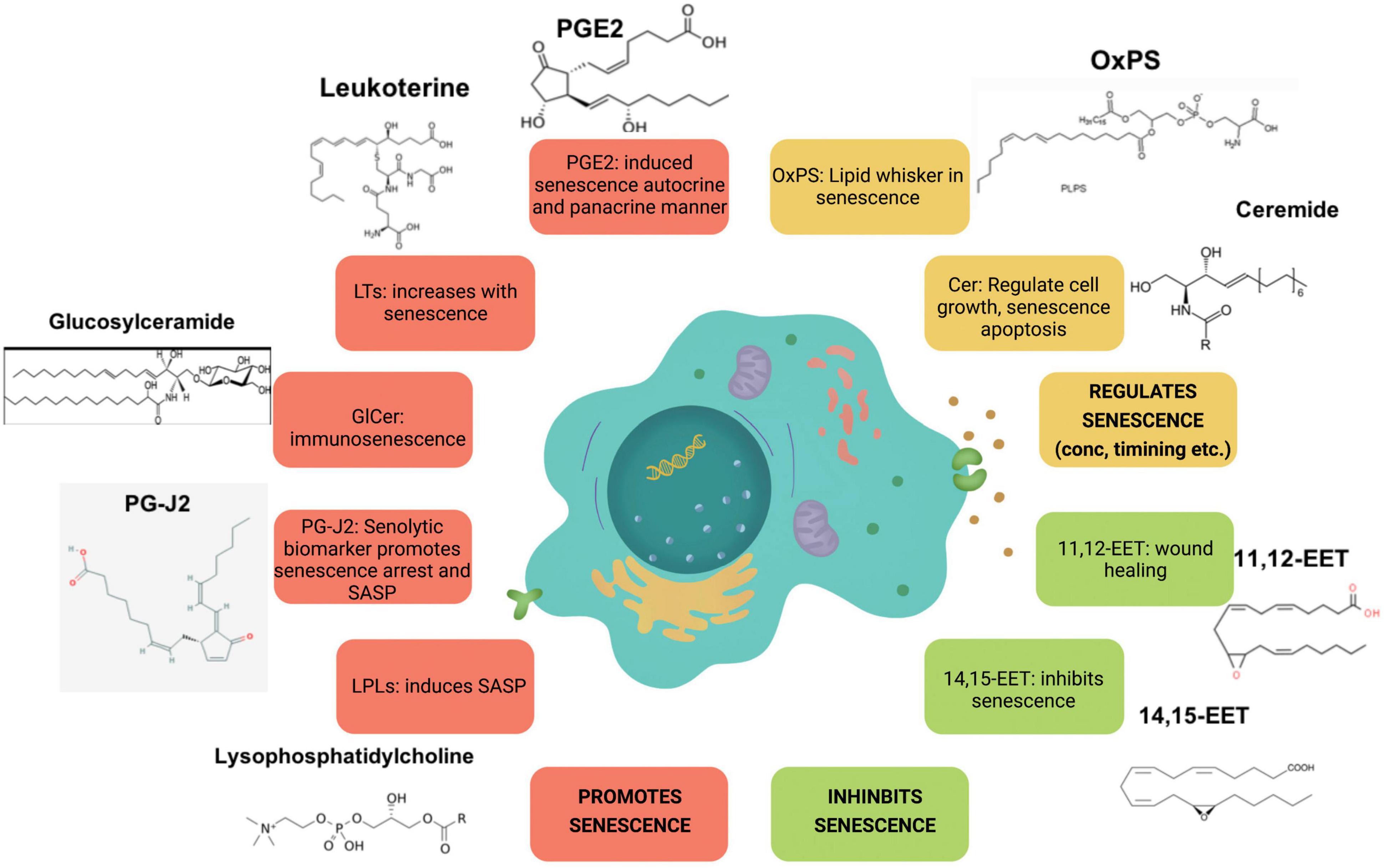
Figure 2. Major lipid species involved in senescence. Species illustrated in red increase with senescence, whereas species in orange and green regulate and inhibit it, respectively.
Major Molecular Players of Cellular Senescence and Their Role in Lipid Regulation
p16
Cellular senescence is initiated and maintained through two critical pathways: p16INK4A/Rb and/or p53/p21cip1. It is important to note that both, p16 and p53, are well known tumor suppressors. p16 inhibits cyclin dependent kinases, CDK4 and CDK6, thus maintaining Rb in a hypophosphorylated form and blocking cell cycle progression (Figure 3). Expression of p16 is a key factor for maintaining long-term senescence (Serrano et al., 1997; Yamakoshi et al., 2009). A recent study isolated high expressing p16-positive cells, specifically macrophages, and showed that these cells exhibit several other markers of senescence, including SA-β-gal, reduced proliferation and accompanying SASP (Liu et al., 2019). This suggests that high expression of p16 is indeed associated with cellular senescence. Additionally, several single nucleotide polymorphisms (SNPs) have been identified in the CDKN2a/b locus (encoding the p16INK4a, ARF, and p15INK4b transcripts) and associated with multiple age-related pathologies (Jeck et al., 2012; Fortney et al., 2015). Furthermore, p16 expression is largely undetectable in young mice, Krishnamurthy et al. (2004), Liu et al. (2009) but is strongly associated with aging in multiple mouse tissues (Burd et al., 2013). Similarly, other “drivers” that accelerate aging features, for example high fat diet, drives p16 expression through epigenetic modulation of its coding region (Zhang et al., 2018). Consistent with p16 being a major player in cellular senescence, genetic ablation of p16-positive cells, using the INK-ATTAC (Baker et al., 2011) and p16-3MR mice (Demaria et al., 2014), reduced senescent cell burden and improved healthspan, both in a progeria model, as well as naturally aged WT animals. Of note, preventing the accumulation of p19ARF-positive cells, another tumor suppressor that regulates p53 stability did not delay or prevent age-related pathologies (Baker et al., 2008), suggesting that p16 is crucial for maintenance of senescence, at least in mice models.
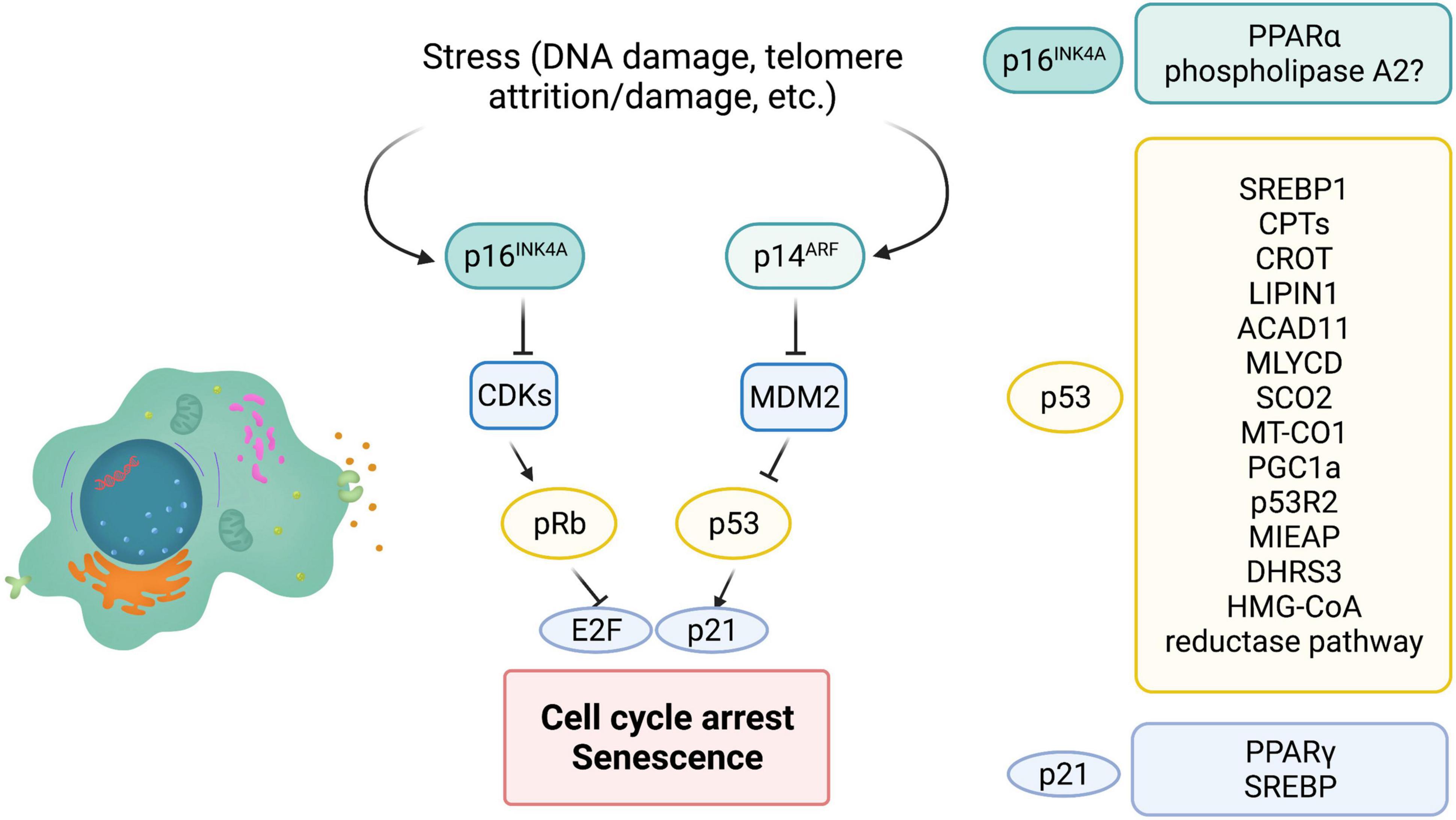
Figure 3. The role of major players of senescence in lipid metabolism. P16INK4a, p53, and p21 are major cell cycle regulators that control cellular senescence. INK4a/ARF encodes both p16 and ARF. MDM2 is an E3 ligase that ubiquitinates and leads to degradation of cellular senescence. ARF can inhibit MDM2 and therefore stabilize p53. P21 is a bonafide target of p53 and is partially responsible for cell cycle arrest and cellular senescence. P16 inhibits cyclin dependent kinases (CDK) thus maintaining Rb in a hypophosphorylated state and preventing E2F transcription factor activity. On the right are modulators of lipid metabolism that are regulated by p16, p53, and p21 (? = Possibly regulated by p16).
In humans, peripheral blood T lymphocytes exhibit increased expression of p16INK4a with age, tobacco smoking and physical inactivity. Similarly, p16-positive senescent T cells increase with age in mice (Yousefzadeh et al., 2021). In addition to aging, senescent T cells have been isolated from a number of tumors including lung and breast cancer (Meloni et al., 2006; Gruber et al., 2008; Urbaniak-Kujda et al., 2009). These senescent T cells from the tumor microenvironment seem to be dysfunctional and have reduced antitumor activity (Liu et al., 2018; Zhao et al., 2020). Interestingly, such senescent T cells with increased p16 expression display unbalanced lipid metabolism, leading to accumulation of lipid droplets (Liu et al., 2021), consistent with senescent cells in other tissues. Inhibition of the group IVA phospholipase A2, reprogrammed metabolism and prevented T cell senescence, suggesting that unbalanced lipid metabolism could be a key driver of T cell senescence (Liu et al., 2021). However, whether p16 directly controls lipid metabolism is still unknown in this model. One recent study did identify a direct link between p16 expression and lipid metabolism. During fasting, inhibition of p16 in primary hepatocytes enhanced activation of PPARα, a transcription factor with a major role in lipid metabolism, and increased fatty acid oxidation (Deleye et al., 2020; Figure 3). Consistent with this, p16 overexpression reduced mitochondrial activity and fatty acid oxidation, and led to accumulation of lipid droplets both in vitro and in vivo. However, p16 is known to have roles outside of senescence and these studies did not analyze cellular senescence in this fasting model. Of note, several studies (described above) suggest that elevated lipid β-oxidation is observed in senescent cells. Therefore, further studies exploring if p16 controls lipid catabolism during senescence will uncover insights into how p16 maintains long-term senescence.
p53
p53 is one of the most well-studied tumor suppressor, and is activated in response to various stressors including DNA damage, telomere attrition, mitochondrial, oxidative or oncogenic stress. Post-translational modification of p53, including phosphorylation, acetylation, sumoylation, ubiquitination and neddylation, regulate its activity. Upon acute stress, p53 is activated and can modulate DNA repair and quiescence (Vousden and Prives, 2009; Kasteri et al., 2018). However, chronic activation of p53 promotes cellular senescence (Sharpless and Depinho, 2006; Salama et al., 2014). p53 indirectly downregulates the expression of several genes required for cell cycle progression. Although the role of p53 in regulating the cell cycle is well-understood, recent studies indicate that p53 can also regulate metabolism and in particular lipid metabolism.
p53 enhances fatty acid oxidation and oxidative phosphorylation (OXPHOS), while inhibiting fatty acid synthesis. The role of p53 in suppressing lipid synthesis is prominent in the p53 knock out mouse models that exhibit obesity (Wang S. J. et al., 2016). Interestingly, induction of cellular senescence increases oxygen consumption and inhibits fatty acid synthesis, suggesting that p53 may play a pivotal role in rewiring the metabolic circuitry of senescent cells (Quijano et al., 2012). Inhibition of fatty acid synthesis by p53 is predominantly through repression of the master regulator of fatty acid synthesis, SREBP1 (Yahagi et al., 2003). SREBPs control the expression of multiple lipogenic enzymes, including acetyl-CoA carboxylase (ACC), ATP citrate lyase (ACLY), and fatty acid synthase (FASN) (Yahagi et al., 2003; Eberle et al., 2004). These observations stem from a model of obese (ob/ob) mice, that displayed elevated p53 and a concomitant downregulation of SREBP-1 and its targets, possibly by reducing promoter activity. Indeed, p53 deletion in the ob/ob mice partially rescues SREBP-1 expression (Yahagi et al., 2003).
Carnitine palmitoyltransferase 1A and 1C (CPT1A, CPT1C), the rate limiting enzyme for fatty acid oxidation and aid in the transport of fatty acids into the mitochondria are transcriptionally regulated by p53 (Sanchez-Macedo et al., 2013). Carnitine O-octanoyltransferase (CROT) another enzyme that facilitates export of fatty acids from the peroxisomes has also been identified as a p53 target (Goldstein et al., 2012). Once exported from the peroxisomes these fatty acids can undergo mitochondrial β-oxidation. Similarly, Acad11 that catalyzes the first step of mitochondrial fatty acid oxidation, is a transcriptional target of p53 (Jiang et al., 2015). DNA damage with doxorubicin, an agent that induces double strand breaks and activates p53, upregulated Acad11 expression in a p53-dependent manner (He et al., 2011; Jiang et al., 2015). Malonyl-CoA decarboxylase (MLYCD) is a mitochondrial enzyme that converts malonyl-CoA to acetyl-CoA and a critical regulator of fatty acid oxidation. In a recent study in mice exhibiting ribosomal stress, MLYCD transcription was controlled through p53 binding to its promoter and intron regions, and reduced lipid accumulation in the liver (Liu et al., 2014). Another bonafide p53 target, Lipin1 has three p53 binding sites in intron 1, and is important for adipose tissue development. DNA damage, particularly double strand breaks (DSBs) by γ-irradiation, that activates p53 leads to increased Lipin1 expression in several tissues including spleen and thymus (Assaily et al., 2011). Recent data in mouse myoblast C2C12 cells, shows that upon glucose starvation p53 upregulates Lipin 1 and fatty acid oxidation (Assaily et al., 2011). Additionally, Lipin1 has also been shown to interact with PGC-1α, another p53 target, to promote fatty acid oxidation (Finck et al., 2006; Sen et al., 2011). Clearly, activated wild-type p53 does seem to play a role in lipid metabolism, particularly fatty acid oxidation. However, whether chronic p53 activation that is known to promote cellular senescence, leads to similar changes has not yet been fully elucidated. Fatty acid oxidation is linked to OXPHOS, and provides reduced equivalents to the OXPHOS pathway. p53 has several targets that play a role in OXPHOS, including cytochrome c oxidase 2 (SCO2) (Matoba et al., 2006) and the mitochondrial encoded cytochrome c oxidase 1 (MT-CO1) (Okamura et al., 1999). p53 is also shown to translocate into the mitochondria and can interact with mitochondrial DNA polymerase γ (Achanta et al., 2005), control mitochondrial biogenesis through PGC1α, regulate mitochondrial DNA copy number and mitochondrial mass through p53-controlled ribonucleotide reductase (p53R2) (Bourdon et al., 2007) and is also involved in clearance of dysfunctional mitochondria by mitophagy through mitochondria-eating protein (Mieap) (Kitamura et al., 2011).
Interestingly, short-chain dehydrogenase reductase (DHRS3) that is localized to lipid droplets has also been identified as a target of p53 (Beilstein et al., 2016). There are two p53 responsible elements in the DHRS3 promoter, and several reports show that activated p53 promotes buildup of lipid droplets concomitant with an increase in DHRS3 (Kirschner et al., 2010; Deisenroth et al., 2011). In addition to FAO, OXPHOS and lipid droplet dynamics, p53 is also involved in cholesterol homeostasis. Caveolin 1 is a structural protein that binds to cholesterol. In an in vitro study, in human dermal fibroblasts, caveolin 1 was induced upon overexpression of p53 (Bist et al., 2000). This led to reduced intracellular cholesterol, suggesting the possible role of p53 in cholesterol efflux. The HMG-CoA reductase pathway is important for cholesterol synthesis and several enzymes in this pathway are p53 targets including 3′-hydroxy-3′-methylglutaryl-coenzyme A reductase, MVA kinase, farnesyl diphosphate synthase and farnesyl diphosphate farnesyl transferase 1 (Laezza et al., 2015). However, there are opposing reports on whether p53 activates or represses the HMG-CoA reductase pathway. Both studies use WT p53 expressing cell lines, however, one study uses astrocytes and glioblastoma cell lines whereas the other uses mouse embryonic fibroblasts, SK-HEP-1 and HCT116 cells (Laezza et al., 2015; Moon et al., 2019; Figure 3). Currently the role of HMG-CoA reductase pathway in cellular senescence is not clear, although a few reports suggest that inhibiting this pathway in pre-malignant cells promotes cellular senescence and inhibits tumorigenesis.
p21
A bonafide transcriptional target of p53 is p21WAF1/CIP1, a 21 kDa protein encoded by the CDKN1A gene (el-Deiry et al., 1993). p21 interacts with cyclin dependent kinases (CDKs), thus maintaining RB in a hypophosphorylated state and inhibiting cell cycle progression (Harper et al., 1993; Chen et al., 1996). Since, p21 binds several cyclin/CDK complexes, including CDK1, 2, 4 and 6, it can promote arrest at any stage of the cell cycle. In contrast p16 specifically binds to inactivate CDK4 and CDK6, thus arresting cells in G0/G1 phase (Harper et al., 1993; Pavletich, 1999). p21 can also bind proliferating cell nuclear antigen (PCNA) (Waga et al., 1994) and inhibit the cell cycle. It is important to note that p21 can be activated in a p53-independent manner (Aliouat-Denis et al., 2005; Abbas and Dutta, 2009). As mentioned above, p16 expression is required to maintain a senescent state. In contrast, p21 is crucial for initiation of senescence (Noda et al., 1994; Hernandez-Segura et al., 2017). Indeed, mice lacking p21WAF1/CIP1 showed defects in embryonic senescence leading to developmental defects (Munoz-Espin et al., 2013; Storer et al., 2013). Of note, embryonic senescence is a transient program thought to be beneficial and is resolved in a timely manner. Furthermore, several reports show that p21 expression does not persist in senescent cells (Stein et al., 1999; Sharpless and Sherr, 2015).
Role of p21 in lipid metabolism is not well understood. p21 does have an apparent role in adipocyte maintenance, however, outside of adipogenic effects not much is known about p21 in regulating lipid metabolism. There are some studies that report p21 as a pro-adipogenic factor. Knockdown or loss of p21 inhibited adipocyte differentiation (Inoue et al., 2008). Consistent with this, phosphorylation of p21 by MPK-38 led to nuclear translocation and increased association with peroxisome proliferator-activated receptor γ (PPARγ), thus inhibiting the role of PPARγ in adipogenesis. Furthermore, in this study expression of p21 rescued the metabolic phenotypes observed. Contradicting these studies, loss of p21 showed an increase in adipocyte hyperplasia (Naaz et al., 2004). In this study loss of p21 led to spontaneous adipose conversion and adipocyte hyperplasia in mice. However, effects in the study of Inoue et al. were observed in the terminal differentiation phase of the adipogenic program, whereas the anti-adipogenic effects were evident in the earlier determination phase of adipogenesis. Interestingly, p21 was identified as a target of SREBPs. Both SREBP-1a and SREBP-2 binding sites exist in the p21 promoter (Inoue et al., 2005). Furthermore, SREBP-1a led to p21 expression and cell cycle inhibition, under conditions of lipid deprivation. Consistent with this observation, SREBP null mice had reduced expression of p21 (Figure 3). It will be of great interest to examine whether expression of p21 leads to differential lipid changes during “acute” versus “chronic” senescence. Although, p16, p53, and p21 are key molecular players in cellular senescence their exact role in modulating lipid metabolism, reprogramming lipid composition and generation of bioactive lipid species is not well explored.
Potential Role of Lipids as Therapeutics in Targeting Senescence
Cellular senescence is a defining feature of aging, as well as a contributor to chronic non-healing wounds and general tissue aging pathology. Several key bioactive lipids such as cytochrome P450 (CYP)-derived epoxyeicosatrienoic acids (EETs), leukoterines, lipoxins described above are known to play a significant role in tissue repair. In fact, EETs have been shown as a viable angiogenic therapeutic strategy for its ability to accelerate wound epithelization (Sander et al., 2013). Long term inhibition of PGE2 degradation significantly reduced pulmonary fibrosis lesions in mouse model of bleomycin-induced lung senescence. In contrast, profibrotic leukotriene synthesis is elevated in IPF. Although PGE2 inhibition holds great promise as a stand-alone treatment or in combination with currently approved therapies for idiopathic pulmonary fibrosis, modulating eicosanoid metabolism in context of senescence can open up therapeutic avenues and provide mechanistic clues into tissue aging. Although the above studies have highlighted the beneficial role of senescent cells in wound healing, it is still a paradox as to how these senescent cells also impair wound healing in aging. A temporal lipidome analysis of senescent cells from different tissues would provide insights into lipid changes that occur in senescent cells of aged tissues, as well as allow for the development of tailored senescence-targeting therapeutics.
Tools to Identify Functional Lipids and Current Challenges
The ubiquitous and structurally diverse nature of lipids molecules and its varied abundance across cells and tissues contributes to the complexity in the process of lipid identification. There are various techniques available for studying individual lipids and the lipidome, each with its own set of constraints. Understanding the benefits and limitations of these approaches is therefore crucial in identifying lipids within a biological model. With the emergence of lipidomics, there has been several advancements in analytical techniques such as soft ionization methods, high resolution mass spectrometry, tandem mass spectrometry, MS imaging and development of spectral libraries and lipid database such as LIPIDMAPS. This has led to not only identifying novel lipids but also unravel mechanisms responsible for pathophysiological conditions, biomarkers for early diagnosis and drug targeting (Quehenberger et al., 2010; Wang M. et al., 2016). Advances in high-resolution lipidomics platforms have made it possible to investigate lipidome changes associated with aging and age-associated diseases (Wong et al., 2019). For example, both targeted and untargeted lipidomics approaches provided insights into phospholipid remodeling in senescent cells, led to the identification of 15d-PGJ2 as a biomarker of senolysis and elevated ceramide C16:0 levels as prognostic marker for functional decline (Wennberg et al., 2018; Millner and Atilla-Gokcumen, 2020; Wiley et al., 2021). Despite advancements made in this field, the complexity in terms of chemistry, diversity, and abundances necessitates the continued pursuit of technical innovation. For example, identifying certain isomers of eicosanoids, a variety of sphingolipids, phospholipid intermediates are still challenging to be quantified accurately. Developing the informatics tools or libraries to interpret a multitude of lipid species and large sets of lipidomics data has been challenging. Nevertheless, a lot of progress has been made toward fine tuning of high-resolution mass spectrometry and precise identification of lipid structures. Multi-omic data integration approaches, dynamic lipidomics to asses turnover kinetics, single cell lipidomics has generated a lot of interest and holds promise in advancing the field forward.
Unanswered Questions in the Field
With advances in technology, we have unraveled that lipids do play a role in cellular senescence. However, we are left with several unanswered and interesting questions: Do different stages of cellular senescence (e.g., initiation versus late senescence) have different lipid compositions? If so is it causal in initiation or maintenance of senescence? While the lipids discussed in this review have been linked to senescence, more research is needed to establish cause or consequence effect. In addition to this, the link between major senescent players- p53, p21, or p16, and lipid alterations is still to be uncovered. Importantly, it will be interesting to understand whether lipids could act as biomarkers for senescence and/or senolysis, hence making intervention possible in the near future.
Cellular senescence, in summary is a cell-fate process associated with several age-related pathologies including frailty, Alzheimer’s disease, Parkinson disease, atherosclerosis, idiopathic pulmonary fibrosis, chronic obstructive pulmonary disease, and osteoarthritis (Schafer et al., 2017; Chinta et al., 2018; Sagiv et al., 2018). Indeed, the National Institutes of Health (NIH) has recently started a new initiative, The Common Fund’s Cellular Senescence Network (SenNet) Program, to better understand the key hallmarks of cellular senescence and map the burden of senescent cells in human. This further highlights the critical need of understanding cellular senescence. Additionally, directed removal of senescent cells or associated SASP improves health considerably, at least in animal models (Zhu et al., 2015; Jeon et al., 2017; Bussian et al., 2018; Guerrero et al., 2019; Zhang et al., 2019). Further understanding the role of lipids in cellular senescence will open new therapeutic approaches for several age-related diseases.
Author Contributions
SH and AUG reviewed the literature, authored the review, contributed to the article, and approved the submitted version.
Funding
This work was supported by NIH grants R00AG049126 and U54AG075931 (AUG).
Conflict of Interest
The authors declare that the research was conducted in the absence of any commercial or financial relationships that could be construed as a potential conflict of interest.
Publisher’s Note
All claims expressed in this article are solely those of the authors and do not necessarily represent those of their affiliated organizations, or those of the publisher, the editors and the reviewers. Any product that may be evaluated in this article, or claim that may be made by its manufacturer, is not guaranteed or endorsed by the publisher.
Acknowledgments
We thank Aakriti Kallianpur for help with the illustrations and Tamil Anthonymuthu for critical discussions on the manuscript.
References
Abbas, T., and Dutta, A. (2009). p21 in cancer: intricate networks and multiple activities. Nat. Rev. Cancer 9, 400–414. doi: 10.1038/nrc2657
Achanta, G., Sasaki, R., Feng, L., Carew, J. S., Lu, W., Pelicano, H., et al. (2005). Novel role of p53 in maintaining mitochondrial genetic stability through interaction with DNA Pol gamma. EMBO J. 24, 3482–3492. doi: 10.1038/sj.emboj.7600819
Aliouat-Denis, C. M., Dendouga, N., Van denWyngaert, I., Goehlmann, H., Steller, U., van deWeyer, I., et al. (2005). p53-independent regulation of p21Waf1/Cip1 expression and senescence by Chk2. Mol. Cancer Res. 3, 627–634. doi: 10.1158/1541-7786.MCR-05-0121
Alkayed, N. J., Narayanan, J., Gebremedhin, D., Medhora, M., Roman, R. J., and Harder, D. R. (1996). Molecular characterization of an arachidonic acid epoxygenase in rat brain astrocytes. Stroke 27, 971–979. doi: 10.1161/01.str.27.5.971
Assaily, W., Rubinger, D. A., Wheaton, K., Lin, Y., Ma, W., Xuan, W., et al. (2011). ROS-mediated p53 induction of Lpin1 regulates fatty acid oxidation in response to nutritional stress. Mol. Cell 44, 491–501. doi: 10.1016/j.molcel.2011.08.038
Badawi, A. F., Liu, Y., Eldeen, M. B., Morrow, W., Razak, Z. R., Maradeo, M., et al. (2005). Age-associated changes in the expression pattern of cyclooxygenase-2 and related apoptotic markers in the cancer susceptible region of rat prostate. Carcinogenesis 26:1650.
Badawi, A. F., Liu, Y., Eldeen, M. B., Morrow, W., Razak, Z. R., Maradeo, M., et al. (2004). Age-associated changes in the expression pattern of cyclooxygenase-2 and related apoptotic markers in the cancer susceptible region of rat prostate. Carcinogenesis 25, 1681–1688. doi: 10.1093/carcin/bgh176
Baek, B. S., Kim, J. W., Lee, J. H., Kwon, H. J., Kim, N. D., Kang, H. S., et al. (2001). Age-related increase of brain cyclooxygenase activity and dietary modulation of oxidative status. J. Gerontol. A Biol. Sci. Med. Sci. 56, B426–B431. doi: 10.1093/gerona/56.10.b426
Baker, D. J., Perez-Terzic, C., Jin, F., Pitel, K. S., Niederlander, N. J., Jeganathan, K., et al. (2008). Opposing roles for p16Ink4a and p19Arf in senescence and ageing caused by BubR1 insufficiency. Nat. Cell Biol. 10, 825–836. doi: 10.1038/ncb1744
Baker, D. J., Wijshake, T., Tchkonia, T., LeBrasseur, N. K., Childs, B. G., van deSluis, B., et al. (2011). Clearance of p16Ink4a-positive senescent cells delays ageing-associated disorders. Nature 479, 232–236. doi: 10.1038/nature10600
Bansal, P., Gaur, S. N., and Arora, N. (2016). Lysophosphatidylcholine plays critical role in allergic airway disease manifestation. Sci. Rep. 6:27430. doi: 10.1038/srep27430
Baraibar, M. A., Hyzewicz, J., Rogowska-Wrzesinska, A., Bulteau, A. L., Prip-Buus, C., Butler-Browne, G., et al. (2016). Impaired energy metabolism of senescent muscle satellite cells is associated with oxidative modifications of glycolytic enzymes. Aging (Albany NY) 8, 3375–3389. doi: 10.18632/aging.101126
Basisty, N., Kale, A., Jeon, O. H., Kuehnemann, C., Payne, T., Rao, C., et al. (2020). A proteomic atlas of senescence-associated secretomes for aging biomarker development. PLoS Biol. 18:e3000599. doi: 10.1371/journal.pbio.3000599
Beilstein, F., Carriere, V., Leturque, A., and Demignot, S. (2016). Characteristics and functions of lipid droplets and associated proteins in enterocytes. Exp. Cell Res. 340, 172–179. doi: 10.1016/j.yexcr.2015.09.018
Bianco, F., Perrotta, C., Novellino, L., Francolini, M., Riganti, L., Menna, E., et al. (2009). Acid sphingomyelinase activity triggers microparticle release from glial cells. EMBO J. 28, 1043–1054. doi: 10.1038/emboj.2009.45
Biran, A., Zada, L., Abou Karam, P., Vadai, E., Roitman, L., Ovadya, Y., et al. (2017). Quantitative identification of senescent cells in aging and disease. Aging Cell 16, 661–671. doi: 10.1111/acel.12592
Bist, A., Fielding, C. J., and Fielding, P. E. (2000). p53 regulates caveolin gene transcription, cell cholesterol, and growth by a novel mechanism. Biochemistry 39, 1966–1972. doi: 10.1021/bi991721h
Borghesan, M., Fafian-Labora, J., Eleftheriadou, O., Carpintero-Fernandez, P., Paez-Ribes, M., Vizcay-Barrena, G., et al. (2019). Small extracellular vesicles are key regulators of non-cell autonomous intercellular communication in senescence via the interferon protein IFITM3. Cell Rep. 27, 3956–3971.e6. doi: 10.1016/j.celrep.2019.05.095
Bourdon, A., Minai, L., Serre, V., Jais, J. P., Sarzi, E., Aubert, S., et al. (2007). Mutation of RRM2B, encoding p53-controlled ribonucleotide reductase (p53R2), causes severe mitochondrial DNA depletion. Nat. Genet. 39, 776–780. doi: 10.1038/ng2040
Buratta, S., Urbanelli, L., Sagini, K., Giovagnoli, S., Caponi, S., Fioretto, D., et al. (2017). Extracellular vesicles released by fibroblasts undergoing H-Ras induced senescence show changes in lipid profile. PLoS One 12:e0188840. doi: 10.1371/journal.pone.0188840
Burd, C. E., Sorrentino, J. A., Clark, K. S., Darr, D. B., Krishnamurthy, J., Deal, A. M., et al. (2013). Monitoring tumorigenesis and senescence in vivo with a p16(INK4a)-luciferase model. Cell 152, 340–351. doi: 10.1016/j.cell.2012.12.010
Bussian, T. J., Aziz, A., Meyer, C. F., Swenson, B. L., van Deursen, J. M., and Baker, D. J. (2018). Clearance of senescent glial cells prevents tau-dependent pathology and cognitive decline. Nature 562, 578–582. doi: 10.1038/s41586-018-0543-y
Cadenas, C., Vosbeck, S., Hein, E. M., Hellwig, B., Langer, A., Hayen, H., et al. (2012). Glycerophospholipid profile in oncogene-induced senescence. Biochim. Biophys. Acta 1821, 1256–1268. doi: 10.1016/j.bbalip.2011.11.008
Campbell, W. B., Imig, J. D., Schmitz, J. M., and Falck, J. R. (2017). Orally active epoxyeicosatrienoic acid analogs. J. Cardiovasc. Pharmacol. 70, 211–224.
Chee, W. Y., Kurahashi, Y., Kim, J., Miura, K., Okuzaki, D., Ishitani, T., et al. (2021). beta-catenin-promoted cholesterol metabolism protects against cellular senescence in naked mole-rat cells. Commun. Biol. 4:357. doi: 10.1038/s42003-021-01879-8
Chen, J., Saha, P., Kornbluth, S., Dynlacht, B. D., and Dutta, A. (1996). Cyclin-binding motifs are essential for the function of p21CIP1. Mol. Cell Biol. 16, 4673–4682. doi: 10.1128/MCB.16.9.4673
Chen, P., Zhang, Q., Zhang, H., Gao, Y., Zhou, Y., Chen, Y., et al. (2021). Carnitine palmitoyltransferase 1C reverses cellular senescence of MRC-5 fibroblasts via regulating lipid accumulation and mitochondrial function. J. Cell Physiol. 236, 958–970. doi: 10.1002/jcp.29906
Chen, W. M., Chiang, J. C., Lin, Y. C., Lin, Y. N., Chuang, P. Y., Chang, Y. C., et al. (2020). Lysophosphatidic acid receptor LPA3 prevents oxidative stress and cellular senescence in Hutchinson-Gilford progeria syndrome. Aging Cell 19:e13064. doi: 10.1111/acel.13064
Chinta, S. J., Woods, G., Demaria, M., Rane, A., Zou, Y., McQuade, A., et al. (2018). Cellular senescence is induced by the environmental neurotoxin paraquat and contributes to neuropathology linked to Parkinson’s disease. Cell Rep. 22, 930–940. doi: 10.1016/j.celrep.2017.12.092
Choi, Y. J., Kim, H. S., Lee, J., Chung, J., Lee, J. S., Choi, J. S., et al. (2014). Down-regulation of oxidative stress and COX-2 and iNOS expressions by dimethyl lithospermate in aged rat kidney. Arch. Pharm. Res. 37, 1032–1038. doi: 10.1007/s12272-014-0332-6
Chong, M., Yin, T., Chen, R., Xiang, H., Yuan, L., Ding, Y., et al. (2018). CD36 initiates the secretory phenotype during the establishment of cellular senescence. EMBO Rep. 19:e45274. doi: 10.15252/embr.201745274
Chung, H. Y., Kim, H. J., Shim, K. H., and Kim, K. W. (1999). Dietary modulation of prostanoid synthesis in the aging process: role of cyclooxygenase-2. Mech. Ageing Dev. 111, 97–106. doi: 10.1016/s0047-6374(99)00061-5
Coppe, J. P., Patil, C. K., Rodier, F., Krtolica, A., Beausejour, C. M., Parrinello, S., et al. (2010). A human-like senescence-associated secretory phenotype is conserved in mouse cells dependent on physiological oxygen. PLoS One 5:e9188. doi: 10.1371/journal.pone.0009188
Correia-Melo, C., Marques, F. D., Anderson, R., Hewitt, G., Hewitt, R., Cole, J., et al. (2016). Mitochondria are required for pro-ageing features of the senescent phenotype. EMBO J. 35, 724–742. doi: 10.15252/embj.201592862
Cox, L. S., and Redman, C. (2017). The role of cellular senescence in ageing of the placenta. Placenta 52, 139–145. doi: 10.1016/j.placenta.2017.01.116
Cutler, R. G., and Mattson, M. P. (2001). Sphingomyelin and ceramide as regulators of development and lifespan. Mech. Ageing Dev. 122, 895–908. doi: 10.1016/s0047-6374(01)00246-9
Dagouassat, M., Gagliolo, J. M., Chrusciel, S., Bourin, M. C., Duprez, C., Caramelle, P., et al. (2013). The cyclooxygenase-2-prostaglandin E2 pathway maintains senescence of chronic obstructive pulmonary disease fibroblasts. Am. J. Respir. Crit. Care Med. 187, 703–714. doi: 10.1164/rccm.201208-1361OC
Dbaibo, G. S., Pushkareva, M. Y., Rachid, R. A., Alter, N., Smyth, M. J., Obeid, L. M., et al. (1998). p53-dependent ceramide response to genotoxic stress. J. Clin. Invest. 102, 329–339. doi: 10.1172/JCI1180
Deisenroth, C., Itahana, Y., Tollini, L., Jin, A., and Zhang, Y. (2011). p53-Inducible DHRS3 is an endoplasmic reticulum protein associated with lipid droplet accumulation. J. Biol. Chem. 286, 28343–28356. doi: 10.1074/jbc.M111.254227
Deleye, Y., Cotte, A. K., Hannou, S. A., Hennuyer, N., Bernard, L., Derudas, B., et al. (2020). CDKN2A/p16INK4a suppresses hepatic fatty acid oxidation through the AMPKalpha2-SIRT1-PPARalpha signaling pathway. J. Biol. Chem. 295, 17310–17322. doi: 10.1074/jbc.RA120.012543
Demaria, M., Ohtani, N., Youssef, S. A., Rodier, F., Toussaint, W., Mitchell, J. R., et al. (2014). An essential role for senescent cells in optimal wound healing through secretion of PDGF-AA. Dev. Cell 31, 722–733. doi: 10.1016/j.devcel.2014.11.012
Di Micco, R., Sulli, G., Dobreva, M., Liontos, M., Botrugno, O. A., Gargiulo, G., et al. (2011). Interplay between oncogene-induced DNA damage response and heterochromatin in senescence and cancer. Nat. Cell Biol. 13, 292–302. doi: 10.1038/ncb2170
Dimri, G. P., Lee, X., Basile, G., Acosta, M., Scott, G., Roskelley, C., et al. (1995). A biomarker that identifies senescent human cells in culture and in aging skin in vivo. Proc. Natl. Acad. Sci. U.S.A. 92, 9363–9367. doi: 10.1073/pnas.92.20.9363
Dou, Z., Ghosh, K., Vizioli, M. G., Zhu, J., Sen, P., Wangensteen, K. J., et al. (2017). Cytoplasmic chromatin triggers inflammation in senescence and cancer. Nature 550, 402–406. doi: 10.1038/nature24050
Dou, Z., Xu, C., Donahue, G., Shimi, T., Pan, J. A., Zhu, J., et al. (2015). Autophagy mediates degradation of nuclear lamina. Nature 527, 105–109. doi: 10.1038/nature15548
Eberle, D., Hegarty, B., Bossard, P., Ferre, P., and Foufelle, F. (2004). SREBP transcription factors: master regulators of lipid homeostasis. Biochimie 86, 839–848. doi: 10.1016/j.biochi.2004.09.018
el-Deiry, W. S., Tokino, T., Velculescu, V. E., Levy, D. B., Parsons, R., Trent, J. M., et al. (1993). WAF1, a potential mediator of p53 tumor suppression. Cell 75, 817–825. doi: 10.1016/0092-8674(93)90500-p
Eren, M., Boe, A. E., Murphy, S. B., Place, A. T., Nagpal, V., Morales-Nebreda, L., et al. (2014). PAI-1-regulated extracellular proteolysis governs senescence and survival in Klotho mice. Proc. Natl. Acad. Sci. U.S.A. 111, 7090–7095. doi: 10.1073/pnas.1321942111
Evangelou, K., Lougiakis, N., Rizou, S. V., Kotsinas, A., Kletsas, D., Munoz-Espin, D., et al. (2017). Universal biomarker assay to detect senescent cells in biological specimens. Aging Cell 16, 192–197. doi: 10.1111/acel.12545
Fafian-Labora, J., Carpintero-Fernandez, P., Jordan, S. J. D., Shikh-Bahaei, T., Abdullah, S. M., Mahenthiran, M., et al. (2019). FASN activity is important for the initial stages of the induction of senescence. Cell Death Dis. 10:318. doi: 10.1038/s41419-019-1550-0
Finck, B. N., Gropler, M. C., Chen, Z., Leone, T. C., Croce, M. A., Harris, T. E., et al. (2006). Lipin 1 is an inducible amplifier of the hepatic PGC-1alpha/PPARalpha regulatory pathway. Cell Metab. 4, 199–210. doi: 10.1016/j.cmet.2006.08.005
Flor, A. C., Wolfgeher, D., Wu, D., and Kron, S. J. (2017). A signature of enhanced lipid metabolism, lipid peroxidation and aldehyde stress in therapy-induced senescence. Cell Death Discov. 3:17075. doi: 10.1038/cddiscovery.2017.75
Fortney, K., Dobriban, E., Garagnani, P., Pirazzini, C., Monti, D., Mari, D., et al. (2015). Genome-wide scan informed by age-related disease identifies loci for exceptional human longevity. PLoS Genet. 11:e1005728. doi: 10.1371/journal.pgen.1005728
Freund, A., Patil, C. K., and Campisi, J. (2011). p38MAPK is a novel DNA damage response-independent regulator of the senescence-associated secretory phenotype. EMBO J. 30, 1536–1548. doi: 10.1038/emboj.2011.69
Giusto, N. M., Roque, M. E., and Ilincheta de Boschero, M. G. (1992). Effects of aging on the content, composition and synthesis of sphingomyelin in the central nervous system. Lipids 27, 835–839. doi: 10.1007/BF02535859
Goldstein, I., Ezra, O., Rivlin, N., Molchadsky, A., Madar, S., Goldfinger, N., et al. (2012). p53, a novel regulator of lipid metabolism pathways. J. Hepatol. 56, 656–662. doi: 10.1016/j.jhep.2011.08.022
Greenberg, M. E., Li, X. M., Gugiu, B. G., Gu, X., Qin, J., Salomon, R. G., et al. (2008). The lipid whisker model of the structure of oxidized cell membranes. J. Biol. Chem. 283, 2385–2396. doi: 10.1074/jbc.M707348200
Greenberg, M. E., Sun, M., Zhang, R., Febbraio, M., Silverstein, R., and Hazen, S. L. (2006). Oxidized phosphatidylserine-CD36 interactions play an essential role in macrophage-dependent phagocytosis of apoptotic cells. J. Exp. Med. 203, 2613–2625. doi: 10.1084/jem.20060370
Gruber, I. V., El Yousfi, S., Durr-Storzer, S., Wallwiener, D., Solomayer, E. F., and Fehm, T. (2008). Down-regulation of CD28, TCR-zeta (zeta) and up-regulation of FAS in peripheral cytotoxic T-cells of primary breast cancer patients. Anticancer Res. 28, 779–784.
Guerrero, A., Herranz, N., Sun, B., Wagner, V., Gallage, S., Guiho, R., et al. (2019). Cardiac glycosides are broad-spectrum senolytics. Nat. Metab. 1, 1074–1088. doi: 10.1038/s42255-019-0122-z
Hall, B. M., Balan, V., Gleiberman, A. S., Strom, E., Krasnov, P., Virtuoso, L. P., et al. (2017). p16(Ink4a) and senescence-associated beta-galactosidase can be induced in macrophages as part of a reversible response to physiological stimuli. Aging (Albany NY) 9, 1867–1884. doi: 10.18632/aging.101268
Handa, J. T., Tagami, M., Ebrahimi, K., Leibundgut, G., Janiak, A., Witztum, J. L., et al. (2015). Lipoprotein(A) with an intact lysine binding site protects the retina from an age-related macular degeneration phenotype in mice (an american ophthalmological society thesis). Trans. Am. Ophthalmol. Soc. 113:T5.
Hannun, Y. A., and Obeid, L. M. (2018). Sphingolipids and their metabolism in physiology and disease. Nat. Rev. Mol. Cell Biol. 19, 175–191. doi: 10.1038/nrm.2017.107
Harper, J. W., Adami, G. R., Wei, N., Keyomarsi, K., and Elledge, S. J. (1993). The p21 Cdk-interacting protein Cip1 is a potent inhibitor of G1 cyclin-dependent kinases. Cell 75, 805–816. doi: 10.1016/0092-8674(93)90499-g
Hayek, M. G., Mura, C., Wu, D., Beharka, A. A., Han, S. N., Paulson, K. E., et al. (1997). Enhanced expression of inducible cyclooxygenase with age in murine macrophages. J. Immunol. 159, 2445–2451.
He, M., Pei, Z., Mohsen, A. W., Watkins, P., Murdoch, G., Van Veldhoven, P.P., et al. (2011). Identification and characterization of new long chain acyl-CoA dehydrogenases. Mol Genet Metab 102, 418–429. doi: 10.1016/j.ymgme.2010.12.005
Heffernan-Stroud, L. A., and Obeid, L. M. (2011). p53 and regulation of bioactive sphingolipids. Adv. Enzyme Regul. 51, 219–228. doi: 10.1016/j.advenzreg.2010.10.003
Hernandez-Segura, A., de Jong, T. V., Melov, S., Guryev, V., Campisi, J., and Demaria, M. (2017). Unmasking transcriptional heterogeneity in senescent cells. Curr. Biol. 27, 2652–2660.e4. doi: 10.1016/j.cub.2017.07.033
Herranz, N., and Gil, J. (2018). Mechanisms and functions of cellular senescence. J. Clin. Invest. 128, 1238–1246. doi: 10.1172/jci95148
Herranz, N., Gallage, S., Mellone, M., Wuestefeld, T., Klotz, S., Hanley, C. J., et al. (2015). mTOR regulates MAPKAPK2 translation to control the senescence-associated secretory phenotype. Nat. Cell Biol. 17, 1205–1217. doi: 10.1038/ncb3225
Hirsova, P., Ibrahim, S. H., Krishnan, A., Verma, V. K., Bronk, S. F., Werneburg, N. W., et al. (2016). Lipid-induced signaling causes release of inflammatory extracellular vesicles from hepatocytes. Gastroenterology 150, 956–967. doi: 10.1053/j.gastro.2015.12.037
Horn, A., and Jaiswal, J. K. (2019). Structural and signaling role of lipids in plasma membrane repair. Curr. Top. Membr. 84, 67–98. doi: 10.1016/bs.ctm.2019.07.001
Idda, M. L., McClusky, W. G., Lodde, V., Munk, R., Abdelmohsen, K., Rossi, M., et al. (2020). Survey of senescent cell markers with age in human tissues. Aging (Albany NY) 12, 4052–4066. doi: 10.18632/aging.102903
Iliff, J. J., Wang, R., Zeldin, D. C., and Alkayed, N. J. (2009). Epoxyeicosanoids as mediators of neurogenic vasodilation in cerebral vessels. Am. J. Physiol. Heart Circ. Physiol. 296, H1352–H1363. doi: 10.1152/ajpheart.00950.2008
Inoue, N., Shimano, H., Nakakuki, M., Matsuzaka, T., Nakagawa, Y., Yamamoto, T., et al. (2005). Lipid synthetic transcription factor SREBP-1a activates p21WAF1/CIP1, a universal cyclin-dependent kinase inhibitor. Mol. Cell Biol. 25, 8938–8947. doi: 10.1128/MCB.25.20.8938-8947.2005
Inoue, N., Yahagi, N., Yamamoto, T., Ishikawa, M., Watanabe, K., Matsuzaka, T., et al. (2008). Cyclin-dependent kinase inhibitor, p21WAF1/CIP1, is involved in adipocyte differentiation and hypertrophy, linking to obesity, and insulin resistance. J. Biol. Chem. 283, 21220–21229. doi: 10.1074/jbc.M801824200
Ito, K., and Ito, K. (2016). Metabolism and the Control of cell fate decisions and stem cell renewal. Annu. Rev. Cell Dev. Biol. 32, 399–409. doi: 10.1146/annurev-cellbio-111315-125134
Ito, Y., Hoare, M., and Narita, M. (2017). Spatial and temporal control of senescence. Trends Cell Biol. 27, 820–832. doi: 10.1016/j.tcb.2017.07.004
James, E. L., Michalek, R. D., Pitiyage, G. N., de Castro, A. M., Vignola, K. S., Jones, J., et al. (2015). Senescent human fibroblasts show increased glycolysis and redox homeostasis with extracellular metabolomes that overlap with those of irreparable DNA damage, aging, and disease. J. Proteome Res. 14, 1854–1871. doi: 10.1021/pr501221g
Jang, I. S., Rhim, J. H., Kim, K. T., Cho, K. A., Yeo, E. J., and Park, S. C. (2006). Lysophosphatidic acid-induced changes in cAMP profiles in young and senescent human fibroblasts as a clue to the ageing process. Mech. Ageing Dev. 127, 481–489. doi: 10.1016/j.mad.2006.01.004
Jeck, W. R., Siebold, A. P., and Sharpless, N. E. (2012). Review: a meta-analysis of GWAS and age-associated diseases. Aging Cell 11, 727–731. doi: 10.1111/j.1474-9726.2012.00871.x
Jeon, O. H., Kim, C., Laberge, R. M., Demaria, M., Rathod, S., Vasserot, A. P., et al. (2017). Local clearance of senescent cells attenuates the development of post-traumatic osteoarthritis and creates a pro-regenerative environment. Nat. Med 23, 775–781. doi: 10.1038/nm.4324
Jiang, D., LaGory, E. L., Kenzelmann Broz, D., Bieging, K. T., Brady, C. A., Link, N., et al. (2015). Analysis of p53 transactivation domain mutants reveals Acad11 as a metabolic target important for p53 pro-survival function. Cell Rep. 10, 1096–1109. doi: 10.1016/j.celrep.2015.01.043
Jun, J. I., and Lau, L. F. (2010). The matricellular protein CCN1 induces fibroblast senescence and restricts fibrosis in cutaneous wound healing. Nat. Cell Biol. 12, 676–685. doi: 10.1038/ncb2070
Kakisaka, K., Cazanave, S. C., Fingas, C. D., Guicciardi, M. E., Bronk, S. F., Werneburg, N. W., et al. (2012). Mechanisms of lysophosphatidylcholine-induced hepatocyte lipoapoptosis. Am. J. Physiol. Gastrointest. Liver Physiol. 302, G77–G84. doi: 10.1152/ajpgi.00301.2011
Kamata, M., Amano, H., Ito, Y., Fujita, T., Otaka, F., Hosono, K., et al. (2019). Role of the high-affinity leukotriene B4 receptor signaling in fibrosis after unilateral ureteral obstruction in mice. PLoS One 14:e0202842. doi: 10.1371/journal.pone.0202842
Kanehira, M., Fujiwara, T., Nakajima, S., Okitsu, Y., Onishi, Y., Fukuhara, N., et al. (2017). An lysophosphatidic acid receptors 1 and 3 axis governs cellular senescence of mesenchymal stromal cells and promotes growth and vascularization of multiple myeloma. Stem Cells 35, 739–753. doi: 10.1002/stem.2499
Kang, K. B., Van Der Zypp, A., Iannazzo, L., and Majewski, H. (2006). Age-related changes in monocyte and platelet cyclooxygenase expression in healthy male humans and rats. Transl. Res. 148, 289–294. doi: 10.1016/j.trsl.2006.06.004
Kasteri, J., Das, D., Zhong, X., Persaud, L., Francis, A., Muharam, H., et al. (2018). Translation control by p53. Cancers (Basel) 10:133. doi: 10.3390/cancers10050133
Kim, J. W., Baek, B. S., Kim, Y. K., Herlihy, J. T., Ikeno, Y., Yu, B. P., et al. (2001). Gene expression of cyclooxygenase in the aging heart. J. Gerontol. A Biol. Sci. Med. Sci. 56, B350–B355. doi: 10.1093/gerona/56.8.b350
Kim, J., Vaish, V., Feng, M., Field, K., Chatzistamou, I., and Shim, M. (2016). Transgenic expression of cyclooxygenase-2 (COX2) causes premature aging phenotypes in mice. Aging (Albany NY) 8, 2392–2406. doi: 10.18632/aging.101060
Kim, Y. M., Shin, H. T., Seo, Y. H., Byun, H. O., Yoon, S. H., Lee, I. K., et al. (2010). Sterol regulatory element-binding protein (SREBP)-1-mediated lipogenesis is involved in cell senescence. J. Biol. Chem. 285, 29069–29077. doi: 10.1074/jbc.M110.120386
Kirschner, R. D., Rother, K., Muller, G. A., and Engeland, K. (2010). The retinal dehydrogenase/reductase retSDR1/DHRS3 gene is activated by p53 and p63 but not by mutants derived from tumors or EEC/ADULT malformation syndromes. Cell Cycle 9, 2177–2188. doi: 10.4161/cc.9.11.11844
Kitamura, N., Nakamura, Y., Miyamoto, Y., Miyamoto, T., Kabu, K., Yoshida, M., et al. (2011). Mieap, a p53-inducible protein, controls mitochondrial quality by repairing or eliminating unhealthy mitochondria. PLoS One 6:e16060. doi: 10.1371/journal.pone.0016060
Krishnamurthy, J., Torrice, C., Ramsey, M. R., Kovalev, G. I., Al-Regaiey, K., Su, L., et al. (2004). Ink4a/Arf expression is a biomarker of aging. J. Clin. Invest. 114, 1299–1307. doi: 10.1172/JCI22475
Krizhanovsky, V., Yon, M., Dickins, R. A., Hearn, S., Simon, J., Miething, C., et al. (2008). Senescence of activated stellate cells limits liver fibrosis. Cell 134, 657–667. doi: 10.1016/j.cell.2008.06.049
Laezza, C., D’Alessandro, A., Di Croce, L., Picardi, P., Ciaglia, E., Pisanti, S., et al. (2015). p53 regulates the mevalonate pathway in human glioblastoma multiforme. Cell Death Dis. 6:e1909. doi: 10.1038/cddis.2015.279
Lehmann, B. D., Paine, M. S., Brooks, A. M., McCubrey, J. A., Renegar, R. H., Wang, R., et al. (2008). Senescence-associated exosome release from human prostate cancer cells. Cancer Res. 68, 7864–7871. doi: 10.1158/0008-5472.CAN-07-6538
Li, R., Xu, X., Chen, C., Yu, X., Edin, M. L., Degraff, L. M., et al. (2012). Cytochrome P450 2J2 is protective against global cerebral ischemia in transgenic mice. Prostaglandins Other Lipid Mediat. 99, 68–78. doi: 10.1016/j.prostaglandins.2012.09.004
Lightle, S. A., Oakley, J. I., and Nikolova-Karakashian, M. N. (2000). Activation of sphingolipid turnover and chronic generation of ceramide and sphingosine in liver during aging. Mech. Ageing Dev. 120, 111–125. doi: 10.1016/s0047-6374(00)00191-3
Liu, J. Y., Souroullas, G. P., Diekman, B. O., Krishnamurthy, J., Hall, B. M., Sorrentino, J. A., et al. (2019). Cells exhibiting strong p16 (INK4a) promoter activation in vivo display features of senescence. Proc. Natl. Acad. Sci. U.S.A. 116, 2603–2611. doi: 10.1073/pnas.1818313116
Liu, X., Hartman, C. L., Li, L., Albert, C. J., Si, F., Gao, A., et al. (2021). Reprogramming lipid metabolism prevents effector T cell senescence and enhances tumor immunotherapy. Sci. Transl. Med. 13:eaaz6314. doi: 10.1126/scitranslmed.aaz6314
Liu, X., Mo, W., Ye, J., Li, L., Zhang, Y., Hsueh, E. C., et al. (2018). Regulatory T cells trigger effector T cell DNA damage and senescence caused by metabolic competition. Nat. Commun. 9:249. doi: 10.1038/s41467-017-02689-5
Liu, Y., He, Y., Jin, A., Tikunov, A. P., Zhou, L., Tollini, L. A., et al. (2014). Ribosomal protein-Mdm2-p53 pathway coordinates nutrient stress with lipid metabolism by regulating MCD and promoting fatty acid oxidation. Proc. Natl. Acad. Sci. U.S.A. 111, E2414–E2422. doi: 10.1073/pnas.1315605111
Liu, Y., Sanoff, H. K., Cho, H., Burd, C. E., Torrice, C., Ibrahim, J. G., et al. (2009). Expression of p16(INK4a) in peripheral blood T-cells is a biomarker of human aging. Aging Cell 8, 439–448. doi: 10.1111/j.1474-9726.2009.00489.x
Lizardo, D. Y., Lin, Y. L., Gokcumen, O., and Atilla-Gokcumen, G. E. (2017). Regulation of lipids is central to replicative senescence. Mol. Biosyst. 13, 498–509. doi: 10.1039/c6mb00842a
Matoba, S., Kang, J. G., Patino, W. D., Wragg, A., Boehm, M., Gavrilova, O., et al. (2006). p53 regulates mitochondrial respiration. Science 312, 1650–1653.
McDonnell, E., Crown, S. B., Fox, D. B., Kitir, B., Ilkayeva, O. R., Olsen, C. A., et al. (2016). Lipids reprogram metabolism to become a major carbon source for histone acetylation. Cell Rep. 17, 1463–1472. doi: 10.1016/j.celrep.2016.10.012
Melk, A., Schmidt, B. M., Takeuchi, O., Sawitzki, B., Rayner, D. C., and Halloran, P. F. (2004). Expression of p16INK4a and other cell cycle regulator and senescence associated genes in aging human kidney. Kidney Int. 65, 510–520. doi: 10.1111/j.1523-1755.2004.00438.x
Meloni, F., Morosini, M., Solari, N., Passadore, I., Nascimbene, C., Novo, M., et al. (2006). Foxp3 expressing CD4+ CD25+ and CD8+CD28- T regulatory cells in the peripheral blood of patients with lung cancer and pleural mesothelioma. Hum. Immunol. 67, 1–12. doi: 10.1016/j.humimm.2005.11.005
Millner, A., and Atilla-Gokcumen, G. E. (2020). Lipid players of cellular senescence. Metabolites 10:339. doi: 10.3390/metabo10090339
Millner, A., Lizardo, D. Y., and Atilla-Gokcumen, G. E. (2020). Untargeted lipidomics highlight the depletion of deoxyceramides during therapy-induced senescence. Proteomics 20:e2000013. doi: 10.1002/pmic.202000013
Modrak, D. E., Leon, E., Goldenberg, D. M., and Gold, D. V. (2009). Ceramide regulates gemcitabine-induced senescence and apoptosis in human pancreatic cancer cell lines. Mol. Cancer Res. 7, 890–896. doi: 10.1158/1541-7786.MCR-08-0457
Molano, A., Huang, Z., Marko, M. G., Azzi, A., Wu, D., Wang, E., et al. (2012). Age-dependent changes in the sphingolipid composition of mouse CD4+ T cell membranes and immune synapses implicate glucosylceramides in age-related T cell dysfunction. PLoS One 7:e47650. doi: 10.1371/journal.pone.0047650
Moon, S. H., Huang, C. H., Houlihan, S. L., Regunath, K., Freed-Pastor, W. A., Morris, J. P. T., et al. (2019). p53 represses the mevalonate pathway to mediate tumor suppression. Cell 176, 564–580.e19. doi: 10.1016/j.cell.2018.11.011
Moreno-Fernandez, R. D., Tabbai, S., Castilla-Ortega, E., Perez-Martin, M., Estivill-Torrus, G., Rodriguez de Fonseca, F., et al. (2018). Stress, depression, resilience and ageing: a role for the LPA-LPA1 pathway. Curr. Neuropharmacol. 16, 271–283. doi: 10.2174/1570159X15666170710200352
Morisseau, C., and Hammock, B. D. (2013). Impact of soluble epoxide hydrolase and epoxyeicosanoids on human health. Annu. Rev. Pharmacol. Toxicol. 53, 37–58. doi: 10.1146/annurev-pharmtox-011112-140244
Munoz-Espin, D., Canamero, M., Maraver, A., Gomez-Lopez, G., Contreras, J., Murillo-Cuesta, S., et al. (2013). Programmed cell senescence during mammalian embryonic development. Cell 155, 1104–1118. doi: 10.1016/j.cell.2013.10.019
Mutlu, A. S., Duffy, J., and Wang, M. C. (2021). Lipid metabolism and lipid signals in aging and longevity. Dev. Cell 56, 1394–1407. doi: 10.1016/j.devcel.2021.03.034
Naaz, A., Holsberger, D. R., Iwamoto, G. A., Nelson, A., Kiyokawa, H., and Cooke, P. S. (2004). Loss of cyclin-dependent kinase inhibitors produces adipocyte hyperplasia and obesity. FASEB J. 18, 1925–1927. doi: 10.1096/fj.04-2631fje
Nacarelli, T., Lau, L., Fukumoto, T., Zundell, J., Fatkhutdinov, N., Wu, S., et al. (2019). NAD(+) metabolism governs the proinflammatory senescence-associated secretome. Nat. Cell Biol. 21, 397–407. doi: 10.1038/s41556-019-0287-4
Narzt, M. S., Pils, V., Kremslehner, C., Nagelreiter, I. M., Schosserer, M., Bessonova, E., et al. (2021). Epilipidomics of senescent dermal fibroblasts identify lysophosphatidylcholines as pleiotropic senescence-associated secretory phenotype (SASP) factors. J. Invest. Dermatol. 141, 993–1006.e15. doi: 10.1016/j.jid.2020.11.020
Neurohr, G. E., Terry, R. L., Lengefeld, J., Bonney, M., Brittingham, G. P., Moretto, F., et al. (2019). Excessive cell growth causes cytoplasm dilution and contributes to senescence. Cell 176, 1083–1097.e18. doi: 10.1016/j.cell.2019.01.018
Noda, A., Ning, Y., Venable, S. F., Pereira-Smith, O. M., and Smith, J. R. (1994). Cloning of senescent cell-derived inhibitors of DNA synthesis using an expression screen. Exp. Cell Res. 211, 90–98. doi: 10.1006/excr.1994.1063
Node, K., Huo, Y., Ruan, X., Yang, B., Spiecker, M., Ley, K., et al. (1999). Anti-inflammatory properties of cytochrome P450 epoxygenase-derived eicosanoids. Science 285, 1276–1279. doi: 10.1126/science.285.5431.1276
Nurminen, T. A., Holopainen, J. M., Zhao, H., and Kinnunen, P. K. (2002). Observation of topical catalysis by sphingomyelinase coupled to microspheres. J. Am. Chem. Soc. 124, 12129–12134. doi: 10.1021/ja017807r
Ogrodnik, M., Salmonowicz, H., Brown, R., Turkowska, J., Sredniawa, W., Pattabiraman, S., et al. (2014). Dynamic JUNQ inclusion bodies are asymmetrically inherited in mammalian cell lines through the asymmetric partitioning of vimentin. Proc. Natl. Acad. Sci. U.S.A. 111, 8049–8054. doi: 10.1073/pnas.1324035111
Ogrodnik, M., Zhu, Y., Langhi, L. G. P., Tchkonia, T., Kruger, P., Fielder, E., et al. (2019). Obesity-induced cellular senescence drives anxiety and impairs neurogenesis. Cell Metab. 29, 1061–1077.e8.
Ojala, P. J., Hirvonen, T. E., Hermansson, M., Somerharju, P., and Parkkinen, J. (2007). Acyl chain-dependent effect of lysophosphatidylcholine on human neutrophils. J. Leukoc. Biol. 82, 1501–1509. doi: 10.1189/jlb.0507292
Okamura, S., Ng, C. C., Koyama, K., Takei, Y., Arakawa, H., Monden, M., et al. (1999). Identification of seven genes regulated by wild-type p53 in a colon cancer cell line carrying a well-controlled wild-type p53 expression system. Oncol. Res. 11, 281–285.
Oskouian, B., Sooriyakumaran, P., Borowsky, A. D., Crans, A., Dillard-Telm, L., Tam, Y. Y., et al. (2006). Sphingosine-1-phosphate lyase potentiates apoptosis via p53- and p38-dependent pathways and is down-regulated in colon cancer. Proc. Natl. Acad. Sci. U.S.A. 103, 17384–17389. doi: 10.1073/pnas.0600050103
Ozcan, S., Alessio, N., Acar, M. B., Mert, E., Omerli, F., Peluso, G., et al. (2016). Unbiased analysis of senescence associated secretory phenotype (SASP) to identify common components following different genotoxic stresses. Aging (Albany NY) 8, 1316–1329. doi: 10.18632/aging.100971
Pack, L. R., Daigh, L. H., and Meyer, T. (2019). Putting the brakes on the cell cycle: mechanisms of cellular growth arrest. Curr. Opin. Cell Biol. 60, 106–113. doi: 10.1016/j.ceb.2019.05.005
Palmieri, M., Almeida, M., Nookaew, I., Gomez-Acevedo, H., Joseph, T. E., Que, X., et al. (2021). Neutralization of oxidized phospholipids attenuates age-associated bone loss in mice. Aging Cell 20:e13442. doi: 10.1111/acel.13442
Papsdorf, K., and Brunet, A. (2019). Linking lipid metabolism to chromatin regulation in aging. Trends Cell. Biol. 29, 97–116. doi: 10.1016/j.tcb.2018.09.004
Pasquare, S. J., Gaveglio, V. L., and Giusto, N. M. (2009). Age-related changes in the metabolization of phosphatidic acid in rat cerebral cortex synaptosomes. Arch. Biochem. Biophys. 488, 121–129. doi: 10.1016/j.abb.2009.07.002
Pavletich, N. P. (1999). Mechanisms of cyclin-dependent kinase regulation: structures of Cdks, their cyclin activators, and Cip and INK4 inhibitors. J. Mol. Biol. 287, 821–828. doi: 10.1006/jmbi.1999.2640
Pincus, Z., Mazer, T. C., and Slack, F. J. (2016). Autofluorescence as a measure of senescence in C. elegans: look to red, not blue or green. Aging (Albany NY) 8, 889–898. doi: 10.18632/aging.100936
Podrez, E. A., Poliakov, E., Shen, Z., Zhang, R., Deng, Y., Sun, M., et al. (2002). A novel family of atherogenic oxidized phospholipids promotes macrophage foam cell formation via the scavenger receptor CD36 and is enriched in atherosclerotic lesions. J. Biol. Chem. 277, 38517–38523. doi: 10.1074/jbc.M205924200
Quehenberger, O., Armando, A. M., Brown, A. H., Milne, S. B., Myers, D. S., Merrill, A. H., et al. (2010). Lipidomics reveals a remarkable diversity of lipids in human plasma. J. Lipid Res. 51, 3299–3305.
Quijano, C., Cao, L., Fergusson, M. M., Romero, H., Liu, J., Gutkind, S., et al. (2012). Oncogene-induced senescence results in marked metabolic and bioenergetic alterations. Cell Cycle 11, 1383–1392. doi: 10.4161/cc.19800
Rajagopalan, S., and Long, E. O. (2012). Cellular senescence induced by CD158d reprograms natural killer cells to promote vascular remodeling. Proc. Natl. Acad. Sci. U.S.A. 109, 20596–20601. doi: 10.1073/pnas.1208248109
Rao, R. P., Yuan, C., Allegood, J. C., Rawat, S. S., Edwards, M. B., Wang, X., et al. (2007). Ceramide transfer protein function is essential for normal oxidative stress response and lifespan. Proc. Natl. Acad. Sci. U.S.A. 104, 11364–11369. doi: 10.1073/pnas.0705049104
Rivera, R., and Chun, J. (2008). Biological effects of lysophospholipids. Rev. Physiol. Biochem. Pharmacol. 160, 25–46. doi: 10.1007/112_0507
Rubinstein, M., and Dvash, E. (2018). Leukotrienes and kidney diseases. Curr. Opin. Nephrol. Hypertens. 27, 42–48. doi: 10.1097/MNH.0000000000000381
Sacket, S. J., Chung, H. Y., Okajima, F., and Im, D. S. (2009). Increase in sphingolipid catabolic enzyme activity during aging. Acta Pharmacol. Sin. 30, 1454–1461. doi: 10.1038/aps.2009.136
Sagiv, A., Bar-Shai, A., Levi, N., Hatzav, M., Zada, L., Ovadya, Y., et al. (2018). p53 in Bronchial club cells facilitates chronic lung inflammation by promoting senescence. Cell Rep. 22, 3468–3479. doi: 10.1016/j.celrep.2018.03.009
Saitou, M., Lizardo, D. Y., Taskent, R. O., Millner, A., Gokcumen, O., and Atilla-Gokcumen, G. E. (2018). An evolutionary transcriptomics approach links CD36 to membrane remodeling in replicative senescence. Mol. Omics 14, 237–246. doi: 10.1039/c8mo00099a
Sakai, T., Imai, J., Takagaki, H., Ui, M., and Hatta, S. (2019). Cytoplasmic OH scavenger TA293 attenuates cellular senescence and fibrosis by activating macrophages through oxidized phospholipids/TLR4. Life Sci. 221, 284–292. doi: 10.1016/j.lfs.2019.02.038
Salama, R., Sadaie, M., Hoare, M., and Narita, M. (2014). Cellular senescence and its effector programs. Genes Dev. 28, 99–114. doi: 10.1101/gad.235184.113
Sanchez-Macedo, N., Feng, J., Faubert, B., Chang, N., Elia, A., Rushing, E. J., et al. (2013). Depletion of the novel p53-target gene carnitine palmitoyltransferase 1C delays tumor growth in the neurofibromatosis type I tumor model. Cell Death Differ. 20, 659–668. doi: 10.1038/cdd.2012.168
Sander, A. L., Sommer, K., Neumayer, T., Fleming, I., Marzi, I., Barker, J. H., et al. (2013). Soluble epoxide hydrolase disruption as therapeutic target for wound healing. J. Surg. Res. 182, 362–367. doi: 10.1016/j.jss.2012.10.034
Schafer, M. J., White, T. A., Iijima, K., Haak, A. J., Ligresti, G., Atkinson, E. J., et al. (2017). Cellular senescence mediates fibrotic pulmonary disease. Nat. Commun. 8:14532. doi: 10.1038/ncomms14532
Sen, N., Satija, Y. K., and Das, S. (2011). PGC-1alpha, a key modulator of p53, promotes cell survival upon metabolic stress. Mol. Cell 44, 621–634. doi: 10.1016/j.molcel.2011.08.044
Serrano, M., Lin, A. W., McCurrach, M. E., Beach, D., and Lowe, S. W. (1997). Oncogenic ras provokes premature cell senescence associated with accumulation of p53 and p16INK4a. Cell 88, 593–602. doi: 10.1016/s0092-8674(00)81902-9
Sharpless, N. E., and Depinho, R. A. (2006). The mighty mouse: genetically engineered mouse models in cancer drug development. Nat. Rev. Drug Discov. 5, 741–754. doi: 10.1038/nrd2110
Sharpless, N. E., and Sherr, C. J. (2015). Forging a signature of in vivo senescence. Nat. Rev. Cancer 15, 397–408. doi: 10.1038/nrc3960
Shimizu, R., Kanno, K., Sugiyama, A., Ohata, H., Araki, A., Kishikawa, N., et al. (2015). Cholangiocyte senescence caused by lysophosphatidylcholine as a potential implication in carcinogenesis. J. Hepatobiliary Pancreat. Sci. 22, 675–682. doi: 10.1002/jhbp.256
Skotland, T., Hessvik, N. P., Sandvig, K., and Llorente, A. (2019). Exosomal lipid composition and the role of ether lipids and phosphoinositides in exosome biology. J. Lipid Res. 60, 9–18. doi: 10.1194/jlr.R084343
Sommer, K., Jakob, H., Badjlan, F., Henrich, D., Frank, J., Marzi, I., et al. (2019). 11,12 and 14,15 epoxyeicosatrienoic acid rescue deteriorated wound healing in ischemia. PLoS One 14:e0209158. doi: 10.1371/journal.pone.0209158
Song, W., Zhang, Y., Wang, J., Ma, T., Hao, L., and Wang, K. (2018). Antagonism of cysteinyl leukotriene receptor 1 (cysLTR1) by montelukast suppresses cell senescence of chondrocytes. Cytokine 103, 83–89. doi: 10.1016/j.cyto.2017.12.021
Stein, G. H., Drullinger, L. F., Soulard, A., and Dulic, V. (1999). Differential roles for cyclin-dependent kinase inhibitors p21 and p16 in the mechanisms of senescence and differentiation in human fibroblasts. Mol. Cell Biol. 19, 2109–2117. doi: 10.1128/MCB.19.3.2109
Storer, M., Mas, A., Robert-Moreno, A., Pecoraro, M., Ortells, M. C., Di Giacomo, V., et al. (2013). Senescence is a developmental mechanism that contributes to embryonic growth and patterning. Cell 155, 1119–1130.
Sun, X., Seidman, J. S., Zhao, P., Troutman, T. D., Spann, N. J., Que, X., et al. (2020). Neutralization of oxidized phospholipids ameliorates non-alcoholic steatohepatitis. Cell Metab. 31, 189–206.e8. doi: 10.1016/j.cmet.2019.10.014
Surowiak, P., Gansukh, T., Donizy, P., Halon, A., and Rybak, Z. (2014). Increase in cyclooxygenase-2 (COX-2) expression in keratinocytes and dermal fibroblasts in photoaged skin. J. Cosmet. Dermatol. 13, 195–201. doi: 10.1111/jocd.12103
Takahashi, A., Okada, R., Nagao, K., Kawamata, Y., Hanyu, A., Yoshimoto, S., et al. (2017). Exosomes maintain cellular homeostasis by excreting harmful DNA from cells. Nat. Commun. 8:15287.
Tepper, A. D., Ruurs, P., Wiedmer, T., Sims, P. J., Borst, J., and van Blitterswijk, W. J. (2000). Sphingomyelin hydrolysis to ceramide during the execution phase of apoptosis results from phospholipid scrambling and alters cell-surface morphology. J. Cell Biol. 150, 155–164. doi: 10.1083/jcb.150.1.155
Trajkovic, K., Hsu, C., Chiantia, S., Rajendran, L., Wenzel, D., Wieland, F., et al. (2008). Ceramide triggers budding of exosome vesicles into multivesicular endosomes. Science 319, 1244–1247. doi: 10.1126/science.1153124
Tung, B. T., Rodriguez-Bies, E., Talero, E., Gamero-Estevez, E., Motilva, V., Navas, P., et al. (2015). Anti-inflammatory effect of resveratrol in old mice liver. Exp. Gerontol. 64, 1–7. doi: 10.1016/j.exger.2015.02.004
Urbanelli, L., Buratta, S., Sagini, K., Tancini, B., and Emiliani, C. (2016). Extracellular vesicles as new players in cellular senescence. Int. J. Mol. Sci. 17:1408. doi: 10.3390/ijms17091408
Urbaniak-Kujda, D., Kapelko-Slowik, K., Wolowiec, D., Dybko, J., Halon, A., Jazwiec, B., et al. (2009). Increased percentage of CD8+CD28- suppressor lymphocytes in peripheral blood and skin infiltrates correlates with advanced disease in patients with cutaneous T-cell lymphomas. Postepy Hig. Med. Dosw. (Online) 63, 355–359.
Venable, M. E., Lee, J. Y., Smyth, M. J., Bielawska, A., and Obeid, L. M. (1995). Role of ceramide in cellular senescence. J. Biol. Chem. 270, 30701–30708. doi: 10.1074/jbc.270.51.30701
Vizioli, M. G., Liu, T., Miller, K. N., Robertson, N. A., Gilroy, K., Lagnado, A. B., et al. (2020). Mitochondria-to-nucleus retrograde signaling drives formation of cytoplasmic chromatin and inflammation in senescence. Genes Dev. 34, 428–445. doi: 10.1101/gad.331272.119
Vousden, K. H., and Prives, C. (2009). Blinded by the light: the growing complexity of p53. Cell 137, 413–431. doi: 10.1016/j.cell.2009.04.037
Waga, S., Hannon, G. J., Beach, D., and Stillman, B. (1994). The p21 inhibitor of cyclin-dependent kinases controls DNA replication by interaction with PCNA. Nature 369, 574–578. doi: 10.1038/369574a0
Wang, M., Wang, C., Han, R. H., and Han, X. (2016). Novel advances in shotgun lipidomics for biology and medicine. Prog. Lipid Res. 61, 83–108. doi: 10.1016/j.plipres.2015.12.002
Wang, S. J., Li, D., Ou, Y., Jiang, L., Chen, Y., Zhao, Y., et al. (2016). Acetylation is crucial for p53-mediated ferroptosis and tumor suppression. Cell Rep. 17, 366–373. doi: 10.1016/j.celrep.2016.09.022
Wennberg, A. M. V., Schafer, M. J., LeBrasseur, N. K., Savica, R., Bui, H. H., Hagen, C. E., et al. (2018). Plasma sphingolipids are associated with gait parameters in the mayo clinic study of aging. J. Gerontol. A Biol. Sci. Med. Sci. 73, 960–965. doi: 10.1093/gerona/glx139
Wiley, C. D. (2020). Role of senescent renal cells in pathophysiology of diabetic kidney disease. Curr. Diab. Rep. 20:33. doi: 10.1007/s11892-020-01314-y
Wiley, C. D., Brumwell, A. N., Davis, S. S., Jackson, J. R., Valdovinos, A., Calhoun, C., et al. (2019). Secretion of leukotrienes by senescent lung fibroblasts promotes pulmonary fibrosis. JCI Insight 4:e130056. doi: 10.1172/jci.insight.130056
Wiley, C. D., Sharma, R., Davis, S. S., Lopez-Dominguez, J. A., Mitchell, K. P., Wiley, S., et al. (2021). Oxylipin biosynthesis reinforces cellular senescence and allows detection of senolysis. Cell Metab. 33, 1124–1136.e5. doi: 10.1016/j.cmet.2021.03.008
Wong, M. W. K., Braidy, N., Pickford, R., Vafaee, F., Crawford, J., Muenchhoff, J., et al. (2019). Plasma lipidome variation during the second half of the human lifespan is associated with age and sex but minimally with BMI. PLoS One 14:e0214141. doi: 10.1371/journal.pone.0214141
Yahagi, N., Shimano, H., Matsuzaka, T., Najima, Y., Sekiya, M., Nakagawa, Y., et al. (2003). p53 Activation in adipocytes of obese mice. J. Biol. Chem. 278, 25395–25400. doi: 10.1074/jbc.M302364200
Yamakoshi, K., Takahashi, A., Hirota, F., Nakayama, R., Ishimaru, N., Kubo, Y., et al. (2009). Real-time in vivo imaging of p16Ink4a reveals cross talk with p53. J. Cell Biol. 186, 393–407. doi: 10.1083/jcb.200904105
Yang, C., Pan, S., Yan, S., Li, Z., Yang, J., Wang, Y., et al. (2014). Inhibitory effect of 14,15-EET on endothelial senescence through activation of mTOR complex 2/Akt signaling pathways. Int. J. Biochem. Cell Biol. 50, 93–100. doi: 10.1016/j.biocel.2014.02.020
Yi, J. K., Xu, R., Jeong, E., Mileva, I., Truman, J. P., Lin, C. L., et al. (2016). Aging-related elevation of sphingoid bases shortens yeast chronological life span by compromising mitochondrial function. Oncotarget 7, 21124–21144. doi: 10.18632/oncotarget.8195
Yosef, R., Pilpel, N., Tokarsky-Amiel, R., Biran, A., Ovadya, Y., Cohen, S., et al. (2016). Directed elimination of senescent cells by inhibition of BCL-W and BCL-XL. Nat Commun. 7:11190. doi: 10.1038/ncomms11190
Yousefzadeh, M. J., Flores, R. R., Zhu, Y., Schmiechen, Z. C., Brooks, R. W., Trussoni, C. E., et al. (2021). An aged immune system drives senescence and ageing of solid organs. Nature 594, 100–105. doi: 10.1038/s41586-021-03547-7
Zhang, M., Du, Y., Lu, R., Shu, Y., Zhao, W., Li, Z., et al. (2016). Cholesterol retards senescence in bone marrow mesenchymal stem cells by modulating autophagy and ROS/p53/p21(Cip1/Waf1) pathway. Oxid. Med. Cell Longev. 2016:7524308. doi: 10.1155/2016/7524308
Zhang, P., Kishimoto, Y., Grammatikakis, I., Gottimukkala, K., Cutler, R. G., Zhang, S., et al. (2019). Senolytic therapy alleviates Abeta-associated oligodendrocyte progenitor cell senescence and cognitive deficits in an Alzheimer’s disease model. Nat. Neurosci. 22, 719–728. doi: 10.1038/s41593-019-0372-9
Zhang, W., Koerner, I. P., Noppens, R., Grafe, M., Tsai, H. J., Morisseau, C., et al. (2007). Soluble epoxide hydrolase: a novel therapeutic target in stroke. J. Cereb. Blood Flow Metab. 27, 1931–1940. doi: 10.1038/sj.jcbfm.9600494
Zhang, X., Xu, G. B., Zhou, D., and Pan, Y. X. (2018). High-fat diet modifies expression of hepatic cellular senescence gene p16(INK4a) through chromatin modifications in adult male rats. Genes Nutr. 13:6. doi: 10.1186/s12263-018-0595-5
Zhao, Y., Shao, Q., and Peng, G. (2020). Exhaustion and senescence: two crucial dysfunctional states of T cells in the tumor microenvironment. Cell Mol. Immunol. 17, 27–35. doi: 10.1038/s41423-019-0344-8
Zhu, Y., Tchkonia, T., Pirtskhalava, T., Gower, A. C., Ding, H., Giorgadze, N., et al. (2015). The Achilles’ heel of senescent cells: from transcriptome to senolytic drugs. Aging Cell 14, 644–658. doi: 10.1111/acel.12344
Keywords: cellular senescence, lipids, aging, bioactive lipids, senolytics
Citation: Hamsanathan S and Gurkar AU (2022) Lipids as Regulators of Cellular Senescence. Front. Physiol. 13:796850. doi: 10.3389/fphys.2022.796850
Received: 17 October 2021; Accepted: 03 January 2022;
Published: 04 March 2022.
Edited by:
Roberto Angelini, Swansea University Medical School, United KingdomReviewed by:
Anthony Donato, The University of Utah, United StatesGunes Ekin Atilla-Gokcumen, University at Buffalo, United States
Copyright © 2022 Hamsanathan and Gurkar. This is an open-access article distributed under the terms of the Creative Commons Attribution License (CC BY). The use, distribution or reproduction in other forums is permitted, provided the original author(s) and the copyright owner(s) are credited and that the original publication in this journal is cited, in accordance with accepted academic practice. No use, distribution or reproduction is permitted which does not comply with these terms.
*Correspondence: Aditi U. Gurkar, YWd1cmthcjFAcGl0dC5lZHU=