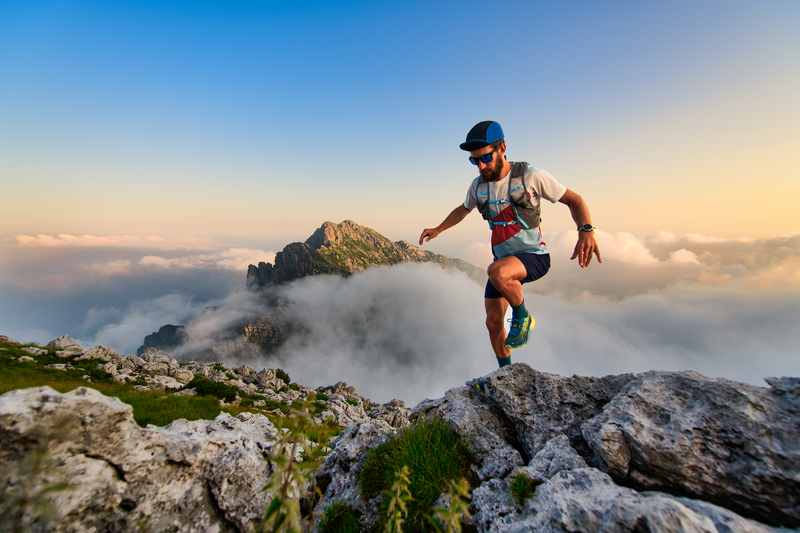
95% of researchers rate our articles as excellent or good
Learn more about the work of our research integrity team to safeguard the quality of each article we publish.
Find out more
ORIGINAL RESEARCH article
Front. Physiol. , 15 December 2022
Sec. Avian Physiology
Volume 13 - 2022 | https://doi.org/10.3389/fphys.2022.1057810
This article is part of the Research Topic Avian Microbiome: from embryonic development to adulthood View all 10 articles
The microbiome is an integral part of chicken health and can affect immunity, nutrient utilization, and performance. The role of bacterial microbiota members in host health is relatively well established, but less attention has been paid to fungal members of the gastrointestinal tract (GIT) community. However, human studies indicate that fungi play a critical role in health. Here, we described fungal communities, or mycobiomes, in both the lumen and mucosa of the chicken ileum and cecum from hatch through 14 days of age. We also assessed the effects of delayed access to feed immediately post-hatch (PH) on mycobiome composition, as PH feed delay is commonly associated with poor health performance. Chicken mycobiomes in each of the populations were distinct and changed over time. All mycobiomes were dominated by Gibberella, but Aspergillus, Cladosporium, Sarocladium, Meyerozyma, and Penicillium were also abundant. Relative abundances of some taxa differed significantly over time. In the cecal and ileal lumens, Penicillium was present in extremely low quantities or absent during days one and two and then increased over time. Meyerozyma and Wickerhamomyces also increased over time in luminal sites. In contrast, several highly abundant unclassified fungi decreased after days one and two, highlighting the need for improved understanding of fungal gut biology. Mycobiomes from chicks fed during the first 2 days PH versus those not fed during the first 2 days did not significantly differ, except during days one and two. Similarities observed among mycobiomes of fed and unfed chicks at later timepoints suggest that delays in PH feeding do not have long lasting effects on mycobiome composition. Together, these results provide a foundation for future mycobiome studies, and suggest that negative health and production impacts of delayed feeding are not likely related to the development of fungal populations in the GIT.
The gastrointestinal (GI) microbiome is a complex and diverse group of microorganisms including bacteria, archaea, fungi, viruses, and protists. The bacterial members (bacteriome) are the most abundant microbial group in the microbiome and have been investigated in detail through 16S-based sequencing. The bacteriome has been shown to play a critical role in host health through its role in nutrition, immune system development, metabolism, and pathogen control (Chambers and Gong, 2011; Oakley et al., 2014; Pan and Yu, 2014; Stanley et al., 2014; Clavijo and Flórez, 2018). The fungal members (mycobiome) are considered part of the “rare biosphere” based on their numerical inferiority in the gut microbiome (Huffnagle and Noverr, 2013). Investigations of the mycobiome through high-throughput sequencing technologies have lagged behind bacteriome studies due to difficulties in isolating DNA from fungal cells, primer design complexities, and database inaccuracies and missing data (Huffnagle and Noverr, 2013; Arfken et al., 2022). However, recent progress in the mycobiome field demonstrates that fungi play a vital role in host health through fungal-bacterial interactions and fungal-host interactions (Mason Katie et al., 2012; Iliev and Leonardi, 2017; Sam et al., 2017; Tso et al., 2018; Leonardi et al., 2022).
Interactions in the gut are complex and often mediated by diverse metabolites released by both the microbes and the host. These metabolic interactions are vital in biological processes including digestion and health (Iliev and Leonardi, 2017; Mims et al., 2021; dos Santos et al., 2020; Kim et al., 2017; Banani et al., 2016; Curbete and Salgado, 2016; Hallen-Adams and Suhr, 2017). Data on the mycobiome in poultry health is limited and most studies have been culture-based with limited organs investigated to date (Shokri et al., 2011; Hume et al., 2012; Byrd et al., 2017; Sokół et al., 2018; Cafarchia et al., 2019). Recently, the GI tract of the chicken was investigated with ITS2-based Illumina sequencing and temporal and spatial changes in the mycobiota were demonstrated (Robinson et al., 2022). The dominant fungal taxa identified in the GI tract was Fusarium pseudonygamai regardless of age of broiler chickens (Robinson et al., 2022). Given the potential impact of the gut fungal communities on chicken health, it is important to investigate the mycobiome as a target of dietary manipulation to enhance animal performance and disease resistance.
In commercial broiler production, newly hatched chicks are often deprived of feed for up to 72 h (Careghi et al., 2005; Mitchell, 2009; van de Ven et al., 2009; Willemsen et al., 2010; de Jong et al., 2017) due variable hatch times (24–48 h), sexing and sorting, vaccination, and transport from the hatchery to farms (Careghi et al., 2005). This post-hatch (PH) delay in access to feed is associated with poor health performance including reduced weight and growth rate (Bigot et al., 2003; Careghi et al., 2005), altered GI development (de Jong et al., 2017), and decreased nutrient utilization (Shinde Tamboli et al., 2018) and breast muscle development (Bigot et al., 2003; Powell et al., 2016). We showed previously that PH delay induces changes in some microbial gut taxa (Proszkowiec-Weglarz et al., 2022), as well as changes in the expression of genes involved in lipogenesis (Richards et al., 2010), cecal development (Qu et al., 2021), calcium and potassium transporters (Proszkowiec-Weglarz et al., 2019), carbohydrate and amino acid utilization (Payne et al., 2019), and the functioning of small intestine gut barrier and tight junction related genes (Proszkowiec-Weglarz et al., 2020). Here, we investigated the cecal and ileal mycobiomes in newly hatched chicks with or without delayed access to feed through 14 days of age.
All animal experiments were approved by the USDA-ARS-BARC Institutional Animal Care and Use Committee. Samples in these studies were obtained concurrently with a previously published study (Proszkowiec-Weglarz et al., 2022) and all animal and experimental procedures were performed as in that study. In brief, 250 fertile Ross 708 broiler chicken eggs were acquired from Perdue Hatchery (Hurlock, MD) and incubated as described previously at USDA-ARS (Proszkowiec-Weglarz et al., 2019; Proszkowiec-Weglarz et al., 2020). Birds were hatched during a 486–496 h window of incubation. Three batches of hatchlings were removed within 180–240 min of occlusion, and randomly assigned among experimental groups (Table 1) such that each battery pen included birds from each batch (14–15 hatchlings per battery pen total). Battery-brooders were heated and equipped with two nipple drinkers and one feeder. Gender of chicks was determined at sampling time and equal proportions of male and female chicks were included in the study. In order to mimic PH feed delay in commercial hatchery operations, hatchlings were randomly divided into two treatment groups (n = 6 battery pens for each treatment), the “fed” group, which received feed immediately upon entry to the pen, and the “unfed” group which did not receive feed during the first 48 h. After 48 h, both groups have equal access to feed. Feed was a commercial type of corn-soybean meal-based starter diet (Proszkowiec-Weglarz et al., 2019; Proszkowiec-Weglarz et al., 2020).
Birds were sampled at days 1 (24 h), 2 (48 h), 3 (72 h), 4 (96 h), 6 (144 h), 8 (192 h), 10 (240 h), 12 (288 h) and 14 (336 h) after the start of feeding. Sampling times were based on prior data (Richards et al., 2010) and adjusted to obtain a comprehensive coverage of the 2 week period PH. Starting at 24 h PH, one chick per pen was randomly selected and sacrificed by cervical dislocation. Organ contents and epithelial scrapings were collected from the ileum (from Meckel’s diverticulum to ileocecal junction) and the middle of the ceca to represent luminal (L) and mucosal (M) samples, respectively. Samples were snap-frozen in liquid nitrogen and stored at −80°C until fungal DNA isolation. Samples from a single experimental group, day 2-unfed-ileum lumen, were removed from all statistical analyses due to insufficient sample size (n = 1).
DNA was extracted from 374 samples as previously described (Arfken et al., 2020). Briefly, DNA was extracted from the ileum and cecum (200 mg of content or 100 mg of scrapings) with the DNeasy PowerSoil kit (Qiagen, Valencia, CA) utilizing a QIAcube instrument (Qiagen) according to the manufacturer’s protocol. DNA concentration and quality were assessed by NanoDrop (ThermoFisher Scientific, Waltsham, MA) and a Tapestation System (Agilent Technologies, Santa Clara, CA, respectively. The ITS region was sequenced utilizing primers ITS3 (5′ GCATCGATGAAGAACGCAGC 3′) and ITS4 (5′ TCCTCCGCTTATTGATATGC 3′) with the Illumina adaptor sequence added to the 5′ end. ITS regions were sequenced with the Illumina MiSeq Sequencing platform, generating 300 bp paired-end reads, respectively.
Sequences with an average quality score less than Q15 across four bases or more were removed using the sliding window option in Trimmomatic 0.38 (Bolger et al., 2014). Reads were then imported into QIIME2 version 2021.11 for further analysis. Cutadapt was used to remove forward and reverse primers from paired reads (Martin, 2011). Amplicon sequence variants (ASVs) were identified using the dada2 plug-in. The QIIME2 formatted UNITE fungal ITS database version 8.3 (clustered at 99%) (Kõljalg et al., 2013) was downloaded and imported into QIIME2. Taxonomic classifications were assigned to ASVs using a naïve bayes classifier trained on the UNITE database. Rarefaction curves were plotted in QIIME2, and a threshold of 10,000 reads was selected as the minimum sequencing depth for each sample. Alpha diversity metrics including the number of ASVs, Pielou’s Evenness, and the Shannon index were calculated within QIIME2 on rarefied data.
Statistical calculations were performed in R (R Core Team, 2022). Feature tables and alpha diversity values were exported from QIIME2 and imported into R using the package qiime2R. Alpha diversity metrics were tested for normality and homogeneity of variances using the Shapiro-Wilke test implemented using the shapiro.test function within the stats package (R Core Team, 2022) and Levene’s Test implemented using the levene Test function in the car package (Fox and Weisberg, 2019). Data were transformed using the Box-Cox transformation, function boxcox, within the package MASS (Venables and Ripley, 2002) when data were not normally distributed. Alpha diversity metrics were compared across time (days 1–14), treatment (fed versus unfed), and site (cecal lumen, cecal mucosa, ileal lumen, ileal mucosa), as well as across all interactions (time x treatment, time x site, site x treatment, and time x treatment x site) using the aov function within the stats package (R Core Team, 2022). Posthoc pairwise testing was performed using the TukeyHSD function within the stats package (R Core Team, 2022).
Multiple functions in vegan (Oksanen et al., 2022) were used to interrogate changes in beta-diversity across samples with respect to time, treatment, and site, as well as interactions between the factors as defined above. Bray-Curtis dissimilarity matrices were constructed using vegdist (Oksanen et al., 2022) on rarefied data, and principle coordinates analysis (PCoA) was performed on Bray Curtis matrices using the cmdscale function in the stats package (R Core Team, 2022). The adonis (Oksanen et al., 2022) function in conjunction with adonis.pair (package: EcolUtils (Salazar, 2022)) were used to test for differences among group centroids, and betadisper (Oksanen et al., 2022) and permutest (Oksanen et al., 2022) were used to test for homogeneity of multivariate dispersions across time, treatment, and site.
Changes in relative abundances within each site and across time (“early”, days 1–4, and “late”, days 6–14) and treatment were assessed using Maaslin2 with default parameters (Mallick et al., 2021). In brief, data were log transformed, normalized using total sum scaling (TSS), standardized using the z-score, and modeled with a linear model. Taxa were assumed to be differentially abundant if q-values <0.2, as recommended by the software. ASVs present in less than 1% of samples were removed prior to analysis to minimize spurious results. All data in the manuscript are plotted with ggplot2 (Wickham, 2016).
Whether a chick was fed or not during the first 2 days PH did not have a large effect on evenness, richness (as measured by the number of ASVs), or the Shannon index (Supplementary Figures S1–S3). The main effects for treatment (fed vs. unfed) were significant across all three metrics (Table 2, Shannon: p = 0.0274, Evenness: p = 0.0009, # ASVs: p = 0.0306), and treatment x site was significant in the number of ASVs (Table 2, p = 0.0117). However, only a single pairwise comparison within a site was significant; a greater number of ASVs were detected in the ileal lumen in fed than unfed chicks (p = 0.0140) (Figure 1). Evenness differed among fed and unfed chicks during days 1 and 2 but this was not statistically significant (Supplementary Figure S2).
FIGURE 1. Boxplot of the number of ASVs observed across all four sites (CE_L = cecal lumen, CE_M = cecal mucosa, IL_L = ileal lumen, IL_M = ileal mucosa) in fed and unfed chicks. The number of ASVs was not significantly different at any site except for the ileal lumen, where a greater number of ASVs was observed in fed compared to unfed chicks. Box represents the interquartile range, with the lower edge of box = 25% percentile, upper edge of box = 75% percentile, the midline = the median, and upper whisker = the largest value within 1.5 times the interquartile range above 75% percentile, lower whisker = the largest value within 1.5 times the interquartile range below 25% percentile. Dots represent outliers. Means not sharing any letter are significantly different by the Tukey-test, alpha = 0.05.
The number of ASVs, Shannon Index, and evenness differed significantly across time, but only in the cecum. In the cecal lumen, significantly fewer ASVs were identified during days 1–4 than in days 8–14 (Supplementary Figure S4; Supplementary Table S1). In the cecal mucosa and lumen, evenness tended to decrease from days 1–8, and then increase from days 8–14. However, in pairwise calculations, in the cecal lumen only day 1 was significantly different than day 8, and in the cecal mucosa, days 1–4 were significantly greater than day 8, and day 1 was greater than day 10 (Supplementary Figure S5; Supplementary Table S2). Shannon diversity showed a similar trend to evenness in the cecal mucosa, with days 1–3 significantly greater than day 8 (Supplementary Figure S6; Supplementary Table S3). All three alpha diversity metrics were significantly different across sites (Shannon: p < 2 × 10–16, Evenness: p < 2 × 10–16, # ASVs: p = 8.2 × 10–7), with evenness, richness, and Shannon higher in the mucosal communities than the luminal ones (Figure 2).
FIGURE 2. Boxplots of all the main effects of all three alpha diversity metrics across sites. Alpha diversity is greater in luminal (CE_L, IL_L) than mucosal (IL_M, IL_M) samples. Plot definitions as in Figure 1.
Overall community structure differed across time (p = 0.001), site (p = 0.001), site x time (p = 0.001), site x treatment (p = 0.005), and site x time x treatment (p = 0.009) (number of permutations = 999). Overall community structure was not significantly different among fed and unfed chicks within each organ (Figure 3), except during days 1 and 2 where fed and unfed communities in the cecum lumen and day 1 in the ileal lumen clustered independently (Supplementary Figures S7, S8). This pattern was not observed in the cecal and ileal mucosa (Supplementary Figures S9, S10). In contrast, communities differed significantly from each other across all sites (p = 0.001, Figure 4). Similarly, differences were observed across time (Figure 5, p = 0.001). In the cecal lumen, most days were significantly different than all other days, while in the cecal mucosa, days 1–2 differed from all other days and days 3–4 differed from a few other days (Supplementary Table S4). In the ileal lumen, the majority of differences occurred between day 1 and all other days, while in the ileal mucosa, days 1 and 2 differed from all others (Supplementary Table S4).
FIGURE 3. Comparison of beta-diversities. Principal coordinates analysis of Bray-Curtis matrices of fed and unfed samples at each site. Community structure was not significantly different among fed and unfed chicks at any site.
FIGURE 4. Comparison of beta-diversities. Principal coordinates analysis of Bray-Curtis matrices at each site. Community structure at all sites differed from all other sites.
FIGURE 5. Comparison of beta-diversities. Principal coordinates analysis of Bray-Curtis matrices of each day within each site. Community structures significantly differed across time.
All communities were dominated by Gibberella (63% in cecum, 38% in ileum) and unidentified Fungi (12% in cecum, 46% in ileum), but additional genera Aspergillus, Penicillium, Sarocladium, Cladosporium, and Meyerozyma were also abundant among all samples (Figure 6). At each site, we compared relative abundances of genera between days 1–4 (“early”) and days 6–14 (“late”). In the cecal and ileal lumen, a greater number of genera (19 and 17, respectively) were detected as differentially abundant over time compared to the mucosal samples, where the relative abundances of only three genera changed in the cecal mucosa. No differentially abundant taxa were found in the ileal mucosa (Table 3). In the cecal lumen, 18 genera including Wallemia, Meyerozyma, Penicillium, and Pyxidiophora (adjusted p-values = 0.002, 0.021, 0.022, 0.045, respectively) increased in relative abundance over time (Figure 7), and unknown genera decreased (Table 3). In the ileal lumen, Coniochaeta levels decreased over time while that of 16 genera increased including Clavispora, Suhomyces, Dipodascus, and Fusarium. In the cecal mucosa, Gibberella increased over time while unknown genera decreased. Few taxa were observed to be differentially abundant in fed versus unfed chicks. However, unknown genera were more abundant in the fed than in the unfed samples in both the cecal lumen and mucosa.
FIGURE 6. Barplot of average relative abundances of each sample group at each site. Only the 20 most abundant taxa are shown, all other are combined into a category “other.” Gibberella and unidentified Fungi dominate all samples, but the proportion of each varies.
TABLE 3. List of genera which are differentially abundant across time and treatment. For each genus, the model coefficient, standard error, p-values, FDR corrected p-values (q-values) are also listed.
FIGURE 7. Boxplots of the top differentially abundant genera in the cecal lumen across time. Y-axis values are the log base two of relative abundance of each genus, X-axis = days.
The mycobiome is becoming increasingly recognized as a critical component of the gut microbiota, with multiple roles in host health including interactions with the immune system, alteration of metabolism, the reduction or exclusion of pathogens, and digestion (Cui et al., 2013; Iliev and Leonardi, 2017; Iliev and Cadwell, 2021). However, few studies have addressed fungal populations in chickens, and the majority utilized culture-based techniques which may not provide a complete inventory of fungal taxa (Shokri et al., 2011; Hume et al., 2012; Byrd et al., 2017; Subramanya et al., 2017; Sokół et al., 2018; Cafarchia et al., 2019). Here, we use next-generation sequencing to investigate developmental changes in the mycobiome in both mucosal and luminal portions of the ileum and cecum and determine whether delays in PH access to feed affect fungal gut communities.
In total, we identified 88 unique fungal genera across 374 samples. Gibberella and unidentified Fungi dominated all samples, with Gibberella present in higher proportion in the cecum (63%) than in the ileum (38%). Two prior mycobiome studies in chicks which utilized ITS2 and Illumina sequencing identified a similar number of genera, 125 (Robinson et al., 2020), and 81 (Robinson et al., 2022), and identified Microascus and Fusarium pseudonygamai as the dominant genera, respectively. Fusaria is the anamorph of Gibberella, thus our findings coincide with these results somewhat. However, unidentified Fungi were also prevalent in our samples, as well as Aspergillus, Cladosporium, Sarocladium, Meyerozyma, and Penicillium. Of these, only Aspergillus was among the most abundant genera in prior studies (Robinson et al., 2022). Although few mycobiome studies have been performed in chickens, a meta-analysis of human mycobiome studies also indicated that only a small proportion of identified gut fungi (15/267 species) were common across studies (Hallen-Adams and Suhr, 2017).
The variable taxonomic composition of the mycobiome can be attributed to the fact that few fungal taxa are suited for permanent colonization of the gut, and are instead transient (Hallen-Adams and Suhr, 2017). Populations are highly influenced by food and the environment as strains enter the gut through ingestion (Penders et al., 2006; Dollive et al., 2013; David et al., 2014; Hallen-Adams and Suhr, 2017; Mims et al., 2021; Robinson et al., 2022). In commercially produced chickens, the majority of mycobiome members present in the early PH days match those present in the hatchery environment, feed, and bedding (Robinson et al., 2022). Further, the most highly abundant taxa are most frequently associated with environmental habitats. Gibberella is a plant pathogen (Desjardins, 2003), Aspergillus spp. are environmental saprobes (Pangging et al., 2022), Cladosporium is ubiquitous among various organic materials (Salvatore et al., 2021) and Penicillium is often associated with food (Yadav et al., 2018).
Due to the critical role of diet and the environment in shaping fungal gut communities, we asked whether delayed PH access to feed affects the development of the chick mycobiome. To mimic conditions in commercial production facilities, we formed two groups, “unfed” which received no feed during the first 2 days PH, and “fed” which received feed upon entry to the battery pen. By day 3, both groups had unlimited access to feed. In the luminal samples of both the cecum and ileum, beta-diversity analyses showed a significantly different community structure in fed and unfed samples during days 1 and 2, but not during later days (Supplementary Figures S7, S8). In the cecal lumen at day 1, fed samples consisted mainly of Gibberella (83%), while unfed communities were composed of Incrucipulum (32%), Cladosporium (25.4%), Coniochaeta (14%), and unidentified Fungi (11%). Incrucipulum and Coniochaeta are environmental taxa, with Incrucipulum often associated with fallen leaves or twigs (Tochihara and Hosoya, 2019), and Coniochaeta found on diverse substrates including soil, plants, butter, and feces (Si et al., 2021). At day 2 Gibberella remained low (4%), Incrucipulum dropped to less than 1%, and Coniochaeta, unidentified Fungi, and Cladosporium comprised the majority of the remainder of the samples. By day 3, Gibberella dominated both fed and unfed samples (84.9% and 69.1%, respectively), and remained the dominant genus through day 14 (fed, 75% and unfed, 74%). In the ileal lumen, fed samples were dominated by Gibberella and unidentified Fungi through all days, but unfed samples on day 1 also contained Purpureocillium (21%), a ubiquitous environmental saprobe found in soil, air, and plant matter (Luangsa-ard et al., 2011), and at day 2, Coniochaeta became dominant (60%). It should be noted that only a single sample comprised the day 2, ileal lumen, unfed experimental group. This sample is described here but excluded from all statistical analyses. Similar to the cecal lumen, by day 3, Gibberella and unidentified Fungi dominated all ileal lumen samples, regardless of whether they received feed immediately PH. Thus, our findings are in line with those prior showing that the mycobiome is greatly influenced by diet (David et al., 2014; Hallen-Adams and Suhr, 2017).
Alpha diversity metrics did not differ significantly across fed and unfed samples, except for the number of ASVs, which was greater in the ileal lumen in fed than in unfed chicks (Figure 1; Supplementary Figure S1). In the cecal lumen, a greater number of ASVs was also identified in fed versus unfed chicks during six of the 9 days tested but was not statistically significant. It is possible that with a greater sample size, statistical significance would be obtained. It is unclear why an increased number of taxa was observed among fed chicks across all time points when PCoA on Bray-Curtis matrices did not show segregation of fed and unfed groups at days past day 2. One explanation is that lowly abundant taxa contribute to the observed differences in ASVs, as Bray-Curtis values are highly influenced by the most abundant taxa. Therefore, it is possible that the withholding of feed during days 1–2 does result in a slight lowering of the number of total taxa present in the ileal lumen through day 14, but likely only among taxa which are present in extremely low abundances.
In contrast, there were no differences in alpha or beta-diversity among fed and unfed samples in the mucosal communities (Supplementary Figures S8, S9). Mucus is a dense layer of polysaccharides and proteins which provides a distinct niche for microbial colonization (Sonnenburg et al., 2004). Microbial residents of the mucosa are often long-term residents of the gut which can withstand variable pH, body temperatures (105–106 in the chicken), low oxygen, and other challenges of the host environment (Hallen-Adams and Suhr, 2017). Luminal communities may also contain taxa capable of surviving in the gut, however, the lumen is also home to numerous environmental taxa which are transient. Thus, mucosal and luminal communities often differ in both bacterial (Chen et al., 2012) and fungal composition (Qiu et al., 2015). Microhabitats may also form within either mucosal or luminal regions which are influenced by the presence of local species and may also affect the spatial organization of taxa within the gut (Eckstein et al., 2020). In our samples, we did not observe a difference in the fed and unfed mucosal samples, suggesting that ingested taxa do not establish in the mucosa.
In agreement with diversity analyses, at all four sites examined, only two unknown genera were detected to be differentially abundant among fed versus unfed chicks, and q-values were only marginally significant (Table 3). Overall, the deprivation of feed during the first 2 days of a chick’s life does not appear to result in substantial long-term changes in either the luminal or mucosal chick mycobiome.
In contrast, at each site, the composition of the mycobiome as measured by beta diversity changed significantly throughout development from hatch through day 14 (Figure 5). A similar pattern was observed in a prior study which examined the luminal mycobiome of broiler chickens across the duodenum, ileum, cecum, and colon on days 3, 7, 14, 21, 27, 35, and 42 (Robinson et al., 2022). Robinson et al. (2022) also observed patterns of alpha diversity which are similar to those in this study including a significant increase in the number of ASVs across days 3, 7, and 14 in the cecal lumen, and no changes in any alpha diversity metric in the ileum. However, they found an increase in Shannon diversity and evenness across day 3, 7, and 14 in the cecal lumen while our data showed a decrease (Robinson 2022). In the ileum, the lack of changes in alpha diversity coupled with significant changes in beta diversity suggest that taxonomic changes drive differences in beta diversity. However, no taxa were detected to be differentially abundant in the ileum mucosa. This may be due to the fact that differential abundance analysis was conducted on combined days 1–4 (early) versus days 6–14 (late), and taxa whose abundances may differ between only two individual days may not have been detected.
Discordance among studies could be caused by methodological or biological differences, or both. For example, Robinson (2022) used male Cobb birds whereas we used a mix of female and male Ross 708 birds, and the mycobiome may vary by gender (Strati et al., 2016) and host genotype (Wu et al., 2021). Robinson (2022) used birds raised in floor pens on shavings whereas we raised birds in battery pens. Furthermore, DNA isolation kits differed among the studies as did the bioinformatic workflows including the choice of database.
Bacterial communities in the gut generally increase in diversity and become more stable over time (Agans et al., 2011; Danzeisen et al., 2013; Oakley and Kogut, 2016; Dill-McFarland et al., 2017). In contrast, mycobiome alpha diversity values are generally high at hatch and then decrease over time (Strati et al., 2016; Arfken et al., 2020). However, trends are often variable during the earliest stages of development following hatch (Ward Tonya et al., 2018; Arfken et al., 2020), and our data support this. For example, the number of ASVs plotted at each timepoint (Supplementary Figure S8), shows that trends across sites vary by age. Thus, at least some proportion of the discrepancies across studies can be attributed to variability in the mycobiome during early development, as well as methodological differences.
Changes in taxonomic composition were also observed across development at all sites except for the ileal mucosa (Table 3; Figure 6). In the cecal lumen, 19 genera were detected as differentially abundant, 15 were detected in the ileal lumen, and 3 in the cecal mucosa. In the cecal lumen, the largest increases in abundance were noted among Wallemia, Meyerozyma, Penicillium, Pyxidiophora, and Kurtzmaniella, while total unidentified Fungi decreased (Figure 7), but in the cecal mucosa, only Gibberella increased over time and total unidentified Fungi decreased (Table 3). In the ileal lumen, Clavispora, Suhomyces, Dipodascus, and Fusarium increased while Coniochaeta decreased. The fact that a greater number of taxa changed over time in luminal samples than in mucosal ones supports the idea that mucosal communities may be less susceptible to changes in diet and/or the environment, and that luminal taxa are more transient.
It is well established that gut microbial composition shifts during early development (Ballou et al., 2016; Moore and Townsend, 2019), and the microbiome plays a key role in maturation of the immune, metabolic, and hormone development in young animals (Stiemsma and Michels, 2018). However, less information is available regarding the mycobiome. Due to the large influence of diet and the environment on fungal communities, the mycobiome is often more variable than bacterial gut communities (Nash et al., 2017; Ward Tonya et al., 2018). Still, a small number of key fungi consistently dominate the neonatal and infant gut (Fujimura et al., 2016), as well as that of chicks (Robinson et al., 2022). Since fungi play a critical role in disease and the establishment of bacterial populations (Stiemsma and Michels, 2018), further studies are needed to establish the role of the mycobiome in early development.
Last, our analyses also indicate that mycobiome communities differ across locations in the GI. Beta diversity analyses show that all sites (cecal lumen, cecal mucosa, ileal lumen, and ileal mucosa) differed significantly from each other (Figure 4). In addition to Gibberella and unidentified Fungi, Aspergillus and Cladosporium were among the most abundant taxa at all sites, along with Meyerozyma and Sarocladium at both cecal sites, Wallemia, Penicillium, and Mucor in the ileal lumen, and unidentified genera within the Ascomycota Phylum, Coniochaeta, and Rhodotorula in the ileal mucosa. Many rare taxa also differed across sites (Supplementary Table S5). The independent clustering of fungal communities at different locations along the GI is supported by prior studies (Robinson et al., 2020; Robinson et al., 2022). However, trends of alpha-diversity in this study differed slightly from recent studies. Robinson (2022) found that at day 42, alpha diversity metrics differed significantly along the GI, and values at each site roughly followed a bell shaped curve with the lowest diversity reported in the crop, then increasing in the ventriculus, peaking in the duodenum and jejunum, and then lowering through the ileum, cecum, and colon (Robinson et al., 2022). In a prior study at day 28, general trends suggested alpha diversity was higher in the upper GI than in the lower GI (Robinson et al., 2020). In our analysis, which included data from days 1–14, alpha diversity metrics in the cecum and ileal lumens were not significantly different from each other (Figure 2). As discussed above in regard to temporal patterns of alpha-diversity, discrepancies in alpha diversity trends across sites are also variable just after hatch (Supplementary Figure S11) or may be due to methodological differences.
Although we did not find differences in alpha diversity values between the cecum and ileum luminal communities, we did find that the number of ASVs, evenness, and the Shannon index values were greater in the mucosal communities than in the luminal ones (Figure 2). In humans, mucosal communities are less diverse than luminal ones (Leonardi et al., 2022), and it is unclear why our data shows the opposite trend. Again, it is possible that trends fluctuate during early development, and later stabilize. Regardless, to our knowledge, this is the first study of mucosal fungal communities in the GI of chickens. Mucosal communities play a critical role in health due to their close association with the host (Luan et al., 2015; Huseyin et al., 2017). Thus, understanding changes in the mucosal mycobiome alongside those which occur in the lumen are integral to understanding and ultimately manipulating host health.
Together, our results indicate that the chick mycobiome is a dynamic component of the gut microbiome which changes throughout development and across sites in the GI tract. We did not find that withholding feed during the first 2 days of life leads to long term consequences in mycobiome composition, suggesting that the developing mycobiome does not play a role in the negative health consequences observed with PH delays in feeding. However, it is likely that the developing mycobiome does play a role in other aspects of chicken health as has been shown in other organisms (Cui et al., 2013; Iliev and Leonardi, 2017; Iliev and Cadwell, 2021). Research to develop fungal probiotics and other therapeutics in chickens is ongoing (Saleh et al., 2014; Sugiharto et al., 2017), and this study provides a foundation by which to advance such efforts and ultimately improve the health, wellbeing, and productivity of chickens.
The datasets presented in this study can be found in online repositories. The names of the repository/repositories and accession number(s) can be found below: https://www.ncbi.nlm.nih.gov/genbank/, PRJNA779402.
The animal study was reviewed and approved by the USDA-ARS-BARC Institutional Animal Care and Use Committee.
MP-W conceived, planned, and performed the experiments and assisted with writing the manuscript, KS assisted with experimental design and writing the manuscript, CD assisted with data analyses and writing the manuscript, AA assisted with experimental design and data analyses, JF performed scientific experiments, ND assisted with the first draft of bioinformatic analyses, AC performed lab analyses, library construction and sequencing, and assisted with writing , LS performed animal work, lab analyses, and assisted with writing.
The work was funded by in house USDA-ARS CRIS # 8042–31000-114-00D and CRIS # 8042-31440-001-00D.
We would like to thank Martine Ferguson for her input on statistical analyses.
The authors declare that the research was conducted in the absence of any commercial or financial relationships that could be construed as a potential conflict of interest.
All claims expressed in this article are solely those of the authors and do not necessarily represent those of their affiliated organizations, or those of the publisher, the editors and the reviewers. Any product that may be evaluated in this article, or claim that may be made by its manufacturer, is not guaranteed or endorsed by the publisher.
The Supplementary Material for this article can be found online at: https://www.frontiersin.org/articles/10.3389/fphys.2022.1057810/full#supplementary-material
Agans R., Rigsbee L., Kenche H., Michail S., Khamis H. J., Paliy O. (2011). Distal gut microbiota of adolescent children is different from that of adults. FEMS Microbiol. Ecol. 77 (2), 404–412. doi:10.1111/j.1574-6941.2011.01120.x
Arfken A. M., Frey J. F., Summers K. L. (2020). Temporal dynamics of the gut bacteriome and mycobiome in the weanling pig. Microorganisms 8 (6), 868. doi:10.3390/microorganisms8060868
Arfken A. M., Summers K. L. (2022). “The gut mycobiome and animal health,” in Gut microbiota, immunity, and health in production animals. Editors M. Kogut, and G. Zhang (Cham: Springer), 85–125.
Ballou A. L., Ali R. A., Mendoza M. A., Ellis J. C., Hassan H. M., Croom W. J., et al. (2016). Development of the chick microbiome: How early exposure influences future microbial diversity. Front. Vet. Sci. 3, 2. doi:10.3389/fvets.2016.00002
Banani H., Marcet-Houben M., Ballester A-R., Abbruscato P., González-Candelas L., Gabaldón T., et al. (2016). Genome sequencing and secondary metabolism of the postharvest pathogen Penicillium griseofulvum. BMC Genomics 17 (1), 19. doi:10.1186/s12864-015-2347-x
Bigot K., Mignon-Grasteau S., Picard M., Tesseraud S. (2003). Effects of delayed feed intake on body, intestine, and muscle development in neonate broilers. Poult. Sci. 82 (5), 781–788. doi:10.1093/ps/82.5.781
Byrd J. A., Caldwell D. Y., Nisbet D. J. (2017). The identification of fungi collected from the ceca of commercial poultry. Poult. Sci. 96 (7), 2360–2365. doi:10.3382/ps/pew486
Cafarchia C., Iatta R., Danesi P., Camarda A., Capelli G., Otranto D. (2019). Yeasts isolated from cloacal swabs, feces, and eggs of laying hens. Med. Mycol. 57 (3), 340–345. doi:10.1093/mmy/myy026
Careghi C., Tona K., Onagbesan O., Buyse J., Decuypere E., Bruggeman V. (2005). The effects of the spread of hatch and interaction with delayed feed access after hatch on broiler performance until seven days of age. Poult. Sci. 84 (8), 1314–1320. doi:10.1093/ps/84.8.1314
Chambers J. R., Gong J. (2011). The intestinal microbiota and its modulation for Salmonella control in chickens. Food Res. Int. 44 (10), 3149–3159. doi:10.1016/j.foodres.2011.08.017
Chen W., Liu F., Ling Z., Tong X., Xiang C. (2012). Human intestinal lumen and mucosa-associated microbiota in patients with colorectal cancer. PLOS ONE 7 (6), e39743. doi:10.1371/journal.pone.0039743
Clavijo V., Flórez M. J. V. (2018). The gastrointestinal microbiome and its association with the control of pathogens in broiler chicken production: A review. Poult. Sci. 97 (3), 1006–1021. doi:10.3382/ps/pex359
Cui L., Morris A., Ghedin E. (2013). The human mycobiome in health and disease. Genome Med. 5 (7), 63. doi:10.1186/gm467
Curbete M. M., Salgado H. R. N. (2016). A critical review of the properties of fusidic acid and analytical methods for its determination. Crit. Rev. Anal. Chem. 46 (4), 352–360. doi:10.1080/10408347.2015.1084225
Danzeisen J. L., Calvert A. J., Noll S. L., McComb B., Sherwood J. S., Logue C. M., et al. (2013). Succession of the Turkey gastrointestinal bacterial microbiome related to weight gain. PeerJ 1, e237. doi:10.7717/peerj.237
David L. A., Maurice C. F., Carmody R. N., Gootenberg D. B., Button J. E., Wolfe B. E., et al. (2014). Diet rapidly and reproducibly alters the human gut microbiome. Nature 505 (7484), 559–563. doi:10.1038/nature12820
de Jong I. C., van Riel J., Bracke M. B. M., van den Brand H. (2017). A 'meta-analysis' of effects of post-hatch food and water deprivation on development, performance and welfare of chickens. PLoS One 12 (12), e0189350. doi:10.1371/journal.pone.0189350
Desjardins A. E. (2003). Gibberella from A (venaceae) to Z (eae). Annu. Rev. Phytopathol. 41 (1), 177–198. doi:10.1146/annurev.phyto.41.011703.115501
Dill-McFarland K. A., Breaker J. D., Suen G. (2017). Microbial succession in the gastrointestinal tract of dairy cows from 2 weeks to first lactation. Sci. Rep. 7, 40864. doi:10.1038/srep40864
Dollive S., Chen Y-Y., Grunberg S., Bittinger K., Hoffmann C., Vandivier L., et al. (2013). Fungi of the murine gut: Episodic variation and proliferation during antibiotic treatment. PLOS ONE 8 (8), e71806. doi:10.1371/journal.pone.0071806
dos Santos J. D., Fugisaki L. R. D. O., Medina R. P., Scorzoni L., Alves MdS., de Barros P. P., et al. (2020). Streptococcus mutans secreted products inhibit Candida albicans induced oral candidiasis. Front. Microbiol. 11, 1605. doi:10.3389/fmicb.2020.01605
Eckstein M-T., Moreno-Velásquez S. D., Pérez J. C. (2020). Gut bacteria shape intestinal microhabitats occupied by the fungus Candida albicans. Curr. Biol. 30 (23), 4799–4807. e4. doi:10.1016/j.cub.2020.09.027
Fujimura K. E., Sitarik A. R., Havstad S., Lin D. L., Levan S., Fadrosh D., et al. (2016). Neonatal gut microbiota associates with childhood multisensitized atopy and T cell differentiation. Nat. Med. 22 (10), 1187–1191. doi:10.1038/nm.4176
Hallen-Adams H. E., Suhr M. J. (2017). Fungi in the healthy human gastrointestinal tract. Virulence 8 (3), 352–358. doi:10.1080/21505594.2016.1247140
Huffnagle G. B., Noverr M. C. (2013). The emerging world of the fungal microbiome. Trends Microbiol. 21 (7), 334–341. doi:10.1016/j.tim.2013.04.002
Hume M. E., Hernandez C. A., Barbosa N. A., Sakomura N. K., Dowd S. E., Oviedo-Rondón E. O. (2012). Molecular identification and characterization of ileal and cecal fungus communities in broilers given probiotics, specific essential oil blends, and under mixed eimeria infection. Foodborne Pathog. Dis. 9 (9), 853–860. doi:10.1089/fpd.2011.1093
Huseyin C. E., O’Toole P. W., Cotter P. D., Scanlan P. D. (2017). Forgotten fungi—The gut mycobiome in human health and disease. FEMS Microbiol. Rev. 41 (4), 479–511. doi:10.1093/femsre/fuw047
Iliev I. D., Cadwell K. (2021). Effects of intestinal fungi and viruses on immune responses and inflammatory bowel diseases. Gastroenterology 160 (4), 1050–1066. doi:10.1053/j.gastro.2020.06.100
Iliev I. D., Leonardi I. (2017). Fungal dysbiosis: Immunity and interactions at mucosal barriers. Nat. Rev. Immunol. 17 (10), 635–646. doi:10.1038/nri.2017.55
Kim D., Sengupta A., Niepa T. H. R., Lee B-H., Weljie A., Freitas-Blanco V. S., et al. (2017). Candida albicans stimulates Streptococcus mutans microcolony development via cross-kingdom biofilm-derived metabolites. Sci. Rep. 7 (1), 41332. doi:10.1038/srep41332
Kõljalg U., Nilsson R. H., Abarenkov K., Tedersoo L., Taylor A. F. S., Bahram M., et al. (2013). Towards a unified paradigm for sequence-based identification of fungi. Mol. Ecol. 22 (21), 5271–5277. doi:10.1111/mec.12481
Leonardi I., Gao I. H., Lin W-Y., Allen M., Li X. V., Fiers W. D., et al. (2022). Mucosal fungi promote gut barrier function and social behavior via Type 17 immunity. Cell 185 (5), 831–846.e14. e14. doi:10.1016/j.cell.2022.01.017
Luan C., Xie L., Yang X., Miao H., Lv N., Zhang R., et al. (2015). Dysbiosis of fungal microbiota in the intestinal mucosa of patients with colorectal adenomas. Sci. Rep. 5, 7980. doi:10.1038/srep07980
Luangsa-ard J., Houbraken J., van Doorn T., Hong S-B., Borman A. M., Hywel-Jones N. L., et al. (2011). Purpureocillium, a new genus for the medically important Paecilomyces lilacinus. FEMS Microbiol. Lett. 321 (2), 141–149. doi:10.1111/j.1574-6968.2011.02322.x
Martin M. (2011). Cutadapt removes adapter sequences from high-throughput sequencing reads. EMBnet. J. 17, 10. Next Generation Sequencing Data AnalysisDO. doi:10.14806/ej17.1.200
Mason Katie L., Erb Downward John R., Falkowski Nicole R., Young Vincent B., Kao John Y., Huffnagle Gary B. (2012). Interplay between the gastric bacterial microbiota and Candida albicans during postantibiotic recolonization and gastritis. Infect. Immun. 80 (1), 150–158. doi:10.1128/IAI.05162-11
Mims T. S., Abdallah Q. A., Stewart J. D., Watts S. P., White C. T., Rousselle T. V., et al. (2021). The gut mycobiome of healthy mice is shaped by the environment and correlates with metabolic outcomes in response to diet. Commun. Biol. 4 (1), 281. doi:10.1038/s42003-021-01820-z
Mitchell M. A. (2009). Chick transport and welfare. Avian Biol. Res. 2 (1-2), 99–105. doi:10.3184/175815509x431894
Moore R. E., Townsend S. D. (2019). Temporal development of the infant gut microbiome. Open Biol. 9 (9), 190128. doi:10.1098/rsob.190128
Nash A. K., Auchtung T. A., Wong M. C., Smith D. P., Gesell J. R., Ross M. C., et al. (2017). The gut mycobiome of the Human Microbiome Project healthy cohort. Microbiome 5 (1), 153. doi:10.1186/s40168-017-0373-4
Oakley B. B., Kogut M. H. (2016). Spatial and temporal changes in the broiler chicken cecal and fecal microbiomes and correlations of bacterial taxa with cytokine gene expression. Front. Vet. Sci. 3, 11. doi:10.3389/fvets.2016.00011
Oakley B. B., Lillehoj H. S., Kogut M. H., Kim W. K., Maurer J. J., Pedroso A., et al. (2014). The chicken gastrointestinal microbiome. FEMS Microbiol. Lett. 360 (2), 100–112. doi:10.1111/1574-6968.12608
Oksanen J., Simpson G., Blanchet F., Kindt R., Legendre P., Minchin P., et al. (2022). Vegan: Community ecology package. R package version 26-2 2022.
Pan D., Yu Z. (2014). Intestinal microbiome of poultry and its interaction with host and diet. Gut Microbes 5 (1), 108–119. doi:10.4161/gmic.26945
Pangging M., Nguyen T. T. T., Lee H. B. (2022). Seven undescribed Aspergillus species from different niches in korea. Mycobiology 50 (4), 189–202. doi:10.1080/12298093.2022.2116158
Payne J. A., Proszkowiec-Weglarz M., Ellestad L. E. (2019). Delayed access to feed alters expression of genes associated with carbohydrate and amino acid utilization in newly hatched broiler chicks. Am. J. Physiol. Regul. Integr. Comp. Physiol. 317 (6), R864–r78. doi:10.1152/ajpregu.00117.2019
Penders J., Thijs C., Vink C., Stelma F. F., Snijders B., Kummeling I., et al. (2006). Factors influencing the composition of the intestinal microbiota in early infancy. Pediatrics 118 (2), 511–521. doi:10.1542/peds.2005-2824
Powell D. J., Velleman S. G., Cowieson A. J., Singh M., Muir W. I. (2016). Influence of chick hatch time and access to feed on broiler muscle development. Poult. Sci. 95 (6), 1433–1448. doi:10.3382/ps/pew047
Proszkowiec-Weglarz M., Miska K. B., Ellestad L. E., Schreier L. L., Kahl S., Darwish N., et al. (2022). Delayed access to feed early post-hatch affects the development and maturation of gastrointestinal tract microbiota in broiler chickens. BMC Microbiol. 22 (1), 206. doi:10.1186/s12866-022-02619-6
Proszkowiec-Weglarz M., Schreier L. L., Kahl S., Miska K. B., Russell B., Elsasser T. H. (2020). Effect of delayed feeding post-hatch on expression of tight junction- and gut barrier-related genes in the small intestine of broiler chickens during neonatal development. Poult. Sci. 99 (10), 4714–4729. doi:10.1016/j.psj.2020.06.023
Proszkowiec-Weglarz M., Schreier L. L., Miska K. B., Angel R., Kahl S., Russell B. (2019). Effect of early neonatal development and delayed feeding post-hatch on jejunal and ileal calcium and phosphorus transporter genes expression in broiler chickens. Poult. Sci. 98 (4), 1861–1871. doi:10.3382/ps/pey546
Qiu X., Zhang F., Yang X., Wu N., Jiang W., Li X., et al. (2015). Changes in the composition of intestinal fungi and their role in mice with dextran sulfate sodium-induced colitis. Sci. Rep. 5 (1), 10416. doi:10.1038/srep10416
Qu Y., Kahl S., Miska K. B., Schreier L. L., Russell B., Elsasser T. H., et al. (2021). The effect of delayed feeding post-hatch on caeca development in broiler chickens. Br. Poult. Sci. 62 (5), 731–748. doi:10.1080/00071668.2021.1912291
R Core Team (2022). A language and environment for statistical computing. Vienna, Austria: R Foundation for Statistical Computing.
Richards M. P., Proszkowiec-Weglarz M., Rosebrough R. W., McMurtry J. P., Angel R. (2010). Effects of early neonatal development and delayed feeding immediately post-hatch on the hepatic lipogenic program in broiler chicks. Comp. Biochem. Physiol. B Biochem. Mol. Biol. 157 (4), 374–388. doi:10.1016/j.cbpb.2010.08.007
Robinson K., Xiao Y., Johnson T. J., Chen B., Yang Q., Lyu W., et al. (2020). Chicken intestinal mycobiome: Initial characterization and its response to bacitracin methylene disalicylate. Appl. Environ. Microbiol. 86 (13), e00304. doi:10.1128/AEM.00304-20
Robinson K., Yang Q., Stewart S., Whitmore M. A., Zhang G. (2022). Biogeography, succession, and origin of the chicken intestinal mycobiome. Microbiome 10 (1), 55. doi:10.1186/s40168-022-01252-9
Saleh A. A., Amber K., El-Magd M. A., Atta M. S., Mohammed A. A., Ragab M. M., et al. (2014). Integrative effects of feeding Aspergillus awamori and fructooligosaccharide on growth performance and digestibility in broilers: Promotion muscle protein metabolism. Biomed. Res. Int. 2014, 946859. doi:10.1155/2014/946859
Salvatore M. M., Andolfi A., Nicoletti R. (2021). The genus cladosporium: A rich source of diverse and bioactive natural compounds. Molecules 26 (13), 3959. doi:10.3390/molecules26133959
Sam Q. H., Chang M. W., Chai L. Y. (2017). The fungal mycobiome and its interaction with gut bacteria in the host. Int. J. Mol. Sci. 18, 330. (Electronic). doi:10.3390/ijms18020330
Shinde Tamboli A. S., Goel A., Mehra M., Rokade J. J., Bhadauria P., Yadav A. S., et al. (2018). Delayed post-hatch feeding affects the performance and immunocompetence differently in male and female broiler chickens. J. Appl. Animal Res. 46 (1), 306–313. doi:10.1080/09712119.2017.1299739
Shokri H., Khosravi A., Nikaein D. (2011). A comparative study of digestive tract mycoflora of broilers with layers. Iran. J. Veterinary Med. 5 (1), 1–4. doi:10.22059/ijvm.2011.22662
Si H. L., Su Y. M., Zheng X. X., Ding M. Y., Bose T., Chang R. L. (2021). Phylogenetic and morphological analyses of Coniochaeta isolates recovered from Inner Mongolia and Yunnan revealed three new endolichenic fungal species. MycoKeys 83, 105–121. doi:10.3897/mycokeys.83.71140
Sokół I., Gaweł A., Bobrek K. (2018). The prevalence of yeast and characteristics of the isolates from the digestive tract of clinically healthy turkeys. Avian Dis. 62 (3), 286–290. doi:10.1637/11780-121117-Reg.1
Sonnenburg J. L., Angenent L. T., Gordon J. I. (2004). Getting a grip on things: How do communities of bacterial symbionts become established in our intestine? Nat. Immunol. 5 (6), 569–573. doi:10.1038/ni1079
Stanley D., Hughes R. J., Moore R. J. (2014). Microbiota of the chicken gastrointestinal tract: Influence on health, productivity and disease. Appl. Microbiol. Biotechnol. 98 (10), 4301–4310. doi:10.1007/s00253-014-5646-2
Stiemsma L. T., Michels K. B. (2018). The role of the microbiome in the developmental origins of health and disease. Pediatrics 141 (4), e20172437. doi:10.1542/peds.2017-2437
Strati F., Di Paola M., Stefanini I., Albanese D., Rizzetto L., Lionetti P., et al. (2016). Age and gender affect the composition of fungal population of the human gastrointestinal tract. Front. Microbiol. 7, 1227. doi:10.3389/fmicb.2016.01227
Subramanya S. H., Sharan N. K., Baral B. P., Hamal D., Nayak N., Prakash P. Y., et al. (2017). Diversity, in-vitro virulence traits and antifungal susceptibility pattern of gastrointestinal yeast flora of healthy poultry, Gallus gallus domesticus. BMC Microbiol. 17 (1), 113. doi:10.1186/s12866-017-1024-4
Sugiharto S., Yudiarti T., Isroli I., Widiastuti E., Putra F. D. (2017). Effect of dietary supplementation with Rhizopus oryzae Chrysonilia crassa on growth performance, blood profile, intestinal microbial population, and carcass traits in broilers exposed to heat stress. Arch. Anim. Breed. 60 (3), 347–356. doi:10.5194/aab-60-347-2017
Tochihara Y., Hosoya T. (2019). Three new species of Incrucipulum (lachnaceae, helotiales, Ascomycota) from Japan. Phytotaxa 403 (1), 25. doi:10.11646/phytotaxa.403.1.2
Tso G. H. W., Reales-Calderon J. A., Tan A. S. M., Sem X., Le G. T. T., Tan T. G., et al. (2018). Experimental evolution of a fungal pathogen into a gut symbiont. Science 362 (6414), 589–595. doi:10.1126/science.aat0537
van de Ven L. J., van Wagenberg A. V., Groot Koerkamp P. W., Kemp B., van den Brand H. (2009). Effects of a combined hatching and brooding system on hatchability, chick weight, and mortality in broilers. Poult. Sci. 88 (11), 2273–2279. doi:10.3382/ps.2009-00112
Ward Tonya L., Dominguez-Bello Maria G., Heisel T., Al-Ghalith G., Knights D., Gale Cheryl A. (2018). Development of the human mycobiome over the first month of life and across body sites. mSystems 3 (3), 001400–e217. doi:10.1128/mSystems.00140-17
Willemsen H., Debonne M., Swennen Q., Everaert N., Careghi C., Han H., et al. (2010). Delay in feed access and spread of hatch: Importance of early nutrition. World's. Poult. Sci. J. 66 (2), 177–188. doi:10.1017/s0043933910000243
Wu X., Xia Y., He F., Zhu C., Ren W. (2021). Intestinal mycobiota in health and diseases: From a disrupted equilibrium to clinical opportunities. Microbiome 9 (1), 60. doi:10.1186/s40168-021-01024-x
Yadav A. N., Verma P., Kumar V., Sangwan P., Mishra S., Panjiar N., et al. (2018). “Chapter 1 - biodiversity of the genus Penicillium in different habitats,” in New and future developments in microbial biotechnology and bioengineering. Editors V. K. Gupta, and S. Rodriguez-Couto (Amsterdam: Elsevier), 3–18.
Keywords: chicken, broiler, fungi, mycobiome, microbiome temporal chicken mycobiome
Citation: Davies CP, Summers KL, Arfken AM, Darwish N, Chaudhari A, Frey JF, Schreier L and Proszkowiec-Weglarz M (2022) Temporal dynamics of the chicken mycobiome. Front. Physiol. 13:1057810. doi: 10.3389/fphys.2022.1057810
Received: 30 September 2022; Accepted: 30 November 2022;
Published: 15 December 2022.
Edited by:
Hai Lin, Shandong Agricultural University, ChinaCopyright © 2022 Davies, Summers, Arfken, Darwish, Chaudhari, Frey, Schreier and Proszkowiec-Weglarz. This is an open-access article distributed under the terms of the Creative Commons Attribution License (CC BY). The use, distribution or reproduction in other forums is permitted, provided the original author(s) and the copyright owner(s) are credited and that the original publication in this journal is cited, in accordance with accepted academic practice. No use, distribution or reproduction is permitted which does not comply with these terms.
*Correspondence: Cary Pirone Davies, Y2FyeS5kYXZpZXNAdXNkYS5nb3Y=
Disclaimer: All claims expressed in this article are solely those of the authors and do not necessarily represent those of their affiliated organizations, or those of the publisher, the editors and the reviewers. Any product that may be evaluated in this article or claim that may be made by its manufacturer is not guaranteed or endorsed by the publisher.
Research integrity at Frontiers
Learn more about the work of our research integrity team to safeguard the quality of each article we publish.