- 1School of Rehabilitation, Capital Medical University, Beijing, China
- 2Department of Spinal and Neural Functional Reconstruction, China Rehabilitation Research Center, Beijing, China
- 3Chinese Institute of Rehabilitation Science, Beijing, China
- 4Center of Neural Injury and Repair, Beijing Institute for Brain Disorders, Beijing, China
- 5Beijing Key Laboratory of Neural Injury and Rehabilitation, Beijing, China
- 6School of Rehabilitation Sciences and Engineering, University of Health and Rehabilitation Sciences, Qingdao, China
The balance of ion concentrations inside and outside the cell is an essential homeostatic mechanism in neurons and serves as the basis for a variety of physiological activities. In the central nervous system, NKCC1 and KCC2, members of the SLC12 cation-chloride co-transporter (CCC) family, participate in physiological and pathophysiological processes by regulating intracellular and extracellular chloride ion concentrations, which can further regulate the GABAergic system. Over recent years, studies have shown that NKCC1 and KCC2 are essential for the maintenance of Cl− homeostasis in neural cells. NKCC1 transports Cl− into cells while KCC2 transports Cl− out of cells, thereby regulating chloride balance and neuronal excitability. An imbalance of NKCC1 and KCC2 after spinal cord injury will disrupt CI− homeostasis, resulting in the transformation of GABA neurons from an inhibitory state into an excitatory state, which subsequently alters the spinal cord neural network and leads to conditions such as spasticity and neuropathic pain, among others. Meanwhile, studies have shown that KCC2 is also an essential target for motor function reconstruction after spinal cord injury. This review mainly introduces the physiological structure and function of NKCC1 and KCC2 and discusses their pathophysiological roles after spinal cord injury.
Introduction
Spinal cord injury (SCI) is a commonly occurring disabling disease that can lead to different degrees of impairment of motor, sensory, and autonomic functions in the segment below the site of injury (McDonald and Sadowsky, 2002). Nearly one million new spinal cord injuries occur annually worldwide, imposing a heavy burden on the families of patients and society as a whole (Cieza et al., 2021; GBD 2016 Neurology Collaborators, 2019a, GBD 2016 Traumatic Brain Injury and Spinal Cord Injury, 2019b). After SCI, in addition to damage to the motor and sensory pathways, injury to other pathways and the adaptive changes in the damaged lower segment result in profound changes in the microenvironment of the spinal cord (O'Shea et al., 2017). Complications such as spasticity and neuropathy can also seriously affect the mood, sleep, quality of life, cognitive function, recreational activities, and even the employment of patients, and can also dramatically affect the recovery process (O'Shea et al., 2017; Finnerup, 2017). Currently, the treatment of direct dysfunction and complications caused by SCI is relatively limited, and the individual differences are significant (Ahuja et al., 2017). A lack of a detailed understanding of the physiological mechanism of spinal cord regulation and the pathophysiological process occurring after SCI injury greatly contributes to the scarcity of available treatment options (McDonald and Sadowsky, 2002; Ahuja et al., 2017).
Over recent years, potassium-chloride co-transporter 2 (KCC2) and sodium-potassium-chloride co-transporter 1 (NKCC1) have been increasingly recognized as playing important roles after SCI (Kaila et al., 2014; Tang, 2020). These two co-transporters proteins are known to exert critical regulatory effects in the recovery from spasticity and neuropathic pain as well as the restoration of motor function after SCI (Kaila et al., 2014; Chen et al., 2018; Côme et al., 2020). This review mainly introduces the physiological functions of KCC2 and NKCC1 and the research progress on their roles in spasticity, neuropathic pain, and motor function recovery following injury to the spinal cord.
Overview of KCC2/NKCC1
Cation-chloride co-transporters (CCC) mediate the coupled movement of Cl−, K+, and/or Na+ across the plasma membrane, which underlies the regulation of intracellular Cl− concentrations and plays an important role in neuronal excitability, transepithelial salt and water movement, and the regulation of cell volume (Xie et al., 2020; Zhang et al., 2021). There are two main subfamilies of CCCs, namely, Na+ (K+)-coupled transporters [NKCC1, NKCC2 and NCC (SLC12A1–3)] and K+-coupled transporters [KCC1–4 (SLC12A4–7)] (Russell, 2000; Arroyo et al., 2013).
The structure and expression of KCC2
KCC2 is the major potassium concentration gradient-driven K+-Cl− efflux co-transporter, functioning to reduce the intracellular Cl− concentration, which is encoded by the solute carrier family 12 member 5 (SLC12A5) gene and consists of 12 transmembrane domains (TMDs) and intracellular N and C termini (Payne et al., 1996). In addition, KCC2 contains an extracellular domain (ECD), formed by a large loop between transmembrane helix 5 (TM5) and TM6, and a C-terminal domain (CTD) immediately following TM12 (Payne, 2012; Xie et al., 2020). The CTD regulates the expression, transport, and activity of KCC2 via phosphorylation and dephosphorylation (Lee et al., 2010). KCC2 has two isoforms—KCC2a and KCC2b—that differ in their N termini and are regulated by different promoters (Agez et al., 2017). Of the two isoforms, the expression of KCC2a is relatively low, KCC2b plays the major role in the mature cerebral cortex, hippocampus, and cerebellum (Markkanen et al., 2014). KCC2b expression is significantly up-regulated in the cerebral cortex and cerebellum of rats within 1 week of birth, and can account for 90% of the total KCC2 protein content in the cortex in adulthood (Uvarov et al., 2009). However, in the spinal cord, the two isoforms are expressed at similar levels, and have similar but not completely overlapping distribution patterns in the anterior and posterior spinal cords (Uvarov et al., 2009; Markkanen et al., 2014). When KCC2 was knocked out, mice developed generalized epilepsy and died shortly after birth from severe motor and respiratory deficits (Hübner et al., 2001). However, when the KCC2b isoform was selectively knocked out, the mice survived for 3 weeks after birth (Woo et al., 2002). The overexpression of KCC2a resulted in a significant negative shift in γ-aminobutyric acid (GABA) reversal potential, like that seen with KCC2b overexpression (Markkanen et al., 2017). This suggested that the physiological function of the KCC2a subtype is similar to that of KCC2b and can compensate for its physiological function, at least to some extent.
The structure and expression of NKCC1
NKCCs belong to the CCC family and mediate the transport of Na+, K+, and Cl− ions into cells. NKCC1 and NKCC2 are the only two currently known NKCC family members (Haas and Forbush, 2000). In humans, NKCC1 is encoded by the SLC12A2 gene located on chromosome 5q23 (Markadieu and Delpire, 2014). Like KCC2, NKCC1 also has 12 α-helical transmembrane domains that are flanked by long non-hydrophobic domains at the N and C termini. NKCC1 contains a total of 1,212 amino acid residues and a relative molecular mass of 131.4 kDa (Gamba et al., 1994; Markadieu and Delpire, 2014). The NKCC1 protein is widely expressed in central and peripheral neurons, especially in the cerebral cortex, striatum, hippocampal pyramidal cells, glial cells, and spinal dorsal root ganglia (Pond et al., 2006; Pitcher et al., 2007; Krystal et al., 2012). In general, NKCC1 expression is much higher in glial cells than in neurons (Virtanen et al., 2020). In addition, NKCC1 is widely distributed in cells of the cardiovascular system, the outer plexiform layer of the distal retina, and the skeletal muscle system (Jaggi et al., 2015). NKCC2 isoforms are only found in renal medullary regions, loops, and periglomerular organs (Payne and Forbush, 1995). In the early stages of mammalian development, the expression of NKCC1 in the central nervous system is higher than that of KCC2 (Medina et al., 2014). As development progresses, the expression of NKCC1 gradually decreases, whereas that of KCC2 gradually increases, resulting in a change in GABA activity from depolarizing to hyperpolarizing (Ben-Ari, 2002; Di Cristo et al., 2018).
The physiological function of KCC2 and NKCC1
KCC2 is the primary Cl− export mechanism in mature mammalian neurons, driving intracellular Cl− concentrations below their electrochemical equilibrium potential, thereby enhancing GABAA hyperpolarization and postsynaptic inhibition levels (Coull et al., 2003; Kahle et al., 2014). In contrast, NKCC1, expressed in afferent neurons, drives the intracellular concentration of Cl− above its equilibrium potential, thereby promoting primary afferent depolarization and presynaptic inhibition, which is critical for gating sensory information from the peripheral to the central nervous system (Kahle et al., 2014; Kaila et al., 2014). The neurotransmitter GABA mainly regulates neuronal excitability through GABA type A receptors (GABAARs), which are ligand-gated Cl− channels (Kahle et al., 2008). The effect of GABAARs on cell function depends on the relative concentration of intracellular Cl−, and the membrane potential is determined by intracellular and extracellular Cl− concentrations and remains relatively constant, when GABAAR channels open, the membrane potential is pulled toward the Cl− equilibrium potential (Chamma et al., 2012). In settings of low KCC2 and high NKCC1 activity, such as in early development or in specific neuropathic pain states, the Cl− influx mechanism overrides the KCC2-mediated Cl− efflux, resulting in high intracellular Cl− concentrations, and GABAAR activation results in depolarization (Kahle et al., 2014; Tillman and Zhang, 2019). When KCC2 activity is high and NKCC1 activity is low, such as in healthy, mature neurons, KCC2-mediated efflux maintains a low intracellular Cl− concentration, enabling GABAAR activation and leading to neuronal hyperpolarization (Tillman and Zhang, 2019).
GABA is the primary inhibitory neurotransmitter in the central nervous system of mature mammals, and its primary role is the reduction of the excitability of neurons, and also involved in regulating muscle tone through the modulation of nerve impulses (Watanabe et al., 2002). These physiological roles of GABA are exerted in an intracellular chloride concentration-depend manner (Kaila et al., 2014; Chamma et al., 2012). Meanwhile, it has been reported that GABAAR activity can also affect the function of KCC2 (Heubl et al., 2017). GABAAR activation can reduce the diffusion coefficient of KCC2, thereby increasing its membrane concentration and stability, while GABAAR blockers can increase the fluidity of KCC2 as well as reduce its density, stability, and activity on the surface of the cell membrane (Heubl et al., 2017). Moreover, GABAAR blockers can also induce the phosphorylation of NKCC1 through the STE20/SPS1-related proline/alanine-rich kinase (SPAK) and oxidative stress-responsive kinase 1 (OSR1) pathway (Heubl et al., 2017). KCC2, NKCC1, and the GABA system affect the activity of the nervous system under a variety of physiological conditions, with intracellular Cl− concentrations serving as the intermediate link.
In addition to their function as ion transporters, KCC2 and NKCC1 also have cell-autonomous regulatory functions in the central nervous system, including the regulation of GABAAR and glycine receptor-mediated reversal potentials and anion currents (Blaesse et al., 2009), as well as physiological functions such as the regulation of cell volume, ion homeostasis, and growth (Olde Engberink et al., 2018; Demian et al., 2019). Studies have shown that the effects of NKCC1 and KCC2 on the regulation of cell osmotic pressure and cell volume are mediated through their ion transport functions (Chew et al., 2019). Meanwhile, KCC2 and NKCC1 also act as synchronization factors during the development of glutamatergic and GABAergic synapses in cortical neurons and their networks (Di Cristo et al., 2018). In addition, the importance of these two co-transporters in GABAergic signaling renders them valuable targets for nervous system drugs, such as those aimed at treating neuropathic pain, spasm, epilepsy, and motor dysfunction, among other conditions.
KCC2/NKCC1 and neurodevelopment
NKCC1 and KCC2 jointly regulate Cl− homeostasis in neurons and thus affect the function of the GABAergic system (Côme et al., 2020). In the early stages of development, because the expression level of KCC2 is relatively low and that of NKCC1 relatively high, intracellular Cl− concentrations remain at a relatively high level (Medina et al., 2014). Under this condition, the GABAAR can play an excitatory role by extruding Cl− from the cell (Medina et al., 2014). As the nervous system develops and matures, the concomitant increase in the expression of KCC2 results in a gradual reduction in the intracellular Cl− concentration, and the function of the GABAAR changes accordingly, which promotes Cl− influx, thereby exerting an inhibitory role (Côme et al., 2019). It has been demonstrated that if the spinal cord is transected during development, the expression of KCC2 is not up-regulated, and the transition of GABA function does not occur (Jean-Xavier et al., 2006). This suggests that the transition of GABA function from depolarizing to hyperpolarizing is related to the maturation of relevant spinal cord pathways. Notably, although it is generally assumed that NKCC1 is downregulated and KCC2 up-regulated during neuronal development, some existing data do not support this generalization (Blaesse et al., 2009). For instance, it cannot be excluded that a relatively small pool of NKCC1 protein in the plasma membrane of immature neurons may be sufficient to exert a dominant effect on the GABA system (Löscher et al., 2013). Additionally, the marked upregulation of KCC2 during development may be accompanied by a substantial increase in ionic conductivity and neuronal growth, which can mask the effect of NKCC1, even if the expression of the latter remains unchanged (Loscher et al., 2013). The transition of GABA function is related to the phosphorylation of serine 940 (Ser-940, S940), an important site for KCC2 activation (Leonzino et al., 2016). In parallel to the up-regulating signaling pathways, there are two threonine residues, Thr-906 and Thr-1007, on the intracellular C-terminal domain of KCC2 that strongly decrease KCC2 activity when phosphorylated (Schulte et al., 2018). Specifically blocking the function of KCC2 can induce a polarity change in GABAergic responses from inhibitory to excitatory. During development, With-No-Lysine kinase 1 (WNK1) -dependent regulation of KCC2 and NKCC1 phosphorylation levels is also an essential basis for the functional transformation of GABA (Gagnon and Delpire, 2010; Lee et al., 2011). WNK is mainly affected by intracellular Cl− concentration and may play a regulatory role on KCC2 and NKCC1 through the oxidative stress-responsive gene 1/Ste20-related proline-alanine-rich kinase (OSR1/SPAK) pathway (Friedel et al., 2015). Combined, these findings suggest that the KCC2 and NKCC1 functions may play a critical role in the change of GABA function during development (Olde Engberink et al., 2018).
NMDA receptor (N-methyl-D-aspartic acid receptor) is a subtype of ionic glutamate receptor; its role in neuroplasticity and excitotoxicity has received increasing attention (Vyklicky et al., 2014). In vitro and in vivo studies revealed that NMDA receptor activity-mediated protein phosphatase 1 (PP1) can inhibit the function of NKCC1 and upstream factors by regulating their dephosphorylation (Gagnon and Delpire, 2010), and the dynamic balance between PP1-dependent dephosphorylation of SER940 at the key site of KCC2 and regulation of protein kinase C (PKC) is an important basis for KCC2 to ensure physiological functions (Lee et al., 2011).
Growth factors play an indispensable role in the construction of neural networks during neural development and regeneration. For instance, brain-derived neurotrophic factor (BDNF) is a key regulator of axon growth, synapse formation, intersynaptic signal transmission, and synaptic plasticity (Wardle and Poo, 2003; Garraway and Huie, 2016; Lee-Hotta et al., 2019). The activation of BDNF affects the levels of intracellular Cl−, which, in turn, affects the function of GABA receptors in various physiological processes in the central nervous system (Zhou et al., 2021). The downstream signaling of Tyrosine Kinase receptor B (TrkB), a major receptor for BDNF, mainly involves 1) Mitogen-activated protein kinases/extracellular regulated kinase (MAPK/ERK) (Uvarov et al., 2006; Revest et al., 2014); 2) Extracellular regulated kinase/Early Growth Response 4 (ERK/Egr4) (Ludwig et al., 2011a; Ludwig et al., 2011b); and 3) Phospholipase C gamma (PLCγ)/FRS-2 (Rivera et al., 2004) (as shown in Figure 2). Among these three downstream signaling pathways, Egr4 is a transcription factor on the promoter of KCC2, which may lead to the upregulation of KCC2 mRNA after its translation and expression (Ludwig et al., 2011a; Ludwig et al., 2011b). Activation of PLCγ seems to be the key to the regulation of KCC2 by the BDNF-TrkB pathway. When PLCγ increases, BDNF inhibits KCC2 expression, while the opposite effect occurs when PLCγ activation is depressed. After SCI, the PLCγ cascade of the BDNF-TrkB pathway is enhanced, thereby repressing KCC2 mRNA transcription (Rivera et al., 2004; Tashiro et al., 2015).
BDNF is a crucial regulator of KCC2.In mature neurons, the effect of BDNF on KCC2 is inhibited. However, after SCI, the effect of BDNF on KCC2 turns into upregulated. It is may be caused due to a reversal way that BDNF affects KCC2 (Boulenguez et al., 2010). Previous studies have shown that exercise therapy such as treadmill training may endogenously increase the activation of the BDNF-TrkB pathway on injured neurons. Then, activating downstream signaling pathways and regulating KCC2/NKCC1 expression, and effectively improve the spasticity and pain after SCI (Tashiro et al., 2015). And this effect can be inhibited by the BDNF blocker TrkB-IgG, suggesting that activation of BDNF is critical to relief from spasticity and pain symptom after SCI (Bilchak et al., 2021).
Thus, BDNF may play opposing roles in the intact, mature nervous system and the injured, immature nervous system (Huang et al., 2017). There is currently no strong evidence indicating that BDNF directly affects NKCC1 expression. How to further explore this dual role of BDNF and apply it to rehabilitation after SCI requires further investigation.
In conclusion, as shown in Figures 1, 2, the regulatory effect of BDNF on the KCC2 and GABAergic systems differs according to the developmental stage and physiological condition (Tashiro et al., 2015; Heubl et al., 2017; Ma et al., 2021). In the mature nervous system, the expression level of KCC2 is high, and GABA can reduce the excitability of the system (Heubl et al., 2017). Under this condition, exogenous BDNF application will downregulate the expression of KCC2 (Huang et al., 2017). However, when the nervous system is immature or SCI occurs, the expression level of KCC2 is low, and GABA plays an excitatory role. Here, BDNF application will increase the expression level of KCC2 and restore the inhibitory effect of GABA.
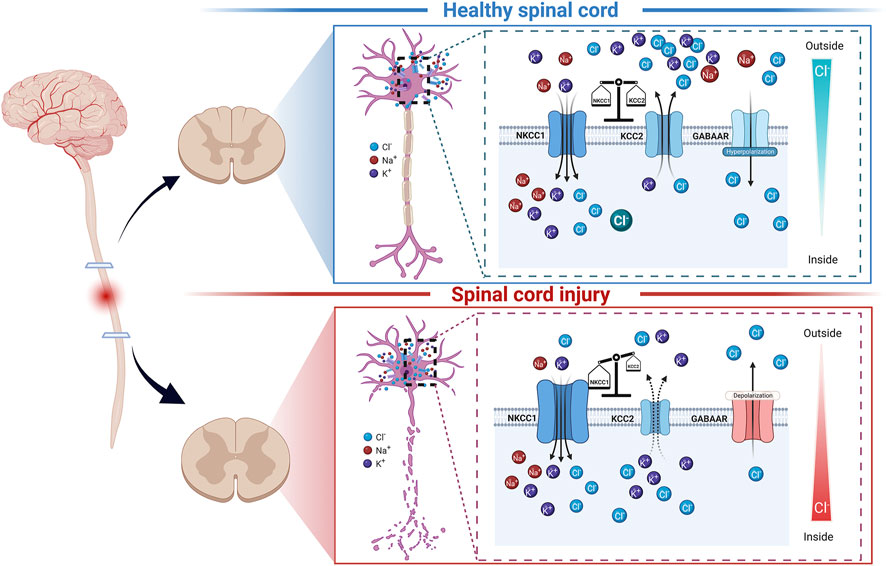
FIGURE 1. Schematic diagram of NKCC1, KCC2, GABAARs, and chloride ion homeostasis in spinal cord neurons under normal conditions and after spinal cord injury. In the mature, healthy spinal cord, the expression of NKCC1 and KCC2 is relatively balanced (top panel). Under this condition, the intracellular chloride ion level is low. GABAARs can play an inhibitory role by transporting chloride ions into cells. When spinal cord injury occurs (bottom panel), the expression of NKCC1 and KCC2 in the segment below the injury is dysregulated under the action of various factors, leading to high intracellular chloride ion levels. In this case, the GABAARs can play an inhibitory role by excluding chloride ions from cells.
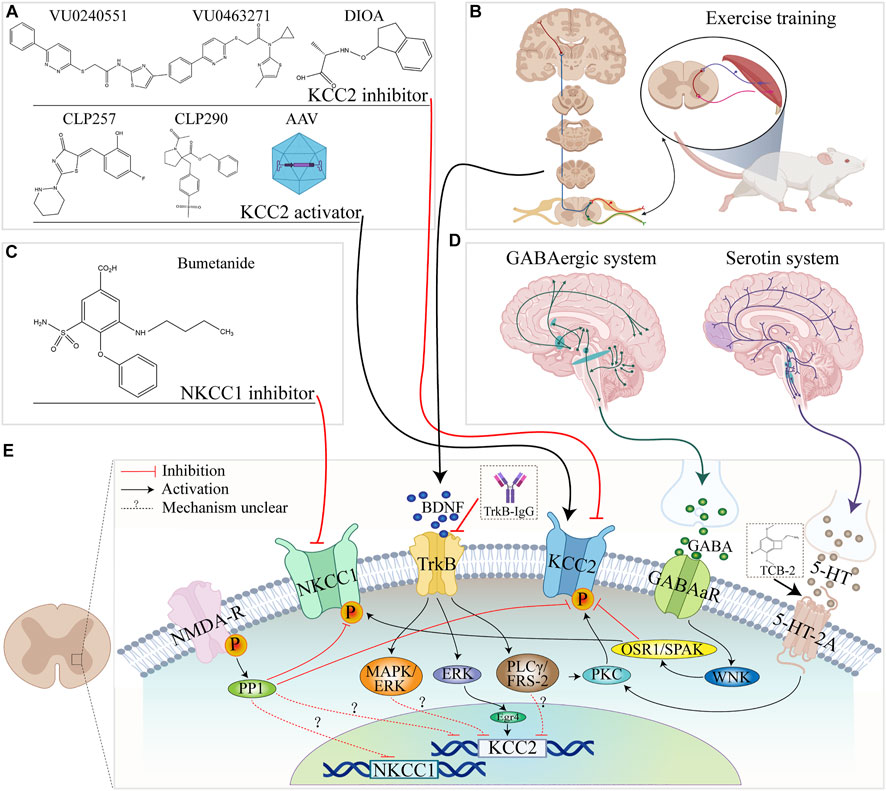
FIGURE 2. Summary of NKCC1 and KCC2 regulatory pathways in the spinal cord and related pathways. (A) The molecular formulae of commonly used KCC2 activators and inhibitors. (B) Exercise training. (C) Molecular formula of the NKCC1 inhibitor bumetanide. (D) Brain origin of 5-HT and GABAergic systems. (E) Schematic diagram of the relationship between KCC2/NKCC1 and upstream factors, channels, and pathways in spinal cord.
KCC2/NKCC1 and spinal cord injury
After SCI, the segment below the site of injury presents a state similar to that seen in the early stages of development (Huang et al., 2017). KCC2 and NKCC1 are reportedly involved in the pathophysiology of SCI, including the appearance of spasticity and neuropathic pain, through a variety of pathways (Boulenguez et al., 2010; Hasbargen et al., 2010). Damage to the descending serotonin (5-HT) fibers after SCI is closely related to the transformation of GABAergic interneuron function and KCC2/NKCC1 may be the common link between the two regulatory imbalances after SCI (Huang and Grau, 2018; Sánchez-Brualla et al., 2018; Tang, 2020). BDNF can also directly participate in this process upstream of KCC2/NKCC1 (Garraway and Huie, 2016; Ma et al., 2021). The treatment of SCI by directly or indirectly regulating KCC2/NKCC1 activity represents a potential strategy for the treatment of SCI, as summarized in Table1 and Figure 2.
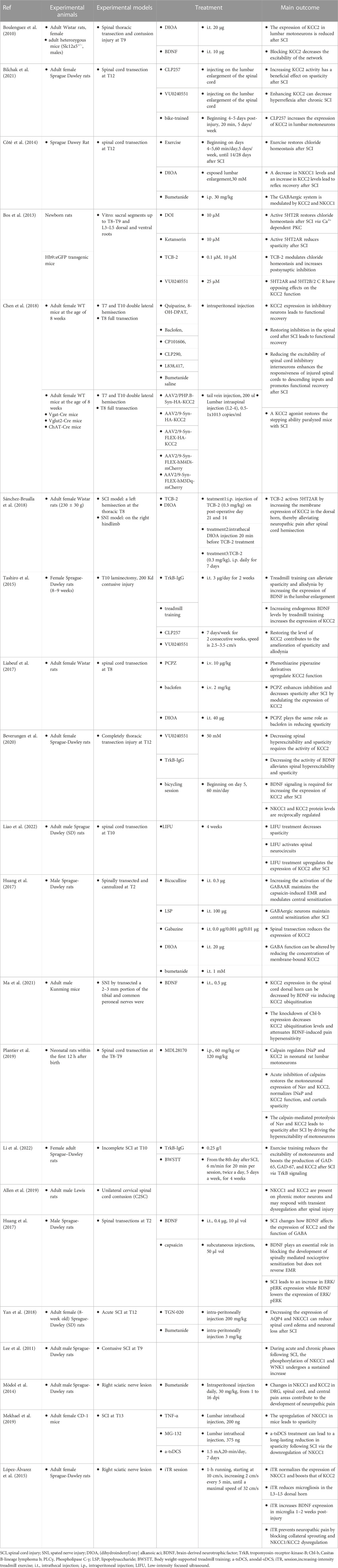
TABLE 1. Summary of animal experiments related to the regulation of KCC2 and NKCC1 after spinal cord injury.
KCC2/NKCC1 and neuropathic pain after spinal cord injury
Neuropathic pain is a common complication after SCI and occurs in approximately 60%–80% of affected patients (Finnerup et al., 2001). Neuropathic pain has a severe social and psychological impact on patients with SCI, and is closely related to their poor overall health, poor quality of life, and high levels of depression (Anderson, 2004; Widerström-Noga, 2017). Pain after SCI is challenging to manage. Approximately 2/3 of the patients do not have a suitable treatment plan, especially for severe neuropathic pain. The latter is a strong predictor of a decline in quality of life after SCI, imposing a heavy burden on both families and society (Burke et al., 2017). Owing to the heterogeneity of etiology, differences in genetic susceptibility, and differences in environmental factors, it is difficult to predict which patients will have neuropathic pain and how patients will respond to specific therapeutic drugs (Kahle et al., 2014). The incomplete understanding of the molecular mechanism underlying neuropathic pain hinders the development of targeted interventions, which underscores the need to identify and develop novel therapeutic strategies for the treatment of this condition, particularly at the molecular level (Shiao and Lee-Kubli, 2018). The KCC2-/NKCC1- mediated regulation of chloride homeostasis has potential as a molecular target for the treatment of neuropathic pain after SCI (Cramer et al., 2008).
Changes in the levels of KCC2 and NKCC1, key factors in the maintenance of Cl− homeostasis, have been implicated in the process of neuropathic pain after SCI (as shown in Figure 1) (Hasbargen et al., 2010; Fakhri et al., 2021). The expression of KCC2 was reported to be downregulated at the site of injury, accompanied by a transient and significant upregulation of NKCC1 expression, and this altered expression trend was consistent with the occurrence of post-neuropathic pain (Cramer et al., 2008). In addition, studies have found that the shift in NKCC1 and KCC2 expression in the spinal cord is also an important intermediate link in the development of neuropathic pain resulting from peripheral nerve injury (Coull et al., 2003; Mòdol et al., 2014; López-Álvarez et al., 2015).
GABAARs are involved in the regulation of the tonic-inhibitory effect in the dorsal horn of the spinal cord, maintaining the relative balance of inhibitory and excitatory systems in the central nervous network (Sivilotti and Woolf, 1994). After SCI, the function of GABAARs also changes to some extent, and their activation can produce depolarizing (excitatory) effects as well as promote the emergence of nociceptive sensitization (Huang and Grau, 2018). Pain sensitivity is reduced in rats with SCI when they are pretreated with GABAAR antagonists before the induction of SCI (Cramer et al., 2008). Similar treatments increase the sensitivity to nociceptive responses in healthy rats (Sorkin et al., 1998). Applying the GABAAR agonist muscimol to the spinal cord prevents thermal hyperalgesia after peripheral nerve injury (Miletic et al., 2003). Transplantation of GABAergic neuron precursors into the dorsal horn also reduces neuropathic pain (Bráz et al., 2012). However, existing GABAAR modulators, such as benzodiazepines or GABAAR agonists, are rarely used to treat neuropathic pain because of their narrow therapeutic window and associated adverse effects, such as sedation and hearing impairment (Kahle et al., 2014). Therefore, correcting the abnormal Cl− concentration gradient in the dorsal horn of the spinal cord by targeting KCC2 and NKCC1 represents a promising therapeutic direction for restoring the inhibitory function of the GABAergic system and ultimately relieving or improving neuropathic pain.
The role of BDNF in neuropathic pain after SCI has also been intensively studied given that it functions upstream of KCC2. Inflammation or nerve injury can inhibit the expression and function of KCC2 in the dorsal horn of the spinal cord through the BDNF/TrkB signaling pathway and promote the occurrence and development of neuropathic pain (Rivera et al., 2002; Miletic and Miletic, 2008). Promoting BDNF expression in normal adult rats can downregulate the level of membrane-bound KCC2 and reduce the inhibitory effect of the GABAergic system (Huang et al., 2017). Meanwhile, promoting BDNF expression in rats with transected spinal cords can enhance the originally attenuated GABAergic inhibitory effect, which, in turn, can increase the expression of KCC2 and relieve hyperalgesia, which may associate with BDNF-/TRKB-induced upregulation of KCC2 expression (Wenner, 2014; Huang et al., 2017; Sanchez-Brualla et al., 2018). In a rat model of neuropathic pain, the administration of neuroheal was found to alter the sensory signal input of the dorsal root ganglia through the P2X4-BDNF-KCC2 pathway, thereby reducing neuropathic pain (Romeo-Guitart and Casas, 2020). Moreover, it has been reported that the effect of BDNF on pain may be mediated via the regulation of the ubiquitination level of KCC2 in the posterior horn of the spinal cord (Ma et al., 2021).
In addition to impaired motor and sensory pathways, 5-HT axons originating from the brainstem are often also impaired in function and display reduced receptor expression after SCI, leading to neural circuit reorganization (Khalki et al., 2018). Damaged 5-HT neurons also play a part in the process of chronic pain, spasms, and autonomic hyperreflexia after SCI (Fauss et al., 2022). The 5HT2A/2B/2C-specific agonist DOI can restore chloride homeostasis through the Ca2+-dependent PKC pathway (Bos et al., 2013). Meanwhile, specific activators of the 5-HT2A receptor can exert an analgesic effect by enhancing the expression of KCC2 in spinal dorsal peduncle neurons (Sánchez-Brualla et al., 2018).
Transient receptor potential vanilloid 1 (TRPV1) is an important protein in the perception of pain (Takayama et al., 2015) and its expression in the dorsal horn of the spinal cord is an essential link in the emergence of neuropathic pain after SCI (Wu et al., 2013; Lee et al., 2021). The regulation of NKCC1 expression mediated by TRPV1 through the activation of the PKC/phosphorylated extracellular signal-regulated kinase (pERK) signaling pathway may be the mechanism underlying its involvement in SCI-related neuropathic pain (Deng et al., 2021).
KCC2/NKCC1 and spasticity after spinal cord injury
The plasticity of nerves after SCI not only engenders beneficial changes in structure and function in the spinal cord but also leads to the appearance of spasticity, which occurs in approximately 60%–80% of affected patients (Finnerup, 2017). Spasticity after SCI can also lead to chronic pain and deformity of the musculoskeletal system, which subsequently affect the mood, sleep, quality of life, cognitive function, and recreational activities of patients, and can also greatly affect their rehabilitation (Andresen et al., 2016; Holtz et al., 2017). Common treatments for spasticity include drugs such as baclofen, chemical neurolysis, botulinum toxin injection, surgery, and electrical stimulation, all of which have drawbacks and differences in efficacy (Walter et al., 2002; Elbasiouny et al., 2010). Spasticity after SCI may share some afferent pathways with neuropathic pain; however, owing to differences in symptoms, studies on neuropathic pain focus more on sensory neurons in the superficial dorsal horn of the spinal cord, while investigations relating to spasticity focus more on motor neurons in the anterior horn. Therefore, its chloride homeostasis is mainly regulated by KCC2 (Côté, 2020).
Disruption of chloride homeostasis after SCI, especially the downregulation of KCC2 in motor neuron membranes, depolarizes the Cl− equilibrium potential and reduces the strength of postsynaptic inhibition (Boulenguez et al., 2010). In healthy rats, pharmacological blockade of KCC2 can reduce the rate-dependent inhibition of the Hoffman reflex (rate-dependent depression, RDD) and produce spasticity like that seen in rats with SCI. Improvements in spasticity-like symptoms have also been reported (Boulenguez et al., 2010). When a KCC2 activator was administered to rats with spinal cord lesions, it was found that the expression of KCC2 was up-regulated in the membranes of motor neurons of the lumbar enlargement, and the spasticity symptoms were alleviated (Bilchak et al., 2021). Body Weight-Supported Treadmill training can also up-regulate KCC2 through the trkB pathway, regulating spinal cord excitability and reducing spasticity (Li et al., 2022). Additionally, (Mekhael et al., 2019), reported that transspinal direct current stimulation in injured mice can reduce spasticity and promote motor function recovery, and that this effect may be related to the downregulation of NKCC1, but not the upregulation of KCC2.
An increase in BDNF expression through exercise followed by the remodeling of KCC2 function was found to be an effective means of improving spasticity symptoms after SCI (Tashiro et al., 2015; Côté et al., 2014; Beverungen et al., 2020). The overexpression of the neurotrophic factors Neurotrofin-3 (NT-3), and Insulin-like growth factor 1 (IGF-1) has also been suggested as a treatment for spasticity after SCI, and their efficacy was speculated to be related to the functional remodeling of KCC2 (Chang et al., 2019; Talifu et al., 2022). In contrast to that found in the neuropathic pain model after SCI, Ryu et al. reported that an increase in the levels of 5-HT2A after SCI did not significantly affect spasticity symptoms or KCC2 expression in motor neurons (Ryu et al., 2021).
When TCB-2 is used to specifically activate the 5-HT2A receptor, it can also be stabilized by KCC2-mediated chloride ion, relieving spasticity after SCI (Bos et al., 2013). Although the downregulation of KCC2 begins within 24 h after SCI, spasticity only appear weeks or even months later, possibly because of the presence of persistent inward currents (PICs), PICs require prolonged depolarization to be fully activated and are inhibited by hyperpolarization (Li et al., 2004; Bras and Liabeuf, 2021). The depolarization of excitatory postsynaptic potentials (EPSPs) following KCC2 downregulation may allow sensory input-evoked depolarization to activate PICs and these may fail to inactivate owing to the inability to hyperpolarize, which ultimately induces spasticity (Li et al., 2004; Bras and Liabeuf, 2021). In a neonatal rat model of SCI, it was also found that calpain can up-regulate the sustained sodium ion current in motor neurons and downregulate KCC2 expression after SCI, resulting in the appearance of spasticity (Plantier et al., 2019). Combined, these observations suggest that the downregulation of KCC2 and the upregulation of PICs may play a synergistic role in the pathophysiology of spasticity.
In brief, the downregulation of KCC2 expression below the injury site after SCI, especially in motor neuron membranes, can depolarize the intracellular membrane potential and exert excitatory effects, and may represent an important component in the occurrence of spasticity (Boulenguez et al., 2010; Bilchak et al., 2021). BDNF, exercise training, or regulation of neurotrophic factor levels can improve spasticity by promoting KCC2 expression (Tashiro et al., 2015; Ma et al., 2021; Talifu et al., 2022).
KCC2 and motor function after spinal cord injury
Substantial evidence supports the use of exercise training in current rehabilitation regimes for the functional recovery of spinal cord injuries (Harvey, 2016). The restoration of chloride homeostasis mediated by NKCC1 and KCC2 may be an important physiological basis for the associated improvement in spinal cord functions (Côté et al., 2014).
The regulation of KCC2 has a unique role in the recovery of motor function after SCI, making this co-transporters an important target for motor reconstruction in the spinal cord following injury (Chen et al., 2018). The administration of the KCC2-specific activator CLP290 could significantly restore the coordinated step function of mice that had completely lost descending control; similar functional recovery was also achieved by adeno-associated virus-mediated overexpression of KCC2 (Chen et al., 2018). However, the authors also indicated that KCC2 promotes the recovery of motor function, but in a manner different from that involved in the relief of spasticity and neuropathic pain, that is, promoting the expression of KCC2 in inhibitory interneurons is the key to the reconstruction of motor function (Chen et al., 2018). Increased KCC2 expression can also be achieved by overexpressing BDNF; however, this approach may have complications, such as spasticity, resulting from motor neuron hyperexcitation (Ziemlińska et al., 2014). The positive and quantitative regulation of KCC2 to promote the recovery of motor function may become the focus of research for SCI rehabilitation.
At present, there is no convincing evidence regarding the existence of a relationship between NKCC1 expression and motor function after SCI. In animal models of brain injury, the long-term administration of the NKCC1-specific inhibitor bumetanide in rats can enhance axonal bud growth after focal cerebral ischemia. In addition, bumetanide treatment can up-regulate KCC2 and BDNF levels, downregulate those of NKCC1, and improve exercise-related behavior in rats after stroke (Mu et al., 2017). Another report also showed that bumetanide improves sensory and motor recovery after traumatic brain injury (Zhang et al., 2017). The results of these studies suggested that in animal models of traumatic SCI, the downregulation of NKCC1 levels may also have the potential to improve neurological outcomes, although this effect may be achieved indirectly through the modulation of chloride homeostasis. Endogenous serotonin levels may also affect the alternate movement pattern of lower limbs by affecting chloride ion homeostasis and the GABAergic system, which is also a potential target for motor reconstruction after SCI (Tang, 2020).
Conclusion
Numerous basic studies have confirmed the notable therapeutic potential of NKCC1 and KCC2 in neuropathic pain, spasticity, and motor function recovery after SCI, and these co-transporters are expected to become key targets in future SCI treatment (Boulenguez et al., 2010; Chen et al., 2018).
To achieve this, key pathways are blocked/activated by pharmacological means, such as the P2X4-BDNF-KCC2 pathway (Ferrini et al., 2013; Romeo-Guitart and Casas, 2020), BDNF-trkb (Miletic and Miletic, 2008; Rivera et al., 2002; Rivera et al., 2004; Huang et al., 2017; Wenner, 2014; Sánchez-Brualla et al., 2018) or direct regulation of KCC2 activity, such as N and C termin (Ferrini et al., 2013) N-terminal loop Chi et al., 2021, phosphorylation of serine 940 (Leonzino et al., 2016) will likely be potential targets for clinical treatment of spinal cord injury and its complications. In addition, through exercise training, modulation of the 5-HTergic system (Bos et al., 2013; Sánchez-Brualla et al., 2018; Ryu et al., 2021), the GABAergic system, and TRPV1 (Deng et al., 2021) to indirectly affect NKCC1 and KCC2 expression levels are also feasible intervention strategies. The targeted regulation of different types of neurons may become a future treatment strategy for SCI and its complications (Chen et al., 2018). As KCC2 and NKCC1 are widely distributed in the nervous system, how to achieve localization, orientation, and quantitative regulation of their levels may be the biggest obstacle to their clinical application in the treatment of SCI. It is necessary to solve these problems through more in-depth and comprehensive research for the benefit of patients with SCI.
Author contributions
Design and concepts: LD and JL; definition of intellectual content: DY, FG, and YY; literature search: ZT, YP, GH, XX, and CZ; data acquisition and tabulation: ZT and GH; manuscript preparation: ZT and YP; Mechanism drawing: ZT; manuscript editing: DY, FG, and YY; manuscript review: LD and JL. All authors approved the final version of the manuscript.
Funding
This study was funded by Supported by Beijing Municipal Natural Science Foundation (7212140), Basic Scientific Research of the Central Public Research Institutes in China (2019CZ-9, 2021CZ-2), Special fund for joint training of doctoral students between university of health and rehabilitation sciences and China Rehabilitation Research Center (2020kfdx-004, 2020kfdx-009), the Scientific Research Foundation of China Rehabilitation Research Center (2022ZX-18).
Acknowledgments
We thank Charlesworth Author Services (https://www.cwauthors.com.cn/) for its linguistic assistance during the preparation of this manuscript. We thank BioRender.com for the support of the diagram drawing.
Conflict of interest
The authors declare that the research was conducted in the absence of any commercial or financial relationships that could be construed as a potential conflict of interest.
Publisher’s note
All claims expressed in this article are solely those of the authors and do not necessarily represent those of their affiliated organizations, or those of the publisher, the editors and the reviewers. Any product that may be evaluated in this article, or claim that may be made by its manufacturer, is not guaranteed or endorsed by the publisher.
References
Agez M., Schultz P., Medina I., Baker D. J., Burnham M. P., Cardarelli R. A., et al. (2017). Molecular architecture of potassium chloride co-transporter KCC2. Sci. Rep. 7, 16452. doi:10.1038/s41598-017-15739-1
Ahuja C. S., Nori S., Tetreault L., Wilson J., Kwon B., Harrop J., et al. (2017). Traumatic spinal cord injury-repair and regeneration. Neurosurgery 80, S9–s22. doi:10.1093/neuros/nyw080
Allen L. L., Seven Y. B., Baker T. L., Mitchell G. S. (2019). Cervical spinal contusion alters Na(+)−K(+)−2Cl− and K(+)−Cl− cation-chloride cotransporter expression in phrenic motor neurons. Respir Physiol Neurobiol, 261, 15–23.
Anderson K. D. (2004). Targeting recovery: Priorities of the spinal cord-injured population. J. Neurotrauma 21, 1371–1383. doi:10.1089/neu.2004.21.1371
Andresen S. R., Biering-SøRENSEN F., Hagen E. M., Nielsen J. F., Bach F. W., Finnerup N. B. (2016). Pain, spasticity and quality of life in individuals with traumatic spinal cord injury in Denmark. Spinal Cord. 54, 973–979. doi:10.1038/sc.2016.46
Arroyo J. P., Kahle K. T., Gamba G. (2013). The SLC12 family of electroneutral cation-coupled chloride cotransporters. Mol. Asp. Med. 34, 288–298. doi:10.1016/j.mam.2012.05.002
Ben-Ari Y. (2002). Excitatory actions of gaba during development: The nature of the nurture. Nat. Rev. Neurosci. 3, 728–739. doi:10.1038/nrn920
Beverungen H., Klaszky S. C., Klaszky M., CôTé M. P. (2020). Rehabilitation decreases spasticity by restoring chloride homeostasis through the brain-derived neurotrophic factor-KCC2 pathway after spinal cord injury. J. Neurotrauma 37, 846–859. doi:10.1089/neu.2019.6526
Bilchak J. N., Yeakle K., Caron G., Malloy D., CôTé M. P. (2021). Enhancing KCC2 activity decreases hyperreflexia and spasticity after chronic spinal cord injury. Exp. Neurol. 338, 113605. doi:10.1016/j.expneurol.2021.113605
Blaesse P., Airaksinen M. S., Rivera C., Kaila K. (2009). Cation-chloride cotransporters and neuronal function. Neuron 61, 820–838. doi:10.1016/j.neuron.2009.03.003
Bos R., Sadlaoud K., Boulenguez P., Buttigieg D., Liabeuf S., Brocard C., et al. (2013). Activation of 5-HT2A receptors upregulates the function of the neuronal K-Cl cotransporter KCC2. Proc. Natl. Acad. Sci. U. S. A. 110, 348–353. doi:10.1073/pnas.1213680110
Boulenguez P., Liabeuf S., Bos R., Bras H., Jean-Xavier C., Brocard C., et al. (2010). Down-regulation of the potassium-chloride cotransporter KCC2 contributes to spasticity after spinal cord injury. Nat. Med. 16, 302–307. doi:10.1038/nm.2107
Bras H., Liabeuf S. (2021). Differential effects of spinal cord transection on glycinergic and GABAergic synaptic signaling in sub-lesional lumbar motoneurons. J. Chem. Neuroanat. 113, 101847. doi:10.1016/j.jchemneu.2020.101847
BráZ J. M., Sharif-Naeini R., Vogt D., Kriegstein A., Alvarez-Buylla A., Rubenstein J. L., et al. (2012). Forebrain GABAergic neuron precursors integrate into adult spinal cord and reduce injury-induced neuropathic pain. Neuron 74, 663–675. doi:10.1016/j.neuron.2012.02.033
Burke D., Fullen B. M., Stokes D., Lennon O. (2017). Neuropathic pain prevalence following spinal cord injury: A systematic review and meta-analysis. Eur. J. Pain 21, 29–44. doi:10.1002/ejp.905
Chamma I., Chevy Q., Poncer J. C., LéVI S. (2012). Role of the neuronal K-Cl co-transporter KCC2 in inhibitory and excitatory neurotransmission. Front. Cell. Neurosci. 6, 5. doi:10.3389/fncel.2012.00005
Chang Y. X., Zhao Y., Pan S., Qi Z. P., Kong W. J., Pan Y. R., et al. (2019). Intramuscular injection of adenoassociated virus encoding human neurotrophic factor 3 and exercise intervention contribute to reduce spasms after spinal cord injury. Neural Plast. 2019, 3017678. doi:10.1155/2019/3017678
Chen B., Li Y., Yu B., Zhang Z., Brommer B., Williams P. R., et al. (2018). Reactivation of dormant relay pathways in injured spinal cord by KCC2 manipulations. Cell 174, 521–535. e13. doi:10.1016/j.cell.2018.06.005
Chew T., Orlando B., Zhang J., Latorraca N., Wang A., Hollingsworth S., et al. (2019). Structure and mechanism of the cation-chloride cotransporter NKCC1. Nature 572, 488–492. doi:10.1038/s41586-019-1438-2
Cieza A., Causey K., Kamenov K., Hanson S. W., Chatterji S., Vos T. (2021). Global estimates of the need for rehabilitation based on the global burden of disease study 2019: A systematic analysis for the global burden of disease study 2019. Lancet 396, 2006–2017. doi:10.1016/S0140-6736(20)32340-0
CôME E., Heubl M., Schwartz E. J., Poncer J. C., LéVI S. (2019). Reciprocal regulation of KCC2 trafficking and synaptic activity. Front. Cell. Neurosci. 13, 48. doi:10.3389/fncel.2019.00048
CôME E., Marques X., Poncer J. C., LéVI S. (2020). KCC2 membrane diffusion tunes neuronal chloride homeostasis. Neuropharmacology 169, 107571. doi:10.1016/j.neuropharm.2019.03.014
CôTé M.-P. (2020). “Chapter 18 - role of chloride cotransporters in the development of spasticity and neuropathic pain after spinal cord injury,” in Neuronal chloride transporters in health and disease. Editor X. TANG (United States: Academic Press).
CôTé M. P., Gandhi S., Zambrotta M., Houlé J. D. (2014). Exercise modulates chloride homeostasis after spinal cord injury. J. Neurosci. 34, 8976–8987. doi:10.1523/JNEUROSCI.0678-14.2014
Coull J. A., Boudreau D., Bachand K., Prescott S. A., Nault F., SíK A., et al. (2003). Trans-synaptic shift in anion gradient in spinal lamina I neurons as a mechanism of neuropathic pain. Nature 424, 938–942. doi:10.1038/nature01868
Cramer S. W., Baggott C., Cain J., Tilghman J., Allcock B., Miranpuri G., et al. (2008). The role of cation-dependent chloride transporters in neuropathic pain following spinal cord injury. Mol. Pain 4, 36. doi:10.1186/1744-8069-4-36
Demian W., Persaud A., Jiang C., Coyaud É., Liu S., Kapus A., et al. (2019). The ion transporter NKCC1 links cell volume to cell mass regulation by suppressing mTORC1. Cell Rep. 27, 1886–1896. doi:10.1016/j.celrep.2019.04.034
Deng S. Y., Tang X. C., Chang Y. C., Xu Z. Z., Chen Q. Y., Cao N., et al. (2021). Improving NKCC1 function increases the excitability of DRG neurons exacerbating pain induced after TRPV1 activation of primary sensory neurons. Front. Cell. Neurosci. 15, 665596. doi:10.3389/fncel.2021.665596
Di Cristo G., Awad P., Hamidi S., Avoli M. (2018). KCC2, epileptiform synchronization, and epileptic disorders. Prog. Neurobiol. 162, 1–16. doi:10.1016/j.pneurobio.2017.11.002
Elbasiouny S. M., Moroz D., Bakr M. M., Mushahwar V. K. (2010). Management of spasticity after spinal cord injury: Current techniques and future directions. Neurorehabil. Neural Repair 24, 23–33. doi:10.1177/1545968309343213
Fakhri S., Abbaszadeh F., Jorjani M. (2021). On the therapeutic targets and pharmacological treatments for pain relief following spinal cord injury: A mechanistic review. Biomed. Pharmacother. 139, 111563. doi:10.1016/j.biopha.2021.111563
Fauss G. N. K., Hudson K. E., Grau J. W. (2022). Role of descending serotonergic fibers in the development of pathophysiology after spinal cord injury (SCI): Contribution to chronic pain, spasticity, and autonomic dysreflexia. Biol. (Basel) 11, 234. doi:10.3390/biology11020234
Ferrini F., Trang T., Mattioli T. A., Laffray S., Del'Guidice T., Lorenzo L. E., et al. (2013). Morphine hyperalgesia gated through microglia-mediated disruption of neuronal Cl⁻ homeostasis. Nat. Neurosci. 16, 183–192. doi:10.1038/nn.3295
Finnerup N. B., Johannesen I. L., Sindrup S. H., Bach F. W., Jensen T. S. (2001). Pain and dysesthesia in patients with spinal cord injury: A postal survey. Spinal Cord. 39, 256–262. doi:10.1038/sj.sc.3101161
Finnerup N. B. (2017). Neuropathic pain and spasticity: Intricate consequences of spinal cord injury. Spinal Cord. 55, 1046–1050. doi:10.1038/sc.2017.70
Friedel P., Kahle K. T., Zhang J., Hertz N., Pisella L. I., Buhler E., et al. (2015). WNK1-regulated inhibitory phosphorylation of the KCC2 cotransporter maintains the depolarizing action of GABA in immature neurons. Sci. Signal. 8, ra65. doi:10.1126/scisignal.aaa0354
Gagnon K. B., Delpire E. (2010). Multiple pathways for protein phosphatase 1 (PP1) regulation of Na-K-2Cl cotransporter (NKCC1) function: The N-terminal tail of the Na-K-2Cl cotransporter serves as a regulatory scaffold for ste20-related proline/alanine-rich kinase (SPAK) and PP1. J. Biol. Chem. 285, 14115–14121. doi:10.1074/jbc.M110.112672
Gamba G., Miyanoshita A., Lombardi M., Lytton J., Lee W., Hediger M., et al. (1994). Molecular cloning, primary structure, and characterization of two members of the mammalian electroneutral sodium-(potassium)-chloride cotransporter family expressed in kidney. J. Biol. Chem. 269, 17713–17722. doi:10.1016/s0021-9258(17)32499-7
Garraway S. M., Huie J. R. (2016). Spinal plasticity and behavior: BDNF-induced neuromodulation in uninjured and injured spinal cord. Neural Plast. 2016, 9857201. doi:10.1155/2016/9857201
GBD 2016 Neurology Collaborators (2019a). Global, regional, and national burden of neurological disorders, 1990-2016: A systematic analysis for the global burden of disease study 2016. Lancet. Neurol. 18, 459–480. doi:10.1016/S1474-4422(18)30499-X
GBD 2016 Traumatic Brain Injury and Spinal Cord Injury (2019b). Global, regional, and national burden of traumatic brain injury and spinal cord injury, 1990-2016: A systematic analysis for the global burden of disease study 2016. Lancet. Neurol. 18, 56–87. doi:10.1016/S1474-4422(18)30415-0
Haas M., Forbush B. (2000). The Na-K-Cl cotransporter of secretory epithelia. Annu. Rev. Physiol. 62, 515–534. doi:10.1146/annurev.physiol.62.1.515
Harvey L. A. (2016). Physiotherapy rehabilitation for people with spinal cord injuries. J. Physiother. 62, 4–11. doi:10.1016/j.jphys.2015.11.004
Hasbargen T., Ahmed M. M., Miranpuri G., Li L., Kahle K. T., Resnick D., et al. (2010). Role of NKCC1 and KCC2 in the development of chronic neuropathic pain following spinal cord injury. Ann. N. Y. Acad. Sci. 1198, 168–172. doi:10.1111/j.1749-6632.2010.05462.x
Heubl M., Zhang J., Pressey J. C., Al Awabdh S., Renner M., Gomez-Castro F., et al. (2017). GABA(A) receptor dependent synaptic inhibition rapidly tunes KCC2 activity via the Cl(-)-sensitive WNK1 kinase. Nat. Commun. 8, 1776. doi:10.1038/s41467-017-01749-0
Holtz K. A., Lipson R., Noonan V. K., Kwon B. K., Mills P. B. (2017). Prevalence and effect of problematic spasticity after traumatic spinal cord injury. Arch. Phys. Med. Rehabil. 98, 1132–1138. doi:10.1016/j.apmr.2016.09.124
Huang Y. J., Grau J. W. (2018). Ionic plasticity and pain: The loss of descending serotonergic fibers after spinal cord injury transforms how GABA affects pain. Exp. Neurol. 306, 105–116. doi:10.1016/j.expneurol.2018.05.002
Huang Y. J., Lee K. H., Grau J. W. (2017). Complete spinal cord injury (SCI) transforms how brain derived neurotrophic factor (BDNF) affects nociceptive sensitization. Exp. Neurol. 288, 38–50. doi:10.1016/j.expneurol.2016.11.001
HüBNER C. A., Stein V., Hermans-Borgmeyer I., Meyer T., Ballanyi K., Jentsch T. J. (2001). Disruption of KCC2 reveals an essential role of K-Cl cotransport already in early synaptic inhibition. Neuron 30, 515–524. doi:10.1016/s0896-6273(01)00297-5
Jaggi A. S., Kaur A., Bali A., Singh N. (2015). Expanding spectrum of sodium potassium chloride Co-transporters in the pathophysiology of diseases. Curr. Neuropharmacol. 13, 369–388. doi:10.2174/1570159x13666150205130359
Jean-Xavier C., Pflieger J. F., Liabeuf S., Vinay L. (2006). Inhibitory postsynaptic potentials in lumbar motoneurons remain depolarizing after neonatal spinal cord transection in the rat. J. Neurophysiol. 96, 2274–2281. doi:10.1152/jn.00328.2006
Kahle K. T., Khanna A., Clapham D. E., Woolf C. J. (2014). Therapeutic restoration of spinal inhibition via druggable enhancement of potassium-chloride cotransporter KCC2-mediated chloride extrusion in peripheral neuropathic pain. JAMA Neurol. 71, 640–645. doi:10.1001/jamaneurol.2014.21
Kahle K. T., Staley K. J., Nahed B. V., Gamba G., Hebert S. C., Lifton R. P., et al. (2008). Roles of the cation-chloride cotransporters in neurological disease. Nat. Clin. Pract. Neurol. 4, 490–503. doi:10.1038/ncpneuro0883
Kaila K., Price T. J., Payne J. A., Puskarjov M., Voipio J. (2014). Cation-chloride cotransporters in neuronal development, plasticity and disease. Nat. Rev. Neurosci. 15, 637–654. doi:10.1038/nrn3819
Khalki L., Sadlaoud K., Lerond J., Coq J. O., Brezun J. M., Vinay L., et al. (2018). Changes in innervation of lumbar motoneurons and organization of premotor network following training of transected adult rats. Exp. Neurol. 299, 1–14. doi:10.1016/j.expneurol.2017.09.002
Krystal A. D., Sutherland J., Hochman D. W. (2012). Loop diuretics have anxiolytic effects in rat models of conditioned anxiety. PLoS One 7, e35417. doi:10.1371/journal.pone.0035417
Lee H. H., Deeb T. Z., Walker J. A., Davies P. A., Moss S. J. (2011). NMDA receptor activity downregulates KCC2 resulting in depolarizing GABAA receptor-mediated currents. Nat. Neurosci. 14, 736–743. doi:10.1038/nn.2806
Lee H. H., Jurd R., Moss S. J. (2010). Tyrosine phosphorylation regulates the membrane trafficking of the potassium chloride co-transporter KCC2. Mol. Cell. Neurosci. 45, 173–179. doi:10.1016/j.mcn.2010.06.008
Lee J. H., Ji H., Ko S. G., Kim W. (2021). JI017 attenuates oxaliplatin-induced cold allodynia via spinal TRPV1 and astrocytes inhibition in mice. Int. J. Mol. Sci. 22, 8811. doi:10.3390/ijms22168811
Lee-Hotta S., Uchiyama Y., Kametaka S. (2019). Role of the BDNF-TrkB pathway in KCC2 regulation and rehabilitation following neuronal injury: A mini review. Neurochem. Int. 128, 32–38. doi:10.1016/j.neuint.2019.04.003
Leonzino M., Busnelli M., Antonucci F., Verderio C., Mazzanti M., Chini B. (2016). The timing of the excitatory-to-inhibitory GABA switch is regulated by the oxytocin receptor via KCC2. Cell Rep. 15, 96–103. doi:10.1016/j.celrep.2016.03.013
Li X., Song X., Fang L., Ding J., Qi L., Wang Q., et al. (2022). Body weight-supported treadmill training ameliorates motoneuronal hyperexcitability by increasing GAD-65/67 and KCC2 expression via TrkB signaling in rats with incomplete spinal cord injury. Neurochem. Res. 47, 1679–1691. doi:10.1007/s11064-022-03561-9
Li Y., Gorassini M. A., Bennett D. J. (2004). Role of persistent sodium and calcium currents in motoneuron firing and spasticity in chronic spinal rats. J. Neurophysiol. 91, 767–783. doi:10.1152/jn.00788.2003
Li Y., Song X., Fang L., Ding J., Qi L., Wang Q. (2022). Body Weight-Supported Treadmill Training Ameliorates Motoneuronal Hyperexcitability by Increasing GAD-65/67 and KCC2 Expression via TrkB Signaling in Rats with Incomplete Spinal Cord Injury. Neurochem Res 47, 1679–1691.
Liabeuf S., Stuhl-gourmand L., Gackière F., Mancuso R., Sanchez Brualla I., Marino P., et al. (2017). Prochlorperazine Increases KCC2 Function and Reduces Spasticity after Spinal Cord Injury. J Neurotrauma 34, 3397–3406.
Liao Y. H., Chen M. X., Chen S. C., Luo K. X., Wang B., Ao L. J. (2022). Low-Intensity Focused Ultrasound Alleviates Spasticity and Increases Expression of the Neuronal K-Cl Cotransporter in the L4-L5 Sections of Rats Following Spinal Cord Injury. Front Cell Neurosci 16, 882127.
LóPEZ-Álvarez V. M., Modol L., Navarro X., Cobianchi S. (2015). Early increasing-intensity treadmill exercise reduces neuropathic pain by preventing nociceptor collateral sprouting and disruption of chloride cotransporters homeostasis after peripheral nerve injury. Pain 156, 1812–1825. doi:10.1097/j.pain.0000000000000268
Loscher W., Puskarjov M., Kaila K. (2013). Cation-chloride cotransporters NKCC1 and KCC2 as potential targets for novel antiepileptic and antiepileptogenic treatments. Neuropharmacology 69, 62–74. doi:10.1016/j.neuropharm.2012.05.045
Ludwig A., Uvarov P., Pellegrino C., Thomas-Crusells J., Schuchmann S., Saarma M., et al. (2011a). Neurturin evokes MAPK-dependent upregulation of Egr4 and KCC2 in developing neurons. Neural Plast. 2011, 1–8. doi:10.1155/2011/641248
Ludwig A., Uvarov P., Soni S., Thomas-Crusells J., Airaksinen M. S., Rivera C. (2011b). Early growth response 4 mediates BDNF induction of potassium chloride cotransporter 2 transcription. J. Neurosci. 31, 644–649. doi:10.1523/JNEUROSCI.2006-10.2011
Ma J. J., Zhang T. Y., Diao X. T., Yao L., Li Y. X., Suo Z. W., et al. (2021). BDNF modulated KCC2 ubiquitylation in spinal cord dorsal horn of mice. Eur. J. Pharmacol. 906, 174205. doi:10.1016/j.ejphar.2021.174205
Markadieu N., Delpire E. (2014). Physiology and pathophysiology of SLC12A1/2 transporters. Pflugers Arch. 466, 91–105. doi:10.1007/s00424-013-1370-5
Markkanen M., Karhunen T., Llano O., Ludwig A., Rivera C., Uvarov P., et al. (2014). Distribution of neuronal KCC2a and KCC2b isoforms in mouse CNS. J. Comp. Neurol. 522, 1897–1914. doi:10.1002/cne.23510
Markkanen M., Ludwig A., Khirug S., Pryazhnikov E., Soni S., Khiroug L., et al. (2017). Implications of the N-terminal heterogeneity for the neuronal K-Cl cotransporter KCC2 function. Brain Res. 1675, 87–101. doi:10.1016/j.brainres.2017.08.034
Mcdonald J. W., Sadowsky C. (2002). Spinal-cord injury. Lancet 359, 417–425. doi:10.1016/S0140-6736(02)07603-1
Medina I., Friedel P., Rivera C., Kahle K. T., Kourdougli N., Uvarov P., et al. (2014). Current view on the functional regulation of the neuronal K(+)-Cl(-) cotransporter KCC2. Front. Cell. Neurosci. 8, 27. doi:10.3389/fncel.2014.00027
Mekhael W., Begum S., Samaddar S., Hassan M., Toruno P., Ahmed M., et al. (2019). Repeated anodal trans-spinal direct current stimulation results in long-term reduction of spasticity in mice with spinal cord injury. J. Physiol. 597, 2201–2223. doi:10.1113/JP276952
Miletic G., Draganic P., Pankratz M. T., Miletic V. (2003). Muscimol prevents long-lasting potentiation of dorsal horn field potentials in rats with chronic constriction injury exhibiting decreased levels of the GABA transporter GAT-1. Pain 105, 347–353. doi:10.1016/s0304-3959(03)00250-1
Miletic G., Miletic V. (2008). Loose ligation of the sciatic nerve is associated with TrkB receptor-dependent decreases in KCC2 protein levels in the ipsilateral spinal dorsal horn. Pain 137, 532–539. doi:10.1016/j.pain.2007.10.016
MòDOL L., Cobianchi S., Navarro X. (2014). Prevention of NKCC1 phosphorylation avoids downregulation of KCC2 in central sensory pathways and reduces neuropathic pain after peripheral nerve injury. Pain 155, 1577–1590. doi:10.1016/j.pain.2014.05.004
Mu X. P., Wang H. B., Cheng X., Yang L., Sun X. Y., Qu H. L., et al. (2017). Inhibition of Nkcc1 promotes axonal growth and motor recovery in ischemic rats. Neuroscience 365, 83–93. doi:10.1016/j.neuroscience.2017.09.036
O'Shea T. M., Burda J. E., Sofroniew M. V. (2017). Cell biology of spinal cord injury and repair. J. Clin. Invest. 127, 3259–3270. doi:10.1172/JCI90608
Olde Engberink A., Meijer J., Michel S. (2018). Chloride cotransporter KCC2 is essential for GABAergic inhibition in the SCN. Neuropharmacology 138, 80–86. doi:10.1016/j.neuropharm.2018.05.023
Payne J. A., Forbush B. (1995). Molecular characterization of the epithelial Na-K-Cl cotransporter isoforms. Curr. Opin. Cell Biol. 7, 493–503. doi:10.1016/0955-0674(95)80005-0
Payne J. A. (2012). Molecular operation of the cation chloride cotransporters: Ion binding and inhibitor interaction. Curr. Top. Membr. 70, 215–237. doi:10.1016/B978-0-12-394316-3.00006-5
Payne J. A., Stevenson T. J., Donaldson L. F. (1996). Molecular characterization of a putative K-Cl cotransporter in rat brain. A neuronal-specific isoform. J. Biol. Chem. 271, 16245–16252. doi:10.1074/jbc.271.27.16245
Pitcher M., Price T., Entrena J., Cervero F. (2007). Spinal NKCC1 blockade inhibits TRPV1-dependent referred allodynia. Mol. Pain 3, 17. doi:10.1186/1744-8069-3-17
Plantier V., Sanchez-Brualla I., Dingu N., Brocard C., Liabeuf S., GackièRE F., et al. (2019). Calpain fosters the hyperexcitability of motoneurons after spinal cord injury and leads to spasticity. Elife 8, e51404. doi:10.7554/eLife.51404
Pond B., Berglund K., Kuner T., Feng G., Augustine G., Schwartz-Bloom R. (2006). The chloride transporter Na(+)-K(+)-Cl- cotransporter isoform-1 contributes to intracellular chloride increases after in vitro ischemia. J. Neurosci. 26, 1396–1406. doi:10.1523/JNEUROSCI.1421-05.2006
Revest J. M., Le Roux A., Roullot-LacarrièRE V., Kaouane N., ValléE M., Kasanetz F., et al. (2014). BDNF-TrkB signaling through Erk1/2 MAPK phosphorylation mediates the enhancement of fear memory induced by glucocorticoids. Mol. Psychiatry 19, 1001–1009. doi:10.1038/mp.2013.134
Rivera C., Li H., Thomas-Crusells J., Lahtinen H., Viitanen T., Nanobashvili A., et al. (2002). BDNF-induced TrkB activation down-regulates the K+-Cl- cotransporter KCC2 and impairs neuronal Cl- extrusion. J. Cell Biol. 159, 747–752. doi:10.1083/jcb.200209011
Rivera C., Voipio J., Thomas-Crusells J., Li H., Emri Z., Sipilä S., et al. (2004). Mechanism of activity-dependent downregulation of the neuron-specific K-Cl cotransporter KCC2. J. Neurosci. 24, 4683–4691. doi:10.1523/JNEUROSCI.5265-03.2004
Romeo-Guitart D., Casas C. (2020). NeuroHeal treatment alleviates neuropathic pain and enhances sensory axon regeneration. Cells 9, 808. doi:10.3390/cells9040808
Russell J. M. (2000). Sodium-potassium-chloride cotransport. Physiol. Rev. 80, 211–276. doi:10.1152/physrev.2000.80.1.211
Ryu Y., Ogata T., Nagao M., Sawada Y., Nishimura R., Fujita N. (2021). Early escitalopram administration as a preemptive treatment strategy against spasticity after contusive spinal cord injury in rats. Sci. Rep. 11, 7120. doi:10.1038/s41598-021-85961-5
Sanchez-Brualla I., Boulenguez P., Brocard C., Liabeuf S., Viallat-Lieutaud A., Navarro X., et al. (2018). Activation of 5-ht2a receptors restores KCC2 function and reduces neuropathic pain after spinal cord injury. Neuroscience 387, 48–57. doi:10.1016/j.neuroscience.2017.08.033
Schulte J. T., Wierenga C. J., Bruining H. (2018). Chloride transporters and GABA polarity in developmental, neurological and psychiatric conditions. Neurosci. Biobehav. Rev. 90, 260–271. doi:10.1016/j.neubiorev.2018.05.001
Shiao R., Lee-Kubli C. A. (2018). Neuropathic pain after spinal cord injury: Challenges and research perspectives. Neurotherapeutics 15, 635–653. doi:10.1007/s13311-018-0633-4
Sivilotti R., Woolf C. J. (1994). The contribution of GABAA and glycine receptors to central sensitization: disinhibition and touch-evoked allodynia in the spinal cord. J Neurophysiol 72, 169–79.
Sorkin L. S., Puig S., Jones D. L. (1998). Spinal bicuculline produces hypersensitivity of dorsal horn neurons: Effects of excitatory amino acid antagonists. Pain 77, 181–190. doi:10.1016/s0304-3959(98)00094-3
Takayama Y., Uta D., Furue H., Tominaga M. (2015). Pain-enhancing mechanism through interaction between TRPV1 and anoctamin 1 in sensory neurons. Proc. Natl. Acad. Sci. U. S. A. 112, 5213–5218. doi:10.1073/pnas.1421507112
Talifu Z., Qin C., Xin Z., Chen Y., Liu J., Dangol S., et al. (2022). The overexpression of insulin-like growth factor-1 and neurotrophin-3 promote functional recovery and alleviate spasticity after spinal cord injury. Front. Neurosci. 16, 863793. doi:10.3389/fnins.2022.863793
Tang B. L. (2020). The expanding therapeutic potential of neuronal KCC2. Cells 9, 240. doi:10.3390/cells9010240
Tashiro S., Shinozaki M., Mukaino M., Renault-Mihara F., Toyama Y., Liu M., et al. (2015). BDNF induced by treadmill training contributes to the suppression of spasticity and allodynia after spinal cord injury via upregulation of KCC2. Neurorehabil. Neural Repair 29, 677–689. doi:10.1177/1545968314562110
Tillman L., Zhang J. (2019). Crossing the chloride channel: The current and potential therapeutic value of the neuronal K(+)-Cl(-) cotransporter KCC2. Biomed. Res. Int. 2019, 8941046. doi:10.1155/2019/8941046
Uvarov P., Ludwig A., Markkanen M., Rivera C., Airaksinen M. S. (2006). Upregulation of the neuron-specific K+/Cl- cotransporter expression by transcription factor early growth response 4. J. Neurosci. 26, 13463–13473. doi:10.1523/JNEUROSCI.4731-06.2006
Uvarov P., Ludwig A., Markkanen M., Soni S., HüBNER C. A., Rivera C., et al. (2009). Coexpression and heteromerization of two neuronal K-Cl cotransporter isoforms in neonatal brain. J. Biol. Chem. 284, 13696–13704. doi:10.1074/jbc.M807366200
Virtanen M. A., Uvarov P., HüBNER C. A., Kaila K. (2020). NKCC1, an elusive molecular target in brain development: Making sense of the existing data, Cells 9, 2607. doi:10.3390/cells9122607
Vyklicky V., Korinek M., Smejkalova T., Balik A., Krausova B., Kaniakova M., et al. (2014). Structure, function, and pharmacology of NMDA receptor channels. Physiol. Res. 63, S191–S203. doi:10.33549/physiolres.932678
Walter J. S., Sacks J., Othman R., Rankin A. Z., Nemchausky B., Chintam R., et al. (2002). A database of self-reported secondary medical problems among VA spinal cord injury patients: Its role in clinical care and management. J. Rehabil. Res. Dev. 39, 53–61. https://pubmed.ncbi.nlm.nih.gov/11926327/
Wardle R. A., Poo M. M. (2003). Brain-derived neurotrophic factor modulation of GABAergic synapses by postsynaptic regulation of chloride transport. J. Neurosci. 23, 8722–8732. doi:10.1523/JNEUROSCI.23-25-08722.2003
Watanabe M., Maemura K., Kanbara K., Tamayama T., Hayasaki H. (2002). GABA and GABA receptors in the central nervous system and other organs. Int. Rev. Cytol. 213, 1–47. doi:10.1016/s0074-7696(02)13011-7
Wenner P. (2014). Homeostatic synaptic plasticity in developing spinal networks driven by excitatory GABAergic currents. Neuropharmacology 78, 55–62. doi:10.1016/j.neuropharm.2013.04.058
WiderströM-Noga E. (2017). Neuropathic pain and spinal cord injury: Phenotypes and pharmacological management. Drugs 77, 967–984. doi:10.1007/s40265-017-0747-8
Woo N. S., Lu J., England R., Mcclellan R., Dufour S., Mount D. B., et al. (2002). Hyperexcitability and epilepsy associated with disruption of the mouse neuronal-specific K-Cl cotransporter gene. Hippocampus 12, 258–268. doi:10.1002/hipo.10014
Wu Z., Yang Q., Crook R. J., O'Neil R. G., Walters E. T. (2013). TRPV1 channels make major contributions to behavioral hypersensitivity and spontaneous activity in nociceptors after spinal cord injury. Pain 154, 2130–2141. doi:10.1016/j.pain.2013.06.040
Xie Y., Chang S., Zhao C., Wang F., Liu S., Wang J., et al. (2020). Structures and an activation mechanism of human potassium-chloride cotransporters. Sci. Adv. 6, eabc5883. doi:10.1126/sciadv.abc5883
Yan X., Liu J., Wang X., Li W., Chen J., Sun H., et al. (2018). Pretreatment with AQP4 and NKCC1 Inhibitors Concurrently Attenuated Spinal Cord Edema and Tissue Damage after Spinal Cord Injury in Rats. Front Physiol 9, 6.
Zhang J., Pu H., Zhang H., Wei Z., Jiang X., Xu M., et al. (2017). Inhibition of Na(+)-K(+)-2Cl(-) cotransporter attenuates blood-brain-barrier disruption in a mouse model of traumatic brain injury. Neurochem. Int. 111, 23–31. doi:10.1016/j.neuint.2017.05.020
Zhang S., Zhou J., Zhang Y., Liu T., Friedel P., Zhuo W., et al. (2021). The structural basis of function and regulation of neuronal cotransporters NKCC1 and KCC2. Commun. Biol. 4, 226. doi:10.1038/s42003-021-01750-w
Zhou W., Xie Z., Li C., Xing Z., Xie S., Li M., et al. (2021). Driving effect of BDNF in the spinal dorsal horn on neuropathic pain. Neurosci. Lett. 756, 135965. doi:10.1016/j.neulet.2021.135965
Keywords: spinal cord injury, CCCS, KCC2, NKCC1, neuropathic pain, spasticity, motor function reconstruction
Citation: Talifu Z, Pan Y, Gong H, Xu X, Zhang C, Yang D, Gao F, Yu Y, Du L and Li J (2022) The role of KCC2 and NKCC1 in spinal cord injury: From physiology to pathology. Front. Physiol. 13:1045520. doi: 10.3389/fphys.2022.1045520
Received: 15 September 2022; Accepted: 05 December 2022;
Published: 15 December 2022.
Edited by:
Paola de los Heros, National Autonomous University of Mexico, MexicoReviewed by:
Maria Chavez-Canales, Institute of Biomedical Research, National Autonomous University of Mexico, MexicoJinwei Zhang, University of Exeter, United Kingdom
Copyright © 2022 Talifu, Pan, Gong, Xu, Zhang, Yang, Gao, Yu, Du and Li. This is an open-access article distributed under the terms of the Creative Commons Attribution License (CC BY). The use, distribution or reproduction in other forums is permitted, provided the original author(s) and the copyright owner(s) are credited and that the original publication in this journal is cited, in accordance with accepted academic practice. No use, distribution or reproduction is permitted which does not comply with these terms.
*Correspondence: Liangjie Du, c3Ryb25nbmVja0BxcS5jb20=; Jianjun Li, Y3JyYzEwMEAxNjMuY29t
†These authors have contributed equally to this work and share first authorship