- 1The 2nd Medical College of Binzhou Medical University, Yantai, Shandong, China
- 2Department of Cardiology, Yantai Yuhuangding Hospital, Yantai, Shandong, China
- 3Medical Department of Qingdao University, Qingdao, Shandong, China
Ischemia/reperfusion (I/R) injury is a common clinical problem after coronary angioplasty, cardiopulmonary resuscitation, and organ transplantation, which can lead to cell damage and death. Mitsugumin 53 (MG53), also known as Trim72, is a conservative member of the TRIM family and is highly expressed in mouse skeletal and cardiac muscle, with minimal amounts in humans. MG53 has been proven to be involved in repairing cell membrane damage. It has a protective effect on I/R injury in multiple oxygen-dependent organs, such as the heart, brain, lung, kidney, and liver. Recombinant human MG53 also plays a unique role in I/R, sepsis, and other aspects, which is expected to provide new ideas for related treatment. This article briefly reviews the pathophysiology of I/R injury and how MG53 mitigates multi-organ I/R injury.
Introduction
Mitsugumin-53 (MG53), also known as TRIM72, is a muscle-specific triple motif family protein from our immunoproteomics library (Weisleder et al., 2008). Compared with other TRIM protein family members, they are very similar in structure which contains the tripartite motif that includes a Ring finger, one zinc-binding motif called B-box, and coiled-coil (CC)moieties. As a unique protein in the TRIM family, the MG53 has a SPRY domain at the carboxy terminus (Reymond et al., 2001; Cai et al., 2009a; Park et al., 2010) and is mainly expressed in myocardium sarcolemma and skeletal muscle sarcolemma (Lee et al., 2010). Previous studies have shown that MG53 can be detected in alveolar epithelial cells and the kidney, which impacts the lungs and kidneys under physiological or pathological conditions (Table 1) (Jia et al., 2014; Duann et al., 2015). While many studies support the protective role of MG53 in cardiovascular disease (Jiang et al., 2021; Zhong et al., 2021), no reports of MG53 on its role in multi-organ Ischemia/reperfusion (I/R) injury have been published. Here, we are intended to review the role of MG53 in I/R injury.
I/R injury
Ischemia is a lack of blood supply to tissue due to obstructed arterial flow, resulting in a shortage of oxygen and nutrients required for cell metabolism. Ischemic injury leads to cellular dysfunction, damage, or death, a process largely dependent on the degree and duration of blood supply disruption. Different organs have different susceptibilities to ischemic injury. After a few minutes of hypoxia in the brain, brain cells begin to suffer irreversible damage, their function is affected, and the process is almost irreversible, while muscle tissue can withstand ischemia for 60–90 min without irreversible damage (Duehrkop and Rieben, 2014). The ideal goal to prevent tissue death after local ischemia is hematologic reconstruction and restoration of blood flow as soon as possible, the benefits of which to body tissues are not as expected. During long-term ischemia, anaerobic metabolism and the accumulation of lactic acid deplete ATP and decrease intracellular pH, leading to dysfunction of ATP enzyme transport, increased intracellular and mitochondrial calcium levels (calcium overload), and eventually cell swelling, rupture, and death (Kalogeris et al., 2012). Although reperfusion restores oxygen levels, the surge of reactive oxygen species promotes neutrophil infiltration of ischemic tissue and exacerbates ischemic injury. (Kalogeris et al., 2012; Brown and Griendling, 2015). In 1960, Jennings et al. found that reperfusion after ischemia aggravated myocardial necrosis in canines for the first time. Even if the normal or near normal coronary blood flow is restored after reperfusion, mechanical dysfunction still existed (Jennings et al., 1960). There are many theories about the molecular mechanism of reperfusion injury, and some of them are pretty powerful, but they have not been fully elucidated. Here are some mainstream views: 1. Overproduction of reactive oxygen species (ROS) 2. intracellular and mitochondrial Ca2+ overload 3. Opening of the mitochondrial permeability transition pore 4. pronounced inflammatory responses 5. endothelial dysfunction (Yellon and Hausenloy, 2007; Kalogeris et al., 2016).
Overview of biological activities of MG53
The role of MG53 in repairing cell membrane damage
The importance of the cell membrane to the organism is self-evident. It is a barrier to prevent extracellular substances from freely entering the cell, to ensure the relative stability of the intracellular environment, and to make various biochemical reactions proceed in an orderly manner (Nicolson, 2013). In order to maintain cell homeostasis, eukaryotic cells protect the integrity of their plasma membranes in some ways, such as active recycling and repair, to deal with various sources of damage (McNeil et al., 2003). Repairing plasma membrane damage is an essential aspect of normal cell physiology, and the disruption of this process can lead to many different pathophysiologies (Cooper and McNeil, 2015). Previous studies showed that MG53 is responsible for plasma membrane repair (Cai et al., 2009a). Acute destruction of the plasma membrane will cause the inside of the cell to be exposed to the external oxidative environment. The oxidation state of MG53 may signal to activate the acute membrane repair process and mediate plasma membrane resealing, in which MG53 interacts with phosphatidylserine and promotes the transport of vesicles containing phosphatidylserine to the site of membrane damage (Figure 1) (Cai et al., 2009a). Many factors regulate the membrane repair process mediated by MG53. The research of Wang et al. showed that membrane cholesterol is an indispensable molecular participant that initiates MG53 translocation in myocardial membrane repair (Wang et al., 2010). Zhu et al. found that Polymerase I and Transcript Release Factor (PTRF) can act as a docking protein of MG53 in the process of membrane repair by binding exposed cholesterol at the injury site, and cells lacking endogenous PTRF show defective transport of MG53 to the injury site (Zhu et al., 2011). MG53 protein acts as an oxidation sensor to gather cell vesicles at the site of membrane damage to form a membrane patch, and the entry of Ca2+promotes the fusion of vesicles and plasma membrane. Ca2+ is necessary for fusing vesicles and plasma membranes but not for vesicle transport (Cai et al., 2009a; Hwang et al., 2011). Cai et al. found that the interaction of MG53, dysferlin, and caveolin-3 (CaV3) is essential for the repair of acute membrane damage in striated muscle (Cai et al., 2009b). They also found that MG53 can regulate the membrane sprouting and exocytosis of muscle cells and regulate this process by interacting with Cav3. (Cai et al., 2009c). It is worth noting that combining Zn2+ with the RING and B-box motifs of MG53 is essential for assembling the cell membrane repair mechanism (Cai et al., 2015). Previous data showed that the formation of disulfide bonds on Cys242 is critical for MG53 oligomerization and the initiation of cell membrane repair (Cai et al., 2009a). Based on this, Hwang et al. found that destroying MG53 oligomerization by chemically modifying cysteine residues before membrane damage may disrupt the MG53-mediated membrane repair process. The leucine zipper motif is essential for the formation of homodimers of MG53, which regulates the redox-dependent oligomerization of MG53, and the CC domain pair also plays an equally important role (Hwang et al., 2011). There are two conserved leucine zipper motifs (LZ1 and LZ2) in the CC domain of MG 53, which are very conservative in different animal species. If LZ1 and LZ2 are mutated, the oligomerization of MG 53 will decrease, and the cell membrane repair function of MG53 will be destroyed. 3Notably, LZ one will weaken the oligomerization of MG53, while LZ2 will not (Hwang et al., 2011).
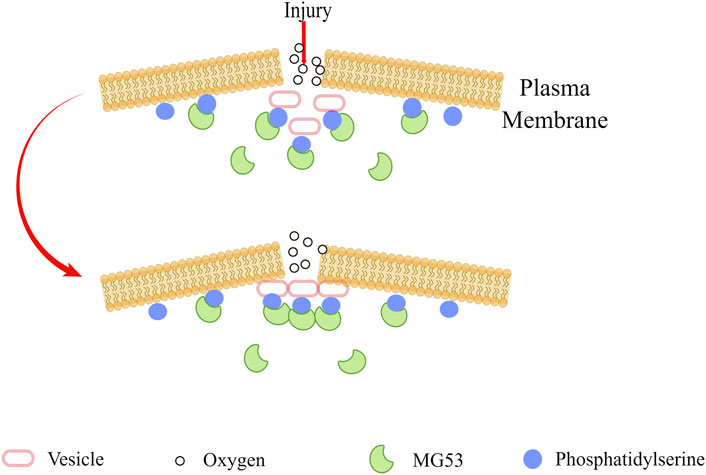
FIGURE 1. When the plasma membrane was damaged, MG53 sensed the oxidized extracellular environment, and by combining with phosphatidylserine, it adhered to the plasma membrane and intracellular vesicles, and finally gathered at the injury site to seal the damaged membrane.
Effect of MG53 on cardiac I/R injury
According to the latest report from WHO, the world’s biggest killer is ischemic heart disease which is responsible for 16% of the world’s total deaths. Since 2000, the most significant increase in fatalities has been for this disease, rising by more than 2 million to 8.9 million deaths in 2019 (Organization, 2020). Although cardiovascular health has improved in recent years, the global health burden of cardiovascular diseases is still high. Therefore, it is urgent to study the possible mechanisms of cardiovascular disease. Murry et al. first reported Ischemic preconditioning (IPC) in 1986. They found that multiple brief episodes of ischemic might delay cell death after coronary artery occlusion, thereby protecting the myocardium from subsequent ischemic damage (Murry et al., 1986). IPC also plays a vital role in other organs, such as the brain, liver, and kidney (Zager et al., 1985; Chen and Simon, 1997; Koti et al., 2003).
It has been confirmed that IPC and ischemic postconditioning (IPostC) can reduce myocardial injury caused by I/R (Murry et al., 1986; Zhao et al., 2002). IPC mainly involves two essential pathways: the reperfusion injury salvage kinase (RISK)pathway and the survivor activating factor enhancement (SAFE) pathway. The RISK pathway consists of PI3K-Akt-GSK3βand ERK1/2 signaling events, whereas the SAFE pathway involves activation of tumor necrosis factor-α and the JAK-STAT3 axis (Crisostomo et al., 2006; Lacerda et al., 2009; Lecour, 2009). In 2003, Zhao et al. first proposed IPostC, which is another form of cardioprotection. IPostC is achieved by shortly interrupting the perfusion cycle in the early stages of perfusion, which has been observed to save coronary artery endothelium and cardiomyocytes (Zhao et al., 2002).
With the deepening of the research, more and more proofs show that MG53 plays a vital role in IPC and IPostC (Cao et al., 2010; Zhang et al., 2011). As shown in the study of Cao et al., MG53 deficiency completely abolished IPC-mediated cardioprotection. In MG53 knockout mice, IPC failed to reduce the area of IR-induced myocardial infarction compared with wild-type mice (Cao et al., 2010). Cao et al. found that the lack of MG53 completely eliminated IPC-induced PI3K activation, indicating that MG53 is necessary for IPC-induced PI3K activation, which is a crucial component of the pro-survival RISK pathway. In contrast, MG53 does not participate in the SAFE pathway because IPC-induced STAT-3 phosphorylation remains intact in MG53-deficient hearts (Cao et al., 2010). The research by Shan et al. showed that during cardiac ischemic preconditioning, the reactive oxygen species level of myocardial cells was increased, which activated protein kinase C-δ(PKC-δ) and induced the secretion of MG53 protein (Shan et al., 2020). The phosphorylation levels of several key pro-survival kinases were significantly increased due to overexpression of MG53, including Akt, GSK3β, and ERK1/2, over their respective control (Fujio et al., 2000; Tong et al., 2002; Shiraishi et al., 2004). The study by Wang et al. found that when I/R injured the heart, MG53 could sense and promote plasma membrane translocation (Wang et al., 2010). Meanwhile, immunostaining of myocardial sections showed that MG53 accumulated in the myocardium, which indicated that the I/R activated the repair mechanism mediated by MG53. MG53 ablation aggravated myocardial damage caused by I/R, manifested by mitochondrial dysfunction and cardiomyocyte loss (Wang et al., 2010). In a recent study, Gumpper-Fedus et al. found that during ischemia-reperfusion-induced oxidative stress, MG53 binds to cardiolipin, a mitochondria-specific phospholipid, preventing damage to mitochondria, and maintains their integrity (Gumpper-Fedus et al., 2022). Wang et al. found that I/R-induced heart damage can lead to necrosis, while MG53 has a regulatory effect on RIPK1-dependent necrosis during I/R injury, which inhibits I/R damage cardiac necrosis by downregulating ubiquitination-dependent RIPK1 expression (Wang et al., 2022).
Although Lemckert et al. demonstrated that MG53 was an effective biomarker for mice heart myocardial injury and dysfunction, the expression of MG53 was extremely low or undetectable in samples of pediatric patients undergoing surgery to correct congenital structural heart defects. This research indicates that MG53 cannot be used as a clinical biomarker of human myocardial damage, nor can it be used as an endogenous cardioprotective agent for ischemic preconditioning or postprocessing (Lemckert et al., 2016). However, Guo et al. reported the expression of MG53 in the human atrium for the first time, which was verified by immunohistochemical staining, quantitative PCR, and western blotting in patients undergoing cardiac surgery (Guo et al., 2018). These differences between studies may be due to different experimental methods or different antibodies used, and further studies are needed to prove whether MG53 is expressed in the human heart. In 2020, Xie et al. used enzyme-linked immunosorbent assay (ELISA) to determine the concentration of MG53 in the serum of 639 patients undergoing coronary angiography. Logistic and linear regression was used to analyze the relationship between MG53 and coronary heart disease. They found that patients with stable cardiovascular disease and acute myocardial infarction had elevated serum MG53 levels (Xie et al., 2020a), and subsequent studies have shown that MG53 is a valuable prognostic indicator for patients with acute myocardial infarction (AMI) (Xie et al., 2020b), which can provide new ideas for clinical practice.
Effects of MG53 on cerebral I/R injury
Stroke is an acute cerebrovascular disease, which is the leading cause of death and long-term disability. The majority of strokes are ischemic brain damage caused by occlusion of cerebral arteries (Virani et al., 2020). An ischemic stroke usually leads to lower blood oxygen levels and hypoperfusion, which in turn leads to neurotoxic events. Cerebral ischemic injury can lead to the formation of cerebral edema and increased intracranial pressure (ICP), which compresses the cerebral vessels and weakens cerebral perfusion in the ischemic area (Simard et al., 2007). Timely restoration of blood flow is necessary to prevent ischemic tissue infarction, but oxygenated blood reperfusion can lead to aggravation of brain damage, also known as I/R injury. Oxidative stress, inflammation, and apoptosis are the primary cellular responses of brain tissue after I/R injury (Fang et al., 2015; Palencia et al., 2015). Brain I/R injury increases the phosphorylation of extracellular signal-regulated kinase 1/2 (ERK1/2), induces the expression of membrane G protein-coupled receptor kinase 2 (GRK2), and reduces the expression of cortical microvascular matrix metalloproteinase-9 (MMP-9) and cytoplasmic GRK2 (Zheng et al., 2007; Sarshoori et al., 2014). Due to the blood-brain barrier (BBB), many neuroprotective agents confirmed within in vitro studies have lost their effect within in vivo experiments. In mouse tissues, MG53 is predominantly expressed in skeletal and cardiac muscles and cannot be detected in neurons (Cai et al., 2009a; Yao et al., 2016). However, Yao et al. provided evidence that I/R injury caused an abundant accumulation of MG53 in the brain tissue. (Yao et al., 2016). Moreover, their research showed that recombinant human MG53 (rhMG53) could cross the BBB to the I/R damaged brain. Intravenous administration before or after ischemia can effectively reduce brain injury, inhibit apoptosis, and activate the pro-survival RISK signaling (Yao et al., 2016). Brain I/R injury leads to the decrease of Akt phosphorylation and the inhibition of GSK3β activation, which can be restored by rhMG53 treatment (Yao et al., 2016). Some studies have shown that I/R-induced brain injury leads to the activation of caspase3, which is the core protein of the apoptotic cascade reaction pathway (Porter and Janicke, 1999; Galluzzi et al., 2012). Caspase3 activation is an essential condition for cell apoptosis, and rhMG53 treatment could effectively suppress caspase3 activation (Chen et al., 2015; Gharibani et al., 2015). MG53 could significantly inhibit the expressions of TNF-α, TLR4, NLRP3, Caspase1, and IL-1β, reduce the neuroinflammatory response, promote the proliferation and migration of human umbilical cord mesenchymal stem cells (hUC-MSCs), inhibit the release of inflammatory cytokines, and resist LPS-induced apoptosis and mitochondrial dysfunction (Guan et al., 2019; Ma et al., 2020). The combination of MG53 and hUC-MSCs promoted neurogenesis by reducing apoptosis and improving PI3K/Akt-GSK3β signaling pathway. MG53 protects hUC-MSCs from inflammatory injury by inhibiting the NLRP3/Caspase-1/IL-1β axis and synergistically enhances its efficacy on the brain damaged by neuroinflammation. RhMG53 protein has a protective effect on H2O2-induced oxidative damage of hUC-MSCs, and promotes the proliferation and migration of hUC-MSCs, reducing cerebral edema and neurological deficits (Guan et al., 2019; Ma et al., 2020).
Effects of MG53 on lung I/R injury
Since the 1980s, with the success of lung transplantation, it has become the main treatment for most end-stage lung diseases. Although lung surgical techniques and perioperative care have improved, lung injury caused by I/R is still a significant cause of early morbidity and mortality after lung transplantation (King et al., 2000). I/R-induced lung injury can occur in acute pulmonary embolism, heart organ transplantation, and cardiopulmonary bypass surgery (Ambrosio and Tritto, 1999; de Perrot et al., 2003; Ng et al., 2006; Yellon and Hausenloy, 2007). The pathogenesis of these lung diseases may be related to lung epithelial cell damage (Matthay et al., 2012; Sweeney et al., 2013). Under normal circumstances, I/R can be understood as hypoxia-reoxygenation in tissues. However, this situation needs to be treated differently in the lungs because the alveoli contain a certain amount of oxygen, which can prevent early hypoxia caused by ischemia and maintain aerobic metabolism (de Perrot et al., 2003). Although the mechanism of lung injury induced by I/R is not completely clear, excessive production of ROS, pulmonary mitochondrial dysfunction, polymorphonuclear leukocyte infiltration, and macrophage isolation may be related to lung injury (Sommer et al., 2011; Campos et al., 2012). MG53 has been detected to be expressed in type I and type II alveolar epithelial cells, and the latter has a higher level of expression. But MG53 is shown to be not expressed in endothelial cells (Jia et al., 2014; Kim et al., 2014). In order to test whether the lack of MG53 can change the lung response after I/R-induced injury, JIA et al. conducted experiments on wild-type and MG53−/− mice which induced complete left lung ischemia and hypoxia by clamping the left pulmonary artery and hilum on the procedure of 1 h ischemia/1 h reperfusion. Compared with the wild-type control, The MG53−/− mouse has a lower survival rate after I/R injury. The ablation of MG53 leads to increased susceptibility of mice to I/R-induced lung injury (Jia et al., 2014), and the overexpression of MG53 has a protective effect on the repair of alveolar epithelial cells. The repair function of MG53 in the lung is related to caveolin 1(Cav1) (Kim et al., 2014). Cav1 is a protein rich in lung endothelial cells and alveolar epithelial type I cell (ATI), which promotes the repair of cell membrane rupture through endocytosis (Dahlin et al., 2004; Corrotte et al., 2013). In another animal experiment, intravenous injection of rhMG53 can have a protective effect on I/R-mediated acute lung injury (ALI) without significant toxicity. It effectively protects against lung epithelial cell damage and restores lung function after ALI (Weisleder et al., 2012; Jia et al., 2014).
Effects of MG53 on renal I/R injury
Kidney disease is a global public health problem. With the aging of the population and the increasing incidence of diabetes and hypertension, kidney failure is also growing. Acute kidney injury (AKI) is one of the most common acute and critical illnesses in various clinical departments, with high morbidity and mortality (Doyle and Forni, 2016). Although a large number of clinical trials have been conducted with multiple interventions, a reliable method to prevent AKI has still not been found (Landoni et al., 2013). More and more scholars have realized that acute kidney injury (AKI) and chronic kidney disease (CKD) are closely related and may promote each other (Hsu and Hsu, 2016). A kidney is very sensitive to I/R, which is the most common cause of AKI. (Kanagasundaram, 2015). After renal tissue ischemia, its metabolism changes from aerobic to anaerobic, and intracellular ATP is consumed, leading to acidosis. Inactivation of Na+/K+ ATPase results in intracellular sodium and water retention, causing cell edema (Pefanis et al., 2019). Reperfusion restores aerobic metabolism in the kidney but produces reactive oxygen species (ROS), which destroy functional cells and induce the death of tubular epithelial cells (Bonventre and Yang, 2011). I/R triggers a series of harmful cellular reactions in the affected organs, ultimately leading to cell necrosis or apoptosis (Bonventre and Yang, 2011). A sign of renal I/R injury is the appearance of necrotic tubule cells (Brady and Singer, 1995).
Although MG53 is expressed at a low concentration in the kidney, it is significantly expressed in the proximal tubule epithelium (PTE). Under normal physiological conditions, the apical surface of the PTE cells has obvious endocytosis and exocytosis (Saito et al., 2010; Christensen et al., 2012). MG53-mediated membrane repair plays a vital role in renal protection. Membrane repair defects caused by MG53 deletion can increase sensitivity to I/R-induced AKI, aggravate I/R-induced AKI, and develop pathological renal phenotypes of interstitial cell damage (Duann et al., 2015). Intravenous injection of rhMG53 before I/R can effectively prevent the occurrence of AKI in the mouse model. At the same time, rhMG53 was also shown to reduce cisplatin-induced AKI without reducing its oncological efficacy. (Duann et al., 2015). Previous studies have shown that the association of rhMG53 with membrane destruction sites requires recognition of lipid signals. MG53 can bind to phosphatidylserine (PS), usually inside the plasma membrane, and may be exposed outside the plasma membrane after injury (Weisleder et al., 2012). AKI caused by I/R can lead to the exposure of phosphatidylserine on PTE cells, which may be the anchoring mechanism of the rhMG53 repair process (Duann et al., 2015). Therefore, targeting MG53 provides a new therapeutic approach to preventing I/R-related AKI.
Effects of MG53 on hepatic I/R injury
Hepatic ischemia-reperfusion injury (HIRI) refers to the pathophysiological process in which liver ischemia is further aggravated when the blood flow is reperfused for a while. In recent years, HIRI has attracted the attention of many researchers because it often occurs in clinical settings, such as liver transplantation, liver resection, hemorrhagic shock, and trauma (Saidi and Kenari, 2014). With the development of various liver operations, HIRI has become an essential factor affecting the morbidity and mortality of the operation (Serracino-Inglott et al., 2001). As a unique and crucial immune organ of the human body, the liver contains various kinds of cells, such as Kupffer cells (KCs), natural killer cells (NK), natural killer cells (NKT), and dendritic cells (DC), which not only play an immune role but also play a pivotal role in I/R injury (Dong et al., 2007). Unlike I/R injury in other organs, there are two types of HIRI, warm IRI and cold IRI (Ikeda et al., 1992; Zhai et al., 2011). Warm IRI usually occurs when the normal blood perfusion of liver tissue is blocked, such as prolonged occlusion of blood flow during hepatectomy, shock, and trauma. Warm IRI can be divided into two distinct phases. The early stage typically occurs minutes to 6 hours after hepatic ischemia. It is characterized by the rapid activation of Kupffer cells to generate ROS, which induces oxidative stress and develops parenchymal vascular damage. The initial stage of liver injury is relatively low in extent, but it triggers a series of subsequent events. In the later stages of warm IRI, neutrophils accumulate in the liver after ischemia and directly damage hepatocytes through the production of ROS and proteases, which finally leads to cell death (Konishi and Lentsch, 2017). Cold IRI often occurs in liver transplantation because the donated liver is preserved in a hypothermic environment (Zhai et al., 2013). Two types of HIRI trigger three types of cell death: necrosis, apoptosis, and autophagy (Hu et al., 2021).
PTRF is indispensable in MG53-mediated membrane repair (Zhu et al., 2011). Although PTRF is expressed in most organs, it is not expressed in the liver (Zhou et al., 2015). Studies have shown that dysferlin is closely related to MG53 in the liver, and the C2A domain of dysferlin plays a key role (Matsuda et al., 2012). The research of Yao et al. showed that MG53 could reduce the damage of hepatocyte membrane induced by HIRI by combining with dysferlin, which could reduce the oxidative stress in HIRI and the death of hepatocytes. But the experiment failed to prove whether other specific proteins were interacting with MG53 in the liver (Yao et al., 2017). Severe liver damage caused by HIRI manifest as increased ALT and AST release. Administration of exogenous rhMG53 reduces ALT and AST in the model (Yao et al., 2017), which may be necessary for future clinical treatment of HIRI. Whether MG53 participates in the inflammatory mechanism of HIRI still needs more experiments to confirm.
Controversy over MG53
Metabolic syndrome is not a single disease but a combination of cardiovascular disease risk factors, such as abdominal obesity, insulin resistance, hyperlipidemia, and hypertension, which increase the risk of cardiovascular atherosclerotic disease and type 2 diabetes (Kassi et al., 2011). Metabolic syndrome has become a global problem and poses a significant health risk (Saklayen, 2018). Research by Song and Wu et al. indicated that MG53 might be a pathogenic factor of diabetes (Song et al., 2013; Yi et al., 2013; Wang and Hill, 2015; Wu et al., 2019). The study by Song et al. showed that the expression of MG53 in the insulin resistance model was significantly increased, and the over-expression of MG53 triggered insulin resistance and metabolic syndrome. In contrast, ablating the MG53 did not produce the symptoms described above (Song et al., 2013). In mechanism, MG53, as an E3 ligase, performs ubiquitin-dependent degradation of insulin receptor (IR) and insulin receptor substrate (IRS1), leading to insulin resistance and metabolic disorders. (Song et al., 2013). Wu et al. demonstrated that high glucose or high insulin induced MG53 secretion in isolated rodent hearts and skeletal muscles. In humans and rodents with diabetes, increased glucose was accompanied by increased circulating MG53 (Wu et al., 2019). Mechanistically, MG53 binds to the extracellular structural domain of the insulin receptor and inhibits insulin signaling (Wu et al., 2019). Liu et al. proposed different mechanisms of MG53-mediated diabetes. They found that overexpression of MG53 was sufficient to induce systemic insulin resistance and impaired glucose uptake by constructing MG53-overexpressed mice using an α -myosin heavy chain (α-MHC) promoter (Liu et al., 2015a). Mechanistically, in addition to MG53-induced dysregulation of IR and IRS-1, it also blocks insulin signaling by upregulating peroxisome proliferator-activated receptor-α (PPAR-α) levels, resulting in cardiac lipid accumulation and, ultimately, diabetic cardiomyopathy (Finck et al., 2003; Liu et al., 2015a).
However, another study differs from previous results; compared with wild-type (WT) littermates, the circulating MG53 in blood samples from diabetic mice was significantly reduced (Wang et al., 2020). Wang et al. established two db/db mouse models, one of which removed MG53 from the blood, and the other continuously increased MG53 in the blood. As a result, the insulin signal and glucose treatment remained unchanged. Treatment of MG53−/− mice with streptozotocin (STZ) leads to abnormal glucose therapy, which indicates that MG53 might have protective ß -cell function (Wang et al., 2020). The evidence provided by Bian et al. shows that the continuously rising circulating MG53 did not affect insulin signal and glucose processing in mice, and it is safe for metabolism and heart function (Bian et al., 2019). Research by Philouze et al. showed that MG 53 was not a key regulator of skeletal muscle insulin signaling pathway, and these findings were consistent with the results of BIAN et al. (Philouze et al., 2021). Ma et al. established a mouse model of metabolic syndrome to investigate the effect on the activity of MG53. They found that the level of circulating MG53 in mice fed with a high-fat diet decreased significantly, but the expression of MG 53 in skeletal muscle and myocardium remained unchanged (Ma et al., 2015). The differences in these results have led to controversy over MG53, which will require more studies to resolve.
The therapeutic potential of recombinant human MG53
To find out whether rhMG53 can play a therapeutic role in heart IRI, Liu et al. demonstrated by using several animal models that administration of rhMG53 reduced infarct size after reperfusion (Liu et al., 2015b). Wang et al. used rhMG53 to treat alkali-induced corneal wounds and found that topical application of rhMG53 significantly improved corneal wound healing in diabetic mice. Compared with WT mice, the cornea of db/db mice undergoes excessive revascularization after alkaline injury, and the addition of rhMG53 alleviates the excessive vascularization (Wang et al., 2020). Sepsis will lead to the down-regulation of MG53 and PPARα. Supplementation of rhMG53 can improve the survival rate and cardiac function, reduce oxidative stress, diminish inflammation and decrease cardiomyocyte apoptosis related to PPARα up-regulation (Han et al., 2020). MG53 supplements can likewise protect the heart from myocardial dysfunction caused by sepsis by up-regulating PPARα expression (Han et al., 2020). As previously mentioned, multiple articles support the value of rhMG53 in therapy, but the safety of rhMG53 needs to be further assessed before its application. Repeated intravenous administration of rhMG53 in rodents and dogs did not produce adverse effects or alter rat blood metabolites (Weisleder et al., 2012; Duann et al., 2015; Wang et al., 2020). These results will help its potential clinical application.
Conclusion
Since I/R injury is a complex pathophysiological process, it can affect multiple organs, such as the heart, brain, lung, kidney, and liver. ATP depletion, ROS production, and elevated intracellular and mitochondrial calcium (calcium overload) are all related to I/R, and their clinical manifestations are diverse, which poses a severe challenge for clinicians. MG53 is mainly expressed in skeletal muscle and myocardium and also plays a unique role in other organs (Figure 2), for example, in promoting the repair of the cell membrane and activating the cardiac RISK pathway to mediate cardiac IPC and PostC. Currently, almost all studies on the function of MG53 are conducted in animal models. Further work is to conduct clinically relevant studies. Although the mechanism related to I/R injury still needs to be developed, we believe that MG53 provides new ideas for exploring the treatment of I/R injury.
Author contributions
BX, conceptualization, investigation, writing—original draft; CW and HC, writing—original draft; DT and LZ, visualization, investigation; LG, visualization, conceptualization; LZ and JY (Corresponding Author), conceptualization, funding acquisition, resources, supervision, writing—review and editing.
Funding
This work was supported by the Key Research and Development Program of Shandong Province (No. 2019GSF108142); National Natural Science Foundation of China (No. 81900310); Science and Technology Program of Yantai City (Nos. 2021MSGY042 and 2021MSGY044).
Acknowledgments
The authors would like to express their gratitude to all participants for related discussions.
Conflict of interest
The authors declare that the research was conducted in the absence of any commercial or financial relationships that could be construed as a potential conflict of interest.
Publisher’s note
All claims expressed in this article are solely those of the authors and do not necessarily represent those of their affiliated organizations, or those of the publisher, the editors and the reviewers. Any product that may be evaluated in this article, or claim that may be made by its manufacturer, is not guaranteed or endorsed by the publisher.
References
Ambrosio G., Tritto I. (1999). Reperfusion injury: Experimental evidence and clinical implications. Am. Heart J. 138, S69–S75. doi:10.1016/s0002-8703(99)70323-6
Bian Z., Wang Q., Zhou X., Tan T., Park K. H., Kramer H. F., et al. (2019). Sustained elevation of mg53 in the bloodstream increases tissue regenerative capacity without compromising metabolic function. Nat. Commun. 10, 4659. doi:10.1038/s41467-019-12483-0
Bonventre J. V., Yang L. (2011). Cellular pathophysiology of ischemic acute kidney injury. J. Clin. Invest. 121, 4210–4221. doi:10.1172/JCI45161
Brady H. R., Singer G. G. (1995). Acute renal failure. Lancet 346, 1533–1540. doi:10.1016/s0140-6736(95)92057-9
Brown D. I., Griendling K. K. (2015). Regulation of signal transduction by reactive oxygen species in the cardiovascular system. Circ. Res. 116, 531–549. doi:10.1161/CIRCRESAHA.116.303584
Cai C., Lin P., Zhu H., Ko J. K., Hwang M., Tan T., et al. (2015). Zinc binding to mg53 protein facilitates repair of injury to cell membranes. J. Biol. Chem. 290, 13830–13839. doi:10.1074/jbc.M114.620690
Cai C., Masumiya H., Weisleder N., Matsuda N., Nishi M., Hwang M., et al. (2009). Mg53 nucleates assembly of cell membrane repair machinery. Nat. Cell Biol. 11, 56–64. doi:10.1038/ncb1812
Cai C., Masumiya H., Weisleder N., Pan Z., Nishi M., Komazaki S., et al. (2009). Mg53 regulates membrane budding and exocytosis in muscle cells. J. Biol. Chem. 284, 3314–3322. doi:10.1074/jbc.M808866200
Cai C., Weisleder N., Ko J. K., Komazaki S., Sunada Y., Nishi M., et al. (2009). Membrane repair defects in muscular dystrophy are linked to altered interaction between mg53, caveolin-3, and dysferlin. J. Biol. Chem. 284, 15894–15902. doi:10.1074/jbc.M109.009589
Campos R., Shimizu M. H., Volpini R. A., de Braganca A. C., Andrade L., Lopes F. D., et al. (2012). N-acetylcysteine prevents pulmonary edema and acute kidney injury in rats with sepsis submitted to mechanical ventilation. Am. J. Physiol. Lung Cell. Mol. Physiol. 302, L640–L650. doi:10.1152/ajplung.00097.2011
Cao C. M., Zhang Y., Weisleder N., Ferrante C., Wang X., Lv F., et al. (2010). Mg53 constitutes a primary determinant of cardiac ischemic preconditioning. Circulation 121, 2565–2574. doi:10.1161/CIRCULATIONAHA.110.954628
Chen H., Tian M., Jin L., Jia H., Jin Y. (2015). Puma is invovled in ischemia/reperfusion-induced apoptosis of mouse cerebral astrocytes. Neuroscience 284, 824–832. doi:10.1016/j.neuroscience.2014.10.059
Chen J., Simon R. (1997). Ischemic tolerance in the brain. Neurology 48, 306–311. doi:10.1212/wnl.48.2.306
Christensen E. I., Birn H., Storm T., Weyer K., Nielsen R. (2012). Endocytic receptors in the renal proximal tubule. Physiol. (Bethesda) 27, 223–236. doi:10.1152/physiol.00022.2012
Cooper S. T., McNeil P. L. (2015). Membrane repair: Mechanisms and pathophysiology. Physiol. Rev. 95, 1205–1240. doi:10.1152/physrev.00037.2014
Corrotte M., Almeida P. E., Tam C., Castro-Gomes T., Fernandes M. C., Millis B. A., et al. (2013). Caveolae internalization repairs wounded cells and muscle fibers. Elife 2, e00926. doi:10.7554/eLife.00926
Crisostomo P. R., Wairiuko G. M., Wang M., Tsai B. M., Morrell E. D., Meldrum D. R. (2006). Preconditioning versus postconditioning: Mechanisms and therapeutic potentials. J. Am. Coll. Surg. 202, 797–812. doi:10.1016/j.jamcollsurg.2005.12.002
Dahlin K., Mager E. M., Allen L., Tigue Z., Goodglick L., Wadehra M., et al. (2004). Identification of genes differentially expressed in rat alveolar type i cells. Am. J. Respir. Cell Mol. Biol. 31, 309–316. doi:10.1165/rcmb.2003-0423OC
de Perrot M., Liu M., Waddell T. K., Keshavjee S. (2003). Ischemia-reperfusion-induced lung injury. Am. J. Respir. Crit. Care Med. 167, 490–511. doi:10.1164/rccm.200207-670SO
Dong Z., Wei H., Sun R., Tian Z. (2007). The roles of innate immune cells in liver injury and regeneration. Cell. Mol. Immunol. 4, 241–252.
Doyle J. F., Forni L. G. (2016). Acute kidney injury: Short-term and long-term effects. Crit. Care 20, 188. doi:10.1186/s13054-016-1353-y
Duann P., Li H., Lin P., Tan T., Wang Z., Chen K., et al. (2015). Mg53-mediated cell membrane repair protects against acute kidney injury. Sci. Transl. Med. 7, 279ra36. doi:10.1126/scitranslmed.3010755
Duehrkop C., Rieben R. (2014). Ischemia/reperfusion injury: Effect of simultaneous inhibition of plasma cascade systems versus specific complement inhibition. Biochem. Pharmacol. 88, 12–22. doi:10.1016/j.bcp.2013.12.013
Fang L., Gao H., Zhang W., Zhang W., Wang Y. (2015). Resveratrol alleviates nerve injury after cerebral ischemia and reperfusion in mice by inhibiting inflammation and apoptosis. Int. J. Clin. Exp. Med. 8, 3219–3226.
Finck B. N., Han X., Courtois M., Aimond F., Nerbonne J. M., Kovacs A., et al. (2003). A critical role for pparalpha-mediated lipotoxicity in the pathogenesis of diabetic cardiomyopathy: Modulation by dietary fat content. Proc. Natl. Acad. Sci. U. S. A. 100, 1226–1231. doi:10.1073/pnas.0336724100
Fujio Y., Nguyen T., Wencker D., Kitsis R. N., Walsh K. (2000). Akt promotes survival of cardiomyocytes in vitro and protects against ischemia-reperfusion injury in mouse heart. Circulation 101, 660–667. doi:10.1161/01.cir.101.6.660
Galluzzi L., Vitale I., Abrams J. M., Alnemri E. S., Baehrecke E. H., Blagosklonny M. V., et al. (2012). Molecular definitions of cell death subroutines: Recommendations of the nomenclature committee on cell death 2012. Cell Death Differ. 19, 107–120. doi:10.1038/cdd.2011.96
Gharibani P., Modi J., Menzie J., Alexandrescu A., Ma Z., Tao R., et al. (2015). Comparison between single and combined post-treatment with s-methyl-n, n-diethylthiolcarbamate sulfoxide and taurine following transient focal cerebral ischemia in rat brain. Neuroscience 300, 460–473. doi:10.1016/j.neuroscience.2015.05.042
Guan F., Huang T., Wang X., Xing Q., Gumpper K., Li P., et al. (2019). The trim protein mitsugumin 53 enhances survival and therapeutic efficacy of stem cells in murine traumatic brain injury. Stem Cell Res. Ther. 10, 352. doi:10.1186/s13287-019-1433-4
Gumpper-Fedus K., Park K. H., Ma H., Zhou X., Bian Z., Krishnamurthy K., et al. (2022). Mg53 preserves mitochondrial integrity of cardiomyocytes during ischemia reperfusion-induced oxidative stress. Redox Biol. 54, 102357. doi:10.1016/j.redox.2022.102357
Guo J., Jia F., Jiang Y., Li Q., Yang Y., Xiao M., et al. (2018). Potential role of MG53 in the regulation of transforming-growth-factor-β1-induced atrial fibrosis and vulnerability to atrial fibrillation Exp. Cell Res 362, 436–443. doi:10.1016/j.yexcr.2017.12.007
Han X., Chen D., Liufu N., Ji F., Zeng Q., Yao W., et al. (2020). MG53 protects against sepsis-induced myocardial dysfunction by upregulating peroxisome proliferator-activated receptor-α. Oxid. Med. Cell. Longev. 2020, 7413693. doi:10.1155/2020/7413693
Hsu R. K., Hsu C. Y. (2016). The role of acute kidney injury in chronic kidney disease. Semin. Nephrol. 36, 283–292. doi:10.1016/j.semnephrol.2016.05.005
Hu C., Zhao L., Zhang F., Li L. (2021). Regulation of autophagy protects against liver injury in liver surgery-induced ischaemia/reperfusion. J. Cell. Mol. Med. 25, 9905–9917. doi:10.1111/jcmm.16943
Hwang M., Ko J. K., Weisleder N., Takeshima H., Ma J. (2011). Redox-dependent oligomerization through a leucine zipper motif is essential for mg53-mediated cell membrane repair. Am. J. Physiol. Cell Physiol. 301, C106–C114. doi:10.1152/ajpcell.00382.2010
Ikeda T., Yanaga K., Kishikawa K., Kakizoe S., Shimada M., Sugimachi K. (1992). Ischemic injury in liver transplantation: Difference in injury sites between warm and cold ischemia in rats. Hepatology 16, 454–461. doi:10.1002/hep.1840160226
Jennings R. B., Sommers H. M., Smyth G. A., Flack H. A., Linn H. (1960). Myocardial necrosis induced by temporary occlusion of a coronary artery in the dog. Arch. Pathol. 70, 68–78.
Jia Y., Chen K., Lin P., Lieber G., Nishi M., Yan R., et al. (2014). Treatment of acute lung injury by targeting mg53-mediated cell membrane repair. Nat. Commun. 5, 4387. doi:10.1038/ncomms5387
Jiang W., Liu M., Gu C., Ma H. (2021). The pivotal role of mitsugumin 53 in cardiovascular diseases. Cardiovasc. Toxicol. 21, 2–11. doi:10.1007/s12012-020-09609-y
Kalogeris T., Baines C. P., Krenz M., Korthuis R. J. (2012). Cell biology of ischemia/reperfusion injury. Int. Rev. Cell Mol. Biol. 298, 229–317. doi:10.1016/B978-0-12-394309-5.00006-7
Kalogeris T., Baines C. P., Krenz M., Korthuis R. J. (2016). Ischemia/reperfusion. Compr. Physiol. 7, 113–170. doi:10.1002/cphy.c160006
Kanagasundaram N. S. (2015). Pathophysiology of ischaemic acute kidney injury. Ann. Clin. Biochem. 52, 193–205. doi:10.1177/0004563214556820
Kassi E., Pervanidou P., Kaltsas G., Chrousos G. (2011). Metabolic syndrome: Definitions and controversies. BMC Med. 9, 48. doi:10.1186/1741-7015-9-48
Kim S. C., Kellett T., Wang S., Nishi M., Nagre N., Zhou B., et al. (2014). Trim72 is required for effective repair of alveolar epithelial cell wounding. Am. J. Physiol. Lung Cell. Mol. Physiol. 307, L449–L459. doi:10.1152/ajplung.00172.2014
King R. C., Binns O. A., Rodriguez F., Kanithanon R. C., Daniel T. M., Spotnitz W. D., et al. (2000). Reperfusion injury significantly impacts clinical outcome after pulmonary transplantation. Ann. Thorac. Surg. 69, 1681–1685. doi:10.1016/s0003-4975(00)01425-9
Konishi T., Lentsch A. B. (2017). Hepatic ischemia/reperfusion: Mechanisms of tissue injury, repair, and regeneration. Gene Expr. 17, 277–287. doi:10.3727/105221617X15042750874156
Koti R. S., Seifalian A. M., Davidson B. R. (2003). Protection of the liver by ischemic preconditioning: A review of mechanisms and clinical applications. Dig. Surg. 20, 383–396. doi:10.1159/000072064
Lacerda L., Somers S., Opie L. H., Lecour S. (2009). Ischaemic postconditioning protects against reperfusion injury via the safe pathway. Cardiovasc. Res. 84, 201–208. doi:10.1093/cvr/cvp274
Landoni G., Bove T., Szekely A., Comis M., Rodseth R. N., Pasero D., et al. (2013). Reducing mortality in acute kidney injury patients: Systematic review and international web-based survey. J. Cardiothorac. Vasc. Anesth. 27, 1384–1398. doi:10.1053/j.jvca.2013.06.028
Lecour S. (2009). Activation of the protective survivor activating factor enhancement (safe) pathway against reperfusion injury: Does it go beyond the risk pathway? J. Mol. Cell. Cardiol. 47, 32–40. doi:10.1016/j.yjmcc.2009.03.019
Lee C. S., Yi J. S., Jung S. Y., Kim B. W., Lee N. R., Choo H. J., et al. (2010). Trim72 negatively regulates myogenesis via targeting insulin receptor substrate-1. Cell Death Differ. 17, 1254–1265. doi:10.1038/cdd.2010.1
Lemckert F. A., Bournazos A., Eckert D. M., Kenzler M., Hawkes J. M., Butler T. L., et al. (2016). Lack of mg53 in human heart precludes utility as a biomarker of myocardial injury or endogenous cardioprotective factor. Cardiovasc. Res. 110, 178–187. doi:10.1093/cvr/cvw017
Liu F., Song R., Feng Y., Guo J., Chen Y., Zhang Y., et al. (2015). Upregulation of MG53 induces diabetic cardiomyopathy through transcriptional activation of peroxisome proliferation-activated receptor α. Circulation 131, 795–804. doi:10.1161/CIRCULATIONAHA.114.012285
Liu J., Zhu H., Zheng Y., Xu Z., Li L., Tan T., et al. (2015). Cardioprotection of recombinant human mg53 protein in a porcine model of ischemia and reperfusion injury. J. Mol. Cell. Cardiol. 80, 10–19. doi:10.1016/j.yjmcc.2014.12.010
Ma H., Liu J., Bian Z., Cui Y., Zhou X., Zhou X., et al. (2015). Effect of metabolic syndrome on mitsugumin 53 expression and function. PLoS One 10, e0124128. doi:10.1371/journal.pone.0124128
Ma S., Wang Y., Zhou X., Li Z., Zhang Z., Wang Y., et al. (2020). MG53 protects hUC-MSCs against inflammatory damage and synergistically enhances their efficacy in neuroinflammation injured brain through inhibiting NLRP3/caspase-1/IL-1β Axis. ACS Chem. Neurosci. 11, 2590–2601. doi:10.1021/acschemneuro.0c00268
Matsuda C., Miyake K., Kameyama K., Keduka E., Takeshima H., Imamura T., et al. (2012). The c2a domain in dysferlin is important for association with mg53 (trim72). PLoS Curr. 4, e5035add8caff4. doi:10.1371/5035add8caff4
Matthay M. A., Ware L. B., Zimmerman G. A. (2012). The acute respiratory distress syndrome. J. Clin. Invest. 122, 2731–2740. doi:10.1172/JCI60331
McNeil P. L., Miyake K., Vogel S. S. (2003). The endomembrane requirement for cell surface repair. Proc. Natl. Acad. Sci. U. S. A. 100, 4592–4597. doi:10.1073/pnas.0736739100
Murry C. E., Jennings R. B., Reimer K. A. (1986). Preconditioning with ischemia: A delay of lethal cell injury in ischemic myocardium. Circulation 74, 1124–1136. doi:10.1161/01.cir.74.5.1124
Ng C. S., Wan S., Arifi A. A., Yim A. P. (2006). Inflammatory response to pulmonary ischemia-reperfusion injury. Surg. Today 36, 205–214. doi:10.1007/s00595-005-3124-2
Nicolson G. L. (2013). Update of the 1972 singer-nicolson fluid-mosaic model of membrane structure. Discov. (Craiova) 1, e3. doi:10.15190/d.2013.3
Organization W. H. (2020). The top 10 causes of death. Geneva, Switzerland: World Health Organization. https://www.who.int/news-room/fact-sheets/detail/the-top-10-causes-of-death (December 9, 2020).
Palencia G., Medrano J. A. N., Ortiz-Plata A., Farfan D. J., Sotelo J., Sanchez A., et al. (2015). Anti-apoptotic, anti-oxidant, and anti-inflammatory effects of thalidomide on cerebral ischemia/reperfusion injury in rats. J. Neurol. Sci. 351, 78–87. doi:10.1016/j.jns.2015.02.043
Park E. Y., Kwon O. B., Jeong B. C., Yi J. S., Lee C. S., Ko Y. G., et al. (2010). Crystal structure of pry-spry domain of human trim72. Proteins 78, 790–795. doi:10.1002/prot.22647
Pefanis A., Ierino F. L., Murphy J. M., Cowan P. J. (2019). Regulated necrosis in kidney ischemia-reperfusion injury. Kidney Int. 96, 291–301. doi:10.1016/j.kint.2019.02.009
Philouze C., Turban S., Cremers B., Caliez A., Lamarche G., Bernard C., et al. (2021). Mg53 is not a critical regulator of insulin signaling pathway in skeletal muscle. PLoS One 16, e0245179. doi:10.1371/journal.pone.0245179
Porter A. G., Janicke R. U. (1999). Emerging roles of caspase-3 in apoptosis. Cell Death Differ. 6, 99–104. doi:10.1038/sj.cdd.4400476
Reymond A., Meroni G., Fantozzi A., Merla G., Cairo S., Luzi L., et al. (2001). The tripartite motif family identifies cell compartments. EMBO J. 20, 2140–2151. doi:10.1093/emboj/20.9.2140
Saidi R. F., Kenari S. K. (2014). Liver ischemia/reperfusion injury: An overview. J. Invest. Surg. 27, 366–379. doi:10.3109/08941939.2014.932473
Saito A., Sato H., Iino N., Takeda T. (2010). Molecular mechanisms of receptor-mediated endocytosis in the renal proximal tubular epithelium. J. Biomed. Biotechnol. 2010, 403272. doi:10.1155/2010/403272
Saklayen M. G. (2018). The global epidemic of the metabolic syndrome. Curr. Hypertens. Rep. 20, 12. doi:10.1007/s11906-018-0812-z
Sarshoori J. R., Asadi M. H., Mohammadi M. T. (2014). Neuroprotective effects of crocin on the histopathological alterations following brain ischemia-reperfusion injury in rat. Iran. J. Basic Med. Sci. 17, 895–902.
Serracino-Inglott F., Habib N. A., Mathie R. T. (2001). Hepatic ischemia-reperfusion injury. Am. J. Surg. 181, 160–166. doi:10.1016/s0002-9610(00)00573-0
Shan D., Guo S., Wu H. K., Lv F., Jin L., Zhang M., et al. (2020). Cardiac ischemic preconditioning promotes mg53 secretion through h2o2-activated protein kinase c-delta signaling. Circulation 142, 1077–1091. doi:10.1161/CIRCULATIONAHA.119.044998
Shiraishi I., Melendez J., Ahn Y., Skavdahl M., Murphy E., Welch S., et al. (2004). Nuclear targeting of akt enhances kinase activity and survival of cardiomyocytes. Circ. Res. 94, 884–891. doi:10.1161/01.RES.0000124394.01180.BE
Simard J. M., Kent T. A., Chen M., Tarasov K. V., Gerzanich V. (2007). Brain oedema in focal ischaemia: Molecular pathophysiology and theoretical implications. Lancet. Neurol. 6, 258–268. doi:10.1016/S1474-4422(07)70055-8
Sommer S. P., Sommer S., Sinha B., Wiedemann J., Otto C., Aleksic I., et al. (2011). Ischemia-reperfusion injury-induced pulmonary mitochondrial damage. J. Heart Lung Transpl. 30, 811–818. doi:10.1016/j.healun.2011.02.001
Song R., Peng W., Zhang Y., Lv F., Wu H. K., Guo J., et al. (2013). Central role of e3 ubiquitin ligase mg53 in insulin resistance and metabolic disorders. Nature 494, 375–379. doi:10.1038/nature11834
Sweeney R. M., Griffiths M., McAuley D. (2013). Treatment of acute lung injury: Current and emerging pharmacological therapies. Semin. Respir. Crit. Care Med. 34, 487–498. doi:10.1055/s-0033-1351119
Tong H., Imahashi K., Steenbergen C., Murphy E. (2002). Phosphorylation of glycogen synthase kinase-3beta during preconditioning through a phosphatidylinositol-3-kinase-dependent pathway is cardioprotective. Circ. Res. 90, 377–379. doi:10.1161/01.res.0000012567.95445.55
Virani S. S., Alonso A., Benjamin E. J., Bittencourt M. S., Callaway C. W., Carson A. P., et al. (2020). Heart disease and stroke statistics-2020 update: A report from the American heart association. Circulation 141, e139–e596. doi:10.1161/CIR.0000000000000757
Wang Q., Bian Z., Jiang Q., Wang X., Zhou X., Park K. H., et al. (2020). Mg53 does not manifest the development of diabetes in db/db mice. Diabetes 69, 1052–1064. doi:10.2337/db19-0807
Wang Q., Park K. H., Geng B., Chen P., Yang C., Jiang Q., et al. (2022). Mg53 inhibits necroptosis through ubiquitination-dependent ripk1 degradation for cardiac protection following ischemia/reperfusion injury. Front. Cardiovasc. Med. 9, 868632. doi:10.3389/fcvm.2022.868632
Wang X., Xie W., Zhang Y., Lin P., Han L., Han P., et al. (2010). Cardioprotection of ischemia/reperfusion injury by cholesterol-dependent mg53-mediated membrane repair. Circ. Res. 107, 76–83. doi:10.1161/CIRCRESAHA.109.215822
Wang Z. V., Hill J. A. (2015). Diabetic cardiomyopathy: Catabolism driving metabolism. Circulation 131, 771–773. doi:10.1161/CIRCULATIONAHA.115.015357
Weisleder N., Takeshima H., Ma J. (2008). Immuno-proteomic approach to excitation-contraction coupling in skeletal and cardiac muscle: Molecular insights revealed by the mitsugumins. Cell Calcium 43, 1–8. doi:10.1016/j.ceca.2007.10.006
Weisleder N., Takizawa N., Lin P., Wang X., Cao C., Zhang Y., et al. (2012). Recombinant mg53 protein modulates therapeutic cell membrane repair in treatment of muscular dystrophy. Sci. Transl. Med. 4, 139ra85. doi:10.1126/scitranslmed.3003921
Wu H. K., Zhang Y., Cao C. M., Hu X., Fang M., Yao Y., et al. (2019). Glucose-sensitive myokine/cardiokine mg53 regulates systemic insulin response and metabolic homeostasis. Circulation 139, 901–914. doi:10.1161/CIRCULATIONAHA.118.037216
Xie H., Wang Y., Zhu T., Feng S., Yan Z., Zhu Z., et al. (2020). Serum mg53/trim72 is associated with the presence and severity of coronary artery disease and acute myocardial infarction. Front. Physiol. 11, 617845. doi:10.3389/fphys.2020.617845
Xie H., Yan Z., Feng S., Zhu T., Zhu Z., Ni J., et al. (2020). Prognostic value of circulating mg53 levels in acute myocardial infarction. Front. Cardiovasc. Med. 7, 596107. doi:10.3389/fcvm.2020.596107
Yao W., Li H., Han X., Chen C., Zhang Y., Tai W. L., et al. (2017). Mg53 anchored by dysferlin to cell membrane reduces hepatocyte apoptosis which induced by ischaemia/reperfusion injury in vivo and in vitro. J. Cell. Mol. Med. 21, 2503–2513. doi:10.1111/jcmm.13171
Yao Y., Zhang B., Zhu H., Li H., Han Y., Chen K., et al. (2016). Mg53 permeates through blood-brain barrier to protect ischemic brain injury. Oncotarget 7, 22474–22485. doi:10.18632/oncotarget.7965
Yellon D. M., Hausenloy D. J. (2007). Myocardial reperfusion injury. N. Engl. J. Med. 357, 1121–1135. doi:10.1056/NEJMra071667
Yi J. S., Park J. S., Ham Y. M., Nguyen N., Lee N. R., Hong J., et al. (2013). Mg53-induced irs-1 ubiquitination negatively regulates skeletal myogenesis and insulin signalling. Nat. Commun. 4, 2354. doi:10.1038/ncomms3354
Zager R. A., Jurkowitz M. S., Merola A. J. (1985). Responses of the normal rat kidney to sequential ischemic events. Am. J. Physiol. 249, F148–F159. doi:10.1152/ajprenal.1985.249.1.F148
Zhai Y., Busuttil R. W., Kupiec-Weglinski J. W. (2011). Liver ischemia and reperfusion injury: New insights into mechanisms of innate-adaptive immune-mediated tissue inflammation. Am. J. Transpl. 11, 1563–1569. doi:10.1111/j.1600-6143.2011.03579.x
Zhai Y., Petrowsky H., Hong J. C., Busuttil R. W., Kupiec-Weglinski J. W. (2013). Ischaemia-reperfusion injury in liver transplantation-from bench to bedside. Nat. Rev. Gastroenterol. Hepatol. 10, 79–89. doi:10.1038/nrgastro.2012.225
Zhang Y., Lv F., Jin L., Peng W., Song R., Ma J., et al. (2011). Mg53 participates in ischaemic postconditioning through the risk signalling pathway. Cardiovasc. Res. 91, 108–115. doi:10.1093/cvr/cvr029
Zhao Z. Q., Corvera J. S., Halkos M. E., Kerendi F., Wang N. P., Guyton R. A., et al. (2002). Inhibition of myocardial injury by ischemic postconditioning during reperfusion: Comparison with ischemic preconditioning. Am. J. Physiol. Heart Circ. Physiol. 285, H579–H588. doi:10.1152/ajpheart.01064.2002
Zheng Y. Q., Liu J. X., Wang J. N., Xu L. (2007). Effects of crocin on reperfusion-induced oxidative/nitrative injury to cerebral microvessels after global cerebral ischemia. Brain Res. 1138, 86–94. doi:10.1016/j.brainres.2006.12.064
Zhong W., Benissan-Messan D. Z., Ma J., Cai C., Lee P. H. U. (2021). Cardiac effects and clinical applications of mg53. Cell Biosci. 11, 115. doi:10.1186/s13578-021-00629-x
Zhou X., Chen M., Wang S., Yu L., Jiang H. (2015). Mg53 protein: A promising novel therapeutic target for myocardial ischemia reperfusion injury. Int. J. Cardiol. 199, 424–425. doi:10.1016/j.ijcard.2015.07.084
Keywords: MG53, ischemia/reperfusion injury (I/R injury), multiple organs, protective, review
Citation: Xu B, Wang C, Chen H, Zhang L, Gong L, Zhong L and Yang J (2022) Protective role of MG53 against ischemia/reperfusion injury on multiple organs: A narrative review. Front. Physiol. 13:1018971. doi: 10.3389/fphys.2022.1018971
Received: 14 August 2022; Accepted: 07 November 2022;
Published: 21 November 2022.
Edited by:
Tara MacDonald, Harvard Medical School, United StatesReviewed by:
Jose Antonio Adams, Mount Sinai Medical Center, United StatesXinyu Zhou, The Ohio State University, United States
Copyright © 2022 Xu, Wang, Chen, Zhang, Gong, Zhong and Yang. This is an open-access article distributed under the terms of the Creative Commons Attribution License (CC BY). The use, distribution or reproduction in other forums is permitted, provided the original author(s) and the copyright owner(s) are credited and that the original publication in this journal is cited, in accordance with accepted academic practice. No use, distribution or reproduction is permitted which does not comply with these terms.
*Correspondence: Lin Zhong, eWl6dW4xOTcxQDEyNi5jb20=; Jun Yang, eWFuZ2p5aGRAMTYzLmNvbQ==