- 1Department of Biomedical Sciences, University of Copenhagen, Copenhagen, Denmark
- 2Department of General Physiology, St. Petersburg State University, St. Petersburg, Russia
- 3Department of Biomedicine, Aarhus University, Aarhus, Denmark
- 4Marshall Institute for Interdisciplinary Research, Marshall University, Huntington, WV, United States
Background: Several local Ca2+ events are characterized in smooth muscle cells. We have previously shown that an inhibitor of the Na,K-ATPase, ouabain induces spatially restricted intracellular Ca2+ transients near the plasma membrane, and suggested the importance of this signaling for regulation of intercellular coupling and smooth muscle cell contraction. The mechanism behind these Na,K-ATPase-dependent “Ca2+ flashes” remains to be elucidated. In addition to its conventional ion transport function, the Na,K-ATPase is proposed to contribute to intracellular pathways, including Src kinase activation. The microtubule network is important for intracellular signaling, but its role in the Na,K-ATPase-Src kinase interaction is not known. We hypothesized the microtubule network was responsible for maintaining the Na,K-ATPase-Src kinase interaction, which enables Ca2+ flashes.
Methods: We characterized Ca2+ flashes in cultured smooth muscle cells, A7r5, and freshly isolated smooth muscle cells from rat mesenteric artery. Cells were loaded with Ca2+-sensitive fluorescent dyes, Calcium Green-1/AM and Fura Red/AM, for ratiometric measurements of intracellular Ca2+. The Na,K-ATPase α2 isoform was knocked down with siRNA and the microtubule network was disrupted with nocodazole. An involvement of the Src signaling was tested pharmacologically and with Western blot. Protein interactions were validated with proximity ligation assays.
Results: The Ca2+ flashes were induced by micromolar concentrations of ouabain. Knockdown of the α2 isoform Na,K-ATPase abolished Ca2+ flashes, as did inhibition of tyrosine phosphorylation with genistein and PP2, and the inhibitor of the Na,K-ATPase-dependent Src activation, pNaKtide. Ouabain-induced Ca2+ flashes were associated with Src kinase activation by phosphorylation. The α2 isoform Na,K-ATPase and Src kinase colocalized in the cells. Disruption of microtubule with nocodazole inhibited Ca2+ flashes, reduced Na,K-ATPase/Src interaction and Src activation.
Conclusion: We demonstrate that the Na,K-ATPase-dependent Ca2+ flashes in smooth muscle cells require an interaction between the α2 isoform Na, K-ATPase and Src kinase, which is maintained by the microtubule network.
Introduction
The Na,K-ATPase extrudes three Na+ ions from the cell in exchange for two K+ ions, powered by the hydrolysis of one ATP molecule (Blanco and Mercer, 1998). This ion transport is essential for maintaining the transmembrane ion gradient, which is important for the cell membrane potential and multiple secondary active transports. The Na,K-ATPase is the heterodimeric plasma membrane protein that consists of, at least, α- and β-subunits (Geering, 2008). The α subunit is responsible for ion translocation and catalytic activities of the enzyme (Blanco et al., 1994). It contains the binding sites for ATP and specific inhibitors of the Na,K-ATPase, cardiotonic steroids. Four isoforms of the α subunit have been identified (Blanco and Mercer, 1998) and their tissue-specific expression profile has been described (Matchkov and Krivoi, 2016). Vascular smooth muscle cells co-express both the α1 and α2 isoforms of Na,K-ATPase but they have been proposed to serve different functions (Matchkov, 2010; Blaustein et al., 2016). Importantly, while the housekeeping α1 isoform Na,K-ATPase is homogeneously distributed over cell membrane and nearly saturated at resting conditions, the α2 isoform forms membrane microdomains and is involved in numerous signaling functions (Matchkov, 2010; Zhang et al., 2019; Blaustein and Hamlyn, 2020). In rodent tissues, the α1 and α2 isoforms of Na,K-ATPase can be differentiated pharmacologically because of their different affinity to cardiotonic steroid, ouabain, i.e., the α2 isoform is approximately 100-fold more sensitive to ouabain than the α1 isoform (Blanco and Mercer, 1998). That is, the rodent α2 isoform can be inhibited by sub-micromolar and micromolar concentrations of ouabain, while 500 μM–1 mM ouabain is needed to inhibit the α1 isoform Na,K-ATPase.
The Na,K-ATPase was also proposed as a plasma membrane receptor that initiates intracellular signaling pathways upon binding with some cardiotonic steroids, including ouabain (Aizman and Aperia, 2003; Aperia, 2007). It has been shown that binding of ouabain to the Na,K-ATPase leads to Src kinase auto-phosphorylation and, thus, its activation (Haas et al., 2002), which initiates several downstream intracellular signaling pathways (Xie, 2003). Although the mechanism of this Na,K-ATPase-dependent Src kinase activation remains debated (Li and Xie, 2009; Weigand et al., 2012; Yosef et al., 2016; Cui and Xie, 2017), its consequences for vascular functions were reported (Zulian et al., 2013; Hangaard et al., 2017; Bouzinova et al., 2018; Staehr et al., 2018; Zhang et al., 2018). In the vascular wall, micromolar concentrations of ouabain inhibit only the α2 isoform Na,K-ATPase and have no significant effect on the global intracellular Ca2+ in smooth muscle cells (Mulvany et al., 1984; Aalkjaer and Mulvany, 1985; Matchkov et al., 2007; Matchkov et al., 2012). However, these concentrations of ouabain also potentiate vascular tone and vasocontraction (Mulvany et al., 1982; Aalkjaer and Mulvany, 1985; Matchkov et al., 2007), and increase blood pressure in vivo (Yuan et al., 1993; Zhang et al., 2005). We have recently demonstrated that these effects of ouabain mediate via Src kinase signaling in the vascular wall (Hangaard et al., 2017; Bouzinova et al., 2018; Staehr et al., 2018).
In previous studies, micromolar concentrations of ouabain did not affect the global intracellular Ca2+ concentration (Mulvany et al., 1984; Aalkjaer and Mulvany, 1985; Matchkov et al., 2007; Matchkov et al., 2012), but did induce spatially restricted Ca2+ transients in cultured aortic smooth muscle cells and in rat mesenteric artery wall (Matchkov et al., 2007). These Ca2+ transients are suggested to be important to control intercellular coupling and vascular tone (Matchkov et al., 2007; Matchkov et al., 2012), but the underlying mechanism is unknown.
Microtubules are an integral component of the cytoskeleton, which mediates many cellular functions, including intracellular signaling (Jepps, 2021). It has been shown that Na,K-ATPase activity can be modulated by microtubules and might serve as an anchorage site at the plasma membrane (Alonso et al., 1998; Casale et al., 2003; Arce et al., 2008). Although the role of the microtubule network in modulation of Src kinase signaling was proposed (Abu-Amer et al., 1997; Yamada et al., 2000; Wu et al., 2008), its importance in this pathway in smooth muscle cells is still unknown. Moreover, whether the microtubule network plays an important role in Na,K-ATPase-dependent Src kinase signaling (Cui and Xie, 2017) remains to be elucidated.
In this study, we aimed to characterize the mechanism behind spatially restricted sub-membrane Ca2+ transients induced in smooth muscle cells by micromolar concentrations of ouabain. Specifically, we have questioned whether these Ca2+ transients are a direct product of local ion homeostasis disbalance because of ion transport inhibition (Blaustein and Lederer, 1999) or a result of the Na,K-ATPase-dependent intracellular signal transduction (Cui and Xie, 2017). We also questioned here the importance of the microtubule network for generation of Ca2+ transients and its interplay with potential signalling pathways. We have addressed these research questions in cultured aortic smooth muscle cells, A7r5 that were originally used to characterize the ouabain-induced Ca2+ transients (Matchkov et al., 2007), and validated our findings in vascular smooth muscle cells isolated from rat mesenteric arteries. We studied the changes in Ca2+ transients produced by a modulation of ion transport by the Na,K-ATPase, by pharmacological intervention with tyrosine phosphorylation and by the α2 isoform Na,K-ATPase downregulation. Involvement of signal transduction was validated with proximity ligation assay and phosphoprotein-specific Western blot analyses. The functional role of microtubule network was studied with pharmacological disruption by nocodazole.
Methods
Intracellular Ca2+ imaging in cultured rat aortic smooth muscle cells—A7r5
Mycoplasma free A7r5 (American Type Culture Collection - ATCC; VA, United States) were cultured in DMEM medium (In Vitro, Denmark) supplemented with 10% fetal calf serum, 1% L-glutamine and 0.1% KPS (kanamycin 2 g, penicillin 1 million IU, streptomycin 1 g in 20 ml phosphate-buffered saline [PBS, in mM: NaCl 137, KCl 2.7, Na2HPO4 8.2, KH2PO4 1.8, at pH 7.4)). Confluent A7r5 cells were detached by nonenzymatic cell dissociation solution (Sigma-Aldrich, Denmark), resuspended in DMEM medium as above and pipetted into 200 µl customer-made imaging chambers with cover glass bottom. The cells were stored for 3 h in humidified 37°C/5% CO2 cell incubator to allow the cells to attach to the bottom prior the experiment imaging experiment.
To measure changes of intracellular Ca2+ transients, the cells were loaded with a mixture of Calcium Green-1/AM (3 μM) and Fura Red/AM (6 μM) for 15 min at 37°C/5% CO2. Both dyes were initially dissolved in DMSO maintaining final concentration ≤0.01%. Calcium Green-1/AM and Fura Red/AM mixture was initially prepared in physiological salt solution (PSS, in mM: 119 NaCl, 3.0 KCl 1.18 KH2PO4, 1.17 MgCl2, 25.0 NaHCO3, 0.026 EDTA and 5.5 glucose, gassed with 5% CO2 in air and adjusted to pH 7.4) and this solution then replaced the cell medium in the imaging chamber.
Prior the imaging protocol, the cells were washed five times with warmed to 37°C PSS that was gassed with 5% CO2 in air. The experiments were performed using an inverted confocal laser scanning microscope (LSM 5 Pa Exciter or LSM780, Zeiss GmbH, Germany) and images were acquired with a water immersion objective (×63, 1.2 W, Zeiss GmbH, Germany).
Both Fura Red and Calcium Green-1 were excited at 488 nm, the emission from dyes was collected above 560 nm and in the interval from 505 to 530 nm, respectively. The elevation in intracellular Ca2+ resulted in increased fluorescence intensity of Calcium Green-1 and decreased fluorescence intensity of Fura Red. Therefore, a combination of these two Ca2+ indicators was used for ratiometric assessment of intracellular Ca2+ changes, as described previously (Matchkov et al., 2007).
PSS in the imaging chamber was kept at 37°C by thermostatically controlled chamber holder and constantly gassed with 5% CO2 in air. The experimental protocol consisted of 5 min baseline recordings, and the pharmacological intervention by replacement of the solution in the chamber to another modified solution and followed by incubation for 15 min prior next 5 min imaging session. Up to three pharmacological interventions per single experimental protocol were done.
Changes in intracellular Ca2+ were analyzed using either the Zeiss LSM Image Examiner program or ZEN software (Zeiss GmbH, Germany). The local Ca2+ events were analyzed as a profile of fluorescence ratio (Calcium Green-1 over Fura Red) either at cell perimeter or in the center of the cell, and plotted for the profile distance, as described previously (Matchkov et al., 2007). Ca2+ transients were counted if elevation of intracellular Ca2+ was 5 or more times above averaged baseline. The number of Ca2+ transients was normalized to the length of profile the fluorescence was measured. The analysis was done blind with custom-made Makro in Excel Spreadsheet Software (Microsoft corp., NM, United States).
siRNA transfection of A7r5 cells
Transfection of A7r5 cells was done as described previously (Matchkov et al., 2008). The siRNA directed against the α2 isoform (Ambion; the sense sequence: 5′-GAGAACATCTCCGTGTCAtt-3′) and another control, nonrelated siRNA directed against enhanced green fluorescent protein (Ambion; the sense sequence: 5′-CCACUACCUGAGCACCCAGtt-3′) were used as previously described (Matchkov et al., 2012). When A7r5 cells were 80% confluent, antibiotics were omitted from DMEM medium for 2 h. The cells were then transfected by TransIT TKO transfection kit (Mirus Bio Co., United States) following the protocol given by the manufacturer. Each culture dish was transfected with 5 μl TKO and 25 nmol/L siRNA in antibiotic-free DMEM medium for 8 h, then the cells were washed out and the medium was replaced back to standard DMEM. The cells were used 72 h later for functional and expressional analyses.
Semi-quantification of the Na,K-ATPase expression
Confluent A7r5 cells were washed in the culture dishes (Falcon, Becton Dickson, Denmark) 3 times in ice-cold PBS solution and collected by scrubbing. The cell suspension was centrifuged at 3,000-g and collected pellet was homogenized in lysis buffer (in mM: Tris‐HCl 10, sucrose 250, EDTA 1, EGTA 1; Triton X‐100 2%, pH 7.4; 1 tablet protease inhibitor per 10 ml and 0.01 mmol−1 of Halt’s phosphatase inhibitor cocktail) and 2x trisglycine sodium dodecyl sulphate (SDS) sample buffer (Invitrogen, MA, United States) with 1 mol/L DTT. The homogenates were ultrasonicated for 45 s and centrifuged at 10,000-g for 10 min at 4°C. The supernatants were collected, and the protein contents were determined using a bicinchoninic acid protein assay reagent kit (Pierce, United States). The sample was adjusted then with 1 M DTT and 2x Tris-glycine SDS sample buffer (Invitrogen, MA, United States) with an approximate ratio of 10:3:3.
Proteins (10 µg) were separated on 14% Tris-glycine gels and electrotransferred onto nitrocellulose membranes, which were then blocked by incubation in 5% nonfat dry milk in PBS with 0.5% vol/vol Tween 20 (PBS-T). The membrane was divided at approximately 64 kDa and the upper part of the membrane was incubated with primary α2 isoform Na,K-ATPase antibody (1:2,000; Millipore, United States [Catalog no. AB9094]) overnight at 5°C in PBS-T. The lower part of the membrane was incubated with pan-actin antibody (1:2,000, Cell Signaling Technology Inc., United States [Catalog no.4968]). Next day, the membranes were washed and incubated with horseradish peroxidase-conjugated secondary antibody (Dako, Denmark) for 1 h and bound antibody was detected by an ECL chemiluminescence kit (Amersham, United Kingdom).
Total protein lysates from rat mesenteric arteries were homogenized in 200 μl of RIPA buffer (in mM): 50 Tris pH 8.5, 150 NaCl, 1% SDS, 1% Nonidet P40, 0.5% Sodium deoxycholate and protease inhibitor cocktail (Roche, Switzerland) for 10 min at 4°C. The supernatant was collected after centrifugation at 11,000-g for 10 min at 4°C followed by protein quantitation using a bicinchoninic acid (BCA) Protein Assay kit (Thermo Fisher Scientific, MA, United States). 20 µg of protein were loaded on SDS-PAGE gels (4%–12% bis–tris; Invitrogen, MA, United States), subjected to electrophoresis, and then transferred onto a polyvinylidene fluoride (PVDF) membrane (Immobilon®-FL, Sigma-Aldrich, Denmark). The membrane was probed with antibodies against Na,K-ATPase α2 isoform (1:2,000; Millipore, United States [Catalog no. AB9094]) and GAPDH (1:10,000; Abcam, United Kingdom [Catalog no.ab181602]). Fluorescently conjugated secondary antibodies (1:10,000; Li-Cor Biosciences [Catalog no.926-32211 and 926-32210]) were used to visualize protein bands. The membrane was imaged and analysed on the Odyssey Infrared Imaging System (Li-Cor Biosciences; version 5.2.5).
The protein expression was semi-quantified as a ratio of intensity of bands for the α2 isoform Na,K-ATPase and either pan-actin or GAPDH proteins. Analysis was done with the ImageJ (National Institutes of Health, MD, United States). The normalized protein ratio is shown in percentage of mean ratio in the control group.
Semi-quantification of Src kinase signaling
A7r5 cells were lysed in lysis buffer as above. Ten µg of protein in Laemmli sample buffer (Bio‐Rad, Hercules, CA) was loaded onto 4–20% precast polyacrylamide stain‐free gels (Criterion TGX Stain‐free precast gel; Bio‐Rad). Total protein load was detected on the stain‐free gels using UV light in imaging system (c600; Azur Biosystems, Dublin, CA). The proteins were electrotransferred onto nitrocellulose membranes that were blocked in 3% bovine serum albumin (BSA) in Tris‐buffered solution (TBS, mmol L−1: 10 Tris‐HCl, 100 NaCl; pH 7.6) with 0.5% Tween-20 (TBST) to identify the phosphorylated Src or in 0.3% iBlock in TBS to identify total Src kinase. Membranes were incubated overnight at 4°C with primary antibodies against phosphorylated pY416 Src (1:500; Cell Signaling Technology Inc., MA, United States [Catalog no. CST-6943]) or total Src (1:200; Santa Cruz Biotechnology, TX, United States [Catalog no. sc-8056]). Next day, the membranes were incubated with horseradish peroxidase (HRP) conjugated secondary antibody (1:2000; Dako, Denmark) for 2 h at room temperature, and bound antibodies were detected by an enhanced chemiluminescence kit (ECL, Amersham, United Kingdom). Analysis was done with ImageJ (National Institutes of Health, MD, United States). Detected protein was normalized as a ratio to total protein load measured in the membrane for the same sample and expressed either as percentage of mean ratio in the control group or as a relative level of phosphorylated Src form over the total expression level of Src kinase.
Proximity ligation assay
PLA technique was used to determine the co-localization of the α2 isoform Na,K-ATPase with Src kinase in cultured A7r5 cells and freshly isolated rat mesenteric artery myocytes. Duolink in situ PLA detection kit 563 (Sigma-Aldrich, Denmark) was used in accordance with the manufacturer’s instructions. Cell isolation from third-order rat mesenteric arteries was conducted as described previously (Jepps et al., 2015). Briefly, cells were fixed in 4% paraformaldehyde in PBS for 20 min and permeabilized in PBS containing 0.1% of Triton X-100 for 5 min. Cells were incubated for 1 h at 37°C in Duolink blocking solution to avoid any unspecific binding. The primary antibodies used against the α2 isoform Na,K-ATPase (1:100; Millipore, United States [Catalog no. 07-674]) and Src (1:100; Santa Cruz Biotechnology, TX, United States [Catalog no. sc-8056]) were diluted in Duolink blocking solution and incubated overnight at 4°C. Combinations of secondary anti-rabbit or anti-mouse antibodies of PLA PLUS and MINUS probes were used followed by hybridization, ligation and amplification steps. Red punctae representing proteins that are located within 40 nm of each other were visualized and quantified using a Zeiss LSM710 upright laser scanning confocal microscope.
Animals
All animal experiments were performed in accordance with Directive 2010/63EU on the protection of animals used for scientific purposes and approved by the national ethics committee, Denmark. Male Wistar rats were purchased from Janvier Labs (France), group-housed in clear plastic containers and underwent at least 1 week of habituation before use. All experiments with dissected mesenteric arteries were performed using 12- to 15-week-old male Wistar rats.
Reagents
Calcium Green-1/AM and Fura Red/AM were obtained from Invitrogen (MA, United States). Nocodazole was obtained from Tocris (United Kingdom). The pNaKtide peptide was obtained from HD Biosciences (China) and stock solution was prepared in water (10 mM) and stored at −20°C. All other chemicals were purchased from Sigma-Aldrich (Denmark). Stock solutions of nocodazole, genistein and PP2 were prepared in DMSO (10 mM) and stored at −20°C. Ouabain stock solution was prepared on the day of experiment (minimum 2 h prior to application) in a concentration of mM in water. Drugs were applied a minimum 15 min prior to measurements/interventions.
Statistical analysis
All statistical analysis was performed using GraphPad Prism 8 (GraphPad Software Inc., San Diego, CA, United States). Different statistical tests were performed throughout the present study, as appropriate, and are defined in the Results section for each data set analysed. The data were subjected to a normality test (Shapiro-Wilk test) that defines the use of either parametric or nonparametric statistical analysis. All data are presented as means ± standard error of the mean (SEM). A probability (P) level of <0.05 was considered significant, and n refers to number of experiments in case of cell culture. The number of culture dishes used at the day of experiment is also indicated. When mesenteric arteries were studied, n refers to number of cells and the number of rats is also provided.
Results
Ouabain-induced Ca2+ flashes depend on the expression of Na,K-ATPase α2 isoform
In A7r5 cells, superfusion with 10 µM ouabain induced spatially restricted [Ca2+]i transients—“[Ca2+]i flashes” (Figure 1A). Similar to a previous report (Matchkov et al., 2007), these Ca2+ flashes were only detected on the cell periphery (Figure 1B), while no ouabain-induced Ca2+ fluctuations were seen in the center of the cells (Figure 1C). Transfection of A7r5 cell with siRNA directed against the α2 isoform suppressed the protein expression of the α2 isoform Na,K-ATPase compared to cells transfected with non-targeted siRNA (Figure 1D&E). The cells transfected with non-targeted siRNA were still able to increase submembrane Ca2+ flashes upon administration of ouabain (Figure 1F). In contrast, A7r5 cells transfected with siRNA targeted against the Na,K-ATPase α2 isoform did not show any ouabain-induced Ca2+ flashes (Figure 1G). The effect of ouabain was concentration dependent. Ca2+ flashes were induced by ouabain at 10−6 and 10−5 M while no significant effect of 10−7 M was seen (Figure 1H).
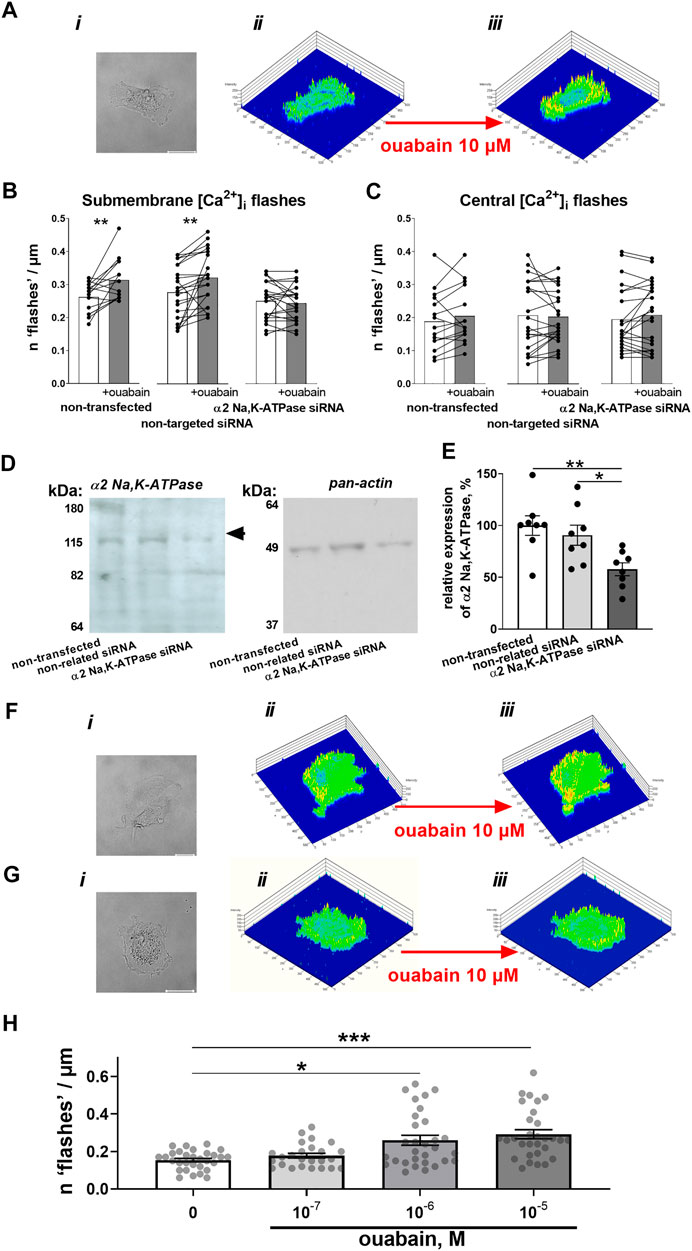
FIGURE 1. Ouabain induces Ca2+ flashes that depend on the expression of the α2 isoform Na,K-ATPase. A7r5 cells (Ai) were loaded with Ca2+ sensitive dyes, Calcium Green-1 and Fura Red, which ratio represents relative intracellular Ca2+ changes. This ratio was assessed under control conditions (Aii) and after incubation with 10 µM ouabain (Aiii). Exposure to ouabain induced significant elevation of submembrane Ca2+ flashes in non-transfected cells (n = 15 of 3 cultures) and cells transfected with non-targeted siRNA (n = 25 of 3 cultures), while cells transfected with siRNA directed against α2 isoform Na,K-ATPase did not show any changes (n = 22 of 3 cultures) (B). No significant changes of intracellular Ca2+ activity in the center of cell was seen in any of the groups (C). The transfection with siRNA directed against α2 isoform Na,K-ATPase but not with non-targeted siRNA reduced the expression of α2 isoform Na,K-ATPase (n = 8) [(D)—representative Western blot, (E) averaged results]. Representative cell images (i), and Calcium Green-1/Fura Red intensity ratio under control conditions (ii) and in the presence of ouabain (iii) for A7r5 cells transfected with non-targeted siRNA (F) and siRNA directed against the α2 isoform Na,K-ATPase (G). The effect of ouabain on the Ca2+ flashes was concentration-dependent [(H); n = 25–30 of 9 cultures]. *, ** and ***, p < 0.05, 0.01 and 0.001 (two-way ANOVA followed by Sidak’s multiple comparisons test in B and C; one-way ANOVA followed by Tukey’s multiple comparisons test in E and Kruskal–Wallis’ test followed by Dunn’s multiple comparison test in (H).
The Na,K-ATPase ion transport is not essential for Ca2+ flashes
We have tested the possibility that Ca2+ flashes originated from changes in activity of the Na+,Ca2+-exchanger, because of inhibition of Na+ extrusion by the Na,K-ATPase with ouabain (Blaustein and Lederer, 1999). When the Na,K-ATPase ion transport was modulated by changes in the extracellular concentrations of transported K+ and Na+ cations, the ability of ouabain to elicit the intracellular Ca2+ flashes was still preserved (Figure 2). Omission of extracellular K+ suppresses the ion pumping by Na,K-ATPase (Holmgren et al., 2000). However, ouabain-induced Ca2+ flashes were not affected (Figure 2A). Reduction of extracellular Na+ to 50 mM, which is expected to potentiate the Na,K-ATPase ion transport, reduced the potency of ouabain to induce the intracellular Ca2+ flashes but did not prevent them (Figure 2B).
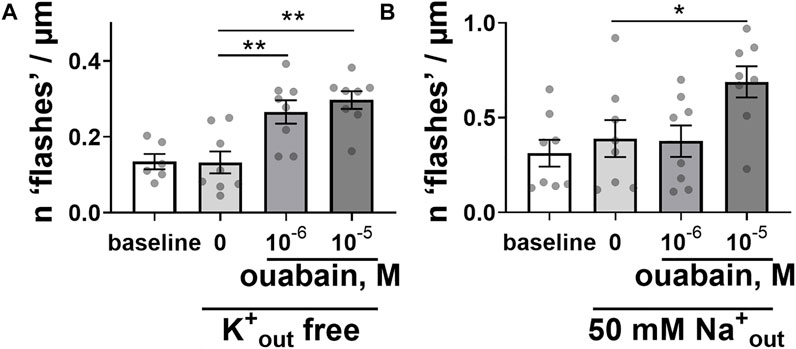
FIGURE 2. Modulation of the Na,K-ATPase ion transport activity did not prevent the ability of ouabain to induce intracellular Ca2+ flashes. Ouabain induced Ca2+ flashes after omission of K+ ions from extracellular solution [(A); n = 6–8 of 5 cultures], and a reduction of extracellular Na+ concentration to 50 mM [(B); n = 8 of 6 cultures]. * and **, p < 0.05 and 0.01 (one-way ANOVA followed by Sidak’s multiple comparisons test).
Activation of the Ca2+ flashes by ouabain requires Src kinase activation by phosphorylation
Micromolar concentrations of ouabain are known to induce auto-phosphorylation and activation of non-receptor tyrosine kinase, Src (Hangaard et al., 2017). We tested the involvement of this pathway in generation of ouabain-induced Ca2+ flashes. An unspecific tyrosine kinase inhibitor, genistein prevented ouabain-induced Ca2+ flashes in A7r5 cells (Figure 3A). Ouabain was also unable to induce Ca2+ flashes in the presence of relatively selective Src family kinase inhibitor, PP2 (Bain et al., 2003) (Figure 3B). Furthermore, a specific inhibitor of the Na,K-ATPase-dependent Src kinase activation with pNaKtide (Li et al., 2009) also prevented the Ca2+ flashes induced by ouabain (Figure 3C).
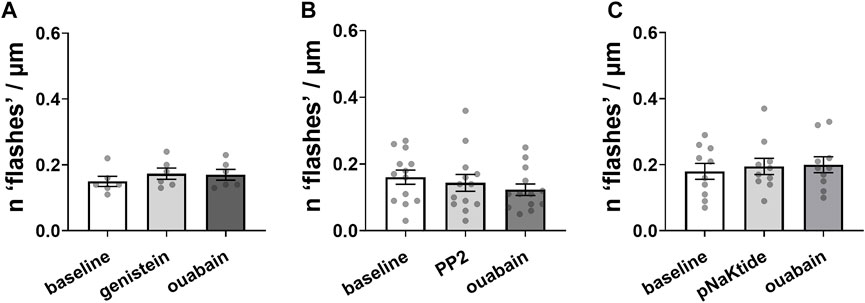
FIGURE 3. Ouabain-induced Ca2+ flashes depend on Src kinase activation by phosphorylation. Inhibition of tyrosine phosphorylation by 50 µM genistein [(A); n = 6 of 3 cultures] or inhibition Src kinase activation with 10 µM PP2 [(B); n = 13 of 7 cultures] prevented Ca2+ flashes induced by 10 µM ouabain. Ca2+ flashes were also not seen in the presence of a specific inhibitor of the Na,K-ATPase-dependent Src kinase activation, pNaKtide (1 µM) [(C); n = 10 of 6 cultures]. The data are compared with a one-way ANOVA followed by Sidak’s multiple comparisons test.
Microtubule network keeps the α2 isoform Na,K-ATPase and Src kinase together allowing the generation of Ca2+ flashes
An interaction between the Na,K-ATPase and tubulin, the main component of the microtubules, has previously been reported (Casale et al., 2003; Zampar et al., 2009). As the microtubule network is considered to play an important role in signal transduction (Gundersen and Cook, 1999), we hypothesized that it might be also involved in the generation of ouabain-induced Ca2+ flashes and tested this hypothesis in A7r5 cells (Figure 4A). We found a close proximity (< 40 nm) of the α2 isoform Na,K-ATPase and Src kinase (Figure 4B). Pre-incubation with nocodazole disrupted microtubules (Lindman et al., 2018) and reduced the number of co-localizations between the α2 isoform Na,K-ATPase and Src kinase compared to control, while ouabain treatment was without any effect (Figures 4A,B). When analysis was done only at the cell edge, the cells pre-treated with nocodazole had reduced co-localization the α2 isoform Na,K-ATPase and Src kinase in comparison with control cells (Figure 4C). This was not associated with changes in the α2 isoform Na,K-ATPase expression, which was similar in A7r5 cells under control conditions and after pre-treatment with nocodazole (Figures 4D,E).
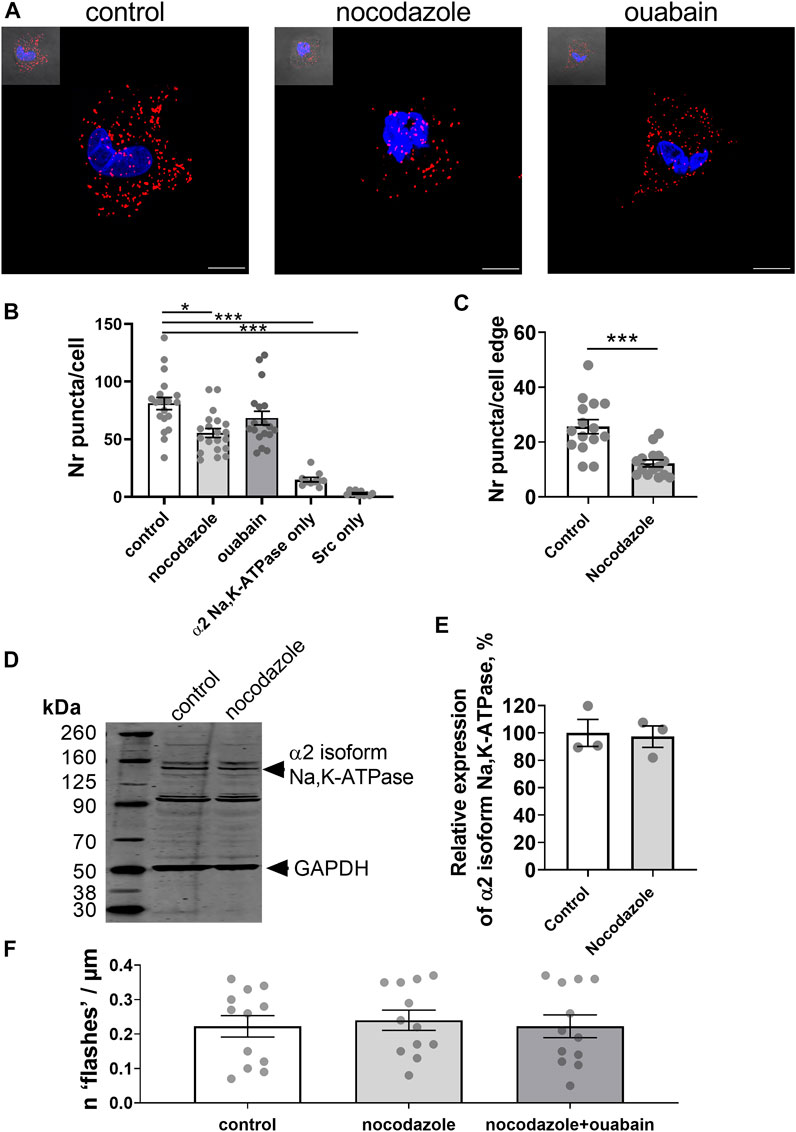
FIGURE 4. Microtubule network enables an interaction between the α2 isoform Na,K-ATPase and Src kinase, and the generation of Ca2+ flashes in A7r5 cells. Representative images of proximity ligation assay in A7r5 cells under control conditions or treated with nocodazole (10 µM) or with ouabain (10 µM) [(A); scale bar = 10 µm]. Red punctae indicate a close (< 40 nm) proximity between the α2 isoform Na,K-ATPase and Src kinase. Nuclei are stained with DAPI and shown in blue. Quantification of the red punctae in the experiment as above [(B); n = 18–20 of 4 cultures]. The red punctae were also counted only at the cellular edge (C) and nocodazole significantly decreased punctae in comparison with the control (n = 15 of 3 cell cultures). Representative Western blot detected the α2 isoform Na,K-ATPase in lysates of A7r5 cell under control conditions and treated with nocodazole (D). GAPDH protein was stained for loading control. Quantification of the α2 isoform Na,K-ATPase expression under control conditions and after incubation with nocodazole [(E); n = 3 of 3 cultures]. Nocodazole (10 µM) abolished the ouabain-induced Ca2+ flashes [(F); n = 12 of 4 cultures]. * and ***, p < 0.05 and 0.001 (Kruskal–Wallis’ test followed by Dunn’s multiple comparison test in B, unpaired t test in C and E, and one-way ANOVA followed by Sidak’s multiple comparisons test in F).
To address the functional importance of an intact microtubule network for generation of ouabain-induced Ca2+ flashes, A7r5 cells were pre-incubated with nocodazole prior to stimulation with ouabain. Ouabain failed to induce Ca2+ flashes after disruption of microtubules with nocodazole (Figure 4F).
Experiments on freshly isolated rat mesenteric artery myocytes (Figure 5A) supported our findings from A7r5 cells (Figure 4). Nocodazole significantly decreased the number of co-localizations between the α2 isoform Na,K-ATPase and Src kinase in freshly isolated vascular smooth muscle cells (Figure 5B), but ouabain did not alter the number of interactions (Figure 5B). Nocodazole also decreased the co-localization at the cell edge (Figure 5C). Western blot analysis of rat mesenteric artery lysates showed that nocodazole did not change the total protein expression of the α2 isoform Na,K-ATPase (Figures 5D,E). Altogether, these results suggest that an intact microtubule network is required to maintain the α2 isoform Na,K-ATPase and Src kinase together allowing Src activation upon binding ouabain to the Na,K-ATPase.
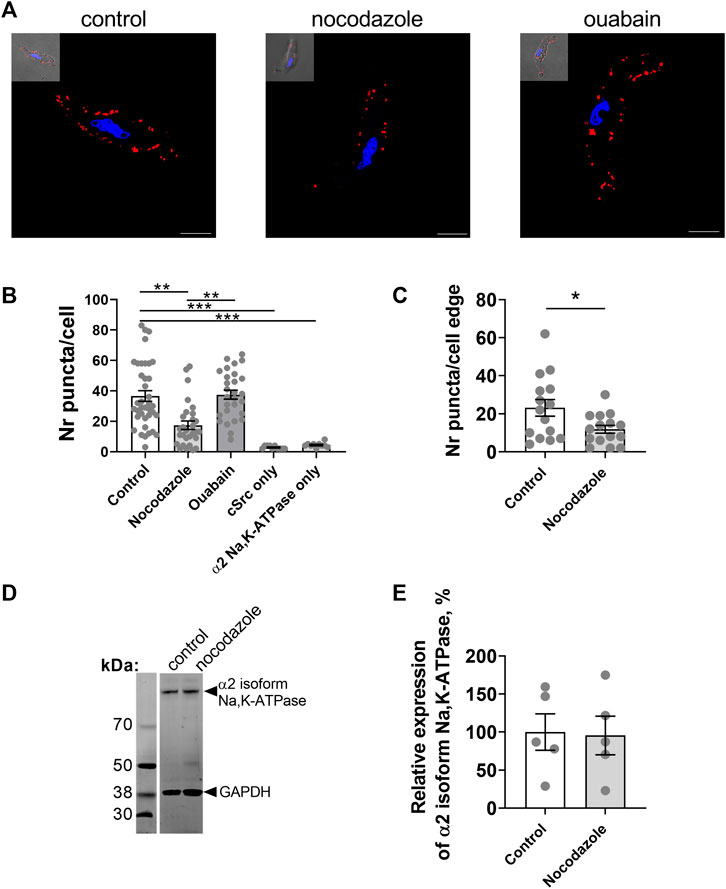
FIGURE 5. The interaction between the α2 isoform Na,K-ATPase and Src kinase in freshly isolated smooth muscle cells from rat mesenteric small artery is controlled by microtubule network. Representative images of proximity ligation assay in myocytes under control conditions and treated with either nocodazole (10 µM) or with ouabain (10 µM) [(A); scale bar = 10 µm]. Red punctae indicate a close proximity (< 40 nm) between the α2 isoform Na,K-ATPase and Src kinase. Nuclei are stained with DAPI and shown in blue. Quantification of the red punctae in the experiment as in (A) is shown in [(B); 10–37 cells from 3–4 rats]. At the cell edge nocodazole significantly decreased the red punctae in comparison with the control [(C); 15 cells from 3 rats]. Representative Western blot with rat mesenteric arteries lysates for the α2 isoform Na,K-ATPase under control conditions and treated with nocodazole (D). GAPDH protein was stained for loading control. Quantification of the α2 isoform Na,K-ATPase expression under control conditions and after incubation with nocodazole [(E); n = 5]. *, ** and ***, denote p < 0.05, 0.01 and 0.001, respectively (Kruskal–Wallis’ test followed by Dunn’s multiple comparison test in B and unpaired t test in C and E (p = 0.90).
Nocodazole reduced Src phosphorylation and prevented ouabain induced Src phosphorylation
Incubation with either nocodazole, ouabain, vehicle (DMSO), or with a combination of nocodazole and ouabain did not change the total Src content of A7r5 cells (Figures 6A,C). However, nocodazole reduced relative Src phosphorylation (Figures 6B,C). Moreover, although ouabain administration elevated relative Src phosphorylation, this was not seen in the presence of nocodazole (Figure 6C).
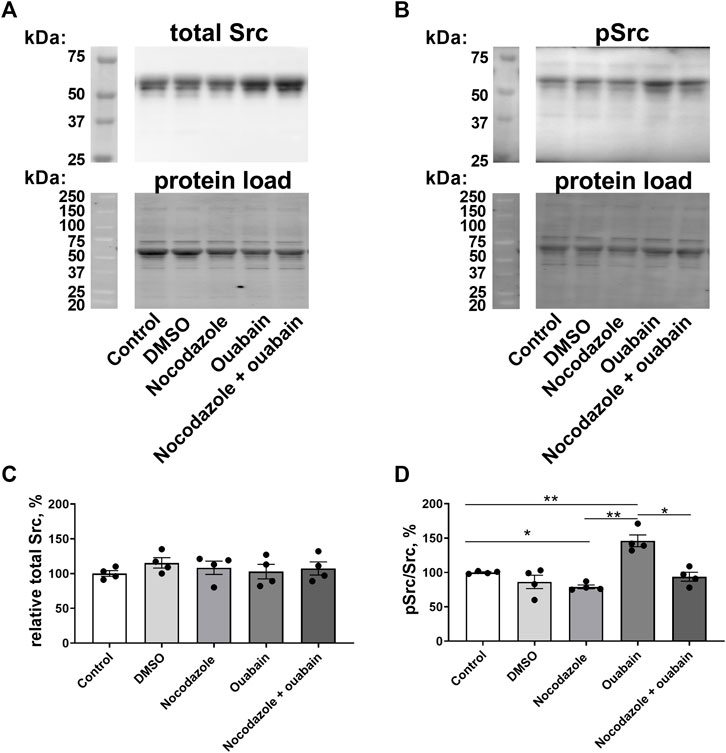
FIGURE 6. Ouabain increased Src kinase phosphorylation, while nocodazole prevented this increase. Representative blots from Western blot experiments where membranes were stained with either antibodies against total Src kinase (A) or with antibody against phosphorylated Src (B). Lower panels in (A,B) show total protein load detected with stain-free membrane. Semi-quantification of total Src expression in A7r5 cells under control conditions, exposed to vehicle (DMSO), pre-incubated with nocodazole (10 µM), with ouabain (10 µM) or with a combination of nocodazole and ouabain (C). Src phosphorylation relative to total Src content under control conditions, exposed to vehicle (DMSO), pre-incubated with nocodazole (10 µM), with ouabain (10 µM) or with a combination of nocodazole and ouabain (D). n = 4, * and **, p < 0.05 and 0.01 (one-way ANOVA followed by Sidak’s multiple comparisons test in (C,D).
Discussion
We showed previously that low concentrations of ouabain induced spatially restricted submembrane [Ca2+]i transients in cultured aortic smooth muscle cells (Matchkov et al., 2007) and suggested the importance of these Ca2+ flashes for synchronization of contraction and intercellular coupling in the vascular wall (Matchkov et al., 2012; Hangaard et al., 2017). In the present study, we demonstrate that the generation of Ca2+ flashes requires the α2 isoform Na,K-ATPase, Src kinase and an intact microtubule network.
The α2 isoform Na,K-ATPase-dependent activation of Src kinase is essential for generation of ouabain-induced Ca2+ flashes in smooth muscle cells
In this study, we show that Ca2+ flashes can be induced with 1 µM and higher concentrations of ouabain and suggest a primary role of Src activation for Ca2+ flash generation, although some modulatory role of membrane potential and trans-membrane ion translocation cannot be excluded completely. Accordingly, it has been suggested previously that ouabain mediates, at least in part, its pro-contractile and pro-hypertensive effects via ion transport independent signaling from the Na,K-ATPase Src activation (Yuan et al., 1993; Song et al., 2014).
Some controversy regarding the involvement of α1 and α2 isoforms of the Na,K-ATPase has been reported previously (Matchkov et al., 2012; Xie et al., 2015; Bouzinova et al., 2018; Staehr et al., 2018; Yu et al., 2018; Kutz et al., 2021). Although a difference in the studied tissues can contribute to the variability, previous studies demonstrated clearly that Src kinase has highest affinity to specific protein-domain on the α1 isoform (Tian et al., 2006; Li et al., 2009; Xie et al., 2015; Yu et al., 2018). However, we found in this study that a specific knock-down of the α2 isoform Na,K-ATPase abolished the ouabain-induced Ca2+ flashes in A7r5 cells. This cannot be because of associated changes in the expression of α1 isoform, as this siRNA knockdown protocol did not affect the expression of α1 isoform Na,K-ATPase, as we reported previously (Matchkov et al., 2012; Bouzinova et al., 2018). It has been shown previously that Src kinase binds to the Na,K-ATPase α1 isoform in E1 conformation (Ye et al., 2013). Changes in the activity of the α2 isoform Na,K-ATPase can modify localized concentration of Na+, which is important for E1/E2 conformation equilibrium of the α1 isoform (Jorgensen et al., 2003). However, an implication of both isoforms of the Na,K-ATPase-dependent in Src activation and Ca2+ flash generation is also possible. Although further analyses are needed, our results from proximity ligation assay demonstrated that the α2 isoform and Src kinase are localized in a proximity within 40 nm from each other. This is the first demonstration of an interaction between the α2 isoform and Src kinase in the cardiovascular system, although another study reported that both α1 and α2 isoforms interact with Src kinase in skeletal muscle cells (Kotova et al., 2006).
In this study, we inhibited the Na,K-ATPase-dependent Ca2+ flashes with two structurally unrelated tyrosine kinase inhibitors as well as with a specific inhibitor of ouabain-induced Src phosphorylation, pNaKtide (Li et al., 2009). This peptide was synthesized and validated for the specific inhibition of Src signaling from the α1 isoform Na,K-ATPase suggesting that this isoform is implicated in the signaling.
The microtubule network is an important component of the Na,K-ATPase-dependent signaling
We found in this study that disruption of the microtubule network with nocodazole prevented the ouabain-induced Ca2+ flashes. The importance of the microtubule network for the enzymatic activity and signaling of the Na,K-ATPase has been suggested previously. That is, the interaction between the Na,K-ATPase and acetylated tubulin was shown in the rat brain (Alonso et al., 1998; Casale et al., 2003; Arce et al., 2008). Tubulin is the main component of microtubules and when acetylated, it can inhibit the enzymatic activity of Na,K-ATPase. Moreover, disruption of the microtubule network with nocodazole dissociated the complex between tubulin and Na,K-ATPase followed by increased activity in the Na,K-ATPase (Casale et al., 2005). Tubulin is able to interact in a direct manner through cytoplasmic domain 5 of the Na,K-ATPase, suggesting a role for the pump as a microtubule-plasma membrane anchorage site (Zampar et al., 2009; Santander et al., 2019). Mechanical response, i.e., membrane deformation and tension in response to osmotic stress, was suggested to regulate this interaction (Rivelli et al., 2012; Nigra et al., 2016). Moreover, the importance of the microtubule network for Src activation and trafficking was highlighted in different cell types (Abu-Amer et al., 1997; Yamada et al., 2000; Suter et al., 2004). Microtubules were also shown to play a key role in the regulation of steady-state Src activation at the plasma membrane (Wu et al., 2008).
In this study, we show a close proximity localization of the Na,K-ATPase α2 isoform and Src kinase, which was disturbed by nocodazole. Although we have not measured the expression of Src kinase in the presence of nocodazole, the unchanged expression of the Na,K-ATPase suggests that the reduced PLA signal after short incubation with nocodazole is a result of reduced interaction of these proteins. Accordingly, nocodazole decreased Src phosphorylation at resting conditions, and prevented its phosphorylation upon ouabain administration. It has been reported previously that the microtubule network is involved in Src vesicle trafficking, where nocodazole disrupted the trajectory of Src vesicles but did not inhibit their movement (Arnette et al., 2016). Surprisingly, administration of ouabain did not change proximity of the Na,K-ATPase α2 isoform and Src kinase. This may suggest that the proximity of these two proteins is important for initiation of intracellular signaling and Ca2+ flash generation but does not imply large intracellular movement of Src kinase. Although the importance of the microtubules for cell division, differentiation, motility, trafficking and intracellular signaling is accepted, very little is known about the role of microtubule network in vascular smooth muscle cells (Brozovich et al., 2016; Jepps, 2021). The importance of intact microtubule network for interaction between the Na,K-ATPase α2 isoform and Src kinase was validated in both cultured A7r5 cells and freshly isolated vascular smooth muscle cells suggesting the importance of this signaling downstream from the Na,K-ATPase for the regulation of vascular function in vivo. Based on this and previous studies on the Kv7.4 channel trafficking (Lindman et al., 2018; van der Horst et al., 2021), we suggest that the intact microtubule network in vascular smooth muscle cells is a key element to maintain vascular function.
The functional implications of Ca2+ flashes
The transient changes in intracellular Ca2+ induced by micromolar ouabain were shown to be spatially restricted to submembrane regions, whereas no fluctuation in Ca2+ was seen in the center of the cells. In accordance with previous reports (Mulvany et al., 1984; Aalkjaer and Mulvany, 1985; Matchkov et al., 2007; Matchkov et al., 2012; Hangaard et al., 2017), ouabain did not induce global changes in intracellular Ca2+ concentration but elicited spatially restricted Ca2+ transients, i.e., Ca2+ flashes. Spatial and temporal changes in intracellular Ca2+ are critical for vascular smooth muscle cells where Ca2+ has been shown to act as a second messenger and initiate contraction (Wamhoff et al., 2006). Moreover, intracellular Ca2+ transients were shown to modulate a phenotype of vascular smooth muscle cells via regulation of transcription factors (Kudryavtseva et al., 2013). Although, functional significance of the ouabain-induced Ca2+ flashes was beyond the scope of this study (Glavind-Kristensen et al., 2004; Matchkov et al., 2007; Matchkov et al., 2012; Hangaard et al., 2015; Hangaard et al., 2017; Bouzinova et al., 2018; Staehr et al., 2018; Kutz et al., 2021), we can suggest based on previous reports that this spatial and temporal Ca2+ signaling could play an important role in vascular remodeling and contraction, intracellular signaling and smooth muscle cell metabolism. This study adds further complexity in the signaling by showing the key role of microtubule network in the Na,K-ATPase-dependent intracellular signaling mediated by Src kinase.
Data availability statement
The raw data supporting the conclusions of this article will be made available by the authors, without undue reservation.
Ethics statement
The animal study was reviewed and approved by the Animal Experiments Inspectorate of the Danish Ministry of Environment and Food.
Author contributions
SR, IK, SP, CA, TJ, and VM contributed to the conception and design of the study. SR, VK, EB, EM, and VM carried out the experiments. SR, VK, EB, EM, SP, CA, TJ, and VM analyzed and interpreted the data. SR, TJ, and VM prepared the draft and finalized the manuscript. All authors provided critical feedback and approved the final version of the manuscript.
Funding
This work was supported by the Independent Research Fund Denmark—Medical Sciences [9039-00409A] awarded to TJ and [8020-00084B] awarded to VM, Lundbeck Foundation grants [R344-2020-952] awarded to VM and [R323-2018-3674] to TJ, and the Novo Nordisk Foundation [NNF19OC0058460].
Acknowledgments
We thank Jane Holbæk Roenn and Viola Smed Mose Larsen (Aarhus University) for technical assistance with Western blot analysis and cell culture. The work by VK and IK was conducted at Aarhus University, Denmark between 2018–2019.
Conflict of interest
The authors declare that the research was conducted in the absence of any commercial or financial relationships that could be construed as a potential conflict of interest.
Publisher’s note
All claims expressed in this article are solely those of the authors and do not necessarily represent those of their affiliated organizations, or those of the publisher, the editors and the reviewers. Any product that may be evaluated in this article, or claim that may be made by its manufacturer, is not guaranteed or endorsed by the publisher.
References
Aalkjaer C., Mulvany M. J. (1985). Effect of ouabain on tone, membrane potential and sodium efflux compared with [3H]ouabain binding in rat resistance vessels. J. Physiol. 362, 215–231. doi:10.1113/jphysiol.1985.sp015672
Abu-Amer Y., Ross F. P., Schlesinger P., Tondravi M. M., Teitelbaum S. L. (1997). Substrate recognition by osteoclast precursors induces C-src/microtubule association. J. Cell Biol. 137, 247–258. doi:10.1083/jcb.137.1.247
Aizman O., Aperia A. (2003). Na, K-ATPase as a signal transducer. Ann. N. Y. Acad. Sci. 986, 489–496. doi:10.1111/j.1749-6632.2003.tb07233.x
Alonso A. C., Nunez-Fernandez M., Beltramo D. M., Casale C. H., Barra H. S. (1998). Na+, K+-ATPase was found to be the membrane component responsible for the hydrophobic behavior of the brain membrane tubulin. Biochem. Biophys. Res. Commun. 253, 824–827. doi:10.1006/bbrc.1998.9859
Aperia A. (2007). New roles for an old enzyme: Na, K-ATPase emerges as an interesting drug target. J. Intern. Med. 261, 44–52. doi:10.1111/j.1365-2796.2006.01745.x
Arce C. A., Casale C. H., Barra H. S. (2008). Submembraneous microtubule cytoskeleton: Regulation of ATPases by interaction with acetylated tubulin. FEBS J. 275, 4664–4674. doi:10.1111/j.1742-4658.2008.06615.x
Arnette C., Frye K., Kaverina I. (2016). Microtubule and actin interplay drive intracellular c-src trafficking. PLoS One 11, e0148996. doi:10.1371/journal.pone.0148996
Bain J., McLauchlan H., Elliott M., Cohen P. (2003). The specificities of protein kinase inhibitors: An update. Biochem. J. 371, 199–204. doi:10.1042/BJ20021535
Blanco G., DeTomaso A. W., Koster J., Xie Z. J., Mercer R. W. (1994). The alpha-subunit of the Na, K-ATPase has catalytic activity independent of the beta-subunit. J. Biol. Chem. 269, 23420–23425. doi:10.1016/s0021-9258(17)31532-6
Blanco G., Mercer R. W. (1998). Isozymes of the Na-K-ATPase: Heterogeneity in structure, diversity in function. Am. J. Physiol. 275, F633–F650.
Blaustein M. P., Chen L., Hamlyn J. M., Leenen F. H., Lingrel J. B., Wier W. G., et al. (2016). Pivotal role of alpha2 Na+ pumps and their high affinity ouabain binding site in cardiovascular health and disease. J. Physiol. 594, 6079–6103. doi:10.1113/JP272419
Blaustein M. P., Hamlyn J. M. (2020). Ouabain, endogenous ouabain and ouabain-like factors: The Na(+) pump/ouabain receptor, its linkage to NCX, and its myriad functions. Cell Calcium 86, 102159. doi:10.1016/j.ceca.2020.102159
Blaustein M. P., Lederer W. J. (1999). Sodium/calcium exchange: Its physiological implications. Physiol. Rev. 79, 763–854. doi:10.1152/physrev.1999.79.3.763
Bouzinova E. V., Hangaard L., Staehr C., Mazur A., Ferreira A., Chibalin A. V., et al. (2018). The α2 isoform Na, K-ATPase modulates contraction of rat mesenteric small artery via cSrc-dependent Ca2+ sensitization. Acta Physiol. 224, e13059. doi:10.1111/apha.13059
Brozovich F. V., Nicholson C. J., Degen C. V., Gao Y. Z., Aggarwal M., Morgan K. G. (2016). Mechanisms of vascular smooth muscle contraction and the basis for pharmacologic treatment of smooth muscle disorders. Pharmacol. Rev. 68, 476–532. doi:10.1124/pr.115.010652
Casale C. H., Previtali G., Barra H. S. (2003). Involvement of acetylated tubulin in the regulation of Na+, K+ -ATPase activity in cultured astrocytes. FEBS Lett. 534, 115–118. doi:10.1016/s0014-5793(02)03802-4
Casale C. H., Previtali G., Serafino J. J., Arce C. A., Barra H. S. (2005). Regulation of acetylated tubulin/Na+, K+-ATPase interaction by L-glutamate in non-neural cells: Involvement of microtubules. Biochim. Biophys. Acta 1721, 185–192. doi:10.1016/j.bbagen.2004.11.003
Cui X., Xie Z. (2017). Protein interaction and Na/K-ATPase-Mediated signal transduction. Molecules 22, E990. doi:10.3390/molecules22060990
Geering K. (2008). Functional roles of Na,K-ATPase subunits. Curr. Opin. Nephrol. Hypertens. 17, 526–532.
Glavind-Kristensen M., Matchkov V., Hansen V. B., Forman A., Nilsson H., Aalkjaer C. (2004). KATP-channel-induced vasodilation is modulated by the Na, K-pump activity in rabbit coronary small arteries. Br. J. Pharmacol. 143, 872–880. doi:10.1038/sj.bjp.0706016
Gundersen G. G., Cook T. A. (1999). Microtubules and signal transduction. Curr. Opin. Cell Biol. 11, 81–94. doi:10.1016/s0955-0674(99)80010-6
Haas M., Wang H., Tian J., Xie Z. (2002). Src-mediated inter-receptor cross-talk between the Na+/K+-ATPase and the epidermal growth factor receptor relays the signal from ouabain to mitogen-activated protein kinases. J. Biol. Chem. 277, 18694–18702. doi:10.1074/jbc.M111357200
Hangaard L., Bouzinova E. V., Staehr C., Dam V. S., Kim S., Xie Z., et al. (2017). Na, K-ATPase regulates intercellular communication in the vascular wall via cSrc kinase dependent connexin43 phosphorylation. Am. J. Physiol. Cell Physiol. 312, C385–C397. doi:10.1152/ajpcell.00347.2016
Hangaard L., Jessen P. B., Kamaev D., Aalkjaer C., Matchkov V. V. (2015). Extracellular calcium-dependent modulation of endothelium relaxation in rat mesenteric small artery: The role of potassium signaling. Biomed. Res. Int. 2015, 758346. doi:10.1155/2015/758346
Holmgren M., Wagg J., Bezanilla F., Rakowski R. F., De Weer P., Gadsby D. C. (2000). Three distinct and sequential steps in the release of sodium ions by the Na+/K+-ATPase. Nature 403, 898–901. doi:10.1038/35002599
Jepps T. A., Carr G., Lundegaard P. R., Olesen S. P., Greenwood I. A. (2015). Fundamental role for the KCNE4 ancillary subunit in Kv7.4 regulation of arterial tone. J. Physiol. 593, 5325–5340. doi:10.1113/JP271286
Jepps T. A. (2021). Kv7 channel trafficking by the microtubule network in vascular smooth muscle. Acta Physiol. 232, e13692. doi:10.1111/apha.13692
Jorgensen P. L., Hakansson K. O., Karlish S. J. (2003). Structure and mechanism of Na, K-ATPase: Functional sites and their interactions. Annu. Rev. Physiol. 65, 817–849. doi:10.1146/annurev.physiol.65.092101.142558
Kotova O., Al-Khalili L., Talia S., Hooke C., Fedorova O. V., Bagrov A. Y., et al. (2006). Cardiotonic steroids stimulate glycogen synthesis in human skeletal muscle cells via a Src- and ERK1/2-dependent mechanism. J. Biol. Chem. 281, 20085–20094. doi:10.1074/jbc.M601577200
Kudryavtseva O., Aalkjaer C., Matchkov V. V. (2013). Vascular smooth muscle cell phenotype is defined by Ca(2+) -dependent transcription factors. FEBS J. 280, 5488–5499. doi:10.1111/febs.12414
Kutz L. C., Cui X., Xie J. X., Mukherji S. T., Terrell K. C., Huang M., et al. (2021). The Na/K-ATPase α1/Src interaction regulates metabolic reserve and Western diet intolerance. Acta Physiol. 232, e13652. doi:10.1111/apha.13652
Li Z., Cai T., Tian J., Xie J. X., Zhao X., Liu L., et al. (2009). NaKtide, a Na/K-ATPase-derived peptide Src inhibitor, antagonizes ouabain-activated signal transduction in cultured cells. J. Biol. Chem. 284, 21066–21076. doi:10.1074/jbc.M109.013821
Li Z., Xie Z. (2009). The Na/K-ATPase/Src complex and cardiotonic steroid-activated protein kinase cascades. Pflugers Arch. 457, 635–644. doi:10.1007/s00424-008-0470-0
Lindman J., Khammy M. M., Lundegaard P. R., Aalkjaer C., Jepps T. A. (2018). Microtubule regulation of Kv7 channels orchestrates cAMP-mediated vasorelaxations in rat arterial smooth muscle. Hypertension 71, 336–345. doi:10.1161/HYPERTENSIONAHA.117.10152
Matchkov V. V., Gustafsson H., Rahman A., Boedtkjer D. M., Gorintin S., Hansen A. K., et al. (2007). Interaction between Na+/K+-pump and Na+/Ca2+-exchanger modulates intercellular communication. Circ. Res. 100, 1026–1035. doi:10.1161/01.RES.0000262659.09293.56
Matchkov V. V., Krivoi I. I. (2016). Specialized functional diversity and interactions of the Na, K-ATPase. Front. Physiol. 7, 179. doi:10.3389/fphys.2016.00179
Matchkov V. V., Larsen P., Bouzinova E. V., Rojek A., Boedtkjer D. M., Golubinskaya V., et al. (2008). Bestrophin-3 (vitelliform macular dystrophy 2-like 3 protein) is essential for the cGMP-dependent calcium-activated chloride conductance in vascular smooth muscle cells. Circ. Res. 103, 864–872. doi:10.1161/CIRCRESAHA.108.178517
Matchkov V. V. (2010). Mechanisms of cellular synchronization in the vascular wall. Mechanisms of vasomotion. Dan. Med. Bull. 57, B4191.
Matchkov V. V., Moeller-Nielsen N., Secher D., Dam V., Nourian Z., Bodtkjer D. M., et al. (2012). The alpha2 isoform of the Na, K-pump is important for intercellular communication, agonist-induced contraction and EDHF-like response in rat mesenteric arteries. Am. J. Physiol. 303, H36–H46.
Mulvany M. J., Aalkjaer C., Petersen T. T. (1984). Intracellular sodium, membrane potential, and contractility of rat mesenteric small arteries. Circ. Res. 54, 740–749. doi:10.1161/01.res.54.6.740
Mulvany M. J., Nilsson H., Flatman J. A., Korsgaard N. (1982). Potentiating and depressive effects of ouabain and potassium-free solutions on rat mesenteric resistance vessels. Circ. Res. 51, 514–524. doi:10.1161/01.res.51.4.514
Nigra A. D., Monesterolo N. E., Rivelli J. F., Amaiden M. R., Campetelli A. N., Casale C. H., et al. (2016). Alterations of hemorheological parameters and tubulin content in erythrocytes from diabetic subjects. Int. J. Biochem. Cell Biol. 74, 109–120. doi:10.1016/j.biocel.2016.02.016
Rivelli J. F., Amaiden M. R., Monesterolo N. E., Previtali G., Santander V. S., Fernandez A., et al. (2012). High glucose levels induce inhibition of Na, K-ATPase via stimulation of aldose reductase, formation of microtubules and formation of an acetylated tubulin/Na, K-ATPase complex. Int. J. Biochem. Cell Biol. 44, 1203–1213. doi:10.1016/j.biocel.2012.04.011
Santander V. S., Campetelli A. N., Monesterolo N. E., Rivelli J. F., Nigra A. D., Arce C. A., et al. (2019). Tubulin-Na(+) , K (+) -ATPase interaction: Involvement in enzymatic regulation and cellular function. J. Cell. Physiol. 234, 7752–7763. doi:10.1002/jcp.27610
Song H., Karashima E., Hamlyn J. M., Blaustein M. P. (2014). Ouabain-digoxin antagonism in rat arteries and neurones. J. Physiol. 592, 941–969. doi:10.1113/jphysiol.2013.266866
Staehr C., Hangaard L., Bouzinova E. V., Kim S., Rajanathan R., Boegh Jessen P., et al. (2018). Smooth muscle Ca(2+) sensitization causes hypercontractility of middle cerebral arteries in mice bearing the familial hemiplegic migraine type 2 associated mutation. J. Cereb. Blood Flow. Metab. 39, 1570–1587. doi:10.1177/0271678X18761712
Suter D. M., Schaefer A. W., Forscher P. (2004). Microtubule dynamics are necessary for SRC family kinase-dependent growth cone steering. Curr. Biol. 14, 1194–1199. doi:10.1016/j.cub.2004.06.049
Tian J., Cai T., Yuan Z., Wang H., Liu L., Haas M., et al. (2006). Binding of Src to Na+/K+-ATPase forms a functional signaling complex. Mol. Biol. Cell 17, 317–326. doi:10.1091/mbc.e05-08-0735
van der Horst J., Rognant S., Abbott G. W., Ozhathil L. C., Hagglund P., Barrese V., et al. (2021). Dynein regulates Kv7.4 channel trafficking from the cell membrane. J. Gen. Physiol. 153, e202012760. doi:10.1085/jgp.202012760
Wamhoff B. R., Bowles D. K., Owens G. K. (2006). Excitation-transcription coupling in arterial smooth muscle. Circ. Res. 98, 868–878. doi:10.1161/01.RES.0000216596.73005.3c
Weigand K. M., Swarts H. G., Fedosova N. U., Russel F. G., Koenderink J. B. (2012). Na, K-ATPase activity modulates src activation: A role for ATP/ADP ratio. Biochim. Biophys. Acta 1818, 1269–1273. doi:10.1016/j.bbamem.2012.01.015
Wu B., Decourt B., Zabidi M. A., Wuethrich L. T., Kim W. H., Zhou Z., et al. (2008). Microtubule-mediated Src tyrosine kinase trafficking in neuronal growth cones. Mol. Biol. Cell 19, 4611–4627. doi:10.1091/mbc.e08-06-0603
Xie J., Ye Q., Cui X., Madan N., Yi Q., Pierre S. V., et al. (2015). Expression of rat Na-K-ATPase α2 enables ion pumping but not ouabain-induced signaling in α1-deficient porcine renal epithelial cells. Am. J. Physiol. Cell Physiol. 309, C373–C382. doi:10.1152/ajpcell.00103.2015
Xie Z. (2003). Molecular mechanisms of Na/K-ATPase-mediated signal transduction. Ann. N. Y. Acad. Sci. 986, 497497–503503. doi:10.1111/j.1749-6632.2003.tb07234.x
Yamada T., Aoyama Y., Owada M. K., Kawakatsu H., Kitajima Y. (2000). Scraped-wounding causes activation and association of C-Src tyrosine kinase with microtubules in cultured keratinocytes. Cell Struct. Funct. 25, 351–359. doi:10.1247/csf.25.351
Ye Q., Lai F., Banerjee M., Duan Q., Li Z., Si S., et al. (2013). Expression of mutant α1 Na/K-ATPase defective in conformational transition attenuates Src-mediated signal transduction. J. Biol. Chem. 288, 5803–5814. doi:10.1074/jbc.M112.442608
Yosef E., Katz A., Peleg Y., Mehlman T., Karlish S. J. (2016). Do src kinase and caveolin interact directly with Na, K-ATPase? J. Biol. Chem. 291, 11736–11750. doi:10.1074/jbc.M116.721084
Yu H., Cui X., Zhang J., Xie J. X., Banerjee M., Pierre S. V., et al. (2018). Heterogeneity of signal transduction by Na-K-ATPase alpha-isoforms: Role of src interaction. Am. J. Physiol. Cell Physiol. 314, C202–C210. doi:10.1152/ajpcell.00124.2017
Yuan C. M., Manunta P., Hamlyn J. M., Chen S., Bohen E., Yeun J., et al. (1993). Long-term ouabain administration produces hypertension in rats. Hypertension 22, 178–187. doi:10.1161/01.hyp.22.2.178
Zampar G. G., Chesta M. E., Carbajal A., Chanaday N. L., Diaz N. M., Casale C. H., et al. (2009). Acetylated tubulin associates with the fifth cytoplasmic domain of Na(+)/K(+)-ATPase: Possible anchorage site of microtubules to the plasma membrane. Biochem. J. 422, 129–137. doi:10.1042/BJ20082410
Zhang J., Lee M. Y., Cavalli M., Chen L., Berra-Romani R., Balke C. W., et al. (2005). Sodium pump alpha2 subunits control myogenic tone and blood pressure in mice. J. Physiol. 569, 243–256. doi:10.1113/jphysiol.2005.091801
Zhang L., Aalkjaer C., Matchkov V. V. (2018). The Na, K-ATPase-Dependent src kinase signaling changes with mesenteric artery diameter. Int. J. Mol. Sci. 19, 2489. doi:10.3390/ijms19092489
Zhang L., Staehr C., Zheng F., Bouzinova E. V., Matchkov V. V. (2019). The Na,K-ATPase in vascular smooth muscle cells. Curr. Top. Membr. 83, 151–175. doi:10.1016/bs.ctm.2019.01.007
Zulian A., Linde C. I., Pulina M. V., Baryshnikov S. G., Papparella I., Hamlyn J. M., et al. (2013). Activation of c-SRC underlies the differential effects of ouabain and digoxin on Ca2+ signaling in arterial smooth muscle cells. Am. J. Physiol. Cell Physiol. 304, C324–C333. doi:10.1152/ajpcell.00337.2012
Keywords: Na,K-ATPase, Src kinase, intracellular Ca2+ signaling, microtubule network, Ca2+ flashes
Citation: Rognant S, Kravtsova VV, Bouzinova EV, Melnikova EV, Krivoi II, Pierre SV, Aalkjaer C, Jepps TA and Matchkov VV (2022) The microtubule network enables Src kinase interaction with the Na,K-ATPase to generate Ca2+ flashes in smooth muscle cells. Front. Physiol. 13:1007340. doi: 10.3389/fphys.2022.1007340
Received: 30 July 2022; Accepted: 05 September 2022;
Published: 23 September 2022.
Edited by:
M. Teresa Perez-Garcia, University of Valladolid, SpainReviewed by:
Manuel F. Navedo, University of California, Davis, United StatesLuis A. Martinez-Lemus, University of Missouri, United States
Copyright © 2022 Rognant, Kravtsova, Bouzinova, Melnikova, Krivoi, Pierre, Aalkjaer, Jepps and Matchkov. This is an open-access article distributed under the terms of the Creative Commons Attribution License (CC BY). The use, distribution or reproduction in other forums is permitted, provided the original author(s) and the copyright owner(s) are credited and that the original publication in this journal is cited, in accordance with accepted academic practice. No use, distribution or reproduction is permitted which does not comply with these terms.
*Correspondence: Vladimir V. Matchkov, dnZtQGJpb21lZC5hdS5kaw==